- 1Department of Respiratory and Critical Care Medicine, Chronic Airways Diseases Laboratory, Huiqiao Medical Center, Nanfang Hospital, Southern Medical University, Guangzhou, China
- 2The Second Clinical College of Guangzhou University of Chinese Medicine, Guangzhou, China
- 3Department of Intensive Care Unit, Fangcun Branch of Guangdong Provincial Hospital of Chinese Medicine, Guangzhou, China
- 4Guangdong Provincial Academy of Chinese Medical Sciences, Guangzhou, China
- 5Department of Respiratory, Longhua District People’s Hospital, Shenzhen, China
- 6Huiqiao Medical Center, Nanfang Hospital, Southern Medical University, Guangzhou, China
- 7Department of Medicine, Division of Infectious Diseases, Rhode Island Hospital, Warren Alpert Medical School of Brown University, Providence, RI, United States
Candida albicans is the most common fungal pathogen and relies on the Hog1-MAPK pathway to resist osmotic stress posed by the environment or during host invasions. Here, we investigated the role of SPT20 in response to osmotic stress. Testing a C. albicans spt20Δ/Δ mutant, we found it was sensitive to osmotic stress. Using sequence alignment, we identified the conserved functional domains between CaSpt20 and ScSpt20. Reconstitution of the Spt20 function in a spt20Δ/CaSPT20 complemented strain found CaSPT20 can suppress the high sensitivity to hyperosmotic stressors, a cell wall stress agent, and antifungal drugs in the Saccharomyces cerevisiae spt20Δ/Δ mutant background. We measured the cellular glycerol accumulation and found it was significantly lower in the C. albicans spt20Δ/Δ mutant strain, compared to the wild type strain SC5314 (P < 0.001). This result was also supported by quantitative reverse transcription-PCR, which showed the expression levels of gene contributing to glycerol accumulation were reduced in Caspt20Δ/Δ compared to wild type (GPD2 and TGL1, P < 0.001), while ADH7 and AGP2, whose expression can lead to glycerol decrease, were induced when cells were exposed to high osmolarity (ADH7, P < 0.001; AGP2, P = 0.002). In addition, we tested the transcription levels of Hog1-dependent osmotic stress response genes, and found that they were significantly upregulated in wild type cells encountering hyperosmolarity, while the expression of HGT10, SKO1, CAT1, and SLP3 were not induced when SPT20 was deleted. Although the transcript of ORF19.3661 and ORF19.4370 in Caspt20Δ/Δ was induced in the presence of 1 M NaCl, the levels were less than what was observed in the wild type (ORF19.3661, P = 0.007; ORF19.4370, P = 0.011). Moreover, the deletion of CaSPT20 in C. albicans reduced phosphorylation levels of Hog1. These findings suggested that SPT20 is conserved between yeast and C. albicans and plays an important role in adapting to osmotic stress through regulating Hog1-MAPK pathway.
Introduction
Candida albicans can be isolated from oral-pharyngeal, gastrointestinal, and urogenital tracts (Calderone and Fonzi, 2001), and has emerged as one of the most common causes of nosocomial bloodstream infections (Wisplinghoff et al., 2004). In order to cause colonization and infection, this successful opportunistic pathogen has to overcome environmental challenges, such as host immune defenses, nutrient limitation, competition with resident microbiota, and physiological extremes including: pH, osmotic, and oxidative stresses (Calderone and Fonzi, 2001; Marotta et al., 2013; Dong et al., 2015). C. albicans has developed a series of complex mechanisms to respond to these challenges.
The high osmolarity glycerol 1 mitogen activated protein kinase signaling transduction pathway, also known as the Hog1-MAPK pathway, can regulate responses to oxidative, osmotic, and heavy metal stress (Enjalbert et al., 2006). Therefore, the Hog1 signal transduction pathway is crucial for C. albicans cells during exposure to stressors encountered during pathogenesis (Alonso-Monge et al., 1999). When cells encounter hyperosmotic conditions, they rapidly trigger the Hog1-MAPK pathway to regulate Hog1-dependent osmotic stress response genes, and the synthesis and accumulation of glycerol. Glycerol is an important compatible cellular solute. When cells encounter osmotic challenge, they can make a comparable change in glycerol content to offset the increasing external osmolarity, thus buffering the osmotic change to maintain normal cell volume and enable survival (Reed et al., 1987).
This important pathway can be influenced by other genes. SPT20, an important component of the SAGA complex, helps to maintain the structural integrity of the SAGA complex (Grant et al., 1997; Sterner et al., 1999), controls about 10% of gene expression (Lee et al., 2000), and is highly conserved in eukaryote cells (Sellam et al., 2009). The interaction between Spt20 and Hog1 is essential for osmotic adaption (Zapater et al., 2007). More specifically, when cells were subjected to osmotic stress, Hog1 was activated and bound to osmostress promoters, then recruited SAGA complex components (including Spt20). Further experiment showed Hog1 co-precipitated the Spt20, suggesting that Hog1 associates with Spt20 (Zapater et al., 2007). The activation of human Hog1 did not correlate with an increased recruitment of hSpt20 subunit under endoplasmic reticulum stress (Nagy et al., 2009). However, p38IP, the human ortholog of the yeast Spt20, can directly bind to p38 and is required for the activation of the mammalian ortholog of Hog1 (Zohn et al., 2006). These previous studies indicate that further work is still needed to explore the interaction between Spt20 and Hog1.
In our previous research, we reported that SPT20 was involved in toleration to high osmotic stress, revealing it was associated with C. albicans virulence (Tan et al., 2014). However, the association between CaSPT20 and Hog1-MAPK signaling pathway in C. albicans is still poorly understood. In this study, we perform quantitative reverse transcription-PCR (qRT-PCR) and western blotting to interrogate the relationship between SPT20 and Hog1-MAPK pathway in C. albicans. We describe the conserved role of SPT20 between S. cerevisiae and C. albicans and report, for the first time, that SPT20 takes part in the C. albicans response to hyperosmotic stress by regulating the Hog1-MAPK pathway.
Materials and Methods
Yeast Strains and Growth Conditions
Wild type C. albicans strain SC5314, the spt20Δ/Δ null mutant, the spt20Δ/SPT20 reconstituted strain, and S. cerevisiae wild type BY4741 were grown in YPD medium (1% yeast extract, 2% peptone, 2% dextrose) at 30°C with shaking. The Saccharomyces cerevisiae spt20Δ mutant strain (LCT1) was cultured in YPD medium supplemented with G418 (Sigma-Aldrich, Shanghai, China). Ampicillin-resistant E. coli was cultured in LB medium with 100 μg/mL ampicillin at 37°C. Strains with pYES-CaSPT20-V5 or pYES2.1/V5-His-TOPO plasmids were cultured in Sc-Ura3 media. All strains were cultured to logarithmic growth stage.
C. albicans and S. cerevisiae strains used in this study are listed in Table 1. All C. albicans strains were derived from the wild type strain SC5314. All S. cerevisiae strains were derived from the wild type BY4741.
Plasmid Construction
All primers and plasmids used in this study are listed in Tables 2, 3, respectively. For the creation of plasmid pYES-CaSPT20-V5, SC5314 genomic DNA was used as a template for CaSPT20ResFwd and CaSPT20ResRev primers, which generated a 2,678 bp DNA fragment containing BamHI and BstEII restriction sites, the promoter, ORF of CaSPT20 but lacked the stop codon.
The amplified fragment described above and plasmid pYES2.1/V5-His/lacZ (Invitrogen, Shanghai, China) were digested with BamHI-HF and BstEII-HF. The two products were purified, ligated, and the resulting plasmid was transformed to DH5α E. coli and colonies were selected on LB plate with 100 μg/mL ampicillin. PCR followed by sequencing were used to validate the correct insertion of pYES-CaSPT20-V5-His/lacZ vector (Supplementary Data).
Generated Strains
Saccharomyces cerevisiae LCT1 was constructed as previously described (Marotta et al., 2013). The template plasmid pFA6a-5FLAG-KanMX6 was a gift from Eishi Noguchi (Noguchi et al., 2008; Addgene plasmid # 15983; http://n2t.net/addgene:15983; RRID: Addgene 15983). In brief, we used ScSPT20DelFwd and ScSPT20DelRev as primers and the plasmid pFA6a-5FLAG-KanMX6 as template to amplify a 1,676 bp DNA fragment containing the kanamycin resistance gene flanked by 20 bp of ScSPT20 5′ and 3′ sequences. The PCR product was transferred to S. cerevisiae wild type strain BY4741 using a transformation method described previously by Gietz (Gietz, 2014). Transformants with the desired insert were selected on YPD media containing 200 μg/mL G418 and verified by PCR (Marotta et al., 2013). The LCT1 and BY4741 strains were transformed with the pYES2.1/V5-His-TOPO vector to generate LCT2 and LCT4 strains, respectively. To create strain LCT3 (Scspt20Δ/CaSPT20), the pYES-CaSPT20-V5 plasmid was transformed to LCT1. All strains were verified by PCR to ensure the correct transformants were used.
