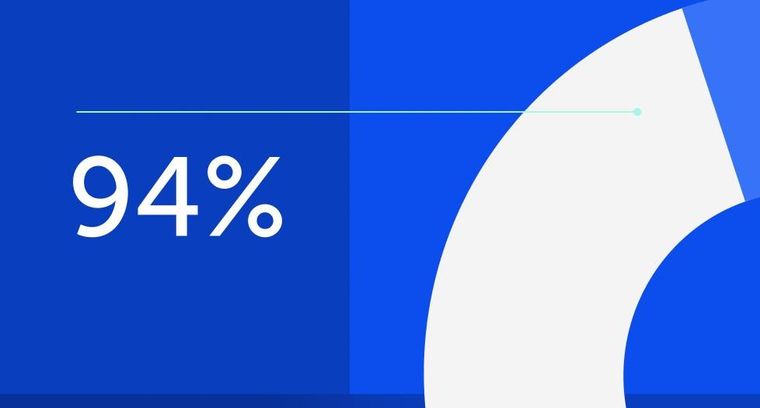
94% of researchers rate our articles as excellent or good
Learn more about the work of our research integrity team to safeguard the quality of each article we publish.
Find out more
REVIEW article
Front. Microbiol., 14 February 2020
Sec. Microbiotechnology
Volume 11 - 2020 | https://doi.org/10.3389/fmicb.2020.00188
Phytases are a group of enzymes that hydrolyze the phospho-monoester bonds of phytates. Phytates are one of the major forms of phosphorus found in plant tissues. Fungi are mainly used for phytase production. The production of fungal phytases has been achieved under three different fermentation methods including solid-state, semi-solid-state, and submerged fermentation. Agricultural residues and other waste materials have been used as substrates for the evaluation of enzyme production in the fermentation process. Nutrients, physical conditions such as pH and temperature, and protease resistance are important factors for increasing phytase production. Fungal phytases are considered monomeric proteins and generally possess a molecular weight of between 14 and 353 kDa. Fungal phytases display a broad substrate specificity with optimal pH and temperature ranges between 1.3 and 8.0 and 37–67°C, respectively. The crystal structure of phytase has been studied in Aspergillus. Notably, thermostability engineering has been used to improve relevant enzyme properties. Furthermore, fungal phytases are widely used in food and animal feed additives to improve the efficiency of phosphorus intake and reduce the amount of phosphorus in the environment.
Phytic acid is known as myo-inositol (1, 2, 3, 4, 5, 6) hexakisphosphate or phytate in salt form, as is shown in Figure 1. It is the major form of storage for phosphorus in plant tissues such as those in cereal grains, oilseeds, pollen, and legumes (Lott et al., 2000). Cereal grains and oilseed meals are major ingredients in animal feed as they are known sources of phosphorus, an essential macro-element required for animal growth (Selle and Ravindran, 2008). However, phosphorus in seeds exists predominately in the form of phytates (salt of phytic acid), and phytate phosphorus is not available to monogastric animals because they possess very low levels of phytase activity in their digestive tracts (Brinch-Pedersen et al., 2002; Vohra and Satyanarayana, 2003). Therefore, phosphate supplementation is required for optimal animal growth (Chen et al., 2008). However, a large amount of undigested phytate phosphorus is excreted along with animal waste and this is known to cause algal blooms and eutrophication in surface waters. Fungal phytases are widely produced in fermentation processes and are commonly used to overcome the nutritional and environmental problems caused by phytates. Currently, phytases are being utilized as a major animal feed additive (Mullaney et al., 2000).
Phytases (myo-inositol hexakisphosphate phosphohydrolases) are a class of phosphatases that catalyze the hydrolysis of phytates to myo-inositol, inositol phosphate, and inorganic phosphates (Wodzinski and Ullah, 1996; Wyss et al., 1998). Phytases were first identified by Suzuki et al. (1907) who found an enzyme present in rice bran. Moreover, phytases are widespread in nature and can be produced from various host sources including plants, animals, and microorganisms (Yao et al., 2011). Based on their catalytic function and structure, the first and most extensively studied group of phytases are classified as histidine acid phosphatases (HAPs) that have been isolated from filamentous fungi, bacteria, yeasts, and plants (Mullaney et al., 2000). Phytases have been commonly detected in many fungal species and are most often characterized by their presence in those fungal species (Mukhametzyanova et al., 2012; Singh and Satyanarayana, 2014). However, the physico-chemical characteristics and catalytic properties of phytases depend upon the different fungal strains that serve as their source. Thus, the phytase production of fungi is dependent upon differing optimum temperatures and pH values that range from neutral to acidic (pH 1–6) or alkaline (pH 8–14) (Yao et al., 2011; Singh and Satyanarayana, 2014). Aspergillus has been most commonly employed for phytase production. Thus, the first generation of commercially available fungal phytase obtained from A. niger was marketed in 1991 and has been applied for use in various industries ever since, such as in the production of human food and animal feed as well as in the preparation of myo-inositol phosphates. Furthermore, phytases have also been used in the semi-synthesis of peroxidase employed in the paper and pulp industries and as a soil amendment and plant growth promoter (Singh et al., 2011). Several fungal strains have been extensively studied for phytase production, purification, characterization and stability, cloning and expression. Consequently, their potential biotechnological applications have been reported (Yao et al., 2011; Lei et al., 2013). The summarization of phytases is shown in Figure 2. This review addresses the properties and potential biotechnological applications of fungal phytases.
Phytases are produced in nature in a wide range of plant and animal tissues and microorganisms such as bacteria, yeast, and fungi (Vohra and Satyanarayana, 2003). Most scientific works have focused on microbial phytases, particularly those obtained from filamentous fungi such as Aspergillus, Myceliophthora, Mucor, Penicillium, Rhizopus, and Trichoderma (Tseng et al., 2000; Sabu et al., 2002; Roopesh et al., 2006; Dailin et al., 2019). Aspergillus ficuum NRRL 3135 has been defined as the most active fungal phytase producer and has most commonly been employed at the commercial level (Chelius and Wodzinski, 1994). Other filamentous fungal species that can produce phytase during the fermentation process are A. carbonarius, A. fumigatus, A. niger, A. oryzae, Cladosporium species, Mucor piriformis, and Rhizopus oligosporus (Howson and Davis, 1983; Casey and Walsh, 2004; Quan et al., 2004; Salmon et al., 2012). Moreover, the phytase activity of some edible mushrooms, such as Agaricus bisporus, Agrocybe pediades, Ceriporia sp., Ganoderma stipitatum, Grifola frondosa, Lentinula edodes, Peniophora lycii, Pleurotus cornucopiae, Schizophyllum commune and Trametes pubescens, has also been reported (Lassen et al., 2001; Collopy and Royse, 2004; da Luz et al., 2012).
The production of fungal phytases has been achieved using three different fermentation methods; namely solid-state (SSF), semi-solid, and submerged fermentation (SmF) (Han et al., 1987; Shivanna and Venkateswaran, 2014). Fungal phytases are commonly produced using solid-state fermentation (SSF) methods, in which agricultural waste and other cheap natural substrates are used as substrates in the SSF process (Sabu et al., 2002; Awad et al., 2014; Huang et al., 2018). Solid-state fermentation is defined as the fermentation process of microorganisms grown on a solid material surface with absence or near absence of free water. However, the process must have enough moisture content to support the growth of microorganisms. Solid-state fermentation of phytase production by fungi has been employed using agricultural waste and other cheap natural materials as substrates. This has been established due to the fact that these substrates can support fungal growth along with their natural metabolism (secreted enzymes). Importantly, fungi can grow on the solid substrate in the same way they typically grow in nature (Bhargav et al., 2008; Kumar and Kanwar, 2012). Furthermore, SSF involving fungi offers high volumetric productivity and high yields of enzyme production. In this method, enzymes can be easily extracted with water and the process is recognized as being less expensive, easier to use and less time-consuming (Bhargav et al., 2008). The process has been widely used in the fermentation industry, particularly for enzyme production (Pandey et al., 1999; Soccol et al., 2017). Several studies of SSF have been performed using filamentous fungi for phytase production, such as A. flavus, A. ficuum, A. niger, A. tubingensis, Ganoderma stipitatum, Grifola frondosa, M. racemosus, Penicillium purpurogenum, R. oligosporus, R. oryzae, S. commune, Thermomyces lanuginosus, and Trametes versicolor. Some of the substrates generally used for phytase production are citrus peels, wheat bran, wheat straw, soybean meal, rice bran, oil cakes, corn cobs, corn bran, and coconut oil cakes. For example, Sabu et al. (2002) have reported on phytase production by R. oligosporus on coconut oil cake substrate in SSF. In a recent study, triticale waste was used as a substrate for the evaluation of phytase production by A. niger (Neira-Vielma et al., 2018). Additionally, phytase production was investigated by SSF using mixed substrates. Roopesh et al. (2006) reported on phytase production by M. racemosus using combinations of wheat bran and various oil cakes which gave the highest phytase activity with 32.2 U/gds. Then, phytase production by Penicillium purpurogenum was investigated by SSF using mixed substrates consisting of corn cob and corn bran (Awad et al., 2014). In a recent study, Kanti and Sudiana (2018) reported on the application of Aspergillus niger, Neurospora sitophila, and R. oryzae on mixed rice straw powder and soybean curd residues. Neurospora sitophila showed the highest level of phytase production at 195.66 U/g followed by A. niger and R. oryzae. However, not only SSF has been investigated for phytase production, but a number of research studies have also investigated phytase production involving SmF and semi-solid fermentation methods (Han et al., 1987; Salmon et al., 2016; Shah et al., 2017). The production of phytase from several fungal strains has been investigated during SmF. For example, phytase production from Aspergillus fumigatus, A. japonicus, A. niger, Muscodor sp., and Ganoderma sp. MR-56 was investigated under SmF conditions using wheat bran as a substrate (Mandviwala and Khire, 2000; Mohan et al., 2004; Alves et al., 2016; Maller et al., 2016; Salmon et al., 2016). Coban and Demirci (2014) have produced and optimized culture conditions for A. ficuum NRRL 3135 using phytase selective medium containing sodium phytate as a substrate. In addition, Kanti and Sudiana (2018) produced phytase under SmF using mixed rice straw power and soybean curd residue as a substrate and Aspergillus niger, Neurospora sitophila, and R. oryzae as fungal strains to increase phytase production. Maximum phytase activity was obtained by N. sitophila (195.66 U/g) at 96 h of incubation. Also, Coban and Demirci (2015a) studied phytase production by optimizing important nutrients using A. ficuum in SmF (glucose, Na-phytate, and CaSO4) and the effect of pH and temperature on phytase activity in bench-top bioreactors by conducting fed-batch fermentations. The results revealed that the optimum glucose, Na-phytate, and CaSO4 concentrations were 126, 14, and 1.1 g/L, respectively. Optimum pH and temperature values were 5.5 and 55°C for A. ficuum phytase activity. Therefore, these conditions indicate that phytase activity increased by improving the media and process conditions. Table 1 presents several examples of fungal phytase sources and a variety of different methods of fermentation that have been employed for phytase production. Various production factors, such as the type of strain, culture conditions, the nature of the substrate, and availability of the nutrients, are all considered critical factors that can affect yields. Consequently, each of those factors should be taken into consideration when fungal phytase production is undertaken (Pandey et al., 2001).
