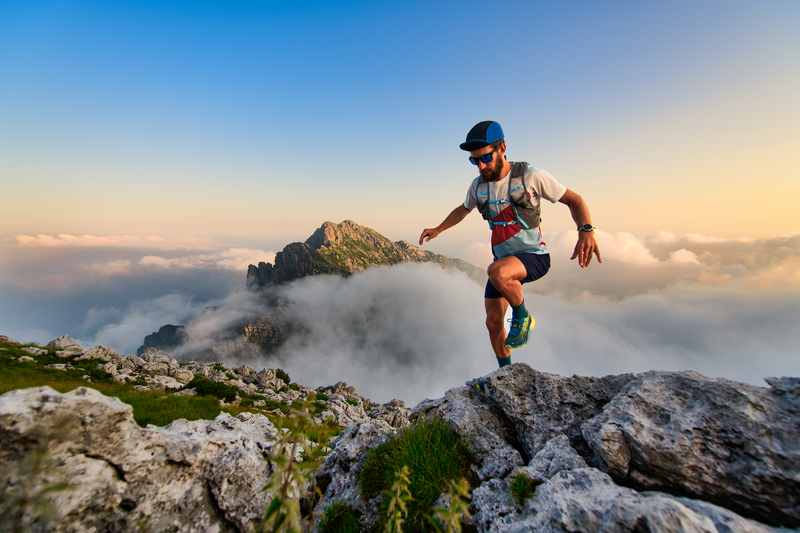
95% of researchers rate our articles as excellent or good
Learn more about the work of our research integrity team to safeguard the quality of each article we publish.
Find out more
HYPOTHESIS AND THEORY article
Front. Microbiol. , 15 April 2020
Sec. Systems Microbiology
Volume 11 - 2020 | https://doi.org/10.3389/fmicb.2020.00589
This article is part of the Research Topic Gut Microbiome Modulation in Ruminants: Enhancing Advantages and Minimizing Drawbacks View all 31 articles
Rumen fermentation affects ruminants productivity and the environmental impact of ruminant production. The release to the atmosphere of methane produced in the rumen is a loss of energy and a cause of climate change, and the profile of volatile fatty acids produced in the rumen affects the post-absorptive metabolism of the host animal. Rumen fermentation is shaped by intracellular and intercellular flows of metabolic hydrogen centered on the production, interspecies transfer, and incorporation of dihydrogen into competing pathways. Factors that affect the growth of methanogens and the rate of feed fermentation impact dihydrogen concentration in the rumen, which in turn controls the balance between pathways that produce and incorporate metabolic hydrogen, determining methane production and the profile of volatile fatty acids. A basic kinetic model of competition for dihydrogen is presented, and possibilities for intervention to redirect metabolic hydrogen from methanogenesis toward alternative useful electron sinks are discussed. The flows of metabolic hydrogen toward nutritionally beneficial sinks could be enhanced by adding to the rumen fermentation electron acceptors or direct fed microbials. It is proposed to screen hydrogenotrophs for dihydrogen thresholds and affinities, as well as identifying and studying microorganisms that produce and utilize intercellular electron carriers other than dihydrogen. These approaches can allow identifying potential microbial additives to compete with methanogens for metabolic hydrogen. The combination of adequate microbial additives or electron acceptors with inhibitors of methanogenesis can be effective approaches to decrease methane production and simultaneously redirect metabolic hydrogen toward end products of fermentation with a nutritional value for the host animal. The design of strategies to redirect metabolic hydrogen from methane to other sinks should be based on knowledge of the physicochemical control of rumen fermentation pathways. The application of new –omics techniques together with classical biochemistry methods and mechanistic modeling can lead to exciting developments in the understanding and manipulation of the flows of metabolic hydrogen in rumen fermentation.
The complex microbial community that inhabits the rumen allows ruminants to digest and transform fibrous carbohydrates unavailable to humans into useful products such as meat, milk, wool and traction. Critical to the symbiosis between the rumen microbiota and the host animal is the anaerobic condition of the rumen, which prevents the complete oxidation of carbohydrates to carbon dioxide (CO2) and water. Instead, carbohydrates are incompletely oxidized to volatile fatty acids (VFA) and gases, with the host animal absorbing and utilizing the VFA as sources and precursors of energy, fat, glucose, and non-essential amino acids (Armstrong and Blaxter, 1957).
Rumen fermentation not only provides the ruminant with VFA. Part of the negative Gibbs energy change (ΔG) associated with fermentation is used by rumen microbes to generate ATP that can be utilized for microbial growth, active transport of substrates, and motility. Microbial growth produces microbial protein, which is the principal (Wallace et al., 1997) and most economical source of amino acids for ruminants. Rumen microorganisms can also synthesize water-soluble vitamins, which thus do not need to be included in most ruminants diets (Weiss, 2017).
A product of rumen fermentation is methane (CH4), which is a potent greenhouse gas when released to the atmosphere, and also a loss of energy for ruminants (Eckard et al., 2010; Martin et al., 2010). Through the formation of CH4 and the profile of VFA produced, rumen fermentation has important consequences for animal productivity and the environment. Understanding how rumen fermentation is controlled can help designing strategies to manipulate it in desired directions. Central to rumen metabolism are the dynamics of metabolic hydrogen ([H]) production and utilization. The idea of understanding rumen energy metabolism as [H] flows through different biochemical pathways is not new (Czerkawski, 1986; Hegarty and Gerdes, 1999). The objectives of this paper are to review and critically examine [H] flows as the unifying principle to understand rumen fermentation. In particular, this paper will discuss (i) The control of the VFA profile and CH4 production by dihydrogen (H2), (ii) The principles underlying the competition for H2, (iii) The potential inhibitory effects of H2 and other intercellular electron (e–) carriers on the rates of fermentation and digestion in the rumen, and (iv) The relationship between the flows of [H] and microbial growth. All of these aspects have implications to animal productivity and the environment mediated by ruminal and post-absorptive metabolism.
The terms in the following list are in some cases defined bearing in mind their main significance with regard to rumen energy metabolism, acknowledging that other aspects might be more important in other areas of science.
Electron (e–) – a negatively charged sub-atomic particle.
Redox reaction – a chemical reaction involving an exchange of one or more e– between two chemical compounds, an e– donor and an e– acceptor.
Reducing potential (Eh) – a measure of the tendency of a chemical compound or a system to donate e– under certain defined conditions.
Proton (p+ or H+) – a positively charged sub-atomic particle released by acids in aqueous solutions.
Metabolic hydrogen ([H]) – the sum of all hydrogen atoms that can be exchanged between molecules in a living cell or a microbial ecosystem, or any other defined living system.
Redox cofactors – intracellular molecules that act as e– acceptors and donors in redox reactions to transfer e– between metabolic intermediates. Redox cofactors have, therefore, different oxidation stages, for example: reduced and oxidized nicotinamide adenine dinucleotide (NADH and NAD+, respectively), reduced and oxidized ferredoxin (Fdred2– and Fdox, respectively), reduced, semi-reduced and oxidized flavin mononucleotide (FMNH2, FMNH and FMN, respectively), reduced and oxidized flavin adenine dinucleotide (FADH2 and FAD, respectively), and reduced and oxidized tocopherols. Some cofactors accept and donate pairs of e– (e.g., ferredoxin), others accept and donate pairs of hydrogen atoms (H = 1 e– + 1 H+, e.g., FAD), or one hydrogen atom (e.g., tocopherols). The NADH/NAD+ pair accepts and donates two e– per H+: NAD+ + [2H] ↔ NADH + H+, with [2H] = 2 H+ + 2 e–.
Fermentation – an incomplete oxidation in which the ultimate e– acceptors are carbon compounds produced in the process itself.
Metabolic hydrogen production (or release) – the transfer of [H] from e– donors that are metabolic intermediates to oxidized intracellular cofactors.
Metabolic hydrogen incorporation – the transfer of [H] from reduced intracellular cofactors to e– acceptors intermediate in metabolism.
Metabolic hydrogen sink (or electron sink) – reduced end product of fermentation whose pathway of formation involves reactions that incorporate [H]. Note that some [H] sinks are not net [H] sinks in the sense that their production involves greater [H] production than incorporation, e.g., the production of butyrate from hexoses.
Reducing equivalents pairs ([2H]) – a pair of hydrogen atoms, or a mole of hydrogen atom pairs, produced or incorporated in a metabolic reaction or pathway. This concept is used to quantify [H] transactions as [2H] production and [2H] incorporation.
Hydrogenases – enzymes that catalyze: (i) The formation of H2 from e– donated by reduced intracellular cofactors (H2-evolving hydrogenases), and/or (ii) The reduction of an intracellular cofactor by H2 (H2-incorporating hydrogenases).
Interspecies hydrogen transfer – process in which H2 produced and released by a microbial cell is incorporated by another microbial cell. Interspecies H2 transfer decreases H2 concentration in the proximity of the H2-producing cell, thermodynamically favoring H2 production.
Intercellular electron carriers – reduced compounds intermediate of fermentation pathways that incorporate [H] in their formation, and are released by some microbial cells and taken up by others e.g., H2, formate, ethanol, lactate and succinate.
Substrate level phosphorylation – generation of ATP in which a phosphate group is donated by a phosphorylated organic compound to phosphorylate ADP to ATP.
Electron transport-linked phosphorylation (ETLP) – generation of ATP from ADP and phosphate driven by a transmembrane electrochemical gradient of H+ or sodium cations. The electrochemical gradient is created by the extrusion of H+ or sodium cations of the microbial cell, which is in turn coupled to an intracellular redox reaction.
Living organisms can generate energy for anabolic functions through thermodynamically favorable flows of [H]. In anaerobic environments such as the rumen, the main e– acceptors are carbon compounds generated in the fermentation process itself, with methanogenesis being the most important [H] disposal pathway. The main pathways of carbohydrate fermentation in the rumen have been reviewed and investigated by Russell and Wallace (1997), Russell (2002), and Hackmann et al. (2017), and the reader is referred to those scientific papers for detailed information. The focus in this subsection will be on reactions central to [H] transfer, and their implications with regard to the balance of [2H] production and incorporation of each fermentation pathway.
It is understood that the mixed rumen microbiota metabolizes over 90% of hexoses (in turn released from the hydrolysis of complex carbohydrates such as cellulose and starch) to pyruvate through the glycolytic pathway. Pyruvate (and also phosphoenolpyruvate in propionate production) is a central branching point at which the different pathways leading to the formation of the three main VFA, acetate, propionate and butyrate, diverge (Russell and Wallace, 1997; Figure 1). Recently, Hackmann et al. (2017) examined complete genomes of various rumen bacteria that have been isolated and grown in pure culture, and found that several bacterial species encoded for incomplete glycolytic pathways as well as several alternative pathways of carbohydrate metabolism, such as the Bifidobacterium pathway.
Hemicelluloses are also abundant as plant structural carbohydrates, and are rich in pentoses such as xylose and arabinose (Scheller and Ulvskov, 2010). In the rumen, pentoses are metabolized through the pentose cycle and to a lesser extent through the transketolase cleavage (Russell and Wallace, 1997). This results in the production of glyceraldehyde-3-phosphate and fructose 6-phosphate, which can enter glycolysis, of acetyl-phosphate, which can be converted to acetate, and of ribose 5-phosphate, which can be used to synthesize nucleotides and histidine (Voet and Voet, 1995; Figure 1).
Glycolysis involves the oxidation of glyceraldehyde-3-phosphate to 1,3-biphosphoglycerate coupled to the reduction of NAD+ to NADH (Voet and Voet, 1995). Metabolic hydrogen is also produced in the first step of acetate and butyrate production, the oxidative decarboxylation of pyruvate to acetyl-CoA (Figure 2). Depending on the hydrogenase catalyzing pyruvate decarboxylation, [H] can reduce Fdox to Fdred2, or CO2 to formate (Russell and Wallace, 1997; Hegarty and Gerdes, 1999; Russell, 2002). Ferredoxins (see section “Definitions”) are iron sulfur proteins that act as e– carriers through the reduction of one iron atom per iron sulfur cluster: 2 Fe2+ + 2 Fe3+ (oxidized form) + e– → 3 Fe2+ + Fe3+ (reduced form) (Gottschalk, 1986).
Figure 2. Main reactions releasing (orange rectangle) and incorporating (green rectangle) metabolic hydrogen ([H]), as connected by intracellular (sky blue rectangle) and intercellular (salmon rectangle) metabolic hydrogen transactions. The stoichiometry of production and incorporation of reducing equivalents is not depicted.
For metabolism of carbohydrates to continue, reduced cofactors need to be re-oxidized (Wolin et al., 1997). Hydrogenases transfer e– from reduced cofactors to H+, forming H2 (Frei, 2013; Figure 2). Dihydrogen does not accumulate in the rumen, as it is transferred from the rumen consortium of bacteria, protozoa and fungi to methanogens (Janssen, 2010). Hydrogenases also catalyze the uptake and incorporation of H2 by methanogens and other hydrogenotrophs (Frey, 2002; Søndergaard et al., 2016). Apart from H2, rumen methanogens and other hydrogenotrophs can also use as [H] donors other intercellular e– carriers such as formate, methanol, ethanol and methylamines (Asanuma et al., 1999; St-Pierre et al., 2015; Patra et al., 2017).
Electrons in reduced cofactors or in H2 or formate can also be disposed through their incorporation into pathways other than methanogenesis (Figure 2). In the randomizing pathway of propionate formation (so called because carbon labeled in position 2 of pyruvate is randomized to positions 2 and 3 of succinate), [H] donated by H2, formate, NADH or lactate is incorporated in the reductions of oxaloacetate to malate and fumarate to succinate (Henderson, 1980; Gottschalk, 1986; Russell and Wallace, 1997; Asanuma et al., 1999). The reduction of fumarate to succinate is coupled to ETLP (De Vries et al., 1973; Gottschalk, 1986; Kröger et al., 2002). Succinate can be metabolized to propionate by the succinate producer itself or it can be transferred to succinate utilizers as an intercellular e– carrier.
Lactate is an intermediate in the non-randomizing pathway of propionate formation (so called because carbon labeled in position 2 in pyruvate appears in position 2 in propionate). Lactate is formed from the reduction of pyruvate with [H] donated by NADH. Lactate can be intracellularly activated to lactyl-CoA, which is then dehydrated to acrylyl-CoA. Acrylyl-CoA is then reduced to propionyl-CoA with reduced flavoprotein (Gottschalk, 1986) or NADH (Hackmann et al., 2017) as [H] donor, in a reaction that has not been found to be coupled with ATP generation through ETLP (Thauer et al., 1977; Seeliger et al., 2002). Lactate can also be excreted and taken up by other microbial cells that convert it to acetate, propionate or butyrate (Chen et al., 2019).