The construction of overexpression strains was described previously (Nobile et al., 2008). The NAT1-TDH3 promoter plasmid pCJN542 (Nobile et al., 2008) was used for gene overexpression. To construct the SPT20 overexpression strain (SPT20-OE) in hog1Δ/Δ mutant background, the PCR product was amplified using the plasmid pCJN542 as template and primers SPT20-OEF and SPT20-OER (Table 2) and then transferred to hog1Δ/Δ mutant strain. By the same method, the PCR product generated using plasmid pCJN542 as template for and primers HOG1-OEF and HOG1-OER (Table 2) was transferred to spt20Δ/Δ mutant strain to generate HOG1 overexpression strain (HOG1-OE). Strains that underwent homologous recombination were selected on YPD+ Nourseothricin (Werner BioAgents, Jena, Germany; 400 μg/mL for SPT20-OE strain and 100 μg/mL for HOG1-OE strain) plates and the recombination events were verified by PCR with primers SPT20-F-2 and NAT1-R for SPT20-OE strain, and primers HOG1-F-2 and NAT1-OER-det for HOG1-OE strain, respectively. Function of this overexpression strategy was verified by real-time PCR with primers HOG1-F and HOG1-R for HOG1-OE strain, and primers SPT20-F and SPT20-R for SPT20-OE strain, respectively.
Sensitivity Assays
Sensitivity to a range of stresses was evaluated using a solid media assay. All investigational strains were grown to mid-log phase under suitable growth conditions and collected by centrifugation. The pellets were suspended in YPD at 2.5 × 107 cells/mL. Ten-fold serial dilutions from 2.5 × 107 to 2.5 × 103 of all strains were prepared, and 4 μL of each of strain dilutions was spotted onto the agar plates with integrated stimuli. Cells were incubated at 30°C for 48 h and then observed for growth differences.
RNA Isolation and qRT-PCR Analysis
The C. albicans strains SC5314, Caspt20Δ/Δ, Caspt20Δ/SPT20 were cultured to logarithmic phase and diluted to OD600 = 0.2. The cultures were incubated at 30°C with shaking for 4 h. 5 × 107 cells were counted with a hemocytometer and then collected by centrifugation. After being washed twice with sterile PBS, the pellets were subjected to 1 M NaCl in YPD, while the control group was added to an equal volume of YPD medium. All the cultures were grown at 30°C with shaking for an additional 30 min. After treatment, cells were collected by gentle centrifugation, and total RNA was extracted using an RNeasy Mini Kit (Qiagen, Shanghai, China) according to the manufacturer’s protocol. The concentration, purity, and integrity of RNA were checked by Nanodrop spectrophotometer. Generally, RNA samples with an A260/A280 ratio between 1.9 and 2.1 were used for further interrogation.
In order to remove potential genomic DNA contamination and synthesize cDNA, we used PrimeScriptTM RT reagent Kit with gDNA Eraser (Perfect Real Time; TaKaRa, Dalian, China) following the manufacturer’s protocol. Real time reactions were prepared using TB GreenTM Premix Ex Taq IITM Kit (Tli RNaseH Plus; TaKaRa, Dalian, China), and quantitative PCR experiments were conducted in a LightCycler480 System (Roche, Switzerland). Transcript levels were normalized against 18S rRNA expression (used as an internal control of gene expression). The gene expression changes were measured in 2–ΔΔCt method. Fold changes of target genes in the spt20 mutant and reconstituted strains were normalized to the untreated wild type strain.
Intracellular Glycerol Assays
The C. albicans production of intracellular glycerol were measured as previously described (Ene et al., 2015). In brief, C. albicans strains SC5314, Caspt20Δ/Δ, and Caspt20Δ/SPT20 were grown overnight. An aliquot of 5 × 107 cells were treated with 1 M NaCl in YPD for 30 min. Subsequently, the intracellular glycerol levels were measured using the Free Glycerol Reagent (Sigma-Aldrich, Shanghai, China) according to the manufacturer’s protocol.
Western Blotting
Candida albicans wild type strain SC5314 and null mutant strain Caspt20Δ/Δ were grown to mid-exponential phase in YPD at 30°C with shaking. Cells were exposed to hyperosmotic stress for a designated period of time by adding 5 M NaCl stock solution to YPD medium to achieve a final concentration of 2 M NaCl. As a control, equal volume of YPD was added instead of NaCl. Following treatment, C. albicans cells were collected and the pellets were washed twice with sterile PBS. To extract protein, pellets were suspended in 200 μL RIPA lysis buffer containing Protease Inhibitor Cocktail (Roche, Shanghai, China), and an equal volume of acid-washed glass beads (Sigma-Aldrich, shanghai, China) was added. The cells were vigorously vortexed for 1 min to mechanically disrupt cell walls then transferred to ice for 1 min, and vortex and chill process repeated six more times. Cell extracts were separated from whole cell debris and glass beads by applying centrifugation at 13,000 rpm at 4°C for 10 min.
The protein concentrations were determined using a Pierce BCA Protein Assay Kit (Thermo Fisher Scientific, Shanghai, China). Equal quantities of protein (40 μg) were loaded onto a 10% gel, analyzed by SDS-PAGE, and then transferred to PVDF membranes. Anti-phospho-P38 antibody (Cell Signaling Technology, Shanghai, China) was used to detect the phosphorylated form of Hog1 (Smith et al., 2004). Total Hog1 level was detected by Hog1 (D-3) antibody (Santa Cruz Biotechnology, Shanghai, China). β-anti-actin antibody (GeneTex, Shenzhen, China) was used as the loading control (Deng and Lin, 2018).
Statistical Analysis
All experiments were performed at least twice as independent replicates. Data were analyzed using SPSS software. Student’s t-test and the analyses of variance (ANOVA) were used to determine statistical significance. A P-value < 0.05 was considered statistically significant.
Results
Conservation of CaSPT20
We have previously reported that SPT20 was involved in regulating virulence and stress responses in C. albicans (Tan et al., 2014). However, little is known about the underlying molecular mechanisms. The amino acid sequence alignment showed there are conserved functional domains between CaSpt20 and ScSpt20 (Supplementary Figure 4). With the hypothesis that C. albicans SPT20 could be functionally conserved with Saccharomyces cerevisiae, we endeavored to determine if CaSPT20 could restore defects in ScSPT20 mutant strains. To this end, we constructed S. cerevisiae strains LCT1 (Scspt20Δ), LCT2 (pYES2.1/V5-His-TOPO in the LCT1 background), LCT3 (pYES2.1/V5-His-TOPO-CaSPT20 in the LCT1 background) and LCT4 (pYES2.1/V5-His-TOPO in the background of the wild type strain BY4741), then performed a series of functional complement assays.
The strains were grown on YPD agar plates supplemented with hyperosmotic stressors (NaCl, sorbitol, and glycerol), ethanol stress, cell wall stress agent SDS, or antifungal agents (amphotericin B, fluconazole, and caspofungin), which directly perturb cell membrane component ergosterol synthesis or FKS required for cell wall synthesis. After cultivation for 48 h, cell growth was observed under the applied stress conditions. The introduction of plasmid pYES2.1/V5-His-TOPO had no influence on cell growth, as seen when comparing growth of LCT4 to BY4741, and LCT2 to LCT1. Deletion of SPT20 impaired normal cell growth of S. cerevisiae, which was in agreement with the results reported by Roberts and Winston (Roberts and Winston, 1996), but growth retardation was exacerbated when cells were associated with the tested hyperosmotic stressors and cell membrane targeting antifungal agent fluconazole. Notably, the cell growth of Scspt20Δ mutant was rescued with complementation of CaSPT20, suggesting that SPT20 is required for the normal cell growth under extracellular osmolarity and cell membrane stressor exposure. However, the decrease in resistance to the other stresses (such as ethanol, SDS, amphotericin B, and caspofungin) seen in Scspt20Δ cells largely matches the decreased growth seen in the BY4741 (Figure 1), indicating that the growth defects of Scspt20Δ cells in these stresses may not be due to the deletion of SPT20. Importantly, complementation of CaSPT20 restores the growth of Scspt20Δ to the wild type levels, supporting that the function of SPT20 is conserved between C. albicans and S. cerevisiae.