Phytase production using different fungal strains is affected by differing culture conditions (Qasim et al., 2017; Neira-Vielma et al., 2018). Optimum conditions for the production of phytases obtained from different fungal strains have been established by changing both nutrient and physical conditions. Additionally, various sources, such as glucose and sucrose, were used as a carbon source, while ammonium sulfate [(NH4)2SO4], ammonium nitrate (NH4NO3), yeast and malt extract were used as nitrogen sources for the fermentation process, as is shown in Table 2. To check the optimum physical conditions for phytase production, such as pH value and temperature, different ranges of the initial pH of the culture medium and different temperatures of incubation have been employed (Alves et al., 2016; Qasim et al., 2017). For an example, the production of phytase from A. flavus ITCC 6720 was investigated by SSF on mustard cake as a substrate. The Optimized conditions of production involved supplementation with 0.5% malt extract and glucose at 58% moisture level, 10% inoculum level, and inoculum age-72 h old. The maximum phytase activity of 34 to 112.25 U/g fermented substrate was produced on the 4th day under an incubation temperature of 37°C and a pH value of 6.0 (Gaind and Singh, 2015), while A. flavus produced maximum phytase (80 U/g of solid substrate) in SSF using wheat bran as a solid substrate at a pH value of 6 after 7 days of the fermentation period at 30°C in the medium containing glucose (2%) as a carbon source and tryptone (1%) as a nitrogen source (Gull et al., 2013). Phytase production by filamentous mushrooms has also been studied. Salmon et al. (2012) reported that a maximal level of phytase (113.7 U/gds) was obtained in wheat bran-based medium involving 50% humidity with 7.5% of the biomass at 33°C and at a pH value of 7.0 over 72 h, which resulted in a 285% level of improvement in terms of the amounts of enzymes obtained. Ganoderma applanatum synthesized phytase in a medium comprised of soybean molasses as a carbon source and yeast extract as a nitrogen source at 30°C at 150 rpm, a pH of 6.0 and a 3% inoculum rate through SmF (Salmon et al., 2016). The production of phytase by fungal strains has been observed at a wide range of initial pH values and temperatures ranging from 4.5–8.0 and 27–50°C, respectively. Various culture conditions used for fungal phytase production by filamentous fungi are presented in Table 2. However, the cultivation of a filamentous fungus is often accompanied by several challenges, such as clumpy growth, high broth viscosity, insufficient oxygen, and mass transfer which results in reduced levels of productivity. Therefore, in order to improve biomass and product formation during cultivation of filamentous microorganisms, the process was performed under microparticle-enhanced cultivation (MPEC). To date, microparticle talc powder (magnesium silicate), aluminum oxide, and titanium oxide have been used in several studies to increase the production of enzymes in the fermentation of filamentous fungi such as A. ficuum, A. niger, A. oryzae, A. sojae, Caldariomyces fumago, Cerrena unicolor, and Pleurotus sapidus (Singh, 2018). Kaup et al. (2008) studied the effects of the different microparticle sizes of talc or aluminum microplates on chloroperoxidase (CPO) formation by Caldariomyces fumago. They observed that small particles (≤42 μm diameter) dispersed C. fumago to singer hypha, while particles around 500 μm diameter did not make any difference in the growth morphology or CPO formation productivity by C. fumago. Driouch et al. (2012) studied A. niger fermentation in submerged culture by adding titanate microparticles (TiSiO4, 8 μm) to the growth medium. They reported that fructofuranosidase and glucoamylase productions were increased by 3.7-fold to 150 U/mL and 9.5-fold to 190 U/mL, respectively when an additional 25 g/L of TiSiO4 was added to the fermentation medium as compared to the control. Driouch et al. (2011) also reported that fructofuranosidase production was increased by 3.5-fold in the presence of microparticles of either 10 g/L of talcum or 20 g/L aluminum oxide using A. niger in the fermentation medium. Additionally, Coban et al. (2015b, c) studied the effects of different microparticles on A. ficuum phytase production. They reported that A. ficuum phytase production was increased and the fungal pellet size was decreased after the addition of microparticles to the batch fermentation. Therefore, the use of a novel method of MPEC could be applied for the purposes of improved biomass and product formation of hydrolytic enzymes during cultivation of filamentous fungi.
The purification of enzymes is necessary in order to study their biochemical properties as well as to understand their structural and functional relationships. Various methods have been used to purify relatively large numbers of protein molecules, while separation is often affected by the differences of the target protein and the properties of other substances present in the sample, such as solubility, precipitation, size, polarity, and the binding affinity of ammonium sulfate/acetone/ethanol precipitation followed by ultrafiltration, ion exchange, and gel filtration chromatography (Vohra and Satyanarayana, 2003; Boyce and Walsh, 2007). Therefore, combinations of two or more methods are commonly used for the purification of fungal phytases (Zhang et al., 2013a; Neira-Vielma et al., 2018). Fungal phytases belong to a class of HAPs. Most fungal phytases are active under acidic pH conditions in the optimum pH range of 2.0–6.0 (Vohra and Satyanarayana, 2003; Singh et al., 2011; Yao et al., 2011; Zhang et al., 2013b), but some fungal phytases, for instance, Agaricus biosporus and Rhizopus microsporus var. microsporus, belong to 5.0–8.0 and 9.5 pH, respectively (Collopy and Royse, 2004; de Oliveira Ornela and Guimaraes, 2019). However, the thermostability of phytases is also essential for their use in animal feed (Dersjant-Li et al., 2015). Fungal phytases are active in the optimum temperature range of 37–67°C. Various studies have reported that different fungal phytases are active under different optimal conditions with regard to pH and temperature (Lassen et al., 2001; Zhu et al., 2011; Neira-Vielma et al., 2018). Fungal phytase obtained from A. niger van Tieghem showed a high degree of specific activity under an optimal temperature range of 52–55°C together with an optimal pH value of 2.5 (Vats and Banerjee, 2005). Moreover, only a few studies have confirmed that fungal phytases are wide molecular mass proteins ranging from 14 to 353 kDa depending on the different fungal strains (Collopy and Royse, 2004; Sariyska et al., 2005). The purification steps and biochemical properties of fungal phytases are presented in Table 3.
Fungal phytases are widely used as a feed additive to increase phosphorus availability and reduce phosphorus excretion in manure (Brinch-Pedersen et al., 2002). However, wild filamentous fungal strains that produce enzymes can rarely meet the industrial demand. Genetic engineering strategies have been used to obtain recombinant strains that produce high levels of enzymes for industrial interests (Correa et al., 2015).
The use of phytase transgenic plants is one of the approaches that may help to mitigate the problems associated with phytate indigestibility. There are two possible strategies for altering phytate levels. One involves blocking the phytate biosynthetic pathway or degrading phytate in developing seeds. The other involves altering the steps of phytate biosynthesis, but this has the potential disadvantage of affecting many other cellular processes associated with inositol phosphates. Expressing phytase transgenes during seed development to modify the final composition of harvested seeds is an alternative development process (Chiera et al., 2004). Recently, heterologous expression of phytases in plants to produce plant seeds containing high phytase levels has received increasing amounts of attention. Previous publications have reported that if cereal grains or seeds contain enough phytase, the supplementation of microbial phytase additives in animal feed will not be required. Additionally, the transgenic plants would access phosphate from the soil that contains phytate-phosphate complexes (Reddy et al., 2017). Ideal phytase transgenic expression is based on the target application, such as with root expression and seed expression. Therefore, the selection of enzyme sources and physical properties with regard to pH stability and thermo-stability can affect the success of the expression. In acidic soil, phytases with a low isoelectric point (pI) are more effective in hydrolyzing phytates in the soil than phytases with high pI values. Notably, in basic soil, phytases with high pI values are preferable to phytases with low pI values. Researchers have made several attempts to reclaim sustainable phosphate utility, plant nutrition, and ecological balance in various studies. There have been many research studies involving phytase transgenic plants. Interestingly, a recombinant fungal phytase has been constructed in soybeans that have been widely used in livestock feed (Li et al., 1997). Chiera et al. (2004) and Li et al. (2009) found that phytase genes obtained from A. ficuum under the regulations of root specific Pyk10 promoters in soybeans and transgenic plants exhibited phytase activity. The development of soybeans containing low seed phytate levels would increase phosphorus availability and eliminate the need for phytase supplementation in animal feed or livestock. Moreover, Lucca et al. (2001) recorded the expression of A. fumigatus phytase in rice (Oryza sativa). Pen et al. (1993) reported that transgenic tobacco seeds express A. niger phytases. These results confirm that transgenic tobacco seeds expressed A. niger phytases and have beneficial effects on phosphate liberation while enhancing the broiler growth rate over commercially produced phytases. Notably, the expression of the A. niger phytase gene with an ER signal peptide into canola (Brassica napus) was recorded. The results indicate that this transgenic plant could accumulate phytase (Peng et al., 2006). Similar results were reported by Wang et al. (2013) who introduced the phytase gene into canola and it greatly boosted phosphorus uptake, plant biomass and seed yields in the presence of a phosphate source. Numerous research studies have proven that enhanced phytate-phosphate availability in soil can be achieved by expressing the phytase gene in transgenic plants, as is shown in Table 4. Some evidence has shown that plant phytase expression may somehow interfere with the refolding of the enzyme or may provide an environment that is not favorable for refolding, and this could affect the enzyme properties. Yoon et al. (2011) reported on the expression levels of six microbial phytases in Chlamydomonas reinhardtii, and concluded that the N-terminal signal peptide and codon optimization affected the degree of efficient expression. Constitutive and inducible mechanisms in plant seeds and microorganisms have been identified. The constitutive and germination-inducible mechanisms in plant seeds and pollen are involved in the regulation of phytate breakdown during germination. The activity of the hydrolytic enzymes and their rate of synthesis were controlled by these two main mechanisms (Greiner, 2007). Li et al. (1997) studied using the phytase gene obtained from A. niger inserted into soybean transformation plasmids under the control of constitutive (35S CaMV promoter) and seed specific promoters (β-conglycinin α’-subunit promoter), with and without a plant signal sequence. They reported that phytase activity was detected in the culture medium obtained from transformants that received constructs containing the plant signal sequence, and this confirmed the expectation that the protein would follow the default secretory pathway. Therefore, the recombinant phytase values obtained from their studies suggested that the additional protein stability would be required to withstand the elevated temperatures involved in soybean growth processing.
In addition, the thermophilic mold Sporotrichum thermophile has been investigated in terms of the cloning and expression of phytase heterologously in bacteria (e.g., Escherichia coli) or yeast (e.g., Pichia pastoris) (Ranjan et al., 2015; Singh et al., 2018). The recombinant phytase (rSt-Phy) of the thermophilic mold S. thermophile (St-Phy) had been cloned and expressed in E. coli by Ranjan et al. (2015). They reported that rSt-Phy was produced in LB medium containing glycerol and glucose with a specific activity of 8000 U/mg total intracellular protein. The supplementation of rSt-Phy to dough had been found to be useful in the dephytinization of tandoori, naan and bread, as well as to increase the amount of inorganic phosphate and reduce the amount of sugars that are present. According to Ranjan and Satyanarayana (2016), in the expression of the codon-optimized phytase gene of S. thermophile (St-Phy) in P. pastoris, the recombinant P. pastoris harboring of phytase gene (rSt-Phy) secreted a 40-fold higher amount of phytase than the native fungal strain. Subsequently, the expression of codon-optimized S. thermophile (rSt-Phy) was used to investigate the glyceraldehyde phosphate dehydrogenase (GAP) promoter in P. pastoris (Maurya et al., 2017). They reported a result of about a 41-fold improvement in rSt-Phy production over the wild type strain. Recently, Mehmood et al. (2019) improved S. thermophile strain ST20 using physical and chemical mutagens for enhanced phytase activity. They used gamma rays and EMS (Ethyl Methane Sulfonate) mutagenesis to enhance the activity of phytase, for which the phytase activity was improved to 387 U/mL at 45°C. In addition, they also reported that the mutants produced through EMS displayed greater potential of phytase production when compared to the parent strain. The developing and improving production of heterologous proteins was determined under constitutive and inducible promoters’ systems (Parashar and Satyanarayana, 2016; Kluge et al., 2018). The expression of phytase genes phyA and appA2 were expressed in P. pastoris (constitutive or inducible) and Saccharomyces cerevisiae (inducible) by Lei and Kim (2005). The pGAPZαA vector and PPICZαA vector were used in the constitutive and inducible expressions for P. pastoris. To obtain the inducible expression in S. cerevisiae, the pYES2 vector was used. Subsequently, in 2017, the production of recombinant acidic phytase was enhanced in P. pastoris under dual promoters of constitutive (AOX) and inducible (Phy-GAP-AOX) conditions that were generated by Maurya. They found that it led to a 1.3-fold improvement in phytase production in mixed fed-batch cultivation when compared to that of Phy-AOX. Consequently, it was suggested that the improvement of the recombinant phytase gene could be beneficial to a number of production processes including the animal feed industry and the commercial bread baking industry. Notably, it can also be of benefit in deriving haloperoxidase and in plant growth-promoting.