The conversion of two molecules of acetyl-CoA to butyrate also involves two [H]-incorporating steps, with NADH as the reductant in the conversion of acetoacetyl-CoA to β-hydroxybutyryl-CoA and of crotonyl-CoA to butyryl-CoA. In the reduction of crotonyl-CoA to butyryl-CoA, Fdox is simultaneously reduced by a second molecule of NADH to Fdred2– in a process called electron bifurcation (Buckel and Thauer, 2013; Figure 3A; see also section “The Role of Dihydrogen as an Intercellular Electron Carrier”). The oxidation of Fdred2– so formed by electron bifurcation can result in H+ extrusion and ATP generation by ETLP (Hackmann and Firkins, 2015; Hackmann et al., 2017).
Figure 3. Examples of (A) Electron bifurcation and (B) Electron confurcation redox reactions. Electron bifurcation in the reduction of Fdox by NADH coupled to the reduction of crotonyl-CoA to butyryl-CoA catalyzed by Bcd-Etf was proposed by Hackmann and Firkins (2015) to operate in rumen butyrivibrios (genera Butyrivibrio and Pseudobutyrivibrio). In the rumen bacterium R. albus the electron-bifurcating hydrogenase HydABC catalyzes the formation of dihydrogen (H2) from NADH and reduced ferredoxin (Fdred2–) (Zheng et al., 2014; Buckel and Thauer, 2018b).
Dihydrogen has a central role in the flows of [H] in the rumen. Genes encoding hydrogenases are widespread in the genomes of rumen bacteria and archaea, highlighting that an important proportion of [H] is transferred and incorporated between cells as H2 (Greening et al., 2019). This agrees with the historical finding by Hungate (1967) of H2 being the main [H] donor for CH4 formation in rumen fermentation.
Interspecies H2 transfer thermodynamically favors the re-oxidation of intracellular cofactors as the H2-consuming microorganisms help decreasing H2 concentration (Wolin et al., 1997; Lubner and Peters, 2017). In the presence of methanogens, or propionate or succinate producers, H2 producers shifted fermentation away from formate, lactate and ethanol toward acetate, and cellulose digestion increased. Production of H2 was stimulated as H2 accumulation was relieved in the presence of methanogens or other hydrogenotrophs (Chen and Wolin, 1977; Bauchop and Mountfort, 1981; Marvin-Sikkema et al., 1990; Wolin et al., 1997).
The participation of ferredoxins is fundamental in H2 formation and incorporation. Ferredoxins have very low standard reducing potentials comparable to the H2/2H+ couple, which allows them to donate e– to a hydrogenase to reduce H+ to H2 (Gottschalk, 1986). In microbial cells ferredoxins are typically more than 90% reduced, which makes them strong e– donors. Reduction of Fdox is thus thermodynamically very unfavorable, and it occurs through flavin-based electron bifurcation, with the donation of e– to Fdox coupled to a stoichiometrical donation of e– by the same e– donor to a strong e– acceptor. In this way Fdox can be reduced by NADH (Figure 3A) or by H2 in bacteria and archaea. In turn, the re-oxidation of Fdred2– can be coupled to energy conservation through the generation of a transmembrane electrochemical gradient. Some of the coupled reductions that can drive the reduction of Fdox are crotonyl-CoA to butyryl-CoA with NADH as the e– donor, NAD+ to NADH with H2 or NADPH as e– donors, NADP+ to NADPH with H2 as e– donor, pyruvate to lactate with NADH as e– donor, and CO2 to formate with NADPH as e– donor. In methanogens, the high potential e– acceptor involved in electron bifurcation in the last step of methanogenesis reaction is the CoM-S-S-CoB heterodisulfide that is split into HS-CoM and HS-CoB by the e– donor F420H2 (reduced 8-hydroxy-5-deazaflavin). The reverse reaction of bifurcation, in which H2 can be produced, is called confurcation (Figure 3B; Nitschke and Russell, 2012; Buckel and Thauer, 2013, 2018a,b).
Genes encoding confurcating hydrogenases that oxidize NADH and Fdred2– to H2 were the most abundant hydrogenases-encoding genes from 501 rumen bacteria genomes from the Hungate culture collection and others. Furthermore, confurcating hydrogenases were the most abundant hydrogenase transcripts in sheep rumens, which shows the importance of this relatively recently discovered e– transfer mechanism in rumen fermentation (Greening et al., 2019). The rumen bacterium Ruminococcus albus, for example, can produce H2 from NADH in confurcation with the oxidation of Fdred2– (Zheng et al., 2014). Greening et al. (2019) found that the expression in R. albus of an 8-gene cluster encoding for an alcohol and aldehyde dehydrogenase involved in the production of ethanol, a ferredoxin H2-evolving hydrogenase, and a sensory hydrogenase, were sharply decreased in co-culture with the H2-utilizer Wolinella succinogenes in comparison to the mono-culture. In the co-culture, NADH was re-oxidized only through confurcation with Fdred2–, as NADH was not utilized as reductant for ethanol production.
The [H]-producing and -incorporating reactions in the different fermentation pathways result in different stoichiometries of [2H] production and incorporation per mole of VFA produced, which can be used to calculate [2H] balances (Marty and Demeyer, 1973). Acetate, and to a lesser extent, butyrate production from glucose, are associated with the net production of [2H]. On the other hand, propionate production implies a net incorporation of [2H]. Thus, propionate competes with CH4 as a [H] sink in rumen fermentation whereas acetate and butyrate formation release [H] that can be utilized by methanogens to reduce CO2 to CH4 (Janssen, 2010). The formation of CH4 in rumen fermentation is thus closely associated to the profile of VFA formed.
In vitro balances of [2H] production and incorporation show that CH4 is the main sink of [H] in the rumen fermentation with functional methanogenesis (Ungerfeld, 2015b). Strictly speaking, it is unknown if CH4 is the main [H] sink in the live animal, because there are no in vivo experimental reports in which the production of both VFA and gases has been simultaneously measured. However, estimations based on the Cabezas-Garcia et al. (2017) meta-analysis suggest that CH4 is also almost surely the most important [H] sink in rumen fermentation in dairy cows on mixed diets (calculation not shown). Furthermore, the importance of CH4 as [H] sink agrees with the abundance in the rumen of sheep of archaeal hydrogenases and reductases (Snelling and Wallace, 2017) and their transcripts (Greening et al., 2019).
The recent discovery that many rumen bacteria encode an incomplete glycolytic pathways and alternative pathways of hexose metabolism (Hackmann et al., 2017) can potentially add complexity and unknown stoichiometries of [2H] production and incorporation associated to the production of each VFA. The formation of glyceraldehyde-3-phosphate in the oxidative pentose phosphate pathway would greatly augment [2H] produced in the conversion of glucose to pyruvate from 2 to 14 [2H] mole/mole glucose. Conversely, acetate production through the Bifidobacterium pathway is not associated to [2H] production or incorporation, in contrast to 4 × [2H] mole/mole glucose if produced through glycolysis or the phosphoketolase pathway. The flows of carbon through different pathways depend on the genetic makeup of the rumen microbial community (i.e., pathways encoded), the abundance of the different microbial populations encoding each pathway, gene expression in the different microorganisms, enzyme and substrate kinetics, and thermodynamic feasibility of reactions.
The presence of genes and transcripts of hydrogenases catalyzing H2 uptake in nitrate and sulfate reduction and reductive acetogenesis, has been reported in sheep rumens (Greening et al., 2019). The reduction of nitrate and sulfate thermodynamically outcompetes methanogenesis (Ungerfeld and Kohn, 2006). However, the concentration of these e– acceptors in the rumen usually limits the rate of [H] incorporation in their reduction, unless they are supplemented to the diet as salts (Van Zijderveld et al., 2010). Nitrate can also be naturally present at high contents in grasses, which can lead to toxicity caused by the absorption and passage to blood of its reduction intermediate nitrite (McKenzie et al., 2004). Reductive acetogenesis, the reduction of CO2 with H2 to acetate, seems to be thermodynamically outcompeted by methanogenesis in the rumen (Kohn and Boston, 2000), although it is functional or even predominates over methanogenesis in the gastrointestinal tract of some termites, cockroaches, kangaroos, pre-ruminant lambs, rodents, pigs, and some humans (Joblin, 1999; Gagen et al., 2012; Klieve et al., 2012). Some reductive acetogens inhabit the rumen, but as they are not obligate hydrogenotrophs it is possible that they survive mainly by metabolizing carbohydrates (Joblin, 1999). That said, a recent study found that reductive acetogenesis was a minor, but not insignificant, [H] sink in the rumen (Raju, 2016). The presence of genes (Denman et al., 2015) and transcripts (Greening et al., 2019) of hydrogenases involved in reductive acetogenesis has also been reported.
The profile of products formed in rumen fermentation has implications for animal productivity and the environment. Despite its importance as the main [H] sink in rumen fermentation, the release of CH4 to the atmosphere represents an energy loss ranging between 2 and 12% of ingested gross energy (Johnson and Johnson, 1995) and was identified early on in ruminant nutrition research as an energy inefficiency in rumen fermentation and an opportunity to improve animal productivity (Czerkawski and Breckenridge, 1975a; Davies et al., 1982). More recently, increasing concerns about climate change have raised interest in the abatement of CH4 emissions from ruminants. Emissions of enteric CH4 are estimated to account for about 6% of total anthropogenic emissions of greenhouse gases expressed as CO2-eq i.e., the sum of the emissions of each greenhouse gas weighted by its global warming potential (Gerber et al., 2013).
The profile of VFA absorbed from the rumen has also consequences on the host animal’s post-absorptive metabolism (Ungerfeld, 2013). Inhibiting methanogenesis can shift fermentation toward propionate production (Janssen, 2010), which is the main glucose precursor in ruminants (Aschenbach et al., 2010). Increased propionate production can be important to animals with high requirements for glucose such as high producing dairy cows in early lactation. On the other hand, propionate is a satiety signal in ruminants and can decrease feed intake (Allen et al., 2009) and milk fat content (Maxin et al., 2011). In turn, an increased supply of acetate increases milk fat percentage (Sheperd and Combs, 1998; Maxin et al., 2011; Urrutia et al., 2019).
Diets that are rich in fiber produce a profile of VFA high in acetate and low in propionate, with a relatively high production of CH4 per unit of digested organic matter. On the other hand, concentrates, which are richer in starch, are fermented to more propionate and less CH4 (Johnson and Johnson, 1995; Janssen, 2010; Ungerfeld, 2013). The diet effect on the acetate to propionate ratio cannot be explained simply by different chemical composition of cellulose and starch, as they are both hydrolyzed to glucose (Janssen, 2010).
Janssen (2010) proposed a mechanism based on methanogens growth rate and the resulting H2 concentration, to explain how concentrates shift rumen fermentation from acetate to propionate and lower CH4 production. The replacement of roughages with concentrates induces changes such as increased rumen outflow rates and lower rumen pH. High rumen outflow rates impose methanogens that are not washed out of the rumen faster growth rates. Based on the Monod relationship of microbial growth, H2 concentration must increase when methanogens grow faster. In turn, greater H2 concentration would thermodynamically inhibit H2 production, and by doing so also inhibit acetate production, which is associated with H2 production. Greater H2 concentration would conversely favor [H] redirection toward alternative [H] sinks such as propionate. In agreement, the concentration of dissolved H2 in the rumen has been reported to associate negatively with acetate and positively with propionate molar percentages (Wang et al., 2017, 2018), although an association with propionate molar percentage was not observed in another study (Wang et al., 2016).
Similarly, methanogens are sensitive to the low rumen pH induced by feeding concentrates. A decrease in rumen pH is expected to decrease methanogens maximum growth rates, and, according to the Monod relationship, H2 concentration would increase if methanogens growth rate is maintained. An increase in H2 concentration would again thermodynamically shift fermentation from acetate to propionate. A similar explanation was provided for the accumulation of H2 and shift from acetate to propionate caused by chemical inhibitors of methanogenesis (Janssen, 2010).
Several experiments with defined cultures comparing the fermentation profile of pure cultures of H2 producers with co-cultures of the same organisms growing with methanogens demonstrate the profound influence of H2 removal by the methanogen on the fermentation profile of the H2-producing microorganism (Wolin et al., 1997). These insightful experiments provide a simple proof of concept for the theory proposed by Janssen (2010): in the absence of methanogens in the mono-culture (i.e., an analogous situation to methanogens completely washed out because a very high rumen outflow rate, or completely inhibited by low pH or an inhibitor of methanogenesis), H2 accumulates, inhibiting H2 formation and decreasing acetate production (i.e., a H2-releasing pathway). This in turn directs [H] toward reduced intermediates of rumen fermentation such as formate, ethanol, lactate and/or succinate (Chung, 1976; Chen and Wolin, 1977; Bauchop and Mountfort, 1981; Marvin-Sikkema et al., 1990; Pavlostathis et al., 1990), and [H] sinks such as propionate (Chen and Wolin, 1977) or butyrate (Chung, 1976). When H2 producers were co-cultured with methanogens they greatly diminished or stopped producing formate, ethanol, lactate and/or succinate, as well as propionate. The co-cultures accumulated less H2, and as CH4 formation removed H2, lower H2 concentration thermodynamically favored acetate production.
Increasing outflow rates in some continuous culture experiments (Isaacson et al., 1975; Stanier and Davies, 1981) agree with the predictions of the Janssen (2010) model. Results of the experiment by Wenner et al. (2017) do not fully agree, as, contrary to the model expectations decreasing pH decreased H2 concentration.
Immediately after feed ingestion, the most readily digestible feed components are rapidly digested and fermented, and H2 concentration rises (Robinson et al., 1981; Janssen, 2010; Guyader et al., 2015). After feed ingestion, increases in H2 emissions have been shown to occur earlier and faster than increases in CH4 (Rooke et al., 2014; Van Lingen et al., 2017; Söllinger et al., 2018), although this pattern has not occurred in all studies (Hillman et al., 1985). The peak in H2 emission preceding the evolution of CH4 production has been modeled by Van Lingen et al. (2019), and interpreted by Rooke et al. (2014) as the consequence of rapid fermentation and H2 production exceeding the capacity of methanogens to utilize all the H2 produced.