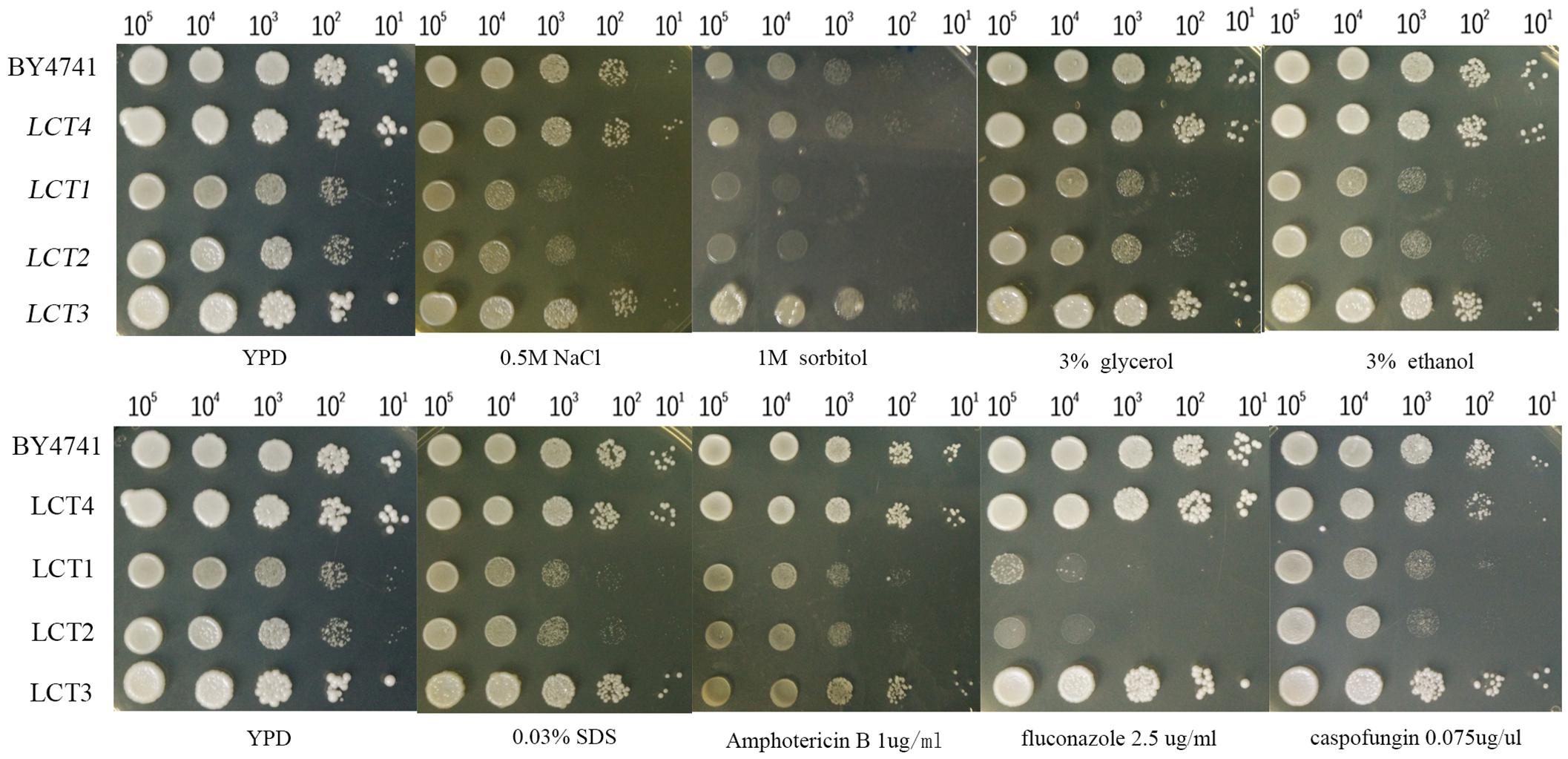
Figure 1. The introduction of CaSPT20 can restore sensitivity to stresses and antifungal agents caused by the loss of ScSPT20. LCT1 (Scspt20Δ), LCT2 (Scspt20Δ+pYES2.1/V5-His-TOPO), LCT3 (Scspt20Δ+pYES- CaSPT20-V5), and LCT4(BY4741+pYES2.1/V5-His-TOPO) were constructed in the background of S. cerevisiae BY4741 and grown at 30°C for 48 h. The experiment was repeated on 3 independent occasions.
Hog1 Phosphorylation Is Reduced by Deletion of CaSPT20
The data demonstrates that Spt20 plays a conserved role in protecting cells from osmotic stress. A well-known contributor in protecting fungi from osmotic stress is the Hog1 pathway (Brewster and Gustin, 2014). When C. albicans is exposed to high osmolarity, Hog1 is phosphorylated and then induces target gene expression to adapt to osmotic stress (Smith et al., 2004; Day et al., 2017). In other words, phosphorylation of Hog1 is the essential step for C. albicans to survive during high osmotic challenge. To test if CaSPT20 affects Hog1 responses to osmotic stress, we extracted protein from the indicated strains subjected to 2 M NaCl in YPD for various time periods, and then performed western blotting. Specific antibodies were used to detect the levels of total Hog1 and phospho-Hog1, respectively. In this assay, β-actin antibody was used as a loading control.
As reported previously (Alonso-Monge et al., 2003; Smith et al., 2004), Hog1 phosphorylation was induced by osmotic treatment. Hog1 phosphorylation peaked after 10 min under high osmotic stimulation in wild type SC5314, however, Caspt20Δ/Δ failed to have the same level of Hog1 phosphorylation after 10 min of osmotic treatment. Indeed, only a very slight increase in phosphorylated Hog1 was observed after 60 min of stimulation (Figure 2A). In stark contrast, reconstitution of SPT20 restored phospho-Hog1 to wild type levels (Figure 2B). In addition, the level of total Hog1 transcription in these three strains remained constant, which was in accord with what Enjalbert et al. reported (Enjalbert et al., 2006), indicating that Hog1 phosphorylation occurs independent of total Hog1 expression levels. Thus, it appears that SPT20 correlated with the phosphorylation of Hog1. We then further explored the effects that deletion of SPT20 has on Hog1 responses and the C. albicans phenotype.
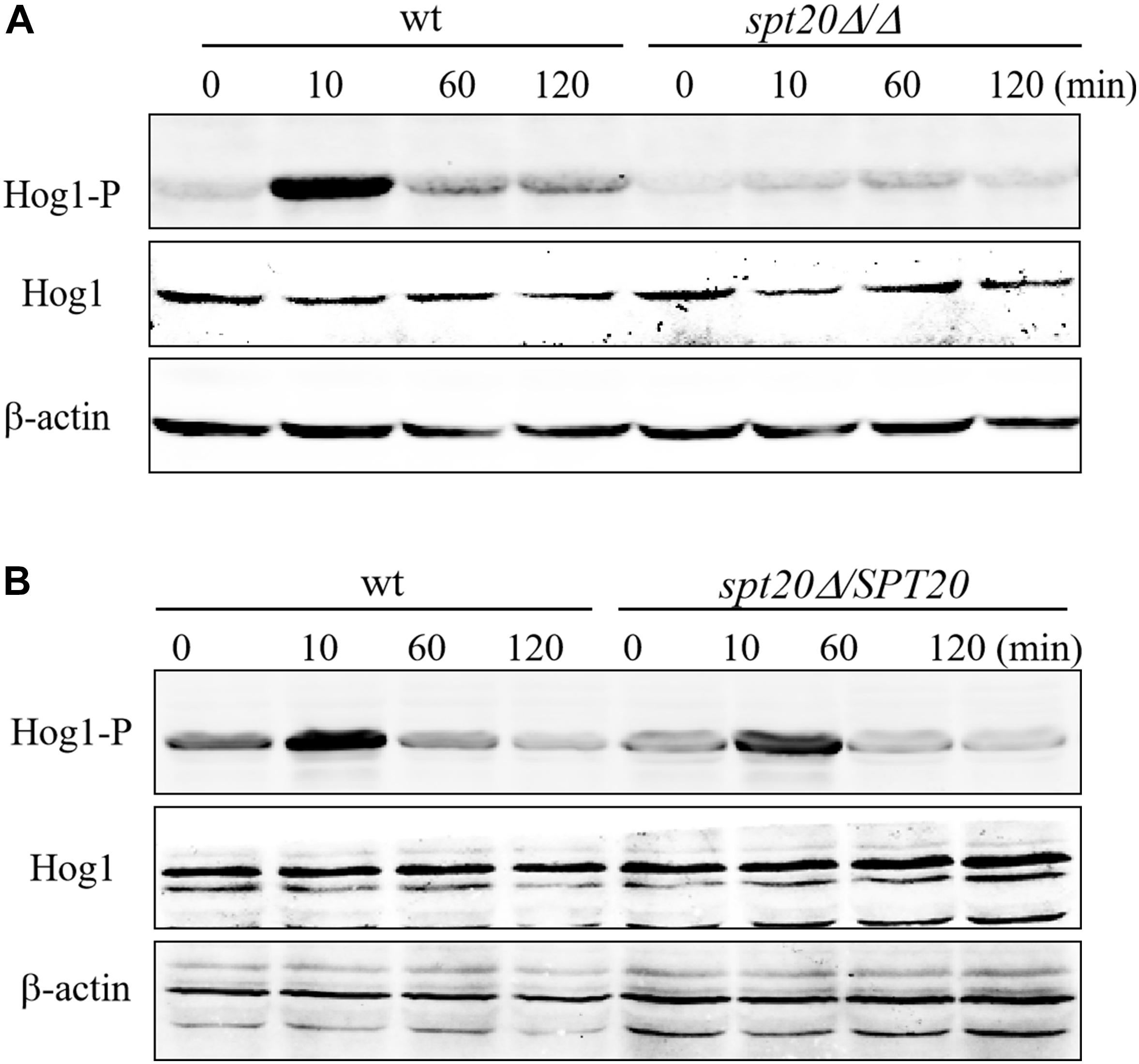
Figure 2. SPT20 affects the phosphorylation level of Hog1 protein. Cells were exposed to 2 M NaCl for the indicated time. phosphorylated Hog1 (Hog1-P) and total Hog1 (Hog1) levels of wild type, SPT20 null mutant (A), and SPT20 reconstitution strain (B) were detected by western blotting with specific antibodies. The beta actin antibody was used as the loading control. This experiment was repeated three times independently.