The crystal structure analysis of phytases derived from bacteria, yeast, fungi, and plants has been reported by several researchers in terms of the distinct fold and biophysical properties that rationalized their structure (Yao et al., 2011). Phytases are classified into four groups according to the relevant catalytic mechanism; [(1) histidine acid phytases (HAPs), (2) β-propeller phytases (BPPs), (3) cysteine phytases (CPs), and (4) purple acid phosphatases (PAPs)] (Dailin et al., 2019). In the case of fungi, few phytase crystal structures from the genus Aspergillus have been studied. The studied Aspergillus species are namely; A. niger (Oakley, 2010), A. ficuum, A. neoniger, and A. fumigatus (Kostrewa et al., 1997; Liu et al., 2004; Xiang et al., 2004). Most fungal acidic phytases belong to HAPs and can be divided into two groups based on the optimum pH value of fungal HAPs, and their sub-classification in type A and B (Bei et al., 2009), PhyA (high specific activity for phytic acid and alkaline), and PhyB (acidic, low specific activity for phytic acid). Furthermore, PhyA is monomeric and PhyB is tetrameric (Wyss et al., 1998). The crystal structure of HAPs revealed three distinct domains; a large α-helical domain, a β-sheet domain, and a small α-helical domain (Dailin et al., 2019). The HAPs are composed of a large α/β-domain with a six-stranded β-sheet surrounded by several α-helices and a small α-domain. The HAPs structure also consists of N-acetylglucosamine residues and disulfide bonds. All 10 cysteine residues are involved in five disulfide bridges, but the disulfide bridge positions and the N-acetylglucosamine numbers are different in the Aspergillus species. Notably, the protein signature of HAPs is represented by the sequence consensus pattern [LIVM]-X-X-[LIVMA]-X-X-[LIVM]-X-R-H-[GN]-X-R-X-[PAS] (Liu et al., 20041). Additionally, the protein sequences of HAPs type A and B obtained from the genus Aspergillus were aligned using CLUSTAL_W and were found to share a conserved active-site motif RHGX1RX2P (Figure 3). PhyA presents an active-site as RHGARYP. With regard to the catalytic importance of amino acid, histidine residue has been reported as a nucleophile in the formation of covalent phosphoenzyme intermediates (McTigue and Van Etten, 1978; Van Etten, 1982). The mechanism of acid phytase in the complex with inorganic phosphate revealed that two phosphates and four calcium ions were bound at the active site. The inorganic phosphate was then subsequently hydrolyzed by an activated water molecule. Finally, the hydrolyzed products amounted to myo-inositol, inositol, and inorganic phosphates (Zhang, 1998; Shin et al., 2001).
Figure 3. Multiple alignments of histidine acid phytase (HAPs) from genus Aspergillus. The alignments were performed using CLUSTAL_W.
Phytase B obtained from A. niger (HAP) was comprised of 460 amino acid residues and contained five disulfide bonds at positions 52–368, 109–453, 197–422, 206–279, and 394–402, most of which were located in loops next to the surface (Kumar et al., 2013). In any case, A. fumigatus phytase consisted of 435 amino acid residues, six N-acetylglucosamine molecules, and five disulfide bonds that were present in the structure at positions 8–17, 48–391, 192–442, 241–259 and 413–421 (Xiang et al., 2004). Oakley (2010) presented a structural phytase model of phytase A obtained from A. niger that consisted of an α/β-domain, an α-domain, and an N-terminal extension. N-acetylglucosamine residues are bound to four sites of the phytase structure (N82, N184, N316, and N353) within the active site (Oakley, 2010). However, thermostability engineering of phytases is of interest for industrial and pharmaceutical applications. Site-directed mutagenesis, random mutation, molecular dynamic simulation, and protein glycosylation are methods of structural modification that are commonly employed when disulfide bonds, hydrogen bonds, ionic interaction and N/O-linked glycosylation are introduced in the phytases. Notably, this effectively improved their thermostability characteristics (Mullaney et al., 2010; Vasudevan et al., 2019). Mullaney et al. (2010) removed disulfide bridge site-directed mutagenesis number 2 from A. ficuum phytase, and this resulted in a complete loss of activity. Moreover, hydrogen bonds and ionic interaction can also support a degree of thermostability in phytases. For example, A. fumigatus phytase is heat resilient as it has a hydrogen bonding network in the E35 to S42 regions and in ionic interactions between R168 and D161 and R248 and D244. In another study, the Mn (2′-deoxyinosine 5′-triphosphate) random mutation method used on a protease-resistant phytase gene of Penicillium sp. developed two mutants with improved thermal stability and optimal temperature tolerance (Zhao et al., 2010). Formerly, using the molecular dynamic simulation, A. niger PhyA and its thermostable mutant possessing a 20% greater level of thermostability, were compared by evaluating the atomic root mean square deviation, the radius of gyration, and the number of hydrogen bonds and salt bridges that were present (Noorbatcha et al., 2013). Protein glycosylation is one of the most common structural modifications employed by biological systems to expand proteome diversity. Glycosylation in A. niger and A. japonicus phytases has been identified for its functional expression and thermostability when expressed in yeast systems (Han and Lei, 1999).
Phytases are of great interest in biotechnological applications in terms of the processing and manufacturing of human and animal nutrition since they have the potential to improve the efficient use of phosphorus and to reduce phytate content in food production and animal feed (Greiner and Konietzny, 2006; Yao et al., 2011; Singh et al., 2016). Monogastric animals, such as swine, poultry, and fish, lack or contain low levels of gastrointestinal phytases and they are unable to utilize the phytate phosphorus that is present in sources of food and animal feed. Therefore, they need inorganic phosphate supplements to meet their nutritional and growth-related needs, which in turn increases feed costs and levels of phosphorus pollution (Naves et al., 2012; Dersjant-Li et al., 2015). Phytase plays an important role in the animal feed industry because it enhances the digestion and absorption of phosphorus and certain other poorly available nutrients in monogastric diet supplements (Munir and Maqsood, 2013; Vasudevan et al., 2017). Various microorganisms are favored sources for industrial enzymes due to the ease of use that is associated with them, along with their cost-effectiveness, fast growth-rate and consistent production levels (Singh et al., 2016; Raveendran et al., 2018). Phytases produced by microorganisms are commonly used as a commercial feed additive. In 1999, the first generation of the fungal phytase obtained from A. niger was made commercially available in markets. At which point, phytases were further developed and became more widely commercially available (Lei et al., 2013; Singh et al., 2016). Several studies have investigated the applications of phytases as a feed additive.
In broilers, the pretreatment of phytases in the digestive system of animals was investigated by feeding them a soybean meal (SBM) diet using A. niger phytase supplementation (Nelson et al., 1968). Nelson et al. (1968) studied the effects of phytase by pretreating corn–soya diets for broilers and reported that the availability of phosphorus increased by 60% when microbial phytase was given to broilers fed low phosphorus diets, while phosphorus concentrations in the chicken manure decreased by 50%. The reports also indicated that the bodyweights of male (13.2%) and female (5.8%) chickens increased after 21 days of phytase supplementation. Simons et al. (1990) and Zyla et al. (2001) reported that the addition of phytase in dietary phosphorus could decrease phosphorus levels in manure and increase body weight. Several studies have been carried out to determine the effect of microbial phytase on poultry growth. Englmaierova et al. (2015) evaluated the effect of different amounts of A. niger phytase on egg quality, along with the calcium and phosphorus digestibility of the hens. The results revealed the highest degree of eggshell percentage in terms of thickness on the index when 350 FTU/kg was applied. Kim et al. (2017) studied the effect of super-dosing phytase on the productive performance and egg quality in laying hens. They reported that the super-posing level of 20,000 FTU/kg phytase in diets had a positive effect on the egg production rate, but had no beneficial effect on egg quality in laying hens. Woyengo and Wilson (2019) reported that the supplementation of phytase at super-dose levels (≥2500 FTU/kg) had a more positive effect with regard to improving the ileal digestibility of energy, protein, P in maize and other forms of nutrient utilization in the modified diets prepared for poultry when compared with the supplementation of phytase administered at the industry recommended level (1,000 FTU/kg). Calcium (Ca) and phosphorus (P) are important nutrients for bone development and the metabolic processes involved with the enzyme cofactors present in poultry diet formulations (Menezes-Blackburn et al., 2015; Li et al., 2017). However, the concentration levels and rations for poultry must be close to their specified requirements. Consequently, a study on the effect of reducing dietary Ca levels and calcium, along with available phosphorus (Ca:aP) ratios in combination supplemented fungal phytases on poultry growth performance, nutrient digestibility, bone ash, and mineralization, was conducted (Delezie et al., 2015; de Souza Nardelli et al., 2018; Ajith et al., 2019).
In pigs, the main active site for microbial phytase is in the stomach and upper part of the small intestine, a circumstance that is similar to poultry. Most of the phytases given to pigs are used to improve dietary phytate-P utilization and to improve their mineral and nutrient digestibility (Humer et al., 2015). The site of supplemental phytase activity in the gastrointestinal tract of young pigs was investigated by Yi and Kornegay (1996). They determined that supplemented A. niger fungal phytase in pig diets revealed that the digesta of the stomachs of pigs showed higher phytase activity than the digesta of the upper small intestine. The phytase activity levels in the stomach, as well as in the upper and lower parts of the small intestine, were 579, 348, and 53 FTU/kg, respectively when pig feed was supplemented with 1050 FTU/kg microbial phytases. Seynaeve et al. (2000) reported that supplementation of 500 FTU/kg A. niger phytase reduced intestine tract ileal digesta and total P (P = 0.09) and IP6-P (P < 0.05) values when compared with the non-treatment group. The supplementation of fungal phytase can reduce total P as well as inorganic P-values in feces and also improve overall growth performance and nutrient digestibility (Seynaeve et al., 2000; Dersjant-Li et al., 2015; McCormick et al., 2017).
Fungal phytase is not only used in the poultry and pig raising industries, but also in fisheries. Several studies on phytase supplementation in fish feed have involved different fish species (Kumar et al., 2012; Lemos and Tacon, 2017). For example, Yan et al. (2002) studied the effects of phytases at levels from 0 to 8,000 FTU/kg (A. niger phytase) in channel catfish (Ictalurus punctatus). They reported that the total phytate content in the stomachs of channel catfish was related to phytase inclusion levels. After feeding channel catfish for 2 h, total phytase content in the stomachs of fish fed with the phytase-supplemented feed was recorded at 92, 68, 50, 9, and 6% and at 500, 1000, 2000, 4000, and 8000 FTU/kg, respectively. A study by Forster et al. (1999) involving phytase-supplemented feed given to rainbow trout Oncorhynchus mykiss reported on the potential for using dietary phytase to improve the nutritive value of canola protein concentrate. Supplementation of phytase in fish feed indicated that dietary phytase improves the nutria value as well as increases the concentration levels of minerals in the plasma, bone, and the entire body. Additionally, it was also found to decrease the level of phosphorus that is discharged into aquatic environments.
In addition to feeding additives, phytic acid is highly present in the flour and wholemeal flour of various types of dough and bread; therefore, phytases have been used as a food additive in fermentation processes and in various applications in the breadmaking process. For example, A. ficuum phytase has been used in legume dephosphorylation processes. It was reported that after mixing and incubating soybean meal with fungal phytase for 15 h, up to 78% of phytate was removed (Han and Wilfred, 1988). Turk and Sandberg (1992) studied the effects of phytase obtained from A. niger in InsP6 degradation during the breadmaking process. They reported that phytase preparation from A. niger used for making dough resulted in increased degradation of phytates. Later, the application of phytases in bread-making was also studied by Haros et al. (2001). Experiments have been performed by adding different levels of fungal phytase in whole wheat bread during the breadmaking process. Their results showed that specific bread volume increased, while crumb texture improved. Furthermore, Rosell et al. (2009) investigated the effects of different breadmaking processes, such as conventional, frozen dough, and frozen partially baked bread, and the effect of the storage period on the technological quality of fresh wholemeal wheat bread by adding A. niger phytase. They reported that the fungal phytase addition could be used in the breadmaking process and in the frozen storage of bread to overcome the detrimental effects of bran on the mineral bioavailability.