The lag period between the CH4 and H2 peaks observed in some studies suggests that, when fermentation is rapid, methanogens growth and/or the expression of genes encoding for methanogenesis enzymes lags behind rapid H2 evolution. In that regard, Söllinger et al. (2018) reported that whereas archaeal 16S rRNA genes abundance peaked at 1 h after feeding, methanogenesis mRNA abundance did not peak until 3 h after feeding. The question becomes what impedes or retards methanogens to respond with more rapid gene expression to make use of the elevated H2 concentrations occurring after a meal. It is possible that temporal increases in outflow rates occurring after feeding episodes (Van Lingen et al., 2019), result in high H2 concentration by increasing methanogens growth rate, as proposed by Janssen (2010). However, increasing the concentration of glucose in a chemostat at a constant pH and outflow rate still decreased CH4 production per mole of glucose fermented (Isaacson et al., 1975), which suggests a limitation of methanogenesis independent of outflow rate or pH to use all of the H2 made available by the rapid fermentation of glucose at high concentration (although H2 was not measured in that experiment). In 48 h batch cultures, there were distinct effects of pH and substrate composition (hay or cracked corn) on H2, CH4 and the acetate to propionate ratio (Russell, 1998), which can also be interpreted as an indication of an effect of the rate of fermentation per se independent of outflow rates or pH. It has also been speculated that the evolution of rumen H2-incorporating hydrogenases in the rumen environment with low H2 concentration may have resulted in low Km but also low Vmax for H2 (Ungerfeld, 2015a). This idea, however, does not agree with the high frequency of genomes of rumen organisms encoding [FeFe]-hydrogenases, and the high abundance of transcripts of various types of [FeFe]-hydrogenases in sheep rumens (Greening et al., 2019), as [FeFe]-hydrogenases have higher Vmax and Km for H2 uptake than [NiFe] hydrogenases (Frey, 2002).
Eighteen percent of rumen CH4 was estimated to be produced from formate as [H] donor (Hungate et al., 1970), and formate can be the [H] donor in fumarate reduction to succinate (Asanuma et al., 1999). It is thus possible that, the same as H2, formate concentration has an influence on CH4 production and the VFA profile. Other than an accumulation of formate as a response to methanogenesis inhibitors in some studies (Ungerfeld et al., 2003; Martinez-Fernandez et al., 2016, 2017), the effects of variables such as outflow rates, pH, or rate of fermentation on formate concentration, have not been investigated to the author’s knowledge. Generating information about the relationship between those variables and formate concentration, and how they relate to methanogens growth rate would be important for evaluating the influence of formate on the VFA profile, and integrating formate to a model of [H] flows in rumen fermentation.
Lactate is another intercellular e– carrier which, except for lactic acidosis, normally does not accumulate in the rumen and is extensively converted to VFA by various lactate utilizers (Chen et al., 2019). Small amounts of lactate have been reported to accumulate as a consequence of inhibiting methanogenesis in some (Amgarten et al., 1981), but not all (Božic et al., 2009; Martinez-Fernandez et al., 2016), studies. In general, lactate accumulation in the rumen is the result of lactate production rate surpassing lactate utilization as a consequence of rapid fermentation. A possible enhancement in the role of lactate as an intermediate of butyrate production in low CH4-producing sheep (Kamke et al., 2016) deserves further study (see section “The Competition for Dihydrogen”).
Succinate concentration in the rumen is typically low as it is rapidly converted to propionate (Blackburn and Hungate, 1963; Immig, 1996). It thus seems that succinate concentration exerts little influence on CH4 production and the VFA profile, although the finding by Kamke et al. (2016) of greater abundance of genes involved in the conversion of succinate to butyrate in low CH4-producing sheep prompts for more investigation.
The principle proposed by Janssen (2010) relating rumen H2 concentration to methanogens growth rates could be in theory extended to other hydrogenotrophs, provided that their pathway of H2 incorporation is thermodynamically feasible. The rate of H2 uptake by a methanogen would follow a Michaelis-Menten kinetics-wise function:
where vmet is the rate of H2 uptake (e.g., mol L–1 min–1), Vmax met is the maximum rate of H2 uptake with non-limiting H2 concentration, [H2] is the concentration of H2, and Km met is the apparent affinity for H2 i.e., the concentration of H2 at which the rate of H2 uptake is half maximal.
If a methanogen was growing in co-culture with another hydrogenotroph whose rate of H2 uptake was limited by H2 concentration, and H2 concentration was above the H2 thresholds (Cord-Ruwisch et al., 1988) of both organisms, it can be deduced (Supplementary Appendix S1) that the proportion of total H2 uptake incorporated into methanogenesis would be equal to:
where valt and Vmaxalt are the rate and the maximum rate of H2 uptake of the alternative hydrogenotroph, respectively, and Km alt is the affinity for H2 of the alternative hydrogenotroph, with the rest of the variables defined as in Eq. 1. This equation does not take into account possible thermodynamic constraints and differences in the efficiency of microbial growth.
Figure 4 shows a simulation of the proportion of H2 uptake incorporated into methanogenesis as a function of H2 concentration according to Eq. 2 in co-cultures of mixed methanogens and various hydrogenotrophs that reduce fumarate to succinate. The Km values for methanogens and fumarate reducers used in the simulation were reported by Asanuma et al. (1999). An equal Vmax was assumed for methanogenesis and fumarate reduction in this simulation. The range of H2 concentration in Figure 4 is based on dissolved H2 concentration measured directly in various in vivo studies (Table 1). It can be seen in Figure 4 that as H2 concentration increases, the proportion of H2 taken up by methanogens decrease and approaches 1/2 (Supplementary Appendix S2). It can be shown that if the Vmax of the alternative hydrogenotroph doubled the Vmax of the methanogens, the proportion of H2 taken up by methanogens would trend to 1/3 as H2 concentration increases (Supplementary Appendix S3).
Figure 4. Simulation of the proportion of dihydrogen taken up by methanogens in co-culture with various fumarate reducers as a function of dissolved H2 concentration. The simulation was conducted based on a kinetic Michaelis-Menten-wise competition for dihydrogen. Apparent Km for H2 uptake were reported by Asanuma et al. (1999). An equal Vmax for dihydrogen uptake is assumed. The range of dissolved H2 concentration is based on Table 1. The sky blue area corresponds approximately to baseline dissolved H2 concentrations (i.e., in between meals). The salmon area corresponds approximately to H2 concentration peaks occurring closely after feeding. The purple area corresponds approximately to the range of H2 concentration that could be observed when methanogenesis is inhibited.
For n hydrogenotrophs (including m methanogens), the proportion of total H2 taken up by m methanogens, can be generalized to:
(3, Supplementary Appendix S4)
At the low baseline H2 concentration prevailing in the rumen (Hungate, 1967), a low Km for H2 incorporation is key in the competition for H2 among thermodynamically feasible H2-incorporating processes. Methanogens have a lower Km for H2 than the fumarate reducers depicted in Figure 4, and consequently they would incorporate most of the H2 at the low H2 concentrations occurring between episodes of feed ingestion. Other Km values reported for methanogens are shown in Table 2, and are similar the Km of methanogens reported by Asanuma et al. (1999). In agreement with the predictions of Figure 4, previous co-culture experiments also show that the production of succinate or propionate by Ruminococcus flavefaciens growing on cellulose (Latham and Wolin, 1977) or by Selenomonas ruminantium growing on glucose or lactate (Chen and Wolin, 1977), decreased in the presence of methanogens compared to the pure cultures, although it continued being thermodynamically feasible, as it did not stop. In those co-culture experiments, H2 concentration (although it was not reported) was kept low by the methanogen, likely situating at the low end of the range of H2 concentration in Figure 4.
As H2 concentration increases, as it occurs after feed ingestion, or when feeding concentrates, or if methanogenesis is inhibited, the Km starts becoming less important to determine the partition of H2 incorporated into competing pathways, and a greater proportion of H2 would be incorporated into fumarate reduction to succinate (Figure 4). When H2 concentration is relatively high, a high Vmax for H2 can potentially become very important to determine the flow of H2 incorporated by a certain microorganism in a particular pathway. If the Vmax is expressed as the flow of H2 incorporated per gram of cell DM or cell protein, rather than the flow of H2 incorporated per volume of culture (or rumen contents), the flow of H2 into each pathway in the system will also depend on the cell density of each microbial species.
The incorporation of [H] into pathways alternative to methanogenesis can be limited by enzyme or substrate kinetics, or thermodynamics (Ungerfeld, 2015a). The addition of an e– acceptor that can be metabolized to VFA can help removing substrate kinetics or thermodynamic constraints. In general, adding to rumen fermentation carboxylic acids that are propionate or butyrate precursors as e– acceptors has had small effects on CH4 production in vitro (Callaway and Martin, 1996; Carro and Ranilla, 2003; Ungerfeld et al., 2003; Newbold et al., 2005; Riede et al., 2013) or in vivo (McGinn et al., 2004; Beauchemin and McGinn, 2006; Kolver and Aspin, 2006; Yang et al., 2012), although larger decreases in CH4 were observed in some experiments (Li et al., 2009; Wood et al., 2009). A likely interpretation is that much of the added propionate and butyrate precursor was not metabolized to the expected final product, and thus little [H] was directed away from CH4 formation (Carro and Ungerfeld, 2015). When methanogenesis was simultaneously inhibited with a chemical compound, increased availability of [H] not incorporated into CH4 favored the conversion of the added carboxylic acids to the expected end products. In the presence of inhibitors of methanogenesis, the addition of propionate precursors malate (Mohammed et al., 2004) or fumarate (Tatsuoka et al., 2008; Ebrahimi et al., 2011) increased propionate production in vitro and decreased H2 accumulation. In contrast, butyrate precursors did not decrease H2 accumulation caused by three inhibitors of methanogenesis in batch cultures (Ungerfeld et al., 2006). Martinez-Fernandez et al. (2017) successfully used phlorglucinol as an e– acceptor to decrease the accumulation of H2 and formate in the rumen of steers whose methanogenesis was inhibited with chloroform. An increase in acetate concentration observed when phlorglucinol was supplemented agrees with previous studies which had shown that phlorglucinol was reduced to acetate by rumen microorganisms using H2 or formate as e– donors (Martinez-Fernandez et al., 2017).
Microbial additives can help removing constraints to the incorporation of [H] into pathways alternative to methanogenesis whose rate is enzyme-limited. Jeyanathan et al. (2014) reviewed the use of direct-fed microbials to manipulate rumen biochemical pathways to decrease CH4 emissions. They proposed two main avenues to decrease CH4 formation in the rumen through the use of microbial additives: (i) Microbial additives that incorporate H2 into pathways alternative to methanogenesis, and (ii) Microbial additives that do not produce H2 in fermentation.
Microbial additives that compete with methanogens for H2 could be dosed into the rumen (Jeyanathan et al., 2014). It may also be possible to stimulate native rumen non-methanogenic hydrogenotrophs. Some fumarate reducers (Asanuma et al., 1999) and reductive acetogens (Chaucheyras et al., 1995; Joblin, 1999) were able to decrease CH4 production when grown in co-culture with methanogens, but because those experiments were conducted with elevated headspace H2, an ability of those organisms to compete for H2 at low concentration cannot be demonstrated (Figure 4). Methanogens would be expected to prevail over reductive acetogens in a co-culture at low H2 concentration due to their lower H2 thresholds (Cord-Ruwisch et al., 1988). Boccazzi and Patterson (2011) isolated rumen reductive acetogens with lower H2 thresholds than other reductive acetogens previously isolated from the rumen and with similar H2 thresholds to reductive acetogens from other environments. Yet, they still had higher H2 thresholds compared to methanogens (Table 3).
Table 3. Dihydrogen thresholds of methanogens and reductive acetogens from the rumen and other environments.
Supplementation of rumen batch cultures with succinate and propionate producers caused mild to moderate decreases in CH4 production (Alazzeh et al., 2012; Mamuad et al., 2014). In another study, supplementing rumen batch cultures with fumarate-reducing enterococci caused large decreases in CH4 and increases in propionate concentration (Kim et al., 2016). A slight decrease in CH4 production per kilogram of ingested feed occurred when supplementing Propionibacterium strains to heifers fed a high-forage (Vyas et al., 2014a), but not a mixed (Vyas et al., 2016), or a high-concentrate (Vyas et al., 2014b), diet. Rumen succinate producers W. succinogenes and Mannheimia succiniciproducens could be interesting candidates to compete with methanogens at low H2 concentrations, as they possess [NiFe]-hydrogenases for H2 uptake (Søndergaard et al., 2016). [NiFe]-hydrogenases have Km for H2 about two orders of magnitude lower than [FeFe] hydrogenases (Frey, 2002). However, the apparent Km of W. succinogenes for H2 was still higher than that of methanogens (Asanuma et al., 1999; Table 2). M. succiniciproducens has been genetically engineered to improve its yield of succinate from glucose (Lee et al., 2006; Choi et al., 2016), which could help increasing its Vmax for H2 uptake.
Nitrate reduction is thermodynamically more favorable than methanogenesis but may result in accumulation of the toxic intermediate nitrite. The addition of nitrite reducers may help avoiding nitrite toxicity while decreasing CH4 production (Jeyanathan et al., 2014). Nitrate should replace other sources of nitrogen on an isonitrogenous basis to avoid increasing the elimination of nitrogen in urine and the formation of nitrous oxide in the rumen, which is another potent greenhouse gas (Petersen et al., 2015). Sulfate reduction can also thermodynamically outcompete methanogenesis, although it generates the toxic reduced end product hydrogen sulfide (Jeyanathan et al., 2014).
Jeyanathan et al. (2014) also proposed that, by avoiding the formation of H2, the combined use of added lactate producers and the lactate utilizer Megasphaera elsdenii could channel [H] into propionate production instead of CH4. In that regard, lactate producers Sharpea and Kandleria were abundant in the rumens of one of two low CH4-producing sheep microbiomes (Kittelmann et al., 2014). Low CH4-producing sheep also had higher rumen concentration of lactate, and the lactate dehydrogenases that differed the most between the low- and the high-producing CH4 sheep associated phylogenetically with S. azabuensis and K. vitulina (Kamke et al., 2016). Several strains of Sharpea and Kandleria that produced predominantly lactate and small amounts of formate, ethanol and acetate, did not change their fermentation products when growing with a methanogen (Kumar et al., 2018).