CaSPT20 Affects Expression of Hog1-Dependent Osmotic Stress Response Genes
As shown above, the level of phosphorylated Hog1 in Caspt20Δ/Δ was much less than what was seen in the wild type strain, suggesting that SPT20 affected the level of phosphorylated Hog1, which prompted us to investigate whether the expression levels of Hog1-dependent osmotic stress response genes were affected by the loss of SPT20. To this end, we measured the expression of Hog1-dependent osmotic stress response genes. A panel of genes was assembled for interrogation as the previous work did, which reported the expression levels of HGT10 (encoding a glycerol permease involved in active glycerol uptake), SKO1 (encoding a transcriptional factor binding to promoters to relieve osmotic stress), CAT1 (one of core stress genes, encoding a key antioxidant enzyme), ORF19.4370 (predicted ORF), ORF19.3661 (encoding a putative deubiquitinating enzyme) and SLP3 (encoding a putative cation conductance protein) were induced in a Hog1-dependent manner (Enjalbert et al., 2006; Marotta et al., 2013). As showed in Figure 3, these Hog1-dependent osmotic stress response genes were significantly upregulated in wild type cells encountering hyperosmolarity, while the expression of HGT10, SKO1, CAT1, and SLP3 were not induced when SPT20 was deleted. Although the transcript of ORF19.3661 and ORF19.4370 in Caspt20Δ/Δ was induced in the presence of 1 M NaCl, it still did not reach the level observed in the wild type (ORF19.3661, P = 0.007; ORF19.4370, P = 0.011). Notably, the transcriptional changes caused by the deletion of SPT20 gene in C. albicans were in accordance with that caused by the loss of HOG1 gene, which also exhibited reduced expression of HGT10, SKO1, CAT1, ORF19.4370, ORF19.3661, and SLP3 in the hog1Δ/Δ mutant strain during 1 M NaCl stimulation (Marotta et al., 2013). Taken together, we can conclude that SPT20 plays a role in appropriate expression of Hog1-dependent osmotic stress response genes.
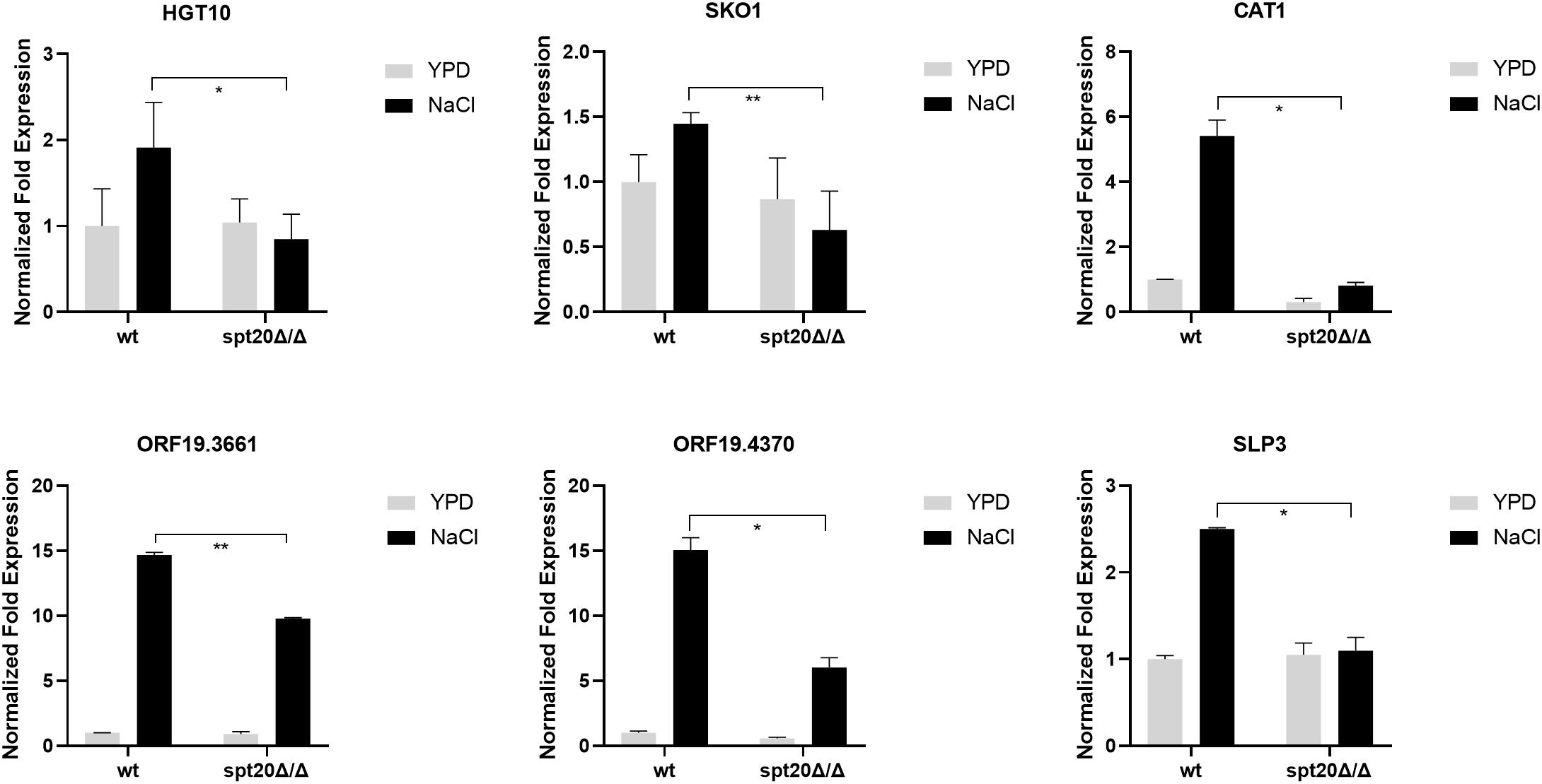
Figure 3. Expression levels of Hog1-dependent osmotic stress response genes changed significantly when SPT20 was deleted in C. albicans. qRT-PCR expression analysis of C. albicans Hog1-dependent osmotic stress response genes in the wild type strain, spt20Δ/Δ and spt20Δ/SPT20. Transcript levels were normalized to C. albicans 18S rRNA expression and fold changes between strains were normalized to untreated wild type which was adjusted to a value of 1. All genes were analyzed in triplicate. Error bar represents Mean ± SD. *P < 0.5, **P < 0.01.
The Cell Growth Defect of Caspt20Δ/Δ Is Rescued by HOG1 Overexpression Under Osmotic Stress
The Hog1-MAPK pathway has been reported to be involved in osmoadaptation, take part in resistance to oxidative stress, and also play a role in morphogenesis changes as well as cell wall biosynthesis (Monge et al., 2006). As we demonstrated earlier, the ability to overcome hyperosmotic stress was impaired in Caspt20Δ/Δ, suggesting CaSPT20 really plays a role in osmostress responses (Tan et al., 2014). To evaluate if SPT20 responds to osmotic stress in cooperation with the Hog1 pathway, cell growth of strains SC5314, Caspt20Δ/Δ, Caspt20Δ/SPT20, Cahog1Δ/Δ and Cahog1Δ/HOG1 were examined during exposure to external hyperosmolarity, comparing growth defects between Caspt20Δ/Δ and Cahog1Δ/Δ strains. As expected, the knockout of CaSPT20 or CaHOG1 both led to impaired cell growth in hyperosmotic conditions imposed by NaCl or sorbitol, and for each, growth was restored to wild type levels when CaSPT20 and CaHOG1 were reconstituted, respectively (Figure 4). This result, along with the affected phosphorylation level of Hog1 and the expression of Hog1-dependent osmotic stress response genes, suggest a link between SPT20 and Hog1-MAPK pathway in C. albicans osmoadaptation. Thus, we hypothesized that SPT20 regulated the Hog1-MAPK pathway to respond to external osmotic stress. In order to evaluate this hypothesis, we constructed the HOG1 overexpression strain in the Caspt20Δ/Δ mutant background (HOG1-OE) and in the wild type background (wt-HOG1-OE), and SPT20 overexpression strain in the Cahog1Δ/Δ mutant background (SPT20-OE). The gene overexpression was verified by quantitative reverse transcription-PCR analysis. In addition, the basal levels of phosphorylated Hog1 and total Hog1 in the HOG1 overexpression strains were significantly increased when compared with wild type (Supplementary Figure 1). We compared the cell growth of strains SC5314, Caspt20Δ/Δ, HOG1-OE, Cahog1Δ/Δ, and SPT20-OE, which were treated with NaCl. As illustrated in Figure 4, we observed that HOG1 overexpression can partially rescue the growth defect caused by SPT20 deletion when cells were exposed to a series of high osmotic stress (YPD plate supplements with 1.8 M NaCl, or 2.2 M NaCl), while overexpressing SPT20 did not confer the ability to resist hyperosmotic stress to the hog1Δ/Δ strain. Furthermore, in order to investigate whether there exists a Spt20-independent manner contributing to the increasing resistance of HOG1-OE strain, we observed the cell growth of wt-HOG1-OE strain, and found that overexpressing HOG1 did not enhance the osmotic tolerance of wild type cells (Supplementary Figure 2). These results indicate that SPT20 influences Hog1 during the C. albicans response to osmotic stress.