Phosphorus (P) is a major and critical component of cells and is a constituent in energy metabolism, and the biosynthesis of acids and cell membranes. It is also an important macronutrient for plant growth and development (Singh and Satyanarayana, 2011). Phosphorus deficiency in soil is a major problem for agricultural producers worldwide. Most soils contain significant amounts of total soil P that occurs in either an organic or inorganic form. A phytic acid is a major form of organic phosphorus in the soil, representing total organic phosphorus content between 10 and 50% (Mullen, 2005). Moreover, it is not readily available to plants as a source of phosphorus because it forms a complex with cations or adsorbs to various soil components. Therefore, the improvement of phosphorus nutrition requires the mobilization of organic and inorganic phosphorus (Richardson et al., 2001). Phosphate solubilizing microorganisms are ubiquitous in soil and can play an important role in the phosphorus cycle in nature as to serve as a readily available source of carbon and energy for their growth and reproduction (Whipps, 1990). In the rhizosphere, plant growth-promoting fungi (PGPF) solubilize or mineralize phosphorus and increase its availability to plants (Zhang et al., 2016; Hossain et al., 2017). Thakur et al. (2017) isolated and characterized extracellular phytase-producing A. fumigatus from the rhizospheric zone of maize fields. Phytase-producing fungi in the rhizosphere have been isolated and studied for their important role in promoting plant growth. Aspergillus was isolated as phytase-producing rhizofungi, and they were found to significantly improve the growth and phosphorus nutrition of Arabidopsis plants (Richardson et al., 2001). Furthermore, various Aspergillus species, such as A. flavus, A. fumigatus, and A. rugulosus, were used to promote the growth of plants (Tarafdar and Rao, 1996; Tarafdar and Gharu, 2006; Gaind and Singh, 2015). Gaind and Nain (2015) isolated various phytate-mineralizing fungi (PMF) and phosphatase-solubilizing fungi (PSF) from the rhizosphere soil of leguminous, cereal, and vegetable crops that belong to Aspergillus, Trichoderma, and Penicillium. They reported that Penicillium chrysogenum solubilizes the organic form of phosphorus and improves the available P in the soil while increasing the level of extractable organic P under alkaline soil conditions to benefit P nutrition. Singh and Satyanarayana (2010) investigated the role of phytase-producing fungi by increasing phosphorus content and promoting the growth of wheat (Triticum aestivum L.) seedlings. Tarafdar and Gharu (2006) also tested the significant role of the phytase producing fungus, Chaetomium globosum, for the improvement in plant biomass, root length, plant P concentration levels, seed and straw yields and seed P contents in wheat and pearl millet crops. According to the findings of a range of studies, it can be concluded that fungal phytase could be used to promote the growth of crop plants and to improve overall productivity levels.
In many parts of the world, humans consume plant-based food products as the main source of raw material food. Plant-based food products compost very important sources of nutrients (carbohydrates, protein, dietary fiber, and vitamins) and non-nutrients (Katina et al., 2005). Phytate is the primary storage compound of phosphorus in plant seeds and grains accounting for up to 90% of the total seed phosphorus (Loewus, 2002). It forms complexes with dietary minerals such as zinc, iron, calcium, magnesium, manganese, and copper, and causes mineral-related deficiency in humans (Lopez et al., 2002; Konietzny and Greiner, 2003). For instance, negative effects on mineral uptake, protein digestibility, carbohydrate, and lipid utilization have been recorded. In spite of the fact that phytates present a number of negative effects on human health, some positive effects have also been reported. Their consumption acts as an anticancer property by interrupting cellular signal transduction and cell cycle inhibition, and by enhancing natural killer cell activity (Kumar et al., 2010). The phytate substrates have been reported in various biomedical and biotechnological applications including those associated with antioxidant properties, as well as being identified as an anticancer agent, and a chelator with neuroprotective properties that can induce autophagy and reduce inflammation (Irshad et al., 2017). In addition, they have also displayed various therapeutic properties as anticancer agents (against colon cancer, breast cancer, hepatocellular carcinoma, prostate cancer, rhabdomyosarcoma, pancreatic cancer, and blood/bone marrow cancer) and have been found to be effective against Parkinson’s disease (Kumar et al., 2010; Irshad et al., 2017).
Phytases are beneficial enzymes for animal nutrition. They held the highest revenue share of 83.6% of the total industry in 20152 and account for annual sales of US$ 350 million. About 70% of monogastric animal feed is supplemented with phytases (Ranjan and Satyanarayana, 2016). The first commercial phytase product was derived from A. niger and was classified as a 3-phytase. It was used in animal feed and was first marketed in 1999 under the trade name Natuphos. It was manufactured by Gist-Brocades (now DSM) and sold by BASF, Ludwigshafen, Germany. Later, the commercial product (Ronozyme® P) belonging to a 6-phytase was derived from Peniophora lycii. Subsequently, a few fungal phytase products have been produced and marketed by other companies over the years (Table 5). On a commercial scale, phytase production is either carried out using phytate-producing fungi or recombinant DNA technology. The commercial products of Allzyme® SSF and Adisseo were produced by naturally secreted enzymes that are synergistic with phytase. However, most fungal phytases used on a commercial scale were derived by using recombinant DNA technology. These commercial products are produced by recombinant filamentous fungal strains (Table 5).
Several commercial phytase products are used as supplements for monogastric animal feeds. The function of phytase in animal feeds and digestive systems is critically important. In addition, different phytases used for animal feed applications differ in their enzymatic properties. For instance, the optimum pH and temperature values of Ronozyme® P were 4–4.5 and 50–55°C, respectively (Lassen et al., 2001). While Natuphos® revealed optimum pH and temperature values at 2.0, 5–5.5, and 65°C, respectively (Wyss et al., 1999; Zhang et al., 2007; Weaver et al., 2009). The performance of commercial phytases was also determined in terms of their enzymatic properties under identical assay conditions. For example, the commercial product named Rovabio was used to investigate growth performance and intestinal viscosity in broiler chicks fed (Lee et al., 2010). Additionally, Ronozyme HiPhos was used to investigate the apparent ileal digestibility of minerals and amino acids in ileorectal anastomosed pigs (Guggenbuhl et al., 2012). Menezes-Blackburn et al. (2015) used Natuphos® and Ronozyme® P in a study involving in vitro stimulation of the digestive tracts of poultry.
Fungal phytases have gained increasing amounts of interest for use in food production and in the feed industries as a way of improving nutrition quality and reducing levels of phosphorus pollution. The study of different biological properties of fungal phytase is important and can assist researchers in improving the levels of phytase activity and stability for nutritional and industrial uses. However, only a few fungal strains have been studied in terms of phytase production. Therefore, the discovery of new fungal species with advanced phytase properties and levels of stability will be necessary. In addition, the cloning and protein engineering of potential phytase producing fungal species will also be extremely advantageous.
KJ, NS, JK, and SL designed the general plan of the review. KJ conducted the necessary literature searches, created figures, and wrote the manuscript. PK and WP conducted a literature search and wrote the manuscript. WP conducted a bioinformatic analysis of the protein sequences. All authors read, revised and approved the final manuscript.
The authors are grateful to the Office of Higher Education Commission (BDC-PG2-159013) for providing support for writing this review article. This research work was partially supported by Chiang Mai University.
The authors declare that the research was conducted in the absence of any commercial or financial relationships that could be construed as a potential conflict of interest.
We would like to thank Samantha C. Karunarathna from the Key Laboratory for Plant Diversity and Biogeography of East Asia, Kunming Institute of Botany, Chinese Academy of Sciences, Kunming, China for his useful suggestions and corrections.
Abid, N., Khatoon, A., Maqbool, A., Irfan, M., Bashir, A., Asif, I., et al. (2017). Transgenic expression of phytase in wheat endosperm increases bioavailability of iron and zinc in grains. Transgenic Res. 26, 109–122. doi: 10.1007/s11248-016-9983-z
Ajith, S., Ghosh, J., Shet, D., ShreeVidhya, S., Punith, B. D., and Elangovan, A. V. (2019). Partial purification and characterization of phytase from Aspergillus foetidus MTCC 11682. AMB Express 9:3. doi: 10.1186/s13568-018-0725-x
Alves, N. M., Guimaraes, L. H. S., Piccoli, R. H., and Cardoso, P. C. (2016). Production and partial characterization of an extracellular phytase produced by Muscodor sp. under submerged fermentation. Adv. Microbiol. 6, 23–32. doi: 10.4236/aim.2016.61003
Awad, G. E. A., Helal, M. M. I., Danial, E. N., and Esawy, M. A. (2014). Optimization of phytase production by Penicillium purpurogenum GE1 under solid state fermentation by using Box–Behnken design. Saudi J. Biol. Sci. 21, 81–88. doi: 10.1016/j.sjbs.2013.06.004
Bei, J., Chen, Z., Fu, J., Jiang, Z., Wang, J., and Wang, X. (2009). Structure-based fragment shuffling of two fungal phytases for combination of desirable properties. J. Biotechnol. 139, 186–193. doi: 10.1016/j.jbiotec.2008.08.011
Berikten, D., and Kivanc, M. (2013). Optimization of solid-state fermentation for phytase production by Thermomyces lanuginosus using response surface methodology. Prep. Biochem. Biotechnol. 44, 834–848. doi: 10.1080/10826068.2013.868357
Bhargav, S., Panda, B. P., Ali, M., and Javed, S. (2008). Solid-state fermentation: an overview. Chem. Biochem. Eng. Q. 22, 49–70.
Bhavsar, K., Shah, P., Soni, S. K., and Khire, J. M. (2008). Influence of pretreatment of agriculture residues on phytase production by Aspergillus niger NCIM 563 under submerged fermentation conditions. Afr. J. Biotechnol. 7, 1101–1106.
Boyce, A., and Walsh, G. (2007). Purification and characterisation of an acid phosphatase with phytase activity from Mucor hiemalis Wehmer. J. Biotechnol. 132, 82–87. doi: 10.1016/j.jbiotec.2007.08.028
Brinch-Pedersen, H., Hatzack, F., Stoger, E., Arcalis, E., Pontopidan, K., and Holm, P. B. (2006). Heat-stable phytases in transgenic wheat (Triticum aestivum L.): deposition pattern, thermostability, and phytate hydrolysis. J. Agric. Food Chem. 54, 4624–4632. doi: 10.1021/jf0600152
Brinch-Pedersen, H., Olesen, A., Rasmussen, S. K., and Holm, P. B. (2000). Generation of transgenic wheat (Triticum aestivum L.) for constitutive accumulation of an Aspergillus phytase. Mol. Breed. 6, 195–206. doi: 10.1023/A:1009690730620
Brinch-Pedersen, H., Sørensen, L. D., and Holm, P. B. (2002). Engineering crop plants: getting a handle on phosphate. Trends Plant Sci. 7, 118–125. doi: 10.1016/S1360-1385(01)02222-1
Buckle, K. A. (1988). Characterization of extra-and intracellular phytases from Rhizopus oligosporus used in tempeh production. Int. J. Food microbiol. 6, 67–79. doi: 10.1016/0168-1605(88)90086-4
Bujna, E., Rezessy-Szabo, J. M., Nguyen, D. V., and Nguyen, D. Q. (2016). Production and some properties of extracellular phytase from Thermomyces lanuginosus IMI 096218 on rice flour as substrate. Mycosphere 7, 1576–1587. doi: 10.5943/mycosphere/si/3b/9
Casey, A., and Walsh, G. (2003). Purification and characterization of extracellular phytase from Aspergillus niger ATCC 9142. Bioresour. Technol. 86, 183–188. doi: 10.1016/S0960-8524(02)00145-1
Casey, A., and Walsh, G. (2004). Identification and characterization of a phytase of potential commercial interest. J. Biotechnol. 110, 313–322. doi: 10.1016/j.jbiotec.2004.03.001
Chadha, B. S., Harmeet, G., Mandeep, M., Saini, H. S., and Singh, N. (2004). Phytase production by the thermophilic fungus Rhizomucor pusillus. World J. Microbiol. Biotechnol. 20, 105–109. doi: 10.1023/B:WIBI.0000
Chelius, M. K., and Wodzinski, R. J. (1994). Strain improvement of Aspergillus niger for phytase production. Appl. Microbiol. Biotechnol. 41, 79–83. doi: 10.1007/BF00166085
Chen, R., Xue, G., Chen, P., Yao, B., Yang, W., Ma, Q., et al. (2008). Transgenic maize plants expressing a fungal phytase gene. Transgenic Res. 17, 633–643. doi: 10.1007/s11248-007-9138-3
Chiera, J. M., Finer, J. J., and Grabau, E. A. (2004). Ectopic expression of a soybean phytase in developing seeds of Glycine max to improve phosphorus availability. Plant Mol. Biol. 56, 895–904. doi: 10.1007/s11103-004-5293-6
Coban, H. B., and Demirci, A. (2014). Screening of phytase producers and optimization of culture conditions for submerged fermentation. Bioprocess Biosyst. Eng. 37, 609–616. doi: 10.1007/s00449-013-1028-x
Coban, H. B., and Demirci, A. (2015a). Improved submerged Aspergillus ficuum phytase production in bench-top bioreactors by optimization of fermentation medium. Acta Aliment. 44, 549–560. doi: 10.1556/066.2015.44.0027
Coban, H. B., Demirci, A., and Turhan, I. (2015b). Enhanced Aspergillus ficuum phytase production in fed-batch and continuous fermentations in the presence of talcum microparticles. Bioprocess Biosyst. Eng. 38, 1431–1436. doi: 10.1007/s00449-015-1384-9
Coban, H. B., Demirci, A., and Turhan, I. (2015c). Microparticle-enhanced Aspergillus ficuum phytase production and evaluation of fungal morphology in submerged fermentation. Bioprocess Biosyst. Eng. 38, 1075–1080. doi: 10.1007/s00449-014-1349-4
Collopy, P. D., and Royse, D. J. (2004). Characterization of phytase activity from cultivated edible mushrooms and their production substrates. J. Agric. Food Chem. 52, 7518–7524. doi: 10.1021/jf040220m
Correa, T. L. R., de Queiroz, M. V., and de Araujo, E. F. (2015). Cloning, recombinant expression and characterization of a new phytase from Penicillium chrysogenum. Microbiol. Res. 170, 205–212. doi: 10.1016/j.micres.2014.06.005
da Luz, J. M. R., Dias Nunes, M., Paes, S., Torres, D., Silva, M., and Kasuya, M. C. (2012). Lignocellulolytic enzyme production of Pleurotus ostreatus growth in agroindustrial wastes. Braz. J. Microbiol. 43, 1508–1515. doi: 10.1590/S1517-838220120004000035
Dailin, D. J., Hanapi, S. Z., Elsayed, E., Sukmawati, D., Wan Azelee, N. I., Eyahmalay, J., et al. (2019). “Fungal phytases: biotechnological applications in food and feed industries,” in Recent Advancement in White Biotechnology Through Fungi. Fungal Biology, eds A. Yadav, S. Singh, S. Mishra, and A. Gupta, (Cham: Springer), 65–99. doi: 10.1007/978-3-030-14846-1_2
Das, S., and Ghosh, U. (2014). Effect of nutritional supplementation of solid state fermentation medium on biosynthesis of phytase from Aspergillus niger NCIM 612. J. Sci. Ind. Res. 73, 593–597.