Lactate produced by Sharpea and Kandleria did not accumulate to high concentrations in the rumen because it seemed to be metabolized by Megasphaera spp. mostly to butyrate, and to propionate via the non-randomizing pathway. The conversion of lactate to butyrate would result in less H2 production compared to acetate production from glucose (Kamke et al., 2016). It seems then that a dual mechanism resulted in lower CH4 production in the low-CH4 producing sheep (Kittelmann et al., 2014; Kamke et al., 2016): (i) Incorporation of [H] in the reduction of pyruvate to lactate by Sharpea and Kandleria instead of H2 release, and (ii) Uptake and conversion of lactate to butyrate and propionate by Megasphaera. In this regard, co-culture experiments comparing the kinetics of uptake of lactate and conversion to acetate, propionate and butyrate by Megasphaera and other microorganisms would be of interest. M. elsdenii had a lower affinity for lactate than for glucose (Russell and Baldwin, 1979), but its rate of lactate uptake was not affected by glucose (Russell and Baldwin, 1978). Interestingly, fermentation extracts of the probiotic Aspergillus oryzae stimulated lactate uptake by M. elsdenii and did not affect its fermentation profile (Waldrip and Martin, 1993).
This approach toward decreasing CH4 formation could thus contemplate the addition of a “microbial team” composed by a lactate producer and a lactate utilizer that metabolizes lactate to propionate or butyrate. Another interesting microbial species could be Fibrobacter succinogenes, a fiber degrader which does not produce H2 and would thus not contribute [H] to methanogenesis and instead incorporate [H] into succinate production (Morgavi et al., 2010). However, the higher Km for H2 of F. succinogenes compared to methanogens (Asanuma et al., 1999) would imply a lower uptake of H2 compared to methanogens at low H2 concentration (Figure 4).
Another strategy would be to inhibit methanogenesis with a chemical compound and simultaneously dose hydrogenotrophs that incorporate H2 into a desirable pathway, or pathways. For example, reductive acetogenesis was enhanced in batch cultures through simultaneous inhibition of methanogenesis and addition of reductive acetogens (Nollet et al., 1997; Le Van et al., 1998; Lopez et al., 1999). The kinetic parameters for H2 and the H2 threshold of the hydrogenotroph of choice would be very important. Compared to the typical rumen fermentation with CH4 as the main [H] sink (Figure 5A), inhibiting methanogenesis results in an increase in the incorporation of [H] into alternative sinks, but also in H2 as a [H] sink (Figure 5B). Adding a hydrogenotroph with a high Vmax for H2 can allow a high flow of H2 incorporation into a desirable fermentation product. However, if the Km of the added hydrogenotroph for H2 were high, H2 would still accumulate, and the magnitude of gaseous H2 losses could be important. A low Km for H2 would also result in H2 accumulation if the Vmax for H2 was low and the rate of fermentation was high, unless the microorganism was dosed in high numbers. Another possibility would be to combine a hydrogenotroph with high Km and Vmax for H2 with another hydrogenotroph with low Km and Vmax. A theoretically ideal situation in which dissolved H2 concentration and H2 emission are at the level of the rumen with functional methanogenesis is depicted in Figure 5C.
Figure 5. Three hypothetical scenarios of manipulation of metabolic hydrogen ([H]) flows in rumen fermentation: (A) Non-intervened rumen fermentation with functional methanogenesis. Methane (CH4) is the main sink of metabolic hydrogen; (B) Methanogenesis is inhibited with a chemical additive. Part of metabolic hydrogen spared from methane formation is redirected toward alternative sinks that are final fermentation products in the rumen with functional methanogenesis. Redirection of metabolic hydrogen toward alternative sinks is incomplete and the concentration of dissolved dihydrogen increases. The ratio of reduced to oxidized cofactors increases and fermentation, understood as the flow of carbon and the rate of metabolic hydrogen production, is inhibited; (C) A theoretical successful situation in which methanogenesis is inhibited with a chemical additive and an added live hydrogenotrophs redirects a greater proportion of metabolic hydrogen toward alternative sinks potentially beneficial to the host animal. Accumulation of dihydrogen is relieved, cofactors can be re-oxidized as in the rumen with functional methanogenesis, and fermentation is not inhibited.
A strategy employing chemical inhibitors of methanogenesis to redirect [H] from CH4 toward nutritionally useful alternative [H] sinks should evaluate possible direct effects of the chemical inhibitors on non-methanogenic rumen microorganisms, so as to avoid affecting processes such as fiber digestion or propionate production. This aspect cannot be studied in mixed cultures or in vivo, because in these systems, changes in non-methanogenic populations can indirectly result from changes in methanogens and CH4 production. The potential toxicity of chemical inhibitors of methanogenesis to non-methanogens should instead be studied in pure cultures. Mevastatin and lovastatin inhibited the growth of methanogens but not of major fermentative rumen bacteria, including major fiber degraders and propionate and butyrate producers (Miller and Wolin, 2001). Chloroform at 2 mM inhibited the growth of reductive acetogens and six other rumen bacteria, including fiber degraders and propionate and butyrate producers (Raju, 2016). In contrast, none of the non-methanogenic microorganisms examined were affected by acetylene at 1 mM (aqueous concentration) or 2-bromoethanesulfonate at 10 mM. The effect of n-butylisocyanide and 5,5′-dithio-bis-(2-nitrobenzoic acid) on reductive acetogens was concentration- and species-dependent, whilst 1,10-phenanthroline inhibited reductive acetogens at all of the concentrations studied (Raju, 2016). 3-Nitrooxypropanol at 0.1 mM did not inhibit the growth of 11 functionally diverse rumen bacteria or Escherichia coli, whilst much lower concentrations inhibited rumen and non-rumen methanogens (Duin et al., 2016).
From an applied point of view, the addition of a hydrogenotroph to the rumen would ideally target post-feeding peaks of dissolved H2 with the added microorganism at its exponential phase of growth. This might be difficult if the microbial additive was administered with the feed, as the added microorganism may be at its lag phase of growth at the peak of feed fermentation and H2 release in the rumen. If adding hydrogenotrophs at their exponential phase of growth in vitro was successful at utilizing H2, further developments toward practical application would need to optimize in vivo the timing, means of administration, and doses of added hydrogenotrophs.
Recently, Muñoz-Tamayo et al. (2019) conducted a growth and calorimetry experiment with three rumen methanogens. They estimated kinetic, thermodynamic, and growth parameters and predicted that in the long term only one methanogen would survive in tri-cultures. Similar conclusions were reached in another recent theoretical analysis (Lynch et al., 2019). Muñoz-Tamayo et al. (2019) discussed that, as the rumen harbors a diverse community of methanogens, ecological factors such as sensitivity to pH, location in association to fluid or particles, and endosymbiosis with protozoa can contribute to explain the existence of diversity despite of thermodynamic and kinetic advantages of some methanogens over others. Some ecological aspects can cause temporal and spatial variations in H2 concentration (Smolenski and Robinson, 1988; Janssen, 2010), which can affect the partition of H2 flows among different hydrogenotrophs. In the conceptual model proposed by Leng (2014), the organization of particle-colonizing microbiota in biofilms results in close proximities between cells releasing and taking up H2, resulting in ample variations in H2 concentration within the rumen.
Greening et al. (2019) reported no differences in the expression of the most abundant H2-evolving hydrogenases in sheep selected by low and high CH4 production. In contrast, there were differences between low- and high-CH4 producing sheep in the expression of H2-incorporating hydrogenases. The expression of methanogens hydrogenases and methyl-CoM reductase were lower, and the expression of fumarate reductase and acetyl-CoA synthase (which incorporate H2 into propionate production and reductive acetogenesis, respectively) were higher, in the low CH4-producing sheep. This can be interpreted as those alternative pathways of [H] incorporation decreasing CH4 formation in low CH4-producing sheep by competing with [H] with methanogenesis. Alternatively, it can also be interpreted as those pathways of [H] incorporation alternative to methanogenesis becoming upregulated in low CH4-producing animals as a response to less CH4 production, due perhaps to animal factors such as greater rumen outflow rate or lower rumen pH (Janssen, 2010).
Söllinger et al. (2018) reported that, among all bacterial functional genes, the greatest increase in mRNA abundance occurring 1 h after feeding corresponded to the fumarate reductase subunit C transcript, denoting a stimulation of propionate randomizing pathway associated to peaks of H2 emission after feeding. However, despite of the increase in the abundance of fumarate reductase transcripts, H2 emissions still increased and propionate concentration did not consistently increase 1 h after feeding. It is possible that the Km of H2 incorporation into propionate production was relatively high, at least under the conditions of that experiment, which would agree with the higher Km for H2 of fumarate reducers compared to methanogens reported by Asanuma et al. (1999) in pure cultures.
It should be considered that competition for intercellular e– carriers other than H2 (and lactate) also occurs. For example, the Km for formate was lower for fumarate reducers compared to methanogens (Asanuma et al., 1999). In the rumen, methanol and methylamines resulting from the metabolism of pectin (Pol and Demeyer, 1988) and betaine and choline (Neill et al., 1978; Mitchell et al., 1979) can be used by methylotrophic methanogens as substrates for CH4 production. Importantly, reductive acetogens have also been reported to use methanol and methylamines as [H] donors (Ragsdale and Pierce, 2008; Jeyanathan et al., 2014), including the rumen acetogen Eubacterium limosum, a methanol-utilizer (Genthner et al., 1981). It is thought that in the typical rumen fermentation, methanogens drop H2 pressure below the threshold for reductive acetogenesis (Ungerfeld and Kohn, 2006). However, it is important to examine both in defined and in mixed cultures the competition between methanogens and reductive acetogens for methanol and methylamines.
The formation of CH4 in the rumen represents an important loss of energy for the animal. Theoretically, inhibiting rumen methanogenesis could divert [H] toward fermentation products with a nutritional value for the animal, and improve animal productivity (Czerkawski and Breckenridge, 1975b; Schulman and Valentino, 1976), although this has not been consistently realized (Ungerfeld, 2018). Compared to the rumen with functional methanogenesis (Figure 5A), inhibiting rumen methanogenesis results in accumulation of H2 in vitro (Ungerfeld, 2015b) and increased H2 emissions in vivo (Ungerfeld, 2018), increased ratio of NADH to NAD+ (Hino and Russell, 1985; Figure 5B), and decreased reducing potential (Sauer and Teather, 1987). These changes indicate hindering in e– disposal and it is important to understand the consequences that this can have on feed fermentation and digestion. Conceptually, there is little doubt that an imbalance between the rates of reduction and re-oxidation of cofactors can halt fermentation (Wolin et al., 1997), because the turnover rates of cofactors are very high compared to their intracellular concentrations (de Graef et al., 1999). This principle has been experimentally verified as an increase in cellulose degradation when fibrolytic fungi were co-cultured with methanogens or with S. ruminantium as hydrogenotrophs (Marvin-Sikkema et al., 1990). The questions are, at which point increases in the ratios of reduced to oxidized cofactors begin to impair fermentation, and how these ratios are in turn affected by H2 pressure (Figure 5B). Whether accumulated H2 can be re-channeled into other pathways (Figure 5C) has been discussed in the preceding section.
High H2 pressure can thermodynamically inhibit NADH oxidoreductases (Gottschalk, 1986). Van Lingen et al. (2016) modeled the effect of H2 pressure on the thermodynamic feasibility of NADH oxidation with and without electron confurcation with reduced Fdred2–. With an NAD+ to NADH ratio of 3, similar to the NAD+ to NADH ratio reported by Hino and Russell (1985) for their control treatments, and in the absence of electron confurcation, NADH oxidation was somewhat under thermodynamic control at H2 partial pressures of between 2 × 10–4 and 2 × 10–3 bar, depending on the intracellular pH (Van Lingen et al., 2016). If rumen headspace H2 was to be at equilibrium with dissolved H2, the corresponding range of dissolved H2 concentrations would be as low as 0.15 to 1.5 μM approximately (calculations not shown), but given the occurrence of H2 supersaturation (Wang et al., 2016) it would likely be higher. The same calculation conducted with NADH oxidation occurring through electron confurcation would yield a considerable higher range of dissolved H2 concentration between 6 and 100 μM, again assuming equilibrium between gaseous and aqueous H2. Therefore, with electron confurcation, the range of dissolved H2 concentration at which NADH oxidation becomes thermodynamically controlled coincides or is even higher than previously reported peaks of dissolved H2 concentration after feed ingestion, or the dissolved H2 concentration reported by Melgar et al. (2019) for methanogenesis inhibition (Table 1). This agrees with the findings by Greening et al. (2019) regarding the importance of confurcating hydrogenases in H2 formation in the rumen. The thermodynamic feasibility of NADH oxidation also depends on the intracellular pH (Van Lingen et al., 2016), which in turn depends on the extracellular pH and the bacterial species (Russell, 1991).
Inhibiting methanogenesis in vitro results in H2 accumulation and consistently inhibits hexoses fermentation as estimated through the stoichiometry of VFA production (Ungerfeld, 2015b). However, the estimation of fermented hexoses from the stoichiometry of VFA production does not consider carbon in fermented hexoses utilized in microbial biomass accretion. In an in vitro study with several inhibitors of methanogenesis, no consistent effects on true organic matter digestibility were found, with some decreases but also lack of effects with other additives (Ungerfeld et al., 2019). Effects of inhibiting methanogenesis in vivo on digestion and fermentation in the rumen are complex to assess with most animal measurements, as apparent digestibility determinations do not consider microbial biomass and overall tract digestibility could be modified by post-ruminal compensations (Ungerfeld, 2018), and rumen VFA concentrations are affected by, apart from VFA production rates, rates of VFA absorption, passage, incorporation into microbial biomass, and by changes in rumen volume (Dijkstra et al., 1993; Kristensen, 2001; Storm et al., 2012; Hall et al., 2015).
It is of course possible that negative effects of inhibiting methanogenesis on fermentation (Ungerfeld, 2015b) are not caused by H2 accumulation per se, and instead some of the chemical inhibitors studied could be toxic to microorganisms other than methanogens. An experimental approach to study the effects of H2 accumulation on the rate of fermentation without the addition of chemical inhibitors of methanogenesis is the addition of H2 gas to the headspace of rumen incubations. In general, adding external H2 to rumen cultures has not consistently resulted in an inhibition of fermentation measured as total VFA concentration or apparent digestibility (Schulman and Valentino, 1976; Patra and Yu, 2013; Broudiscou et al., 2014; Qiao et al., 2015). A factor potentially masking the effects of H2 gas added to microbial cultures headspace is lack of equilibrium with dissolved H2 (Wang et al., 2016). In that regard, two in vivo studies in which dissolved H2 was indirectly delivered through the reaction of elemental magnesium (Mg) with water, reported decreases in total VFA concentration as a result of the augmented dissolved H2 concentration (Wang et al., 2017; Ma et al., 2019), although the limitations of VFA concentration as a metric of rumen fermentation pointed out above are again acknowledged.