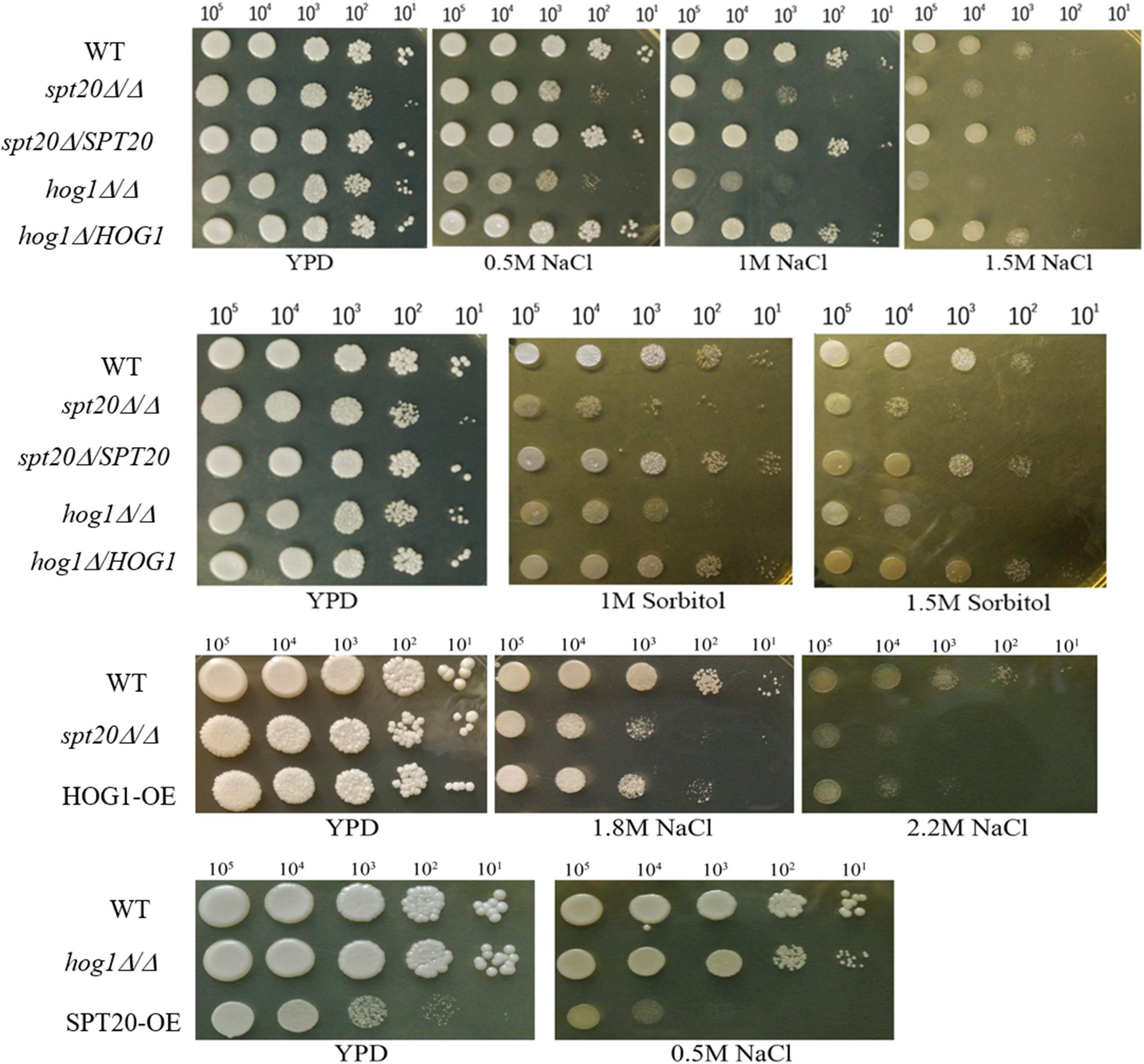
Figure 4. The cell growth defect of Caspt20Δ/Δ is similar to that of Cahog1Δ/Δ and is rescued by HOG1 overexpression. Ten-fold serial dilutions of strains were spotted on appropriate plates to evaluate the cell growth defects. Cells were grown at 30°C and then photographed. The experiment was repeated on three independent occasions.
CaSPT20 Regulates Glycerol Accumulation in C. albicans
To investigate the correlation between CaSPT20 and Hog1-MAPK pathway in hyperosmotic stress response, we measured the intracellular glycerol accumulation of strains SC5314, Caspt20Δ/Δ, and Caspt20Δ/SPT20 after exposure to 1 M NaCl for 30 min. Our results showed the basal glycerol contents of these three strains were almost the same, and they all increased strikingly under hyperosmotic condition. However, the ability to accumulate intracellular glycerol was impaired in Caspt20Δ/Δ strain (P < 0.001) (Figure 5A).
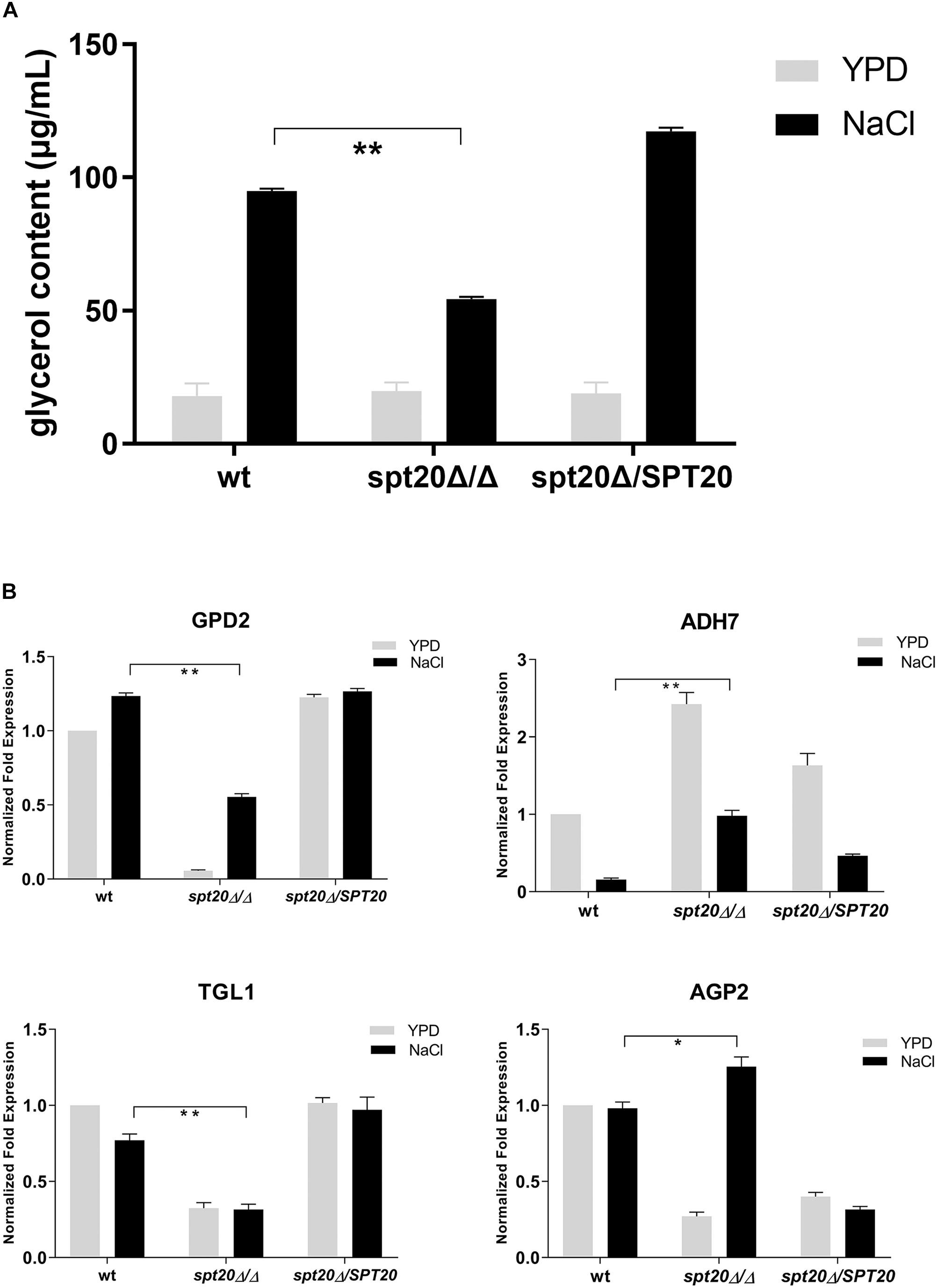
Figure 5. CaSPT20 regulates intracellular glycerol accumulation under hyperosmotic condition. (A) CaSPT20 has an important effect on intracellular glycerol content under hyperosmotic condition. 5 × 107 cells with or without treatment (1 M NaCl or YPD) were grown at 30°C for an additional 30 min and then used for glycerol measurement. The ability of spt20Δ/Δ strain to accumulate cellular glycerol under osmotic stress was impaired. (B) SPT20 affects the expression of genes involved in glycerol accumulation. qRT-PCR analysis was used to measure the expression of genes involved in glycerol accumulation following exposure to 1 M NaCl. The following C. albicans strains were evaluated: wild type SC5314, spt20Δ/Δ, and spt20Δ/SPT20 and genes were analyzed in triplicate. Expression levels were normalized to 18S rRNA. Fold changes between strains were normalized to untreated wild type, which was adjusted to a value of 1. Three independent biological replicates were conducted. Error bar represents Mean ± SD. *P < 0.01, **P < 0.001.