de Oliveira Ornela, P. H., and Guimaraes, L. H. S. (2019). Purification and characterization of an alkalistable phytase produced by Rhizopus microsporus var. microsporus in submerged fermentation. Process Biochem. 81, 70–76. doi: 10.1016/j.procbio.2019.03.015
de Souza Nardelli, N. B., de Paula Naves, L., de Oliveira, D. H., Junior, A. A. P. G., Lima, E. M. C., de Oliveira, E. C., et al. (2018). Supplementation of fungal and/or bacterial phytase in broiler diets formulated with reduced phosphorus level and different calcium contents. R. Bras. Zootec. 47, 1–8. doi: 10.1590/rbz4720170297
Delezie, E., Bierman, K., Nollet, L., and Maertens, L. (2015). Impacts of calcium and phosphorus concentration, their ratio, and phytase supplementation level on growth performance, foot pad lesions, and hock burn of broiler chickens. J. Appl. Poult. Res. 24, 115–126. doi: 10.3382/japr/pfv011
Dersjant-Li, Y., Awati, A., Schulze, H., and Partridge, G. (2015). Phytase in non-ruminant animal nutrition: a critical review on phytase activities in the gastrointestinal tract and influencing factors. J. Sci. Food Agric. 95, 878–896. doi: 10.1002/jsfa.6998
Driouch, H., Hansch, R., Wucherpfennig, T., Krull, R., and Wittmann, C. (2012). Improved enzyme production by bio-pellets of Aspergillus niger: targeted morphology engineering using titanate microparticles. Biotechnol. Bioeng. 109, 462–471. doi: 10.1002/bit.23313
Driouch, H., Roth, A., Dersch, P., and Wittmann, C. (2011). Filamentous fungi in good shape: microparticles for tailor-made fungal morphology and enhanced enzyme production. Bioeng. Bugs 2, 100–104. doi: 10.4161/bbug.2.2.13757
Ebune, A., Al-Asheh, S., and Duvnjak, Z. (1995). Production of phytase during solid state fermentation using Aspergillus ficuum NRRL 3135 in canola meal. Bioresour. Technol. 53, 7–12. doi: 10.1016/0960-8524(95)00041-C
Englmaierova, M., Skrivan, M., Skrivanova, E., Bubancova, I., Cermak, L., and Vlckova, J. (2015). Effects of a low-phosphorus diet and exogenous phytase on performance, egg quality, and bacterial colonisation and digestibility of minerals in the digestive tract of laying hens. Czech J. Anim. Sci. 60, 542–549. doi: 10.17221/8596-CJAS
Erpel, F., Restovic, F., and Arce-Johnson, P. (2016). Development of phytase-expressing chlamydomonas reinhardtii for monogastric animal nutrition. BMC Biotechnol. 16:29. doi: 10.1186/s12896-016-0258-9
European Union (2004). List of the authorised additives in feedingstuffs published in application of Article 9t (b) of Council Directive 70/524/EEC concerning additives in feedingstuffs. O. J. C. 50, 1–144.
European Commission (2004). Commission regulation (EC) No 1465/2004 of 17 August 2004 concerning the permanent authorisation of an additive in feedingstuffs. Off. J. Eur. Union L 270, 11–13.
Forster, I., Higgs, D. A., Dosanjh, B. S., Rowshandeli, M., and Parr, J. (1999). Potential for dietary phytase to improve the nutritive value of canola protein concentrate and decrease phosphorus output in rainbow trout (Oncorhynchus mykiss) held in 11 C fresh water. Aquaculture 179, 109–125. doi: 10.1016/S0044-8486(99)00156-8
Gaind, S., and Nain, L. (2015). Soil–phosphorus mobilization potential of phytate mineralizing fungi. J. Plant Nutr. 38, 2159–2175. doi: 10.1080/01904167.2015.1014561
Gaind, S., and Singh, S. (2015). Production, purification and characterization of neutral phytase from thermotolerant Aspergillus flavus ITCC 6720. Int. Biodeter. Biodegr. 99, 15–22. doi: 10.1016/j.ibiod.2014.12.013
Giles, C. D., Richardson, A. E., Cade-Menun, B. J., Mezeli, M. M., Brown, L. K., Menezes-Blackburn, D., et al. (2018). Phosphorus acquisition by citrate-and phytase-exuding Nicotiana tabacum plant mixtures depends on soil phosphorus availability and root intermingling. Physiol. Plant 163, 356–371. doi: 10.1111/ppl.12718
Greiner, R. (2007). “Phytate-degrading enzymes: regulation of synthesis in microorganisms and plants,” in Inositol Phosphates: Linking Agriculture and the Environment, eds B. L. Turner, A. E. Richardson, and E. J. Mullaney, (Wallingford: Centre for Agriculture and Bioscience International), 78–96. doi: 10.1079/9781845931520.0078
Greiner, R., and Konietzny, U. (2006). Phytase for food application. Food Technol. Biotechnol. 44, 125–140.
Greiner, R., and Konietzny, U. (2012). “Update on characteristics of commercial phytases”, in Proceedings of the International Phytase Summit, ed. A. B Vista (Rome: AB Agri Ltd.), 96–107.
Guggenbuhl, P., Wache, Y., Simoes Nunes, C., and Fru, F. (2012). Effects of a 6-phytase on the apparent ileal digestibility of minerals and amino acids in ileorectal anastomosed pigs fed on a corn–soybean meal–barley diet. J. Anim. Sci. 4, 182–184. doi: 10.2527/jas.53892
Gulati, H., Chadha, B., and Saini, H. (2007). Production, purification and characterization of thermostable phytase from thermophilic fungus Thermomyces lanuginosus TL-7. Acta Microbiol. Immunol. Hung. 54, 121–138. doi: 10.1556/AMicr.54.2007.2.3
Gull, I., Hameed, A., Aslam, M. S., and Athar, M. A. (2013). Optimization of phytase production in solid state fermentation by different fungi. Afr. J. Microbiol. Res. 7, 5207–5212. doi: 10.5897/AJMR2013.6136
Gunashree, B. S., and Venkateswaran, G. (2008). Effect of different cultural conditions for phytase production by Aspergillus niger CFR 335 in submerged and solid-state fermentations. J. Ind. Microbiol. Biotechnol. 35, 1587–1596. doi: 10.1007/s10295-008-0402-1
Gupta, R. K., Gangoliya, S. S., and Singh, N. K. (2014). Isolation of thermotolerant phytase producing fungi and optimisation of phytase production by Aspergillus niger NRF9 in solid state fermentation using response surface methodology. Biotechnol. Bioproc. Eng. 19, 996–1004. doi: 10.1007/s12257-014-0175-5
Haefner, S., Knietsch, A., Scholten, E., Braun, J., Lohscheidt, M., and Zelder, O. (2005). Biotechnological production and applications of phytases. Appl. Microbiol. Biotechnol. 68, 588–597. doi: 10.1007/s00253-005-0005-y
Han, Y., and Lei, X. G. (1999). Role of glycosylation in the functional expression of an Aspergillus niger phytase (phyA) in Pichia pastoris. Arch. Biochem. Biophys. 364, 83–90. doi: 10.1006/abbi.1999.1115
Han, Y. W., Gallagher, D. J., and Wilfred, A. G. (1987). Phytase production by Aspergillus ficuum on semisolid substrate. J. Ind. Microbiol. 2, 195–200. doi: 10.1007/BF01569540
Han, Y. W., and Wilfred, A. G. (1988). Hydrolysis of phytate in soybean and cottonseed meals by Aspergillus ficuum phytase. J. Agric. Food Chem. 36, 259–262. doi: 10.1021/jf00080a006
Haritha, K., and Sambasivarao, K. R. S. (2009). Phytase production by Rhizopus oligosporus MTCC556 under submerged fermentation conditions. Asian J. Biol. Sci. 4, 270–273.
Haros, M., Rosell, C. M., and Benedito, C. (2001). Use of fungal phytase to improve breadmaking performance of whole wheat bread. J. Agric. Food Chem. 49, 5450–5454. doi: 10.1021/jf010642l
Hossain, M. M., Sultana, F., and Islam, S. (2017). “Plant growth-promoting fungi (PGPF): phytostimulation and induced systemic resistance,” in Plant-Microbe Interactions in Agro-Ecological Perspectives, eds D. P. Singh, H. B. Singh, and R. Prabha, (Singapore: Springer), 135–191. doi: 10.1007/978-981-10-6593-4
Howson, S. J., and Davis, R. P. (1983). Production of phytate-hydrolysing enzyme by some fungi. Enzyme Microb. Technol. 5, 377–382. doi: 10.1016/0141-0229(83)90012-1
Huang, S. J., Chen, C. H., and Tsai, S. Y. (2018). Phytase production by Grifola Frondosa and its application in inositol-enriched solid-state fermentation brown rice. Int. J. Food Eng. 4, 263–267. doi: 10.18178/ijfe.4.4.263-267
Humer, E., Schwarz, C., and Schedle, K. (2015). Phytate in pig and poultry nutrition. J. Anim. Physiol. Anim. Nutr. 99, 605–625. doi: 10.1111/jpn.12258
Irshad, M., Asgher, M., Bhatti, K. H., Zafar, M., and Anwar, Z. (2017). Anticancer and nutraceutical potentialities of phytase/phytate. Int. J. Pharmacol. 13, 808–817. doi: 10.3923/ijp.2017.808.817
Jafari-Tapeh, H., Hamidi-Esfahani, Z., and Azizi, M. H. (2012). Culture condition improvement for phytase production in solid state fermentation by Aspergillus ficuum using statistical method. ISRN Chem. Eng. 2012, 1–5. doi: 10.5402/2012/479167
Kanti, A., and Sudiana, I. M. (2018). “Production of phytase, amylase and cellulase by Aspergillus, Rhizophus and Neurospora on mixed rice rtraw powder and soybean curd residue,” in Proceedings of the IOP Conference Series: Earth and Environmental Science (Bristol: IOP Publishing), 1–9. doi: 10.1088/1755-1315/166/1/012010
Karthik, N., Binod, P., and Pandey, A. (2018). SSF production, purification and characterization of chitin deacetylase from Aspergillus flavus. Biocatal. Biotransformation 36, 296–306. doi: 10.1080/10242422.2017.1393417
Katina, K., Arendt, E., Liukkonen, K. H., Autio, K., Flander, L., and Poutanen, K. (2005). Potential of sourdough for healthier cereal products. Trends Food Sci. Technol. 16, 104–112. doi: 10.1016/j.tifs.2004.03.008
Kaup, B. A., Ehrich, K., Pescheck, M., and Schrader, J. (2008). Microparticle-enhanced cultivation of filamentous microorganisms: increased chloroperoxidase formation by Caldariomyces fumago as an example. Biotechnol. Bioeng. 99, 491–498. doi: 10.1002/bit.21713
Kim, J. H., Pitargue, F. M., Jung, H., Han, G. P., Choi, H. S., and Kil, D. Y. (2017). Effect of superdosing phytase on productive performance and egg quality in laying hens. Asian Australas. J. Anim. Sci. 30, 994–998. doi: 10.5713/ajas.17.0149
Kluge, J., Terfehr, D., and Kuck, U. (2018). Inducible promoters and functional genomic approaches for the genetic engineering of filamentous fungi. Appl. Microbiol. Biotechnol. 102, 6357–6372. doi: 10.1007/s00253-018-9115-1
Konietzny, U., and Greiner, R. (2003). “Phytic acid: nutritional impact,” in Encyclopaedia of Food Science and Nutrition, eds B. Caballero, L. Trugo, and P. Finglas, (London: Elsevier), 4555–4563. doi: 10.1016/b0-12-227055-x/00923-8
Kostrewa, D., Gruninger-Leitch, F., D’Arcy, A., Broger, C., Mitchell, D., and van Loon, A. P. (1997). Crystal structure of phytase from Aspergillus ficuum at 2.5 Å resolution. Nat. Struct. Biol. 4, 185–190. doi: 10.1038/nsb0397-185
Kumar, A., and Kanwar, S. S. (2012). Lipase production in solid-state fermentation (SSF): recent developments and biotechnological applications. Dyn. Biochem. Process Biotechnol. Mol. Biol. 6, 13–27.