Ultimately, if means to efficiently redirect [H] to useful sinks (Figure 5C) could be designed, the extent to which the accumulation of H2 can hinder NADH re-oxidation and fermentation would be unimportant from a practical standpoint, as H2 would not accumulate when inhibiting methanogenesis (Figure 5C). A perhaps more realistic scenario intermediate between Figures 5B,C in which dissolved H2 concentration was only partially relieved, but the rate of digestion and fermentation was not affected, can also be conceived.
Pathways of [H] flow alternative to H2 formation can result in the production of other intercellular e– carriers, such as lactate, ethanol, and formate, or final fermentation products such as propionate, all of which also help NADH oxidation (Van Lingen et al., 2016). Formate, succinate or ethanol have been shown to accumulate along with H2 when methanogenesis was inhibited in vitro (Slyter, 1979; Asanuma et al., 1998; Ungerfeld et al., 2003) and in vivo (Martinez-Fernandez et al., 2016, 2017; Melgar et al., 2019), so it is important to understand if the accumulation of those metabolites could potentially inhibit cofactors re-oxidation and fermentation. The effects of lactate, ethanol, formate and propionate on fermentation and digestion are best studied in experiments in which those metabolites are externally added to rumen fermentation as pure compounds. Immig (1996) found that adding formate or succinate to rumen batch cultures did not affect total VFA production. Asanuma et al. (1998) found that added formate was stoichiometrically recovered as CH4 and total VFA production was unaffected. Infusion of formic acid into the rumen of sheep did not affect overall tract apparent digestibility (Vercoe and Blaxter, 1965). It is possible that as formate has a high rate of diffusion and is rapidly converted to CH4 (Leng, 2014), its accumulation may not affect cofactors re-oxidation and fermentation rate.
Lactate is metabolized to VFA in the rumen (Chen et al., 2019). Even though excess lactate accumulation can inhibit fermentation, this effect would likely be caused by low pH rather than by an impairment of [H] transfer and co-factor re-oxidation. A potential effect of lactate accumulation on fermentation independent of pH would have to be evaluated through, for example, a comparison of the addition of sodium lactate against sodium chloride.
The effects of adding ethanol to rumen cultures was dose-depending, and a decrease in cellulose digestibility occurred with the highest dose (Chalupa et al., 1964), whereas no effects on total VFA concentration was found in another in vitro experiment (Pradhan and Hemken, 1970). Emery et al. (1959) found a tendency to decrease OM and N digestibility when feeding ethanol to sheep. However, negative effects of high doses of ethanol on rumen fermentation can be mediated through direct toxicity related to bacterial membranes leakages caused by ethanol (Ingram, 1990), rather than through impairing re-oxidation of cofactors.
As an end product of fermentation, propionate is removed by absorption, passage and incorporation into microbial biomass. The removal of propionate would be thought to occur rapidly enough so as to avoid accumulation causing inhibition of fermentation. In agreement, in several experiments intrarruminal infusion of propionate did not affect overall tract digestibility of various feed fractions (Rook et al., 1963; Sheperd and Combs, 1998; Noziere et al., 2000; Oba and Allen, 2003).
Flows of [H] in the rumen can affect microbial growth through at least three mechanisms: (i) Variation in the generation of ATP; (ii) Provision of precursors for biosynthesis; (iii) Provision of reducing power. The mechanisms through which this might occur will be developed in this section.
Hydrolysis of ATP is necessary to drive otherwise thermodynamically unfeasible anabolic processes, such as protein synthesis. The rate of ATP generation in fermentation depends on the rate of fermentation, the fermentation profile, and the ATP generated in each fermentation pathway. Acetate production generates ATP through substrate level phosphorylation, the same as propionate non-randomizing pathway. In propionate randomizing pathway, butyrate production, and methanogenesis, ATP is also generated through ETLP (Russell and Wallace, 1997; Hackmann and Firkins, 2015). Production of less reduced fermentation products such as lactate and ethanol generates ATP only in glycolysis and results in less microbial growth per unit of substrate degraded compared to acetate production and methanogenesis (Wolin et al., 1997).
One should bear in mind that increasing ATP generation does not necessarily mean maximizing the “efficiency” of fermentation. As the proportion of the ΔG of a pathway coupled to ATP generation increases, the net ΔG approaches zero, and the pathway slows down approaching equilibrium. For example, methanogens possessing cytochromes can generate more ATP per mole of CH4 produced and have higher growth yields when growing on elevated H2 concentration compared to methanogens without cytochromes, but on the other hand they have greater H2 thresholds. Methylotrophic methanogens of the order Methanosarcinales have cytochromes and they have evolved to live in environments with low H2 concentration by acquiring the capacity of using one carbon compounds as substrates for methanogenesis (Thauer et al., 2008; Vanwonterghem et al., 2016; Lynch et al., 2019).
Organic matter catabolized in the rumen is partitioned into fermentation products i.e., VFA and gases, and microbial biomass. The proportion of carbon in fermented carbohydrates diverted toward microbial cell production increases as the microbial biomass produced per mole of ATP hydrolyzed (YATP) increases (Leng and Nolan, 1984) and as more moles of ATP are generated per mole of hexoses fermented. Also, each fermentation pathway can contribute different intermediate compounds to microbial anabolism.
Microbial biomass is more reduced than substrate fermented, and consequently, alterations in the flows of [H] could affect [H] available for microbial biomass accretion. For example, inhibiting methanogenesis could result in increased [H] disposal into microbial biomass formation (Czerkawski, 1986). Anabolic processes such as amino acids and fatty acids synthesis demand [H] and may be stimulated as a consequence of the inhibition of CH4 production (Chalupa, 1977; Ungerfeld, 2015b). Deamination of reduced amino acids was inhibited by reducing power in the form of NADH, and conversely, was stimulated by methylene blue, an oxidizing agent (Hino and Russell, 1985). Later results, however, could not confirm an increase in the incorporation of [H] into microbial amino acids when methanogenesis was inhibited in vitro (Ungerfeld et al., 2019).
Early work in the past century established the foundations to understand fermentation and [H] dynamics in the rumen. Hungate (1967) demonstrated the central role of H2 in CH4 production. The principles and importance of interspecies H2 transfer was illustrated in several ingenious experiments in which H2 producers were co-cultured with methanogens (Wolin et al., 1997). A model to explain how the diet influences the VFA profile and CH4 formation through changes in methanogens rate of growth and H2 concentration (Janssen, 2010) has been an important advancement in this area. Electron confurcation has been incorporated into rumen fermentation models (Van Lingen et al., 2016, 2019), and recent experimental work with comparative genomics and metatranscriptomics revealed the importance of electron bifurcation and confurcation in H2 dynamics in the rumen. Shifts in fermentation in defined cultures were studied at the level of gene expression (Greening et al., 2019).
In comparison, fewer studies (Chen and Wolin, 1977; Latham and Wolin, 1977) have examined the competition for H2 between methanogens and other hydrogenotrophs, such as succinate and propionate producers, at the basal H2 concentrations resulting from fermentation-evolving H2. A recent experiment studied the competition for [H] between a methanogen and lactate producers Sharpea and Kandleria (Kumar et al., 2018). Pure cultures of rumen hydrogenotrophs, such as those isolated in the Hungate 1000 Project (Kelly, 2016), could be screened for kinetic parameters of H2 incorporation and H2 thresholds. This information could be used to predict the outcome of the competition for H2 between methanogens and other hydrogenotrophs with models similar to the ones generated by Muñoz-Tamayo et al. (2019) and Lynch et al. (2019) for competition between methanogens. The ability of non-methanogenic hydrogenotrophs to compete for H2 could be evaluated in co-culture with methanogens if they are also fermentative H2 producers themselves (e.g., R. flavefaciens, S. ruminantium), or in tri-cultures with methanogens and a H2-producing organism if they incorporate H2 but do not produce it (e.g., F. succinogenes, Succinivibrio dextrinosolvens, Succinimonas amylolytica, reductive acetogens).
Understanding the physicochemical control of rumen fermentation can help optimizing the design of strategies to direct [H] from CH4 to other sinks. In this regard, H2 concentration is highly influential on the thermodynamics and kinetics of fermentation pathways (Janssen, 2010). Research is needed on dissolved H2 concentration (Table 1) and H2 gradients under different conditions, especially when methanogenesis is inhibited. Dissolved H2 concentration has been generally estimated by measuring the concentration of H2 gas in the gas phase and assuming equilibrium with dissolved H2 in the fluid (Kohn and Boston, 2000; Ungerfeld and Kohn, 2006; Janssen, 2010), but H2 has been shown to be supersaturated in the rumen (Wang et al., 2016). It would be important to incorporate H2 supersaturation factors in future models of rumen fermentation, but more results with different diets, time after feeding, and other factors such as methanogenesis inhibition, are needed so that H2 supersaturation is not modeled as a constant.
The application of genomics and transcriptomics has advanced our understanding of the relationships between the abundance and expression of genes encoding for hydrogenases and rumen [H] flows (Greening et al., 2019). The combination of –omics techniques with classical biochemistry and microbiology methods may make possible the isolation and kinetic characterization of H2-incorporating hydrogenases. The application of proteomics to understand methanogenesis and flows of [H] through changes in hydrogenases and other enzymes involved in [H] transactions is also of much interest (Snelling and Wallace, 2017). Recently, metabolomics has been applied toward the understanding of differences between dairy cows with high and low feed utilization efficiency associated to high and low CH4 production (Shabat et al., 2016) and toward understanding the responses to methanogenesis inhibitors (Martinez-Fernandez et al., 2018). Finally, experimental advances must be interpreted in the light of basic physicochemical knowledge of thermodynamics and kinetics to develop mathematical and conceptual mechanistic models (Janssen, 2010; Van Lingen et al., 2016) for designing new strategies of manipulation of [H] flows in the rumen and predicting their outcomes.
EU reviewed and conceptualized the ideas, and wrote, edited and submitted the manuscript.
Support from Agencia Nacional de Investigación y Desarrollo ANID through project Fondecyt 1190574 is gratefully acknowledged.
The author declares that the research was conducted in the absence of any commercial or financial relationships that could be construed as a potential conflict of interest.
The Supplementary Material for this article can be found online at: https://www.frontiersin.org/articles/10.3389/fmicb.2020.00589/full#supplementary-material
Alazzeh, A. Y., Sultana, H., Beauchemin, K. A., Wang, Y., Holo, H., Harstad, O. M., et al. (2012). Using strains of Propionibacteria to mitigate methane emissions in vitro. Acta Agric. Scand. A 62, 263–272. doi: 10.1080/09064702.2013.773056
Allen, M. S., Bradford, B. J., and Oba, M. (2009). Board-invited review: the hepatic oxidation theory of the control of feed intake and its application to ruminants. J. Anim. Sci. 87, 3317–3334. doi: 10.2527/jas.2009-1779
Amgarten, M., Schatzmann, H. J., and Wüthrich, A. (1981). ‘Lactate type’ response of ruminal fermentation to chloral hydrate, chloroform and trichloroethanol. J. Vet. Pharmacol. Ther. 4, 241–248. doi: 10.1111/j.1365-2885.1981.tb00736.x
Armstrong, D. G., and Blaxter, K. L. (1957). The utilization of acetic, propionic and butyric acids by fattening sheep. Br. J. Nutr. 11, 413–425. doi: 10.1079/BJN19570063
Asanuma, N., Iwamoto, M., and Hino, T. (1998). Formate metabolism by ruminal microorganisms in relation to methanogenesis. Anim. Sci. Technol. 69, 576–584. doi: 10.2508/chikusan.69.576
Asanuma, N., Iwamoto, M., and Hino, T. (1999). Effect of the addition of fumarate on methane production by ruminal microorganisms in vitro. J. Dairy Sci. 82, 780–787. doi: 10.3168/jds.s0022-0302(99)75296-3
Aschenbach, J. R., Kristensen, N. B., Donkin, S. S., Hammon, H. M., and Penner, G. B. (2010). Gluconeogenesis in dairy cows: the secret of making sweet milk from sour dough. IUBMB Life 62, 869–877. doi: 10.1002/iub.400
Bauchop, T., and Mountfort, D. O. (1981). Cellulose fermentation by a rumen anaerobic fungus in both the absence and the presence of rumen methanogens. Appl. Environ. Microbiol. 42, 1103–1110. doi: 10.1128/aem.42.6.1103-1110.1981
Beauchemin, K. A., and McGinn, S. M. (2006). Methane emissions from beef cattle: effects of fumaric acid, essential oil, and canola oil1. J. Anim. Sci. 84, 1489–1496. doi: 10.2527/2006.8461489x
Blackburn, T. H., and Hungate, R. E. (1963). Succinic acid turnover and propionate production in the bovine rumen. Appl. Microbiol. 11, 132–135. doi: 10.1128/aem.11.2.132-135.1963
Boccazzi, P., and Patterson, J. A. (2011). Using hydrogen-limited anaerobic continuous culture to isolate lowhydrogen threshold ruminal acetogenic bacteria. Agric. Food Anal. Bacteriol. 1, 33–44.