Glycerol biosynthesis is catalyzed by glycerol-3-phosphate dehydrogenase (encoded by GPD1/GPD2) and glycerol-3-phosphatase (encoded by RHR2) (Hohmann, 2002; Fan et al., 2005). Meanwhile, the increased cellular glycerol concentration is also forced by regulated activities of triacylglycerol lipases (encoded by TGL1/TGL2) (Wei et al., 2009), alcohol dehydrogenase (encoded by ADH) (Blomberg and Adler, 1989), and amino acid permease (encoded by AGP2) (Marotta et al., 2013). To further examine the Spt20 influence on cellular glycerol, we examined expression of these genes involved in glycerol accumulation. GPD2, which was reported to increase to a greater extent than GPD1 and RHR2 levels in response to osmostress (Smith et al., 2004; Enjalbert et al., 2006; Jacobsen et al., 2018), was suppressed when CaSPT20 was knocked out. Although GPD2 was induced in the presence of 1 M NaCl, its expression still did not reach the level observed in the wild type (P < 0.001). In contrast, ADH7 was significantly induced in the Caspt20Δ/Δ mutant, both in the absence and presence of osmotic stress (6-fold in the presence of 1 M NaCl compared to wild type; P < 0.001). TGL1 was under-expressed in Caspt20Δ/Δ after exposure to osmotic stress (0.31 for the mutant versus 0.76 for wt strain; P < 0.001). When compared to wild type, AGP2, a gene involved in membrane permeability, experienced reduced expression in the absence of salt exposure in the Caspt20Δ/Δ strain, but following osmotic stress treatment, it significantly elevated and was 1.25-fold higher than the expression found in the wild type strain (P = 0.002) (Figure 5B). The decreased expression of GPD2 can reduce the accumulation of intercellular glycerol directly. The expression of ADH7 can reduce the yield of NADH, then lead to the decreasing production of glycerol (Blomberg and Adler, 1989). Meanwhile, the reduced transcript level of TGL1 blocks the process of transforming triglycerides to glycerol (Jandrositz et al., 2005; Wei et al., 2009), while the upregulation of AGP2 can cause increasing membrane permeability, so that the intracellular glycerol can spread to extracellular environments. Taken together, these findings suggest that CaSPT20 participates in the process of glycerol accumulation, since GPD2, ADH7, TGL1 and AGP2 expression were sharply affected when SPT20 was deleted from C. albicans.
Discussion
Osmoregulation by homeostatic mechanisms is crucial in C. albicans in order to keep appropriate cell volume, turgor, as well as a suitable intracellular environment for all kinds of biochemical reactions (Hohmann et al., 2007; Fuchs and Mylonakis, 2009). In this paper, we show that CaSpt20 has functional similarity with ScSpt20 and can be used to reconstitute a mutation in the homologous gene. The increased sensitivity of Caspt20Δ/Δ to hyperosmolarity is due to its reduced phosphorylation levels of Hog1, thereby causing downregulation of osmotic stress response genes and decrease in glycerol accumulation, suggesting that SPT20 is involved in resistance to high osmolarity. These findings give us new insight into the role of SPT20 in C. albicans response to osmotic stress, and indicate a new relationship between Spt20 and Hog1.
As an indispensable component of the SAGA complex, SPT20 has gained enough attention on its function. It was reported that Spt20 was involved in endoplasmic reticulum stress response in human (Nagy et al., 2009), hypoxic response (Hickman et al., 2011) and the functional interaction between other SAGA components and TBP in yeast (Roberts and Winston, 1997), and the calcineurin-mediated Cl– homeostasis in Schizosaccharomyces pombe (Zhou et al., 2013). Furthermore, SPT20 is required for normal cell growth (Roberts and Winston, 1996) and is essential for yeast survival at high osmolarity (Zapater et al., 2007). Here, we demonstrated that knockout of ScSPT20 caused significantly further growth defects associated with the tested hyperosmotic stressors (NaCl and sorbitol) compared to a wild type control exposed to the same conditions. Additionally, the ability of Caspt20Δ/Δ mutant strain to resist hyperosmolarity was greatly impaired (Figure 4). These results suggested that SPT20 is required for the normal cell growth under osmotic condition, which was in accord with the previous work that reported SPT20 is essential for yeast survival at high osmolarity (Zapater et al., 2007). The similar phenotypes between Scspt20Δ and Caspt20Δ/Δ mutant strain, and increasing resistance to osmotic stress due to the complement of CaSPT20, supported that the function of SPT20 was conserved.
The Hog1-MAPK pathway is critical for C. albicans to respond to osmotic stress. Hog1, the core component in this pathway, has a strong functional preservation from yeast to mammals (Sheikh-Hamad and Gustin, 2004), and its rapid phosphorylation is an essential step in osmotic toleration (Brewster and Gustin, 2014). Our findings suggest that Spt20 regulates Hog1 activation in C. albicans response to hyperosmotic stress. The evidences are the following. First, the cell growth defect of Caspt20Δ/Δ was similar to that of Cahog1Δ/Δ (Figure 4). Second, the phosphorylation level of Hog1 was significantly decreased because of the absence of SPT20 (Figure 2). Our western blotting result showed that, as reported previously (Smith et al., 2004), Hog1 was rapidly but transiently phosphorylated during C. albicans salt exposure. However, phosphorylation levels were comparably lower in Caspt20Δ/Δ, suggesting that CaSPT20 affected the process of Hog1 phosphorylation. Hog1 phosphorylation is a dynamic event (Alonso-Monge et al., 2003; Smith et al., 2004). The kinetics of phosphorylation were different in these two strains: wild type strain peaked at about 10 min after exposure to stress, while the mutant strain peaked at about 60 min. However, in both wild type and mutant strains, the peak levels of phospho-Hog1 were diminished over time. Third, overexpressing HOG1 in the spt20Δ/Δ mutant background can partially rescue the growth defect when spt20Δ/Δ mutant strain was exposed to osmotic stress, while overexpressing SPT20 in the hog1Δ/Δ mutant background was not able to restore its ability to respond to hyperosmolarity (Figure 4). Meanwhile, the HOG1-OE strain demonstrated higher basal level of phosphorylated Hog1 and total Hog1 protein than wild type and spt20Δ/Δ mutant strain (Figure 2 and Supplementary Figure 1B), which may contribute to tolerate its osmostress, since C. albicans regulates the phosphorylation of Hog1 to respond to hyperosmolarity (Smith et al., 2004). Though the basal phosphorylated Hog1 level of wt-HOG1-OE strain was enhanced as well, overexpression of HOG1 did not increase the resistance to osmolarity in wild type. Our working hypothesis is that the phospho-Hog1 level in wild type may be similar to that in wt-HOG1-OE strain under osmotic exposure which leads to similar cell growth. Additionally, overexpression HOG1 did not revert the growth defect of Caspt20Δ/Δ mutant strain to a level comparable to the wild type, suggesting that besides the Hog1-MAPK pathway, there may exist another mechanism that accounts for SPT20 response to osmotic stress. Strikingly, SPT20 overexpression in the hog1Δ/Δ mutant background reduced the cell growth in the YPD plate. This phenotype was not due to the changes in the shape of fungal cells, since the SPT20-OE strain cells grew as unicellular yeast and the shape was similar to that of wild type and hog1Δ/Δ mutant strain (Supplementary Figure 3). SPT20 is crucial for the structural integrity of SAGA complex (Grant et al., 1997; Sterner et al., 1999), thus, overexpressing SPT20 may change the structure of SAGA complex, which would hamper the normal gene expression and then impair normal cell growth.
We noticed that, compared to wild type, the transcript level of genes involved in glycerol accumulation was either reduced or induced in the Caspt20Δ/Δ mutant strain (Figure 5B). However, the changing patterns of these genes were similar to that of wild type. When C. albicans cells were subjected to osmotic stress, the activation of the Hog1-MAPK pathway can regulate the synthesis and accumulation of glycerol (San José et al., 1996; Monge et al., 2006), along with up-regulation of genes contributing to increase the intercellular glycerol levels and down-regulation of genes contributing to reduce the glycerol levels. Although the magnitude of Hog1 activation was significantly decreased in Caspt20Δ/Δ mutant strain (Figure 2A), the reduced phosphorylated Hog1 can still induce or repress the related gene expression to cope with osmolarity. Strikingly, the fold induction of GPD2 in Caspt20Δ/Δ mutant strain is greater than that in wild type. We hypothesized that the repressed expression of ADH7 and TGL1, together with the up-regulation of AGP2 contribute to the decrease of intracellular glycerol, which may in turn lead to a greater fold induction of GPD2 in Caspt20Δ/Δ mutant strain response to osmotic stress. Furthermore, in contrast to the induction of GPD2 expression reported previously (Enjalbert et al., 2006; Marotta et al., 2013), there was no increase in GPD2 expression in wild type cells when osmotic stress was imposed. However, the transcript level of GPD2 is dynamic and related to the incubation time upon osmotic stress (Enjalbert et al., 2006) and the C. albicans wild type strains used in these studies were different, thus we speculated that the incubation time and the wild type strains were associated with the different GPD2 expression.
Our study has limitations that need to be taken into account and addressed in the future. First, although the growth defects of Scspt20Δ were rescued with complementation of CaSPT20 (Figure 1), C. albicans SPT20 gene was not codon optimized prior to expression in S. cerevisiae, which should be noted as a limitation in our study because it may lead to mistranslation. Also, in future work, we plan to evaluate a Caspt20Δ/hog1Δ double mutant strain to further assess genetic epistasis between SPT20 and HOG1.
Conclusion
In conclusion, we confirm that SPT20 is functionally conserved between S. cerevisiae and C. albicans, and report that SPT20 plays a critical role in C. albicans response to hyperosmotic stress through regulating Hog1-MAPK pathway, through both expression and phosphorylation (Figure 6). The reduced Hog1 phosphorylation in Caspt20Δ/Δ mutant can explain its high sensitivity to osmotic stress, indicating a relationship between Spt20 and Hog1 in the response to altered osmotic conditions.