Kumar, K., Dixit, M., Khire, J., and Pal, S. (2013). Atomistic details of effect of disulfide bond reduction on active site of phytase B from Aspergillus niger: a MD Study. Bioinformation 9, 963–967. doi: 10.6026/97320630009963
Kumar, V., Sinha, A. K., Makkar, H. P., and Becker, K. (2010). Dietary roles of phytate and phytase in human nutrition: a review. Food Chem. 120, 945–959. doi: 10.1016/j.foodchem.2009.11.052
Kumar, V., Sinha, A. K., Makkar, H. P. S., de Boeck, G., and Becker, K. (2012). Phytate and phytase in fish nutrition. J. Anim. Physiol. Anim. Nutr. 96, 335–364. doi: 10.1111/j.1439-0396.2011.01169.x
Lassen, S. F., Breinholt, J., Ostergaard, P. R., Brugger, R., Bischoff, A., Wyss, M., et al. (2001). Expression, gene cloning, and characterization of five novel phytases from four basidiomycete fungi: Peniophora lycii, Agrocybe pediades, a Ceriporia sp., and Trametes pubescens. Appl. Environ. Microbiol. 67, 4701–4707. doi: 10.1128/AEM.67.10.4701-4707.2001
Lata, S., Rastogi, S., Kapoor, A., and Imran, M. (2013). Optimization of culture conditions for the production of phytase from Aspergillus heteromorphus MTCC 10685. Int. J. Adv. Biotechnol. Res. 4, 224–235.
Lee, S. Y., Kim, J. S., Kim, J. M., An, B. K., and Kang, C. W. (2010). Effects of multiple enzyme (Rovabio® Max) containing carbohydrolases and phytase on growth performance and intestinal viscosity in broiler chicks fed corn-wheat-soybean meal based diets. Asian Australas. J. Anim. Sci. 23, 1198–1204. doi: 10.5713/ajas.2010.90592
Lei, X. G., and Kim, T. (2005). “Expression of microbial phytases in yeast systems and characterization of the recombinant enzymes,” in Microbial Enzymes and Biotransformations, ed. J. L. Barredo, (Totowa, NJ: Humana Press), 209–224. doi: 10.1385/1-59259-846-3:209
Lei, X. G., Weaver, J. D., Mullaney, E., Ullah, A. H., and Azain, M. J. (2013). Phytase, a new life for an “old” enzyme. Annu. Rev. Anim. Biosci. 1, 283–309. doi: 10.1146/annurev-animal-031412-103717
Lemos, D., and Tacon, A. G. J. (2017). Use of phytases in fish and shrimp feeds: a review. Rev. Aquacult. 9, 266–282. doi: 10.1111/raq.12138
Li, G., Yang, S., Li, M., Qiao, Y., and Wang, J. (2009). Functional analysis of an Aspergillus ficuum phytase gene in Saccharomyces cerevisiae and its root-specific secretory expression in transgenic soybean plants. Biotechnol. Lett. 31, 1297–1303. doi: 10.1007/s10529-009-9992-6
Li, J., Hegeman, C. E., Hanlon, R. W., Lacy, G. H., Denbow, D. M., and Grabau, E. A. (1997). Secretion of active recombinant phytase from soybean cell-suspension cultures. Plant Physiol. 114, 1103–1111. doi: 10.1104/pp.114.3.1103
Li, X., Zhang, D., and Bryden, W. L. (2017). Calcium and phosphorus metabolism and nutrition of poultry: are current diets formulated in excess? Anim. Prod. Sci. 57, 2304–2310. doi: 10.1071/AN17389
Liu, Q., Huang, Q., Lei, X. G., and Hao, Q. (2004). Crystallographic snapshots of Aspergillus fumigatus phytase, revealing its enzymatic dynamics. Structure 12, 1575–1583. doi: 10.1016/j.str.2004.06.015
Loewus, F. (2002). “Biosynthesis of phytate in food grains and seeds,” in Food Phytates, eds N. R. Reddy and S. K. Sathe, (Boca Raton, FL: CRC Press), 53–61.
Lopez, H. W., Leenhardt, F., Coudray, C., and Remesy, C. (2002). Minerals and phytic acid interactions: is it a real problem for human nutrition? Int. J. Food Sci. Technol. 37, 727–739. doi: 10.1046/j.1365-2621.2002.00618.x
Lott, J. N. A., Ockenden, I., Raboy, V., and Batten, G. D. (2000). Phytic acid and phosphorus in crop seeds and fruits: a global estimate. Seed Sci. Res. 10, 11–33. doi: 10.1017/S0960258500000039
Lucca, P., Hurrell, R., and Potrykus, I. (2001). Genetic engineering approaches to improve the bioavailability and the level of iron in rice grains. Theor. Appl. Genet. 102, 392–397. doi: 10.1007/s001220051
Maller, A., de Quadros, T. C. O., Junqueira, O. M., Grana, A. L., de Lima Montaldi, A. P., Alarcon, R., et al. (2016). Biochemical effect of a histidine phosphatase acid (phytase) of Aspergillus japonicus var. Saito on performance and bony characteristics of broiler. Springerplus 5:1418. doi: 10.1186/s40064-016-3082-8
Mandviwala, T. N., and Khire, J. M. (2000). Production of high activity thermostable phytase from thermotolerant Aspergillus niger in solid state fermentation. J. Ind. Microbiol. Biotechnol. 24, 237–243. doi: 10.1038/sj.jim.2900811
Maurya, A. K., Parashar, D., and Satyanarayana, T. (2017). Bioprocess for the production of recombinant HAP phytase of the thermophilic mold Sporotrichum thermophile and its structural and biochemical characteristics. Int. J. Biol. Macromol. 94, 36–44. doi: 10.1016/j.ijbiomac.2016.09.102
McCormick, K., Walk, C. L., Wyatt, C. L., and Adeola, O. (2017). Phosphorus utilization response of pigs and broiler chickens to diets supplemented with antimicrobials and phytase. Anim. Nutr. 3, 77–84. doi: 10.1016/j.aninu.2016.11.004
McTigue, J. J., and Van Etten, R. L. (1978). An essential arginine residue in human prostatic acid phosphate. Biochim. Biophys. Acta 523, 422–429. doi: 10.1016/0005-2744(78)90043-8
Mehmood, A., Baneen, U., Zaheer, A., Sajid, M. W., Hussain, A., Saleem, S., et al. (2019). Physical and chemical mutagens improved Sporotrichum thermophile, strain ST20 for enhanced phytase activity. Saudi J. Biol. Sci. 26, 1485–1491. doi: 10.1016/j.sjbs.2019.07.006
Menezes-Blackburn, D., Gabler, S., and Greiner, R. (2015). Performance of seven commercial phytases in an in vitro simulation of poultry digestive tract. J. Agric. Food Chem. 63, 6142–6149. doi: 10.1021/acs.jafc.5b01996
Mohan, S., Antony, M. V., and Kasinathan, P. (2004). Submerged fermentation of phytase by Aspergillus niger and Aspergillus fumigatus. Asian J. Microbiol. Biotechnol. Environ. Sci. 6, 107–111.
Mohsin, S., Maqbool, A., Ashraf, M., and Malik, K. A. (2017). Extracellular secretion of phytase from transgenic wheat roots allows utilization of phytate for enhanced phosphorus uptake. Mol. Biotechnol. 59, 334–342. doi: 10.1007/s12033-017-0020-0
Mukhametzyanova, A. D., Akhmetova, A. I., and Sharipova, M. R. (2012). Microorganisms as phytase producers. Microbiology 81, 267–275. doi: 10.1134/S0026261712030095
Mullaney, E. J., Daly, C. B., and Ullah, A. H. J. (2000). Advances in phytase research. Adv. Appl. Microbiol. 47, 157–199. doi: 10.1016/S0065-2164(00)47004-8
Mullaney, E. J., Locovare, H., Sethumadhavan, K., Boone, S., Lei, X. G., and Ullah, A. H. J. (2010). Site-directed mutagenesis of disulfide bridges in Aspergillus niger NRRL 3135 phytase (PhyA), their expression in Pichia pastoris and catalytic characterization. Appl. Microbiol. Biotechnol. 87, 1367–1372. doi: 10.1007/s00253-010-2542-2
Mullen, M. D. (2005). “Phosphorus in soils: biological interactions,” in Encyclopedia of Soils in the Environment, ed. D. Hillel, (Amsterdam: Elsevier Limited), 210–215.
Munir, K., and Maqsood, S. (2013). A review on role of exogenous enzyme supplementation in poultry production. Emir. J. Food Agric. 25, 66–80. doi: 10.9755/ejfa.v25i1.9138
Nampoothiri, K. M., Tomes, G. J, Krishnankutty, R., Szakacs, G., Nagy, V., Soccol, C., and Pandey, A. (2004). Thermostable phytase production by Thermoascus aurantiacus in submerged fermentation. Appl. Biochem. Biotechnol. 118, 205–214. doi: 10.1385/ABAB:118:1-3:205
Naves, L., Correa, A., Bertechini, A., Gomide, E. M., and dos Santos, C. D. (2012). Effect of pH and temperature on the activity of phytase products used in broiler nutrition. Braz. J. Poult. Sci. 14, 159–232. doi: 10.1590/S1516-635X2012000300004
Neira-Vielma, A. A., Aguilar, C. N., Ilyina, A., Contreras-Esquivel, J. C., Carneiro-da-Cunha, M. G., Michelena-Álvarez, G., et al. (2018). Optimized production of phytase by solid-state fermentation using triticale as substrate and source of inducer. Afr. J. Biotechnol. 17, 81–90. doi: 10.5897/AJB2017.16267
Nelson, T. S., Shieh, T. R., Wodzinski, R. J., and Ware, J. H. (1968). The availability of phytate phosphorus in soybean meal before and after treatment with a mold phytase. Poult. Sci. 47, 1842–1848. doi: 10.3382/ps.0471842
Noorbatcha, I. A., Sultan, A. M., Salleh, H. M., and Amid, A. (2013). Understanding thermostability factors of Aspergillus niger PhyA phytase: a molecular dynamics study. Protein J. 32, 309–316. doi: 10.1007/s10930-013-9489-y
Oakley, A. J. (2010). The structure of Aspergillus niger phytase PhyA in complex with a phytate mimetic. Biochem. Biophys. Res. Commun. 397, 745–749. doi: 10.1016/j.bbrc.2010.06.024
Pandey, A., Selvakumar, P., Soccol, C. R., and Poonam, N. (1999). Solid state fermentation for the production of industrial enzymes. Curr. Sci. 77, 149–162.
Pandey, A., Szakacs, G., Soccol, C. R., Rodriguez-Leon, J. A., and Soccol, V. T. (2001). Production, purification and properties of microbial phytases. Bioresour. Technol. 77, 203–214. doi: 10.1016/S0960-8524(00)00139-5
Papagianni, M., Nokes, S., and Filer, K. (1999). Production of phytase by Aspergillus niger in submerged and solid-state fermentation. Process Biochem. 35, 397–402. doi: 10.1016/S0032-9592(99)00088-6
Papagianni, M., Nokes, S. E., and Filer, K. (2001). Submerged and solid-state phytase fermentation by Aspergillus niger: effects of agitation and medium viscosity on phytase production, fungal morphology and inoculum performance. Food Technol. Biotechnol. 39, 319–326.