Božic, A. K., Anderson, R. C., Carstens, G. E., Ricke, S. C., Callaway, T. R., Yokoyama, M. T., et al. (2009). Effects of the methane-inhibitors nitrate, nitroethane, lauric acid, Lauricidin§and the Hawaiian marine algae Chaetoceros on ruminal fermentation in vitro. Bioresour. Technol. 100, 4017–4025. doi: 10.1016/j.biortech.2008.12.061
Breznak, J. A., Switzer, J. M., and Seitz, H. J. (1988). Sporomusa termitida sp. nov., an H2/CO2-utilizing acetogen isolated from termites. Arch. Microbiol. 150, 282–288. doi: 10.1007/BF00407793
Broudiscou, L. P., Offner, A., and Sauvant, D. (2014). Effects of inoculum source, pH, redox potential and headspace di-hydrogen on rumen in vitro fermentation yields. Animal 8, 931–937. doi: 10.1017/s1751731114000640
Buckel, W., and Thauer, R. K. (2013). Energy conservation via electron bifurcating ferredoxin reduction and proton/Na+ translocating ferredoxin oxidation. Biochim. Biophys. Acta 1827, 94–113. doi: 10.1016/j.bbabio.2012.07.002
Buckel, W., and Thauer, R. K. (2018a). Flavin-based electron bifurcation, a new mechanism of biological energy coupling. Chem. Rev. 118, 3862–3886. doi: 10.1021/acs.chemrev.7b00707
Buckel, W., and Thauer, R. K. (2018b). Flavin-based electron bifurcation, ferredoxin, flavodoxin, and anaerobic respiration with protons (Ech) or NAD+ (Rnf) as electron acceptors: a historical review. Front. Microbiol. 9:401. doi: 10.3389/fmicb.2018.00401
Cabezas-Garcia, E. H., Krizsan, S. J., Shingfield, K. J., and Huhtanen, P. (2017). Between-cow variation in digestion and rumen fermentation variables associated with methane production. J. Dairy Sci. 100, 4409–4424. doi: 10.3168/jds.2016-12206
Callaway, T. R., and Martin, S. A. (1996). Effects of organic acid and monensin treatment on in vitro mixed ruminal microorganism fermentation of cracked corn. J. Anim. Sci. 74, 1982–1989. doi: 10.2527/1996.7481982x
Carro, M. D., and Ranilla, M. J. (2003). Influence of different concentrations of disodium fumarate on methane production and fermentation of concentrate feeds by rumen micro-organisms in vitro. Br. J. Nutr. 90, 617–623. doi: 10.1079/bjn2003935
Carro, M. D., and Ungerfeld, E. M. (2015). “Utilization of organic acids to manipulate ruminal fermentation and improve ruminant productivity,” in Rumen Microbiology: From Evolution to Revolution, eds A. K. Puniya, R. Singh, and D. N. Kamra, (New Dehli: Springer), 177–197. doi: 10.1007/978-81-322-2401-3_13
Chalupa, W. (1977). Manipulating rumen fermentation. J. Anim. Sci. 46, 585–599. doi: 10.2527/jas1977.453585x
Chalupa, W., Evans, J. L., and Stillions, M. C. (1964). Influence of ethanol on rumen fermentation and nitrogen metabolism. J. Anim. Sci. 23, 802–807. doi: 10.2527/jas1964.233802x
Chaucheyras, F., Fonty, G., Bertin, G., and Gouet, P. (1995). In vitro H2 utilization by a ruminal acetogenic bacterium cultivated alone or in association with an archaea methanogen is stimulated by a probiotic strain of Saccharomyces cerevisiae. Appl. Environ. Microbiol. 61, 3466–3467. doi: 10.1128/aem.61.9.3466-3467.1995
Chen, L., Shen, Y., Wang, C., Ding, L., Zhao, F., Wang, M., et al. (2019). Megasphaera elsdenii lactate degradation pattern shifts in rumen acidosis models. Front. Microbiol. 10:162. doi: 10.3389/fmicb.2019.00162
Chen, M., and Wolin, M. J. (1977). Influence of CH4 production by Methanobacterium ruminantium on the fermentation of glucose and lactate by Selenomonas ruminantium. Appl. Environ. Microbiol. 34, 756–759. doi: 10.1128/aem.34.6.756-759.1977
Choi, S., Song, H., Lim, S. W., Kim, T. Y., Ahn, J. H., Lee, J. W., et al. (2016). Highly selective production of succinic acid by metabolically engineered Mannheimia succiniciproducens and its efficient purification. Biotechnol. Bioeng. 113, 2168–2177. doi: 10.1002/bit.25988
Chung, K. T. (1976). Inhibitory effects of H2 on growth of Clostridium cellobioparum. Appl. Environ. Microbiol. 31, 342–348. doi: 10.1128/aem.31.3.342-348.1976
Cord-Ruwisch, R., Seitz, H. J., and Conrad, R. (1988). The capacity of hydrogenotrophic anaerobic bacteria to compete for traces of hydrogen depends on the redox potential of the terminal electron acceptor. Arch. Microbiol. 149, 350–357. doi: 10.1007/bf00411655
Czerkawski, J. W., and Breckenridge, G. (1975a). New inhibitors of methane production by rumen micro- organisms. Experiments with animals and other practical possibilities. Br. J. Nutr. 34, 447–457. doi: 10.1017/s0007114575000505
Czerkawski, J. W., and Breckenridge, G. (1975b). New inhibitors of methane production by rumen micro-organisms. Development and testing of inhibitors in vitro. Br. J. Nutr. 34, 429–446. doi: 10.1017/S0007114575000499
Davies, A., Nwaonu, H. N., Stanier, G., and Boyle, F. T. (1982). Properties of a novel series of inhibitors of rumen methanogenesis; in vitro and in vivo experiments including growth trials on 2,4-bis (trichloromethyl)-benzo [1, 3]dioxin-6-carboxylic acid. Br. J. Nutr. 47, 565–576. doi: 10.1079/bjn19820068
de Graef, M. R., Alexeeva, S., Snoep, J. L., and Teixeira de Mattos, M. J. (1999). The steady-state internal redox state (NADH/NAD) reflects the external redox state and is correlated with catabolic adaptation in Escherichia coli. J. Bacteriol. 181, 2351–2357. doi: 10.1128/jb.181.8.2351-2357.1999
De Vries, W., van Wyck-Kapteyn, W. M., and Stouthamer, A. H. (1973). Generation of ATP during cytochrome-linked anaerobic electron transport in propionic acid bacteria. J. Gen. Microbiol. 76, 31–41. doi: 10.1099/00221287-76-1-31
Denman, S. E., Martínez-Fernández, G., Shinkai, T., Mitsumori, M., and McSweeney, C. S. (2015). Metagenomic analysis of the rumen microbial community following inhibition of methane formation by a halogenated methane analogue. Front. Microbiol. 6:1087. doi: 10.3389/fmicb.2015.01087
Dijkstra, J., Boer, H., Van Bruchem, J., Bruining, M., and Tamminga, S. (1993). Absorption of volatile fatty acids from the rumen of lactating dairy cows as influenced by volatile fatty acid concentration, pH and rumen liquid volume. Br. J. Nutr. 69, 385–396. doi: 10.1079/bjn19930041
Duin, E. C., Wagner, T., Shima, S., Prakash, D., Cronin, B., Yanez-Ruiz, D. R., et al. (2016). Mode of action uncovered for the specific reduction of methane emissions from ruminants by the small molecule 3-nitrooxypropanol. Proc. Natl. Acad. Sci. U.S.A. 113, 6172–6177. doi: 10.1073/pnas.1600298113
Ebrahimi, S. H., Mohinia, M., Singhala, K. K., Miria, V. H., and Tyagi, A. K. (2011). Evaluation of complementary effects of 9,10-anthraquinone and fumaric acid on methanogenesis and ruminal fermentation in vitro. Arch. Anim. Nutr. 65, 267–277. doi: 10.1080/1745039X.2011.594345
Eckard, R. J., Grainger, C., and De Klein, C. A. M. (2010). Options for the abatement of methane and nitrous oxide from ruminant production: a review. Livestock Sci. 130, 47–56. doi: 10.1016/j.livsci.2010.02.010
Emery, R. S., Lewis, T. R., Everett, J. P., and Lassiter, C. A. (1959). Effect of ethanol on rumen fermentation. J. Dairy Sci. 42, 1182–1186. doi: 10.3168/jds.S0022-0302(59)90710-6
Frei, M. (2013). Lignin: characterization of a multifaceted crop component. Sci. World J. 2013:436517. doi: 10.1155/2013/436517
Frey, M. (2002). Hydrogenases: hydrogen-activating enzymes. Chembiochem 3, 153–160. doi: 10.1002/1439-7633(20020301)3:2/3<153::aid-cbic153>3.0.co;2-b
Gagen, E. J., Mosoni, P., Denman, S. E., Al Jassim, R., McSweeney, C. S., and Forano, E. (2012). Methanogen colonisation does not significantly alter acetogen diversity in lambs isolated 17 h after birth and raised aseptically. Microb. Ecol. 64, 628–640. doi: 10.1007/s00248-012-0024-z
Genthner, B. R., Davis, C. L., and Bryant, M. P. (1981). Features of rumen and sewage sludge strains of Eubacterium limosum, a methanol- and H2-CO2-utilizing species. Appl. Environ. Microbiol. 42, 12–19. doi: 10.1128/aem.42.1.12-19.1981
Gerber, P. J., Hristov, A. N., Henderson, B., Makkar, H., Oh, J., Lee, C., et al. (2013). Technical options for the mitigation of direct methane and nitrous oxide emissions from livestock: a review. Animal 7, 220–234. doi: 10.1017/S1751731113000876
Greening, C., Geier, R., Wang, C., Woods, L. C., Morales, S. E., McDonald, M. J., et al. (2019). Diverse hydrogen production and consumption pathways influence methane production in ruminants. ISME J. 13, 2617–2632. doi: 10.1038/s41396-019-0464-2
Guyader, J., Eugene, M., Meunier, B., Doreau, M., Morgavi, D. P., Silberberg, M., et al. (2015). Additive methane-mitigating effect between linseed oil and nitrate fed to cattle. J. Anim. Sci. 93, 3564–3577. doi: 10.2527/jas.2014-8196
Hackmann, T. J., and Firkins, J. (2015). Electron transport phosphorylation in rumen butyrivibrios: unprecedented ATP yield for glucose fermentation to butyrate. Front. Microbiol. 6:622. doi: 10.3389/fmicb.2015.00622
Hackmann, T. J., Ngugi, D. K., Firkins, J. L., and Tao, J. (2017). Genomes of rumen bacteria encode atypical pathways for fermenting hexoses to short-chain fatty acids. Environ. Microbiol. 19, 4670–4683. doi: 10.1111/1462-2920.13929
Hall, M. B., Nennich, T. D., Doane, P. H., and Brink, G. E. (2015). Total volatile fatty acid concentrations are unreliable estimators of treatment effects on ruminal fermentation in vivo. J. Dairy Sci. 98, 3988–3999. doi: 10.3168/jds.2014-8854
Hegarty, R. S., and Gerdes, R. (1999). Hydrogen production and transfer in the rumen. Recent Adv. Anim. Nutr. Aust. 12, 37–44.
Henderson, C. (1980). The influence of extracellular hydrogen on the metabolism of Bacteroides rurninicola, Anaerovibrio lipolytica and Selenomonas ruminantiurn. J. Gen. Microbiol. 119, 485–491. doi: 10.1099/00221287-119-2-485
Hillman, K., Lloyd, D., and Williams, A. G. (1985). Use of a portable quadrupole mass spectrometer for the measurement of dissolved gas concentrations in ovine rumen liquor in situ. Curr. Microbiol. 12, 335–340.
Hino, T., and Russell, J. B. (1985). Effect of reducing-equivalent disposal and NADH/NAD on deamination of amino acids by intact rumen microorganisms and their cell extracts. Appl. Environ. Microbiol. 50, 1368–1374. doi: 10.1128/aem.50.6.1368-1374.1985
Hungate, R. E. (1967). Hydrogen as an intermediate in the rumen fermentation. Arch. Mikrobiol. 59, 158–164. doi: 10.1007/bf00406327
Hungate, R. E., Smith, W., Bauchop, T., Yu, I., and Rabinowitz, J. C. (1970). Formate as an intermediate in the bovine rumen fermentation. J. Bacteriol. 102, 389–397. doi: 10.1128/jb.102.2.389-397.1970
Immig, I. (1996). The rumen and hindgut as source of ruminant methanogenesis. Environ. Monit. Assess. 42, 57–72. doi: 10.1007/BF00394042
Ingram, L. O. (1990). Ethanol tolerance in bacteria. Crit. Rev. Biotechnol. 9, 305–319. doi: 10.3109/07388558909036741
Isaacson, H. R., Hinds, F. C., Bryant, M. P., and Owens, F. N. (1975). Efficiency of energy utilization by mixed rumen bacteria in continuous culture. J. Dairy Sci. 58, 1645–1659. doi: 10.3168/jds.s0022-0302(75)84763-1
Janssen, P. H. (2010). Influence of hydrogen on rumen methane formation and fermentation balances through microbial growth kinetics and fermentation thermodynamics. Anim. Feed Sci. Technol. 160, 1–22. doi: 10.1016/j.anifeedsci.2010.07.002
Jeyanathan, J., Martin, C., and Morgavi, D. P. (2014). The use of direct-fed microbials for mitigation of ruminant methane emissions: a review. Animal 8, 250–261. doi: 10.1017/s1751731113002085
Joblin, K. N. (1999). Ruminal acetogens and their potential to lower ruminant methane emissions. Aust. J. Agric. Res. 50, 1307–1313. doi: 10.1071/AR99004
Johnson, K. A., and Johnson, D. E. (1995). Methane emissions from cattle. J. Anim. Sci. 73, 2483–2492. doi: 10.2527/1995.7382483x
Kamke, J., Kittelmann, S., Soni, P., Li, Y., Tavendale, M., Ganesh, S., et al. (2016). Rumen metagenome and metatranscriptome analyses of low methane yield sheep reveals a Sharpea-enriched microbiome characterised by lactic acid formation and utilisation. Microbiome 4:56. doi: 10.1186/s40168-016-0201-2
Kelly, W. J. (2016). RMG Network. Available at: https://rmgnetworks.com/documents/Hardware_and_Software_Requirements_for_Client_Applications_IVS_Enterprise_Server_Version_12.5.pdf (accessed March 5, 2020).
Kim, S. H., Mamuad, L. L., Kim, D. W., Kim, S. K., and Lee, S. S. (2016). Fumarate reductase-producing enterococci reduce methane production in in vitro rumen fermentation. J. Microbiol. Biotechnol. 26, 558–566. doi: 10.4014/jmb.1512.12008
Kittelmann, S., Pinares-Patiño, C. S., Seedorf, H., Kirk, M. R., Ganesh, S., McEwan, J. C., et al. (2014). Two different bacterial community types are linked with the low-methane emission trait in sheep. PLoS One 9:e103171. doi: 10.1371/journal.pone.0103171
Klieve, A. V., Ouwerkerk, D., and Maguire, A. J. (2012). Archaea in the foregut of macropod marsupials: PCR and amplicon sequence-based observations. J. Appl. Microbiol. 113, 1065–1075. doi: 10.1111/j.1365-2672.2012.05428.x
Kohn, R. A., and Boston, R. C. (2000). “The role of thermodynamics in controlling rumen metabolism,” in Modelling Nutrient Utilization in Farm Animals, eds J. P. McNamara, J. France, and D. E. Beever, (Wallingford, CT: CABI), 11–24. doi: 10.1079/9780851994499.0011
Kolver, E. S., and Aspin, P. W. (2006). “Supplemental fumarate did not influence milksolids or methane production from dairy cows fed high quality pasture,” in Proceedings of the New Zealand Society of Animal Production (Wellington: New Zealand Society of Animal Production), 409–415.
Kristensen, N. B. (2001). Rumen microbial sequestration of [2-13C]acetate in cattle. J. Anim. Sci. 79, 2491–2498.