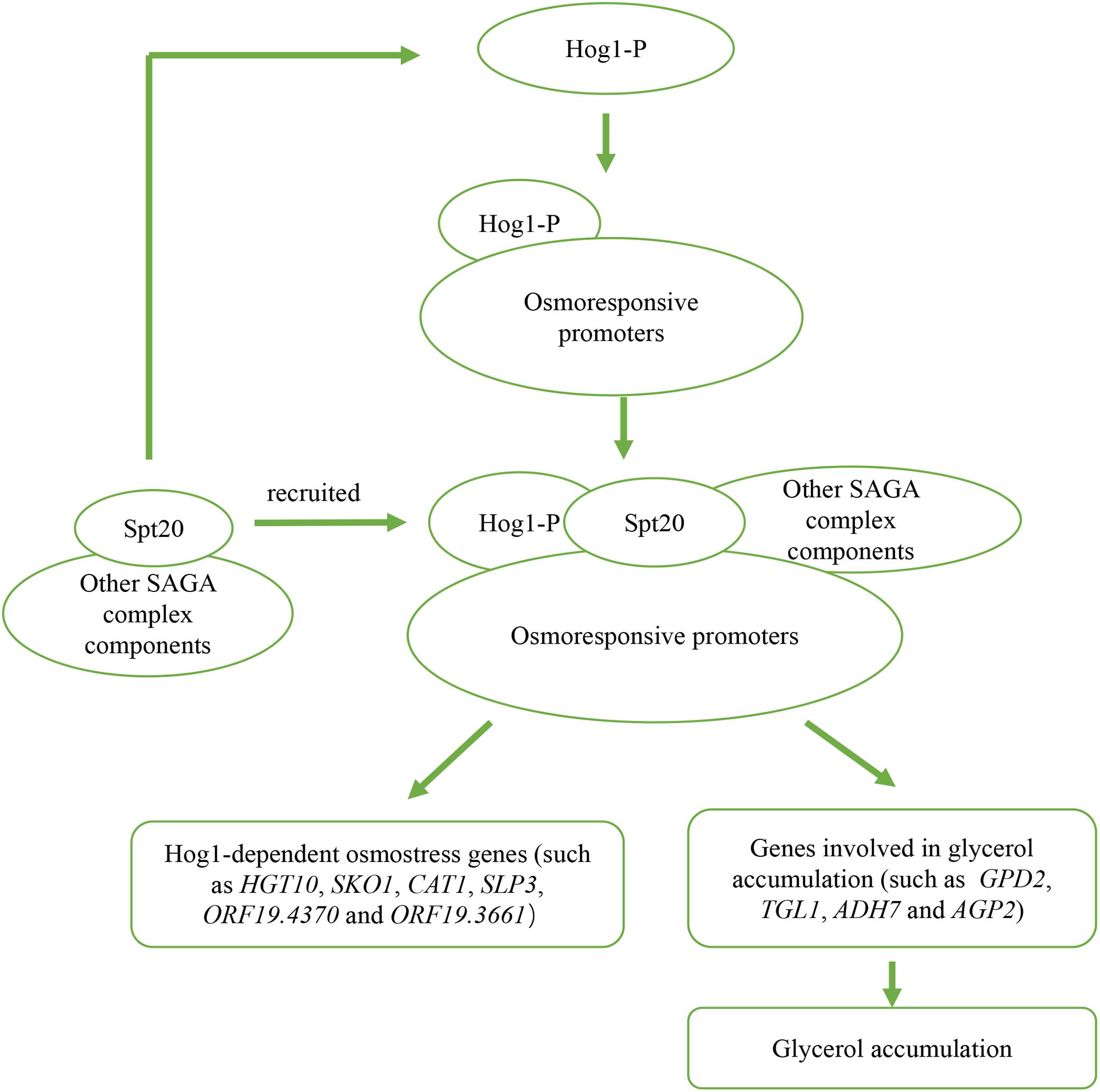
Figure 6. Outline of two potential mechanisms of Spt20 in C. albicans response to osmotic stress. Previous work found that when cells are subjected to high osmolarity, Hog1 is activated and bind to osmostress promoters, then recruits SAGA complex components to induce expression of Hog1-dependent osmostress response genes and genes involved in glycerol accumulation. In our study, we found that Spt20 regulates the phosphorylation of Hog1 in response to osmotic stress.
Data Availability Statement
All datasets generated for this study are included in the article/Supplementary Material.
Author Contributions
RC, LW, BF, XT, and EM designed the study. LW, RC, SL, and QW performed the experiments. SL, HW, and LL helped to analyze the data. LW, BF, XT, and EM wrote the manuscript. XT contributed to reagents and materials, and supervised the project.
Funding
This work was supported by the Science and Technology Planning Project of Guangdong Province, China (Grant 2015A050502026) and National Natural Science Foundation of China (Grant 81570012) to XT. The funders had no role in study design, data collection and analysis, decision to publish, or preparation of the manuscript. All other authors have no financial disclosures.
Conflict of Interest
The authors declare that the research was conducted in the absence of any commercial or financial relationships that could be construed as a potential conflict of interest.
Acknowledgments
We thank Ching-Hsuan Lin for providing the C. albicans hog1Δ/Δ mutant strain and the hog1Δ/HOG1 strain (Liang et al., 2014), Aaron P. Mitchell for providing plasmid pCJN542, and Eishi Noguchi for providing plasmid pFA6a-5FLAG-KanMX6 (Addgene plasmid # 15983).
Supplementary Material
The Supplementary Material for this article can be found online at: https://www.frontiersin.org/articles/10.3389/fmicb.2020.00213/full#supplementary-material
References
Alonso-Monge, R., Navarro-García, F., Molero, G., Diez-Oreias, R., Gustin, M., Pla, J., et al. (1999). Role of the mitogen-activated protein kinase Hog1p in morphogenesis and virulence of Candida albicans. J. Bacteriol. 181, 3058–3068. doi: 10.1128/jb.181.10.3058-3068.1999
Alonso-Monge, R., Navarro-García, F., Román, E., Negredo, A. I., Eisman, B., Nombela, C., et al. (2003). The Hog1 Mitogen-Activated Protein Kinase Is Essential in the Oxidative Stress Response and Chlamydospore Formation in Candida albicans. Eukaryot. Cell. 2, 351–361. doi: 10.1128/ec.2.2.351-361.2003
Blomberg, A., and Adler, L. (1989). Roles of glycerol and glycerol-3-phosphate dehydrogenase (n.d.) in acquired osmotolerance of Saccharomyces cerevisiae. J. Bacteriol. 171, 1087–1092. doi: 10.1128/jb.171.2.1087-1092.1989
Brewster, J. L., and Gustin, M. C. (2014). Hog1: 20 years of discovery and impact. Sci. Signal. 7:re7. doi: 10.1126/scisignal.2005458
Calderone, R. A., and Fonzi, W. A. (2001). Virulence factors of Candida albicans. Trends. Microbiol. 9, 327–335. doi: 10.1016/S0966-842X(01)02094-7
Day, A. M., Herrero-de-Dios, C. M., MacCallum, D. M., Brown, A. J. P., and Quinn, J. (2017). Stress-induced nuclear accumulation is dispensable for Hog1-dependent gene expression and virulence in a fungal pathogen. Scientif. Rep. 7, 14340–14340. doi: 10.1038/s41598-017-14756-4
Deng, F. S., and Lin, C. H. (2018). Cpp1 phosphatase mediated signaling crosstalk between Hog1 and Cek1 mitogen-activated protein kinases is involved in the phenotypic transition in Candida albicans. Med. Mycol. 56, 242–252. doi: 10.1093/mmy/myx027
Dong, Y., Yu, Q., Chen, Y., Xu, N., Zhao, Q., Jia, C., et al. (2015). The Ccz1 mediates the autophagic clearance of damaged mitochondria in response to oxidative stress in Candida albicans. Int. J. Biochem. Cell. Biol. 69, 41–51. doi: 10.1016/j.biocel.2015.10.002
Ene, I. V., Walker, L. A., Schiavone, M., Lee, K. K., Martin-Yken, H., Daque, E., et al. (2015). Cell wall remodeling enzymes modulate fungal cell wall elasticity and osmotic stress resistance. MBio 6:e00986. doi: 10.1128/mBio.00986-15
Enjalbert, B., Smith, D. A., Cornell, M. J., Alam, I., Nicholls, S., Brown, A. J., et al. (2006). Role of the Hog1 stress-activated protein kinase in the global transcriptional response to stress in the fungal pathogen Candida albicans. Mol. Biol. Cell. 17, 1018–1032. doi: 10.1091/mbc.e05-06-0501
Fan, J., Whiteway, M., and Shen, S. H. (2005). Disruption of a gene encoding glycerol 3-phosphatase from Candida albicans impairs intracellular glycerol accumulation-mediated salt-tolerance. FEMS Microbiol. lett. 245, 107–116. doi: 10.1016/j.femsle.2005.02.031
Fuchs, B. B., and Mylonakis, E. (2009). Our paths might cross: the role of the fungal cell wall integrity pathway in stress response and cross talk with other stress response pathways. Eukaryot. Cell. 8, 1616–1625. doi: 10.1128/EC.00193-09
Gietz, R. D. (2014). “Yeast transformation by the LiAc/SS carrier DNA/PEG method,” in The Yeast Genetics, Methods in Molecular Biology, ed Edn, eds J. Smith, and D. Burke, (New York, NY: Humana Press), 1–12. doi: 10.1007/978-1-4939-1363-3_1
Grant, P. A., Duggan, L., Côté, J., Roberts, S. M., Brownell, J. E., Candau, R., et al. (1997). Yeast Gcn5 functions in two multisubunit complexes to acetylate nucleosomal histones: characterization of an Ada complex and the SAGA (Spt/Ada) complex. Genes. Dev. 11, 1640–1650. doi: 10.1101/gad.11.13.1640
Hickman, M. J., Spatt, D., and Winston, F. (2011). The Hog1 mitogen-activated protein kinase mediates a hypoxic response in Saccharomyces cerevisiae. Genetics 188, 325–338. doi: 10.1534/genetics.111.128322
Hohmann, S. (2002). Osmotic stress signaling and osmoadaptation in yeasts. Microbiol. Mol. Biol. Rev. 66, 300–372. doi: 10.1128/mmbr.66.2.300-372.2002
Hohmann, S., Krantz, M., and Nordlander, B. (2007). Yeast osmoregulation. Methods Enzymol. 428, 29–45. doi: 10.1016/S0076-6879(07)28002-4
Jacobsen, M. D., Beynon, R. J., Gethings, L. A., Claydon, A. J., Langridge, J. I., Vissers, J. P. C., et al. (2018). Specificity of the osmotic stress response in Candida albicans highlighted by quantitative proteomics. Scientif. Rep. 8, 14492–14492. doi: 10.1038/s41598-018-32792-6