Parashar, D., and Satyanarayana, T. (2016). Enhancing the production of recombinant acidic α-amylase and phytase in Pichia pastoris under dual promoters [constitutive (GAP) and inducible (AOX)] in mixed fed batch high cell density cultivation. Process Biochem. 51, 1315–1322. doi: 10.1016/j.procbio.2016.07.027
Pen, J., Venvoerd, T. C., van Paridon, P. A., Beudeker, R. F., VandenElzen, P. J. M., Geerse, K., et al. (1993). Phytase containing transgenic seeds as a novel feed additive for improved phosphorus utilization. Biotechnology 11, 811–814. doi: 10.1038/nbt0793-811
Peng, R. H., Yao, Q. H., Xiong, A. S., Cheng, Z. M., and Li, Y. (2006). Codon-modifications and an endoplasmic reticulum-targeting sequence additively enhance expression of an Aspergillus phytase gene in transgenic canola. Plant Cell Rep. 25, 124–132. doi: 10.1007/s00299-005-0036-y
Qasim, S. S., Shakir, K. A., Al-Shaibani, A. B., and Walsh, M. K. (2017). Optimization of culture conditions to produce phytase from Aspergillus tubingensis SKA. Food Sci. Nutr. 8, 733–745. doi: 10.4236/fns.2017.87052
Quan, C. S., Tian, W. J., Fan, S. D., and Kikuchi, J. I. (2004). Purification and properties of a low-molecular-weight phytase from Cladosporium sp. FP-1. J. Biosci. Bioeng. 97, 260–266. doi: 10.1016/S1389-1723(04)70201-7
Ramachandran, S., Roopesh, K., Nampoothiri, K. M., Szakacs, G., and Pandey, A. (2005). Mixed substrate fermentation for the production of phytase by Rhizopus spp. using oilcakes as substrates. Process Biochem. 40, 1749–1754. doi: 10.1016/j.procbio.2004.06.040
Ranjan, B., and Satyanarayana, T. (2016). Recombinant HAP phytase of the thermophilic mold Sporotrichum thermophile: expression of the codon-optimized phytase gene in Pichia pastoris and applications. Mol. Biotechnol. 58, 137–147. doi: 10.1007/s12033-015-9909-7
Ranjan, B., Singh, B., and Satyanarayana, T. (2015). Characteristics of recombinant phytase (rSt-Phy) of the thermophilic mold Sporotrichum thermophile and its applicability in dephytinizing foods. Appl. Biochem. Biotechnol. 177, 1753–1766. doi: 10.1007/s12010-015-1851-4
Raveendran, S., Parameswaran, B., Ummalyma, S. B., Abraham, A., Mathew, A. K., Madhavan, A., et al. (2018). Applications of microbial enzymes in food industry. Food Technol. Biotech. 56, 16–30. doi: 10.17113/ftb.56.01.18.5491
Reddy, C. S., Kim, S. C., and Kaul, T. (2017). Genetically modified phytase crops role in sustainable plant and animal nutrition and ecological development: a review. 3 Biotech. 7, 195–195. doi: 10.1007/s13205-017-0797-3
Richardson, A. E., Hadobas, P. A., and Hayes, J. E. (2001). Extracellular secretion of Aspergillus phytase from Arabidopsis roots enables plants to obtain phosphorus from phytate. Plant J. 25, 641–649. doi: 10.1046/j.1365-313x.2001.00998.x
Rodriguez-Fernandez, D. E., Angel Rodriguez-Leon, J. A., de Carvalho, J. C., Thomaz-Soccol, V., Luis Parada, J. L., and Soccol, C. R. (2010). Recovery of phytase produced by solid-state fermentation on citrus peel. Braz. Arch. Biol. Technol. 53, 1487–1496. doi: 10.1590/S1516-89132010000600026
Rodriguez-Fernandez, D. E., Parada, J. L., Medeiros, A. B., de Carvalho, J. C., Lacerda, L. G., Rodriguez-Leon, J. A., et al. (2013). Concentration by ultrafiltration and stabilization of phytase produced by solid-state fermentation. Process Biochem. 48, 374–379. doi: 10.1016/j.procbio.2012.12.021
Rodriguez-Fernandez, D. E., Rodriguez-Leon, J. A., De Carvalho, J. C., Karp, S. G., Sturm, W., Parada, J. L., et al. (2012). Influence of airflow intensity on phytase production by solid-state fermentation. Bioresour. Technol. 118, 603–606. doi: 10.1016/j.biortech.2012.05.032
Roopesh, K., Ramachandran, S., Nampoothiri, K. M., Szakacs, G., and Pandey, A. (2006). Comparison of phytase production on wheat bran and oilcakes in solid-state fermentation by Mucor racemosus. Bioresour. Technol. 97, 506–511. doi: 10.1016/j.biortech.2005.02.046
Rosell, C. M., Santos, E., Penella, J. M. S., and Haros, M. (2009). Wholemeal wheat bread: a comparison of different breadmaking processes and fungal phytase addition. J. Cereal Sci. 50, 272–277. doi: 10.1016/j.jcs.2009.06.007
Sabu, A., Sarita, S., Pandey, A., Bogar, B., Szakacs, G., and Soccol, C. R. (2002). Solid-state fermentation for production of phytase by Rhizopus oligosporus. Appl. Biochem. Biotech. 102, 251–260. doi: 10.1385/ABAB:102-103:1-6:251
Saithi, S., and Tongta, A. (2016). Phytase production of Aspergillus niger on soybean meal by solid-state fermentation using a rotating drum bioreactor. Agric. Agric. Sci. Procedia 11, 25–30. doi: 10.1016/j.aaspro.2016.12.005
Salmon, D. N., Piva, L. C., Binati, R. L., Vandenberghe, L. P. d. S., Soccol, V. T., Soccol, C. R., et al. (2011). Formulated products containing a new phytase from Schyzophyllum sp. phytase for application in feed and food processing. Braz. Arch. Biol. Technol. 54, 1069–1074. doi: 10.1590/S1516-89132011000600001
Salmon, D. N. X., Fendrich, R. C., Cruz, M. A., Montibeller, V. W., Vandenberghe, L. P. S., Soccol, C. R., et al. (2016). Bioprocess for phytase production by Ganoderma sp. MR-56 in different types of bioreactors through submerged cultivation. Biochem. Eng. J. 114, 288–297. doi: 10.1016/j.bej.2016.07.015
Salmon, D. N. X., Piva, L. C., Binati, R. L., Rodrigues, C., de Souza Vandenberghe, L. P., Soccol, C. R., et al. (2012). A bioprocess for the production of phytase from Schizophyllum commune: studies of its optimization, profile of fermentation parameters, characterization and stability. Bioproc. Biosyst. Eng. 35, 1067–1079. doi: 10.1007/s00449-012-0692-6
Sandhya, A., Sridevi, A., Suvarnalatha, D., and Narasimha, G. (2015). Production and optimization of phytase by Aspergillus niger. Pharm. Lett. 7, 148–153.
Sanni, D. M., Lawal, O. T., and Enujiugha, V. N. (2019). Purification and characterization of phytase from Aspergillus fumigatus isolated from African Giant Snail (Achatina fulica). Biocatal. Agric. Biotechnol. 17, 225–232. doi: 10.1016/j.bcab.2018.11.017
Sariyska, M. V., Gargova, S. A., Koleva, L. A., and Angelov, A. I. (2005). Aspergillus niger phytase: purification and characterization. Biotechnol. Biotechnol. Equip. 19, 98–105. doi: 10.1080/13102818.2005.10817235
Selle, P. H., and Ravindran, V. (2008). Phytate-degrading enzymes in pig nutrition. Livest. Sci. 113, 99–122. doi: 10.1016/j.livsci.2007.05.014
Seynaeve, M., Janssens, G., Hesta, M., Van Nevel, C., and De Wilde, R. O. (2000). Effects of dietary Ca/P ratio, P level and microbial phytase supplementation on nutrient digestibilities in growing pigs: breakdown of phytic acid, partition of P and phytase activity along the intestinal tract. J. Anim. Physiol. Anim. Nutr. 83, 193–204. doi: 10.1046/j.1439-0396.2000.00246.x
Shah, P. C., Kumar, V. R., Dastager, S. G., and Khire, J. M. (2017). Phytase production by Aspergillus niger NCIM 563 for a novel application to degrade organophosphorus pesticides. AMB Express 7, 1–11. doi: 10.1186/s13568-017-0370-9
Shahryari, Z., Fazaelipoor, M. H., Setoodeh, P., Nair, R. B., Taherzadeh, M. J., and Ghasemi, Y. (2018). Utilization of wheat straw for fungal phytase production. Int. J. Recycl. Org. Waste Agric. 7, 345–355. doi: 10.1007/s40093-018-0220-z
Shin, S., Ha, N. C., Oh, B. C., Oh, T. K., and Oh, B. H. (2001). Enzyme mechanism and catalytic property of β propeller phytase. Structure 9, 851–858. doi: 10.1016/S0969-2126(01)00637-2
Shivanna, G. B., and Venkateswaran, G. (2014). Phytase production by Aspergillus niger CFR 335 and Aspergillus ficuum SGA 01 through submerged and solid-state fermentation. Sci. World J. 2014, 1–6. doi: 10.1155/2014/392615
Simon, O., and Igbasan, F. (2002). In vitro properties of phytases from various microbial origins. J. Food Sci. Technol. 37, 813–822. doi: 10.1046/j.1365-2621.2002.00621.x
Simons, P. C. M., Versteegh, H. A. J., Jongbloed, A. W., Kemme, P. A., Slump, P., Bos, K. D., et al. (1990). Improvement of phosphorus availability by microbial phytase in broilers and pigs. Br. J. Nutr. 64, 525–540. doi: 10.1079/BJN19900052
Singh, B. (2018). Engineering fungal morphology for enhanced production of hydrolytic enzymes by Aspergillus oryzae SBS50 using microparticles. 3 Biotech. 8, 1–7. doi: 10.1007/s13205-018-1308-x
Singh, B., Kunze, G., and Satyanarayana, T. (2011). Developments in biochemical aspects and biotechnological applications of microbial phytases. Biotechnol. Mol. Biol. Rev. 6, 69–87.
Singh, B., and Satyanarayana, T. (2008). Improved phytase production by a thermophilic mould Sporotrichum thermophile in submerged fermentation due to statistical optimization. Bioresour. Technol. 99, 824–830. doi: 10.1016/j.biortech.2007.01.007
Singh, B., and Satyanarayana, T. (2010). Plant growth promotion by an extracellular HAP-phytase of a thermophilic mold Sporotrichum thermophile. Appl. Biochem. Biotechnol. 160, 1267–1276. doi: 10.1007/s12010-009-8593-0
Singh, B., and Satyanarayana, T. (2011). “Plant growth promotion by phytases and phytase-producing microbes due to amelioration in phosphorus availability,” in Microorganisms in Sustainable Agriculture and Biotechnology, eds T. Satyanarayana and B. N. Johri, (Dordrecht: Springer), 3–15. doi: 10.1007/978-94-007-2214-9_1
Singh, B., and Satyanarayana, T. (2014). Fungal phytases: characteristics and amelioration of nutritional quality and growth of non-ruminants. J. Anim. Physiol. Anim. Nutr. 99, 646–660. doi: 10.1111/jpn.12236
Singh, B., Sharma, K. K., Kumari, A., Kumar, A., and Gakhar, S. K. (2018). Molecular modeling and docking of recombinant HAP-phytase of a thermophilic mould Sporotrichum thermophile reveals insights into molecular catalysis and biochemical properties. Int. J. Biol. Macromol. 115, 501–508. doi: 10.1016/j.ijbiomac.2018.04.086
Singh, R., Kumar, M., Mittal, A., and Mehta, P. K. (2016). Microbial enzymes: industrial progress in 21st century. 3 Biotech 6, 1–15. doi: 10.1007/s13205-016-0485-8
Soccol, C. R., da Costa, E. S. F., Letti, L. A. J., Karp, S. G., Woiciechowski, A. L., and de Souza Vandenberghe, L. P. (2017). Recent developments and innovations in solid state fermentation. Biotechnol. Res. Innov. 1, 52–71. doi: 10.1016/j.biori.2017.01.002
Spier, M. R., Greiner, R., Angel Rodriguez-Leon, J. A., Woiciechowski, A. L., Pandey, A., Soccol, V. T., et al. (2008). Phytase production using citric pulp and other residues of the agroindustry in SSF by fungal isolates. Food Technol. Biotechnol. 46, 178–182.