Kröger, A., Biel, S., Simon, J., Gross, R., Unden, G., and Lancaster, C. R. D. (2002). Fumarate respiration of Wolinella succinogenes: enzymology, energetics and coupling mechanism. Biochim. Biopys. Acta 1553, 23–38. doi: 10.1016/S0005-2728(01)00234-1
Kumar, S., Treloar, B. P., Teh, K. H., McKenzie, C. M., Henderson, G., Attwood, G. T., et al. (2018). Sharpea and Kandleria are lactic acid producing rumen bacteria that do not change their fermentation products when co-cultured with a methanogen. Anaerobe 54, 31–38. doi: 10.1016/j.anaerobe.2018.07.008
Latham, M. J., and Wolin, M. J. (1977). Fermentation of cellulose by Ruminococcus flavefaciens in the presence and absence of Methanobacterium ruminantium. Appl. Environ. Microbiol. 34, 297–301. doi: 10.1128/aem.34.3.297-301.1977
Le Van, T. D., Robinson, J. A., Ralph, J., Greening, R. C., Smolenski, W. J., Leedle, J. A. Z., et al. (1998). Assessment of reductive acetogenesis with indigenous ruminal bacterium populations and Acetitomaculum ruminis. Appl. Environ. Microbiol. 64, 3429–3436. doi: 10.1128/aem.64.9.3429-3436.1998
Lee, S. J., Song, H., and Lee, S. Y. (2006). Genome-based metabolic engineering of Mannheimia succiniciproducens for succinic acid production. Appl. Environ. Microbiol. 72, 1939–1948. doi: 10.1128/AEM.72.3.1939-1948.2006
Leng, R. A. (2014). Interactions between microbial consortia in biofilms: a paradigm shift in rumen microbial ecology and enteric methane mitigation. Anim. Prod. Sci. 54, 519–543. doi: 10.1071/AN13381
Leng, R. A., and Nolan, J. V. (1984). Nitrogen metabolism in the rumen. J. Dairy Sci. 67, 1072–1089. doi: 10.3168/jds.S0022-0302(84)81409-5
Li, X. Z., Yan, C., Choi, S. H., Long, R., Jin, G., and Song, M. (2009). Effects of addition level and chemical type of propionate precursors in dicarboxylic acid pathway on fermentation characteristics and methane production by rumen microbes in vitro. Asian Aust. J. Anim. Sci. 22, 82–89. doi: 10.5713/ajas.2009.80413
Lopez, S., McIntosh, F. M., Wallace, R. J., and Newbold, C. J. (1999). Effect of adding acetogenic bacteria on methane production by mixed rumen microorganisms. Anim. Feed Sci. Technol. 78, 1–9. doi: 10.1016/s0377-8401(98)00273-9
Lubner, C. E., and Peters, J. W. (2017). Electron bifurcation makes the puzzle pieces fall energetically into place in methanogenic energy conservation. Chembiochem 18, 2295–2297. doi: 10.1002/cbic.201700533
Lynch, T. A., Wang, Y., van Brunt, B., Pacheco, D., and Janssen, P. H. (2019). Modelling thermodynamic feedback on the metabolism of hydrogenotrophic methanogens. J. Theor. Biol. 477, 14–23. doi: 10.1016/j.jtbi.2019.05.018
Ma, Z. Y., Zhang, X. M., Wang, M., Wang, R., Jiang, Z. Y., Tan, Z. L., et al. (2019). Molecular hydrogen produced by elemental magnesium inhibits rumen fermentation and enhances methanogenesis in dairy cows. J. Dairy Sci. 102, 5566–5576. doi: 10.3168/jds.2018-15647
Mamuad, L., Kim, S. H., Jeong, C. D., Choi, Y. J., Jeon, C. O., and Lee, S.-S. (2014). Effect of fumarate reducing bacteria on in vitro rumen fermentation, methane mitigation and microbial diversity. J. Microbiol. 52, 120–128. doi: 10.1007/s12275-014-3518-1
Martin, C., Morgavi, D. P., and Doreau, M. (2010). Methane mitigation in ruminants: from microbe to the farm scale. Animal 4, 351–365. doi: 10.1017/S1751731109990620
Martinez-Fernandez, G., Denman, S. E., Cheung, J., and McSweeney, C. S. (2017). Phloroglucinol degradation in the rumen promotes the capture of excess hydrogen generated from methanogenesis inhibition. Front. Microbiol. 8:1871. doi: 10.3389/fmicb.2017.01871
Martinez-Fernandez, G., Denman, S. E., Yang, C., Cheung, J., Mitsumori, M., and McSweeney, C. S. (2016). Methane inhibition alters the microbial community, hydrogen flow, and fermentation response in the rumen of cattle. Front. Microbiol. 7:1122. doi: 10.3389/fmicb.2016.01122
Martinez-Fernandez, G., Duval, S., Kindermann, M., Schirra, H. J., Denman, S. E., and McSweeney, C. S. (2018). 3-NOP vs. halogenated compound: methane production, ruminal fermentation and microbial community response in forage fed cattle. Front. Microbiol. 9:1582. doi: 10.3389/fmicb.2018.01582
Marty, R. J., and Demeyer, D. I. (1973). The effect of inhibitors of methane production on fermentation pattern and stoichiometry in vitro using rumen contents from sheep given molasses. Br. J. Nutr. 30, 369–376. doi: 10.1079/bjn19730041
Marvin-Sikkema, F. D., Richardson, A. J., Stewart, C. S., Gottschal, J. C., and Prins, R. A. (1990). Influence of hydrogen-consuming bacteria on cellulose degradation by anaerobic fungi. Appl. Environ. Microbiol. 56, 3793–3797. doi: 10.1128/aem.56.12.3793-3797.1990
Maxin, G., Rulquin, H., and Glasser, F. (2011). Response of milk fat concentration and yield to nutrient supply in dairy cows. Animal 5, 1299–1310. doi: 10.1017/s1751731111000206
McGinn, S. M., Beauchemin, K. A., Coates, T., and Colombatto, D. (2004). Methane emissions from beef cattle: effects of monensin, sunflower oil, enzymes, yeast, and fumaric acid. J. Anim. Sci. 82, 3346–3356. doi: 10.2527/2004.82113346x
McKenzie, R. A., Rayner, A. C., Thompson, G. K., Pidgeon, G. F., and Burren, B. R. (2004). Nitrate-nitrite toxicity in cattle and sheep grazing Dactyloctenium radulans (button grass) in stockyards. Aust. Vet. J. 82, 630–634. doi: 10.1111/j.1751-0813.2004.tb12612.x
Melgar, A., Harper, M. T., Oh, J., Giallongo, F., Young, M. E., Ott, T. L., et al. (2019). Effects of 3-nitrooxypropanol on rumen fermentation, lactational performance, and resumption of ovarian cyclicity in dairy cows. J. Dairy Sci. 103, 410–432. doi: 10.3168/jds.2019-17085
Miller, T. L., and Wolin, M. J. (2001). Inhibition of growth of methane-producing bacteria of the Ruminant Forestomach by Hydroxymethylglutaryl SCoA reductase inhibitors. J. Dairy Sci. 84, 1445–1448. doi: 10.3168/jds.S0022-0302(01)70177-4
Mitchell, A. D., Chappell, A., and Knox, K. L. (1979). Metabolism of Betaine in the Ruminant. J. Anim. Sci. 49, 764–774. doi: 10.2527/jas1979.493764x
Mohammed, N., Lila, Z. A., Ajisaka, N., Hara, K., Mikuni, K., Hara, K., et al. (2004). Inhibition of ruminal microbial methane production by β-cyclodextrin iodopropane, malate and their combination in vitro. J. Anim. Physiol. Anim. Nutr. 88, 188–195. doi: 10.1111/j.1439-0396.2004.00456.x
Morgavi, D. P., Forano, E., Martin, C., and Newbold, C. J. (2010). Microbial ecosystem and methanogenesis in ruminants. Animal 4, 1024–1036. doi: 10.1017/S1751731110000546
Muñoz-Tamayo, R., Popova, M., Tillier, M., Morgavi, D. P., Morel, J. P., Fonty, G., et al. (2019). Hydrogenotrophic methanogens of the mammalian gut: functionally similar, thermodynamically different-A modelling approach. PLoS One 14:e0226243. doi: 10.1371/journal.pone.0226243
Neill, A. R., Grime, D. W., and Dawson, R. M. (1978). Conversion of choline methyl groups through trimethylamine into methane in the rumen. Biochem. J. 170, 529–535. doi: 10.1042/bj1700529
Newbold, C. J., Lopez, S., Nelson, N., Ouda, J. O., Wallace, R. J., and Moss, A. R. (2005). Propionate precursors and other metabolic intermediates as possible alternative electron acceptors to methanogenesis in ruminal fermentation in vitro. Br. J. Nutr. 94, 27–35. doi: 10.1079/bjn20051445
Nitschke, W., and Russell, M. J. (2012). Redox bifurcations: mechanisms and importance to life now, and at its origin: a widespread means of energy conversion in biology unfolds. Bioessays 34, 106–109. doi: 10.1002/bies.201100134
Nollet, L., Demeyer, D. I., and Verstraete, W. (1997). Effect of 2-bromoethanesulfonic acid and Peptostreptococcus productus ATCC 35244 addition on stimulation of reductive acetogenesis in the ruminal ecosystem by selective inhibition of methanogenesis. Appl. Environ. Microbiol. 63, 194–200. doi: 10.1128/aem.63.1.194-200.1997
Noziere, P., Martin, C., Remond, D., Kristensen, N. B., Bernard, R., and Doreau, M. (2000). Effect of composition of ruminally-infused short-chain fatty acids on net fluxes of nutrients across portal-drained viscera in underfed ewes. Br. J. Nutr. 83, 521–531. doi: 10.1017/s0007114500000660
Oba, M., and Allen, M. S. (2003). Dose-response effects of intrauminal infusion of propionate on feeding behavior of lactating cows in early or midlactation. J. Dairy Sci. 86, 2922–2931. doi: 10.3168/jds.S0022-0302(03)73889-2
Patra, A., Park, T., Kim, M., and Yu, Z. (2017). Rumen methanogens and mitigation of methane emission by anti-methanogenic compounds and substances. J. Anim. Sci. Biotechnol. 8:13. doi: 10.1186/s40104-017-0145-9
Patra, A. K., and Yu, Z. (2013). Effects of gas composition in headspace and bicarbonate concentrations in media on gas and methane production, degradability, and rumen fermentation using in vitro gas production techniques. J. Dairy Sci. 96, 4592–4600. doi: 10.3168/jds.2013-6606
Pavlostathis, S. G., Miller, T. L., and Wolin, M. J. (1990). Cellulose fermentation by continuous cultures of Ruminococcus albus and Methanobrevibacter smithii. Appl. Microbiol. Biotechnol. 33, 109–116. doi: 10.1007/bf00170581
Petersen, S. O., Hellwing, A. L., Brask, M., Hojberg, O., Poulsen, M., Zhu, Z., et al. (2015). Dietary nitrate for methane mitigation leads to nitrous oxide emissions from dairy cows. J. Environ. Qual. 44, 1063–1070. doi: 10.2134/jeq2015.02.0107
Pol, A., and Demeyer, D. I. (1988). Fermentation of methanol in the sheep rumen. Appl. Environ. Microbiol. 54, 832–834. doi: 10.1128/aem.54.3.832-834.1988
Pradhan, K., and Hemken, R. W. (1970). Utilization of ethanol and its effect on fatty acid patterns in ruminants. J. Dairy Sci. 53, 1739–1746. doi: 10.3168/jds.S0022-0302(70)86472-4
Qiao, J. Y., Tana, Z. L., Guan, L. L., Tang, S. X., Zhou, C. S., Hana, X. F., et al. (2015). Effects of hydrogen in headspace and bicarbonate in media onrumen fermentation, methane production and methanogenic population using in vitro gas production techniques. Anim. Feed Sci. Technol. 206, 19–28. doi: 10.1016/j.anifeedsci.2015.05.004
Ragsdale, S. W., and Pierce, E. (2008). Acetogenesis and the Wood–Ljungdahl pathway of CO2 fixation. Biochim. Biophys. Acta 1784, 1873–1898. doi: 10.1016/j.bbapap.2008.08.012
Raju, P. (2016). Homoacetogenesis as an Alternative Hydrogen Sink in the Rumen. Ph.D. thesis, University of Massey, Auckland.
Riede, S., Boguhn, J., and Breves, G. (2013). Studies on potential effects of fumaric acid on rumen microbial fermentation, methane production and microbial community. Arch. Anim. Nutr. 67, 368–380. doi: 10.1080/1745039x.2013.830518
Robinson, J. A., Strayer, R. F., and Tiedje, J. M. (1981). Method for measuring dissolved hydrogen in anaerobic ecosystems: application to the rumen. Appl. Environ. Microbiol. 41, 545–548. doi: 10.1128/aem.41.2.545-548.1981
Rook, J. A., Balch, C. C., Campling, R. C., and Fisher, L. J. (1963). The utilization of acetic, propionic and butyric acids by growing heifers. Br. J. Nutr. 17, 399–406. doi: 10.1079/bjn19630043
Rooke, J. A., Wallace, R. J., Duthie, C. A., McKain, N., de Souza, S. M., Hyslop, J. J., et al. (2014). Hydrogen and methane emissions from beef cattle and their rumen microbial community vary with diet, time after feeding and genotype. Br. J. Nutr. 112, 398–407. doi: 10.1017/s0007114514000932
Russell, J. B. (1991). Intracellular pH of acid-tolerant ruminal bacteria. Appl. Environ. Microbiol. 57, 3383–3384. doi: 10.1128/aem.57.11.3383-3384.1991
Russell, J. B. (1998). The importance of pH in the regulation of ruminal acetate to propionate ratio and methane production in vitro. J. Dairy Sci. 81, 3222–3230. doi: 10.3168/jds.s0022-0302(98)75886-2
Russell, J. B. (2002). Rumen Microbiology and its Role in Ruminant Nutrition. Ithaca, NY: James B Russell.