Jandrositz, A., Petschnigg, J., Zimmermann, R., Natter, K., Scholze, H., Hermetter, A., et al. (2005). The lipid droplet enzyme Tgl1p hydrolyzes both steryl esters and triglycerides in the yeast, Saccharomyces cerevisiae. Biochim. Biophys. Acta 1735, 50–58. doi: 10.1016/j.bbalip.2005.04.005
Lee, T. I., Causton, H. C., Holstege, F. C., Shen, W. C., Hannett, N., Jennings, E. G., et al. (2000). Redundant roles for the TFIID and SAGA complexes in global transcription. Nature 405, 701–704. doi: 10.1038/35015104
Liang, S. H., Cheng, J. H., Deng, F. S., Tsai, P. A., and Lin, C. H. (2014). A novel function for Hog1 stress-activated protein kinase in controlling white-opaque switching and mating in Candida albicans. Eukaryot. Cell. 13, 1557–1566. doi: 10.1128/EC.00235-14
Marotta, D. H., Nantel, A., Sukala, L., Teubl, J. R., and Rauceo, J. M. (2013). Genome-wide transcriptional profiling and enrichment mapping reveal divergent and conserved roles of Sko1 in the Candida albicans osmotic stress response. Genomics 102, 363–371. doi: 10.1016/j.ygeno.2013.06.002
Monge, R. A., Román, E., Nombela, C., and Pla, J. (2006). The MAP kinase signal transduction network in Candida albicans. Microbiology 152, 905–912. doi: 10.1099/mic.0.28616-0
Nagy, Z., Riss, A., Romier, C., le Guezennec, X., Dongre, A. R., Orpinell, M., et al. (2009). The human SPT20-containing SAGA complex plays a direct role in the regulation of endoplasmic reticulum stress-induced genes. Mol. Cell. Biol. 29, 1649–1660. doi: 10.1128/MCB.01076-08
Nobile, C. J., Solis, N., Myers, C. L., Fay, A. J., Deneault, J. S., Nantel, A., et al. (2008). Candida albicans transcription factor Rim101 mediates pathogenic interactions through cell wall functions. Cell. Microbiol. 10, 2180–2196. doi: 10.1111/j.1462-5822.2008.01198.x
Noguchi, C., Garabedian, M. V., Malik, M., and Noguchi, E. (2008). A vector system for genomic FLAG epitope-tagging in Schizosaccharomyces pombe. Biotechnol. J. 3, 1280–1285. doi: 10.1002/biot.200800140
Reed, R. H., Chudek, J. A., Foster, R., and Gadd, G. M. (1987). Osmotic significance of glycerol accumulation in exponentially growing yeasts. Appl. Environ. Microbiol. 53, 2119–2123. doi: 10.1128/aem.53.9.2119-2123.1987
Roberts, S. M., and Winston, F. (1996). SPT20/ADA5 encodes a novel protein functionally related to the TATA-binding protein and important for transcription in Saccharomyces cerevisiae. Mol. Cell. Biol. 16, 3206–3213. doi: 10.1128/mcb.16.6.3206
Roberts, S. M., and Winston, F. (1997). Essential functional interactions of SAGA, a Saccharomyces cerevisiae complex of Spt, Ada, and Gcn5 proteins, with the Snf/Swi and Srb/mediator complexes. Genetics 147, 451–465.
San José, C., Monge, R. A., Pérez-Díaz, R., Pla, J., and Nombela, C. (1996). The mitogen-activated protein kinase homolog HOG1 gene controls glycerol accumulation in the pathogenic fungus Candida albicans. J. Bacteriol. 178, 5850–5852. doi: 10.1128/jb.178.19.5850-5852.1996
Sellam, A., Askew, C., Epp, E., Lavoie, H., Whiteway, M., and Nantel, A. (2009). Genome-wide mapping of the co-activator Ada2p yields insight into the functional roles of SAGA/ADA complex in Candida albicans. Mol. Biol. Cell. 20, 2389–2400. doi: 10.1091/mbc.e08-11-1093
Sheikh-Hamad, D., and Gustin, M. C. (2004). MAP kinases and the adaptive response to hypertonicity: functional preservation from yeast to mammals. Am. J. Physiol. Renal Physiol. 287, F1102–10. doi: 10.1152/ajprenal.00225.2004
Smith, D. A., Nicholls, S., Morgan, B. A., Brown, A. J., and Quinn, J. (2004). A conserved stress-activated protein kinase regulates a core stress response in the human pathogen Candida albicans. Mol. Biol. Cell. 15, 4179–4190. doi: 10.1091/mbc.e04-03-0181
Sterner, D. E., Grant, P. A., Roberts, S. M., Duggan, L. J., Belotserkovskaya, R., Pacella, L. A., et al. (1999). Functional organization of the yeast SAGA complex: distinct components involved in structural integrity, nucleosome acetylation, and TATA-binding protein interaction. Mol. Cell. Biol. 19, 86–98. doi: 10.1128/MCB.19.1.8610.1091/mbc.e05-06-0501
Tan, X., Fuchs, B. B., Wang, Y., Chen, W., Yuen, G. J., Chen, R. B., et al. (2014). The role of Candida albicans SPT20 in filamentation, biofilm formation and pathogenesis. PLoS One 9:e94468. doi: 10.1371/journal.pone.0094468
Wei, M., Fabrizio, P., Madia, F., Hu, J., Ge, H., Li, L. M., et al. (2009). Tor1/Sch9-regulated carbon source substitution is as effective as calorie restriction in life span extension. PLoS Genet. 5:e1000467. doi: 10.1371/journal.pgen.1000467
Wisplinghoff, H., Bischoff, T., Tallent, S. M., Seifert, H., Wenzel, R. P., and Edmond, M. B. (2004). Nosocomial bloodstream infections in US hospitals: analysis of 24,179 cases from a prospective nationwide surveillance study. Clin. Infect. Dis. 39, 309–317. doi: 10.1086/421946
Zapater, M., Sohrmann, M., Peter, M., Posas, F., and de Nadal, E. (2007). Selective requirement for SAGA in Hog1-mediated gene expression depending on the severity of the external osmostress conditions. Mol. Cell. Biol. 27, 3900–3910. doi: 10.1128/MCB.00089-07
Zhou, N., Lei, B. K., Zhou, X., Yu, Y., and Lv, H. (2013). Yi chuan = Hereditas. SJR 35, 1135–1142. doi: 10.3724/sp.j.1005.2013.01135
Keywords: Candida albicans, glycerol, Hog1-MAPK, osmotic stress, SPT20
Citation: Wang L, Chen R, Weng Q, Lin S, Wang H, Li L, Fuchs BB, Tan X and Mylonakis E (2020) SPT20 Regulates the Hog1-MAPK Pathway and Is Involved in Candida albicans Response to Hyperosmotic Stress. Front. Microbiol. 11:213. doi: 10.3389/fmicb.2020.00213
Received: 25 October 2019; Accepted: 30 January 2020;
Published: 21 February 2020.
Edited by:
Dominique Sanglard, Université de Lausanne, SwitzerlandReviewed by:
Adnane Sellam, Laval University, CanadaJan Quinn, Newcastle University, United Kingdom
Copyright © 2020 Wang, Chen, Weng, Lin, Wang, Li, Fuchs, Tan and Mylonakis. This is an open-access article distributed under the terms of the Creative Commons Attribution License (CC BY). The use, distribution or reproduction in other forums is permitted, provided the original author(s) and the copyright owner(s) are credited and that the original publication in this journal is cited, in accordance with accepted academic practice. No use, distribution or reproduction is permitted which does not comply with these terms.
*Correspondence: Xiaojiang Tan, dHhqemRrQDEyNi5jb20=
†These authors have contributed equally to this work
‡These authors share senior authorship