Spier, M. R., Salmon, D. N., Binati, R. L., Piva, L. C., Medeiros, A. B., and Soccol, C. R. (2012). Stability of new macromycetes phytases under room, cooling and freezing temperatures of storage. World Acad. Sci. Eng. Technol. 6, 427–431.
Suzuki, U., Yoshimura, K., and Takaishi, M. (1907). Ueber ein enzym “phytase” das “anhydro-oxy-methylen diphosphorsaure” spaltet. Bull. Coll. Agric. Tokyo Imper. Univ. 7, 503–512.
Tarafdar, J. C., and Gharu, A. (2006). Mobilization of organic and poorly soluble phosphates by Chaetomium globosum. Appl. Soil Ecol. 32, 273–283. doi: 10.1016/j.apsoil.2005.08.005
Tarafdar, J. C., and Rao, A. V. (1996). Contribution of Aspergillus strains to acquisition of phosphorus by wheat (Triticum aestivum L.) and chick pea (Cicer arietinum Linn.) grown in a loamy sand soil. Appl. Soil Ecol. 3, 109–114. doi: 10.1016/0929-1393(95)00084-4
Thakur, N., Kumar, P., and Chand, D. (2017). Enhanced production of phytase from thermotolerant Aspergillus fumigatus isolated from rhizospheric zone of maize fields. J. Innov. Pharm. Biol. Sci. 4, 114–120.
Tseng, Y. H., Fang, T. J., and Tseng, S. M. (2000). Isolation and characterization of a novel phytase from Penicillium simplicissimum. Folia Microbiol. 45, 121–127. doi: 10.1007/BF02817409
Turk, M., and Sandberg, A. S. (1992). Phytate degradation during breadmaking: effect of phytase addition. J. Cereal Sci. 15, 281–294. doi: 10.1016/S0733-5210(09)80126-4
Uchida, H., Arakida, S., Sakamoto, T., and Kawasaki, H. (2006). Expression of Aspergillus oryzae phytase gene in Aspergillus oryzae RIB40 niaD-. J. Biosci. Bioeng. 102, 564–567. doi: 10.1263/jbb.102.564
Ullah, A. H. J., Sethumadhavan, K., Mullaney, E. J., Ziegelhoffer, T., and Austin-Phillips, S. (2003). Fungal phyA gene expressed in potato leaves produces active and stable phytase. Biochem. Biophys. Res. Commun. 306, 603–609. doi: 10.1016/S0006-291X(03)01002-7
Van Etten, R. L. (1982). Human prostatic acid phosphatase: a histidine phosphatase. Ann. N. Y. Acad. Sci. 390, 27–51. doi: 10.1111/j.1749-6632.1982.tb40302.x
Vasudevan, U. M., Jaiswal, A. K., Shyam, K., and Pandey, A. (2019). Thermostable phytase in feed and fuel industries. Bioresour. Technol. 278, 400–407. doi: 10.1016/j.biortech.2019.01.065
Vasudevan, U. M., Shyam, K., Vidya, J., and Pandey, A. (2017). Microbial phytase: impact of advances in genetic engineering in revolutionizing its properties and applications. Bioresour. Technol. 245, 1790–1799. doi: 10.1016/j.biortech.2017.05.060
Vats, P., and Banerjee, U. C. (2002). Studies on the production of phytase by a newly isolated strain of Aspergillus niger var teigham obtained from rotten wood-logs. Process Biochem. 38, 211–217. doi: 10.1016/S0032-9592(02)00079-1
Vats, P., and Banerjee, U. C. (2005). Biochemical characterisation of extracellular phytase (myo-inositol hexakisphosphate phosphohydrolase) from a hyper-producing strain of Aspergillus niger van Teighem. J. Ind. Microbiol. Biotechnol. 32, 141–147. doi: 10.1007/s10295-005-0214-5
Vohra, A., and Satyanarayana, T. (2003). Phytases: microbial surces, production, purification, and potential biotechnological applications. Crit. Rev. Biotechnol. 23, 29–60. doi: 10.1080/713609297
Wang, Y., Ye, X., Ding, G., and Xu, F. (2013). Overexpression of phyA and appA genes improves soil organic phosphorus utilisation and seed phytase activity in Brassica napus. PLoS One 8:e60801. doi: 10.1371/journal.pone.0060801
Weaver, J. D., Ullah, A. H., Sethumadhavan, K., Mullaney, E. J., and Lei, X. G. (2009). Impact of assay conditions on activity estimate and kinetics comparison of Aspergillus niger PhyA and Escherichia coli AppA2 phytases. J. Agric. Food Chem. 57, 5315–5320. doi: 10.1021/jf900261n
Whipps, J. M. (1990). “Carbon economy,” in The Rhizosphere, ed. J. M. Lynch, (Chichester: John Wiley and Sons Ltd.), 59–97.
Wodzinski, R. J., and Ullah, A. H. J. (1996). “Phytase,” in Advances in Applied Microbiology, eds S. L. Neidleman and A. I. Laskin, (Cambridge, MA: Academic Press), 263–302.
Woyengo, T. A., and Wilson, J. (2019). Enhancing nutrient utilization in maize for broiler chickens by superdosing phytase. Anim. Feed Sci. Technol. 252, 34–40. doi: 10.1016/j.anifeedsci.2019.04.005
Wyss, M., Brugger, R., Kronenberger, A., Remy, R., Fimbel, R., Oesterhelt, G., et al. (1998). Biochemical characterization of fungal phytases (myo-inositol hexakisphosphate phosphohydrolases): catalytic properties. Appl. Environ. Microbiol. 65, 367–373. doi: 10.1128/aem.65.2.367-373.1999
Wyss, M., Brugger, R., Kronenberger, A., Remy, R., Fimbel, R., Oesterhelt, G., et al. (1999). Biochemical characterization of fungal phytases (myo-inositol hexakisphosphate phosphohydrolases): catalytic properties. Appl. Environ. Microbiol. 65, 367–373. doi: 10.1128/aem.65.2.367-373.1999
Xiang, T., Liu, Q., Deacon, A. M., Koshy, M., Kriksunov, I. A., Lei, X. G., et al. (2004). Crystal structure of a heat-resilient phytase from Aspergillus fumigatus, carrying a phosphorylated histidine. J. Mol. Biol. 339, 437–445. doi: 10.1016/j.jmb.2004.03.057
Xu, L., Zhang, G., Wang, H., and Ng, T. B. (2012). Purification and characterization of phytase with a wide pH adaptation from common edible mushroom Volvariella volvacea (Straw mushroom). Indian J. Biochem. Biophys. 49, 49–54.
Yan, W., Reigh, R. C., and Xu, Z. (2002). Effects of fungal phytase on utilization of dietary protein and minerals, and dephosphorylation of phytic acid in the alimentary tract of channel catfish Ictalurus punctatus fed an all-plant-protein diet. J. World Aquacult. Soc. 33, 10–22. doi: 10.1111/j.1749-7345.2002.tb00473.x
Yao, M. Z., Zhang, Y. H., Lu, W. L., Hu, M. Q., Wang, W., and Liang, A. H. (2011). Phytases: crystal structures, protein engineering and potential biotechnological applications. J. Appl. Microbiol. 112, 1–14. doi: 10.1111/j.1365-2672.2011.05181.x
Yi, Z., and Kornegay, E. T. (1996). Sites of phytase activity in the gastrointestinal tract of young pigs. Anim. Feed Sci. Technol. 61, 361–368. doi: 10.1016/0377-8401(96)00959-5
Yoon, S. M., Kim, S. Y., Li, K. F., Yoon, B. H., Choe, S., and Kuo, M. M. (2011). Transgenic microalgae expressing Escherichia coli AppA phytase as feed additive to reduce phytate excretion in the manure of young broiler chicks. Appl. Microbiol. Biotechnol. 91, 553–563. doi: 10.1007/s00253-011-3279-2
Zhang, G. Q., Chen, Q. J., Sun, J., Wang, H. X., and Han, C. H. (2013a). Purification and characterization of a novel acid phosphatase from the split gill mushroom Schizophyllum commune. J. Basic Microbiol. 53, 868–875. doi: 10.1002/jobm.201200218
Zhang, G. Q., Dong, X. F., Wang, Z. H., Zhang, Q., Wang, H. X., and Tong, J. M. (2010). Purification, characterization, and cloning of a novel phytase with low pH optimum and strong proteolysis resistance from Aspergillus ficuum NTG-23. Bioresour. Technol. 101, 4125–4131. doi: 10.1016/j.biortech.2010.01.001
Zhang, G. Q., Wu, Y. Y., Ng, T. B., Chen, Q. J., and Wang, H. X. (2013b). A phytase characterized by relatively high pH tolerance and thermostability from the shiitake mushroom Lentinus edodes. Biomed. Res. Int. 2013, 1–7. doi: 10.1155/2013/540239
Zhang, L., An, L., Gao, X., and Wang, Y. (2003). Properties of A. ficuum AS3. 324 phytase expressed in tobacco. Process Biochem. 40, 213–216. doi: 10.1016/j.procbio.2003.12.005
Zhang, S., Gan, Y., and Xu, B. (2016). Application of plant-growth-promoting fungi Trichoderma longibrachiatum T6 enhances tolerance of wheat to salt stress through improvement of antioxidative defense system and gene expression. Front. Plant Sci. 7:1405. doi: 10.3389/fpls.2016.01405
Zhang, W., Mullaney, E. J., and Lei, X. G. (2007). Adopting selected hydrogen bonding and ionic interactions from Aspergillus fumigatus phytase structure improves the thermostability of Aspergillus niger PhyA phytase. Appl. Environ. Microbiol. 73, 3069–3076. doi: 10.1128/AEM.02970-06
Zhang, Z. Y. (1998). Protein-tyrosine phosphatases: biological function, structural characteristics, and mechanism of catalysis. Crit. Rev. Biochem. Mol. Biol. 33, 1–52. doi: 10.1080/10409239891204161
Zhao, Q., Liu, H., Zhang, Y., and Zhang, Y. (2010). Engineering of protease-resistant phytase from Penicillium sp.: high thermal stability, low optimal temperature and pH. J. Biosci. Bioeng. 110, 638–645. doi: 10.1016/j.jbiosc.2010.08.003
Zhou, S., Liu, Z., Xie, W., Yu, Y., Ning, C., Yuan, M., et al. (2019). Improving catalytic efficiency and maximum activity at low pH of Aspergillus neoniger phytase using rational design. Int. J. Biol. Macromol. 131, 1117–1124. doi: 10.1016/j.ijbiomac.2019.03.140
Zhu, M. J., Wang, H. W., and Ng, T. B. (2011). Purification and identification of a phytase from fruity bodies of the winter mushroom, Flammulina velutipes. Afr. J. Biotechnol. 10, 17845–17852. doi: 10.5897/AJB10.2625
Keywords: phytase, phytase production, purification, genetic engineering, biotechnological applications
Citation: Jatuwong K, Suwannarach N, Kumla J, Penkhrue W, Kakumyan P and Lumyong S (2020) Bioprocess for Production, Characteristics, and Biotechnological Applications of Fungal Phytases. Front. Microbiol. 11:188. doi: 10.3389/fmicb.2020.00188
Received: 26 August 2019; Accepted: 27 January 2020;
Published: 14 February 2020.
Edited by:
Alberto Jiménez, University of Salamanca, SpainReviewed by:
Satyanarayana Tulasi, University of Delhi, IndiaCopyright © 2020 Jatuwong, Suwannarach, Kumla, Penkhrue, Kakumyan and Lumyong. This is an open-access article distributed under the terms of the Creative Commons Attribution License (CC BY). The use, distribution or reproduction in other forums is permitted, provided the original author(s) and the copyright owner(s) are credited and that the original publication in this journal is cited, in accordance with accepted academic practice. No use, distribution or reproduction is permitted which does not comply with these terms.
*Correspondence: Saisamorn Lumyong, c2Fpc2Ftb3JuLmxAY211LmFjLnRo; c2Nib2kwMDlAZ21haWwuY29t
Disclaimer: All claims expressed in this article are solely those of the authors and do not necessarily represent those of their affiliated organizations, or those of the publisher, the editors and the reviewers. Any product that may be evaluated in this article or claim that may be made by its manufacturer is not guaranteed or endorsed by the publisher.
Research integrity at Frontiers
Learn more about the work of our research integrity team to safeguard the quality of each article we publish.