Russell, J. B., and Baldwin, R. L. (1978). Substrate preferences in rumen bacteria: evidence of catabolite regulatory mechanisms. Appl. Environ. Microbiol. 36, 319–329. doi: 10.1128/aem.36.2.319-329.1978
Russell, J. B., and Baldwin, R. L. (1979). Comparison of substrate affinities among several rumen bacteria: a possible determinant of rumen bacterial competition. Appl. Environ. Microbiol. 37, 531–536. doi: 10.1128/aem.37.3.531-536.1979
Russell, J. B., and Wallace, R. J. (1997). “Energy-yielding and energy-consuming reactions,” in The Rumen Microbial Ecosystem, eds P. N. Hobson and C. S. Stewart, (London: Blackie Academic & Professional), 246–282. doi: 10.1007/978-94-009-1453-7_6
Sauer, F. D., and Teather, R. M. (1987). Changes in oxidation reduction potentials and volatile fatty acids production by rumen bacteria when methane synthesis is inhibited. J. Dairy Sci. 70, 1835–1840. doi: 10.3168/jds.s0022-0302(87)80222-9
Scheller, H. V., and Ulvskov, P. (2010). Hemicelluloses. Annu. Rev. Plant Biol. 61, 263–289. doi: 10.1146/annurev-arplant-042809-112315
Schulman, M. D., and Valentino, D. (1976). Factors influencing rumen fermentation: effect of hydrogen on formation of propionate. J. Dairy Sci. 59, 1444–1451. doi: 10.3168/jds.s0022-0302(76)84383-4
Seeliger, S., Janssen, P. H., and Schink, B. (2002). Energetics and kinetics of lactate fermentation to acetate and propionate via methylmalonyl-CoA or acrylyl-CoA. FEMS Microbiol. Lett. 211, 65–70. doi: 10.1111/j.1574-6968.2002.tb11204.x
Shabat, S. K., Sasson, G., Doron-Faigenboim, A., Durman, T., Yaacoby, S., Berg Miller, M. E., et al. (2016). Specific microbiome-dependent mechanisms underlie the energy harvest efficiency of ruminants. ISME J. 10, 2958–2972. doi: 10.1038/ismej.2016.62
Sheperd, A. C., and Combs, D. K. (1998). Long-term effects of acetate and propionate on voluntary feed intake by midlactation cows. J. Dairy Sci. 81, 2240–2250. doi: 10.3168/jds.s0022-0302(98)75803-5
Slyter, L. L. (1979). Monensin and dichloroacetamide influences on methane and volatile fatty acid production by rumen bacteria in vitro. Appl. Environ. Microbiol. 37, 283–288. doi: 10.1128/aem.37.2.283-288.1979
Smolenski, W. J., and Robinson, J. A. (1988). In situ rumen hydrogen concentrations in steers fed eight times daily, measured using a mercury reduction detector. FEMS Microbiol. Ecol. 4, 95–100. doi: 10.1111/j.1574-6968.1988.tb02652.x
Snelling, T. J., and Wallace, R. J. (2017). The rumen microbial metaproteome as revealed by SDS-PAGE. BMC Microbiol. 17:9. doi: 10.1186/s12866-016-0917-y
Söllinger, A., Tveit, A. T., Poulsen, M., Noel, S. J., Bengtsson, M., Bernhardt, J., et al. (2018). Holistic assessment of rumen microbiome dynamics through quantitative metatranscriptomics reveals multifunctional redundancy during key steps of anaerobic feed degradation. mSystems 3:e00038-18. doi: 10.1128/mSystems.00038-18
Søndergaard, D., Pedersen, C. N. S., and Greening, C. (2016). HydDB: a web tool for hydrogenase classification and analysis. Sci. Rep. 6:34212. doi: 10.1038/srep34212
Stanier, G., and Davies, A. (1981). Effects of the antibiotic monensin and an inhibitor of methanogenesis on in vitro continuous rumen fermentations. Br. J. Nutr. 45, 567–578. doi: 10.1079/bjn19810135
Storm, A. C., Kristensen, N. B., and Hanigan, M. D. (2012). A model of ruminal volatile fatty acid absorption kinetics and rumen epithelial blood flow in lactating Holstein cows. J. Dairy Sci. 95, 2919–2934. doi: 10.3168/jds.2011-4239
St-Pierre, B., Cersosimo, L. M., Ishaq, S. L., and Wright, A. D. (2015). Toward the identification of methanogenic archaeal groups as targets of methane mitigation in livestock animals. Front. Microbiol. 6:776. doi: 10.3389/fmicb.2015.00776
Tatsuoka, N., Hara, K., Mikuni, K., Hara, K., Hashimoto, H., and Itabashi, H. (2008). Effects of the essential oil cyclodextrin complexes on ruminal methane production in vitro. Anim. Sci. J. 79, 68–75. doi: 10.1111/j.1740-0929.2007.00499.x
Thauer, R. K., Jungermann, K., and Decker, K. (1977). Energy conservation in chemotrophic anaerobic bacteria. Bacteriol. Rev. 41, 100–180. doi: 10.1128/mmbr.41.1.100-180.1977
Thauer, R. K., Kaster, A. K., Seedorf, H., Buckel, W., and Hedderich, R. (2008). Methanogenic archaea: ecologically relevant differences in energy conservation. Nat. Rev. Microbiol. 6, 579–591. doi: 10.1038/nrmicro1931
Ungerfeld, E. M. (2013). A theoretical comparison between two ruminal electron sinks. Front. Microbiol. 4:319. doi: 10.3389/fmicb.2013.00319
Ungerfeld, E. M. (2015a). Limits to dihydrogen incorporation into electron sinks alternative to methanogenesis in ruminal fermentation. Front. Microbiol. 6:1272. doi: 10.3389/fmicb.2015.01272
Ungerfeld, E. M. (2015b). Shifts in metabolic hydrogen sinks in the methanogenesis-inhibited ruminal fermentation: a meta-analysis. Front. Microbiol. 6:37. doi: 10.3389/fmicb.2015.00037
Ungerfeld, E. M. (2018). Inhibition of rumen methanogenesis and ruminant productivity: a meta-analysis. Front. Vet. Sci. 5:113. doi: 10.3389/fvets.2018.00113
Ungerfeld, E. M., Aedo, M. F., Martínez, E. D., and Saldivia, M. (2019). Inhibiting methanogenesis in Rumen batch cultures did not increase the recovery of metabolic hydrogen in microbial amino acids. Microorganisms 7:115. doi: 10.3390/microorganisms7050115
Ungerfeld, E. M., and Kohn, R. A. (2006). “The role of thermodynamics in the control of ruminal fermentation,” in Ruminant Physiology, eds K. Sejrsen, T. Hvelplund, and M. O. Nielsen, (Wageningen: Wageningen Academic Publishers), 55–85.
Ungerfeld, E. M., Rust, S. R., and Burnett, R. (2003). Use of some novel alternative electron sinks to inhibit ruminal methanogenesis. Reprod. Nutr. Dev. 43, 189–202. doi: 10.1051/rnd:2003016
Ungerfeld, E. M., Rust, S. R., and Burnett, R. (2006). Effects of butyrate precursors on electron relocation when methanogenesis is inhibited in ruminal mixed cultures. Lett. Appl. Microbiol. 42, 567–572.
Urrutia, N., Bomberger, R., Matamoros, C., and Harvatine, K. J. (2019). Effect of dietary supplementation of sodium acetate and calcium butyrate on milk fat synthesis in lactating dairy cows. J. Dairy Sci. 102, 5172–5181. doi: 10.3168/jds.2018-16024
Van Lingen, H. J., Edwards, J. E., Vaidya, J. D., Van Gastelen, S., Saccenti, E., Van den Bogert, B., et al. (2017). Diurnal dynamics of gaseous and dissolved metabolites and microbiota composition in the bovine rumen. Front. Microbiol. 8:425. doi: 10.3389/fmicb.2017.00425
Van Lingen, H. J., Fadel, J. G., Moraes, L. E., Bannink, A., and Dijkstra, J. (2019). Bayesian mechanistic modeling of thermodynamically controlled volatile fatty acid, hydrogen and methane production in the bovine rumen. J. Theor. Biol. 480, 150–165. doi: 10.1016/j.jtbi.2019.08.008
Van Lingen, H. J., Plugge, C. M., Fadel, J. G., Kebreab, E., Bannink, A., and Dijkstra, J. (2016). Thermodynamic driving force of hydrogen on rumen microbial metabolism: a theoretical investigation. PLoS One 11:e0161362. doi: 10.1371/journal.pone.0161362
Van Zijderveld, S. M., Gerrits, W. J. J., Apajalahti, J. A., Newbold, J. R., Dijkstra, J., Leng, R. A., et al. (2010). Nitrate and sulfate: effective alternative hydrogen sinks for mitigation of ruminal methane production in sheep. J. Dairy Sci. 93, 5856–5866. doi: 10.3168/jds.2010-3281
Vanwonterghem, I., Evans, P. N., Parks, D. H., Jensen, P. D., Woodcroft, B. J., Hugenholtz, P., et al. (2016). Methylotrophic methanogenesis discovered in the archaeal phylum Verstraetearchaeota. Nat. Microbiol. 1:16170. doi: 10.1038/nmicrobiol.2016.170
Vercoe, J. E., and Blaxter, K. L. (1965). The metabolism of formic acid in sheep. Br. J. Nutr. 19, 523–530. doi: 10.1079/bjn19650047
Vyas, D., Alazzeh, A., McGinn, S. M., McAllister, T. A., Harstad, O. M., Holo, H., et al. (2016). Enteric methane emissions in response to ruminal inoculation of Propionibacterium strains in beef cattle fed a mixed diet. Anim. Prod. Sci. 56, 1035–1040. doi: 10.1071/AN14801
Vyas, D., McGeough, E. J., McGinn, S. M., McAllister, T. A., and Beauchemin, K. A. (2014a). Effect of Propionibacterium spp. on ruminal fermentation, nutrient digestibility, and methane emissions in beef heifers fed a high-forage diet. J. Anim. Sci. 92, 2192–2201. doi: 10.2527/jas.2013-7492
Vyas, D., McGeough, E. J., Mohammed, R., McGinn, S. M., McAllister, T. A., and Beauchemin, K. A. (2014b). Effects of Propionibacterium strains on ruminal fermentation, nutrient digestibility and methane emissions in beef cattle fed a corn grain finishing diet. Animal 8, 1807–1815. doi: 10.1017/s1751731114001657
Waldrip, H. M., and Martin, S. A. (1993). Effects of an Aspergillus oryzae fermentation extract and other factors on lactate utilization by the ruminal bacterium Megasphaera elsdenii. J. Anim. Sci. 71, 2770–2776. doi: 10.2527/1993.71102770x
Wallace, R. J., Onodera, R., and Cotta, M. A. (1997). “Metabolism of nitrogen-containing compounds,” in The Rumen Microbial Ecosystem, eds P. N. Hobson, and C. S. Stewart, (London: Blackie Academic & Professional), 283–328. doi: 10.1007/978-94-009-1453-7_7
Wang, M., Ungerfeld, E. M., Wang, R., Zhou, C. S., Basang, Z. Z., Ao, S. M., et al. (2016). Supersaturation of dissolved hydrogen and methane in rumen of Tibetan sheep. Front. Microbiol. 7:850. doi: 10.3389/fmicb.2016.00850
Wang, M., Wang, R., Zhang, X. M., Ungerfeld, E. M., Long, D., Mao, H. X., et al. (2017). Molecular hydrogen generated by elemental magnesium supplementation alters rumen fermentation and microbiota in goats. Br. J. Nutr. 118, 401–410. doi: 10.1017/S0007114517002161
Wang, R., Wang, M., Ungerfeld, E. M., Zhang, X. M., Long, D. L., Mao, H. X., et al. (2018). Nitrate improves ammonia incorporation into rumen microbial protein in lactating dairy cows fed a low-protein diet. J. Dairy Sci. 101, 9789–9799. doi: 10.3168/jds.2018-14904
Weiss, W. P. (2017). A 100-year review: from ascorbic acid to zinc—Mineral and vitamin nutrition of dairy cows. J. Dairy Sci. 100, 10045–10060. doi: 10.3168/jds.2017-12935
Wenner, B. A., de Souza, J., Batistel, F., Hackmann, T. J., Yu, Z., and Firkins, J. L. (2017). Association of aqueous hydrogen concentration with methane production in continuous cultures modulated to vary pH and solids passage rate. J. Dairy Sci. 100, 5378–5389. doi: 10.3168/jds.2016-12332
Wolin, M. J., Miller, T. L., and Stewart, C. S. (1997). “Microbe-microbe interactions,” in The Rumen Microbial Ecosystem, eds P. N. Hobson, and C. S. Stewart, (London: Blackie Academic & Professional), 467–491.
Wood, T. A., Wallace, R. J., Rowe, A., Price, J., Yáñez-Ruiz, D. R., Murray, P., et al. (2009). Encapsulated fumaric acid as a feed ingredient to decrease ruminal methane emissions. Anim. Feed Sci. Technol. 152, 62–71. doi: 10.1016/j.anifeedsci.2009.03.006
Yang, C. J., Mao, S. Y., Long, L. M., and Zhu, W. Y. (2012). Effect of disodium fumarate on microbial abundance, ruminal fermentation and methane emission in goats under different forage: concentrate ratios. Animal 6, 1788–1794. doi: 10.1017/s1751731112000857
Zheng, Y., Kahnt, J., Kwon, I. H., Mackie, R. I., and Thauer, R. K. (2014). Hydrogen formation and its regulation in Ruminococcus albus: involvement of an electron-bifurcating [FeFe]-hydrogenase, of a non-electron-bifurcating [FeFe]-hydrogenase, and of a putative hydrogen-sensing [FeFe]-hydrogenase. J. Bacteriol. 196, 3840–3852. doi: 10.1128/jb.02070-14
Keywords: rumen, hydrogen, redox, fermentation, microorganisms, metabolism, kinetics, thermodynamics
Citation: Ungerfeld EM (2020) Metabolic Hydrogen Flows in Rumen Fermentation: Principles and Possibilities of Interventions. Front. Microbiol. 11:589. doi: 10.3389/fmicb.2020.00589
Received: 19 January 2020; Accepted: 18 March 2020;
Published: 15 April 2020.
Edited by:
Amlan Kumar Patra, West Bengal University of Animal and Fishery Sciences, IndiaReviewed by:
Roderick Ian Mackie, University of Illinois at Urbana-Champaign, United StatesCopyright © 2020 Ungerfeld. This is an open-access article distributed under the terms of the Creative Commons Attribution License (CC BY). The use, distribution or reproduction in other forums is permitted, provided the original author(s) and the copyright owner(s) are credited and that the original publication in this journal is cited, in accordance with accepted academic practice. No use, distribution or reproduction is permitted which does not comply with these terms.
*Correspondence: Emilio M. Ungerfeld, ZW1pbGlvLnVuZ2VyZmVsZEBpbmlhLmNs
Disclaimer: All claims expressed in this article are solely those of the authors and do not necessarily represent those of their affiliated organizations, or those of the publisher, the editors and the reviewers. Any product that may be evaluated in this article or claim that may be made by its manufacturer is not guaranteed or endorsed by the publisher.
Research integrity at Frontiers
Learn more about the work of our research integrity team to safeguard the quality of each article we publish.