- Department of Engineering, Aarhus University, Aarhus, Denmark
Some acetogenic bacteria are capable of using solid electron donors, such as a cathode or metallic iron [Fe(0)]. Acetogens using a cathode as electron donor are of interest for novel applications such as microbial electrosynthesis, while microorganisms using Fe(0) as electron donor cause detrimental microbial induced corrosion. The capacity to use solid electron donors strongly differs between acetogenic strains, which likely relates to their extracellular electron transfer (EET) mechanism. Different EET mechanisms have been proposed for acetogenic bacteria, including a direct mechanism and a H2 dependent indirect mechanism combined with extracellular hydrogenases catalyzing the H2 evolution reaction on the cathode or Fe(0) surface. Interestingly, low H2 partial pressures often prevail during acetogenesis with solid electron donors. Hence, an additional mechanism is here proposed: the maintenance of low H2 partial pressures by microbial H2 consumption, which thermodynamically favors the H2 evolution reaction on the cathode or Fe(0) surface. This work elaborates how the H2 partial pressure affects the H2 evolution onset potential and the H2 evolution rate on a cathode, as well as the free energy change of the anoxic corrosion reaction. In addition, the H2 consumption characteristics, i.e., H2 threshold (thermodynamic limit for H2 consumption) and H2 consumption kinetic parameters, of acetogenic bacteria are reviewed and evidence is discussed for strongly different H2 consumption characteristics. Different acetogenic strains are thus expected to maintain different H2 partial pressures on a cathode or Fe(0) surface, while those that maintain lower H2 partial pressures (lower H2 threshold, higher H2 affinity) more strongly increase the H2 evolution reaction. Consequently, I hypothesize that the different capacities of acetogenic bacteria to use solid electron donors are related to differences in their H2 consumption characteristics. The focus of this work is on acetogenic bacteria, but similar considerations are likely also relevant for other hydrogenotrophic microorganisms.
Introduction
Acetogenic bacteria are a phylogenetically diverse group of microorganisms that share a unique metabolism for energy conservation and carbon fixation, i.e., the Wood–Ljungdahl pathway (Drake et al., 2008). This pathway reduces the electron acceptor CO2 with the electron donor H2 to acetyl-CoA for carbon fixation or to acetate or other organic compounds (e.g., ethanol) for energy conservation:
With ΔG0 acetogenesis the Gibbs free energy change of acetogenesis in standard conditions (pH 0, all concentrations 1 M and all partial pressures 1 atm), in contrast to ΔG0’acetogenesis (−95.6 kJ⋅mol–1) in physiological standard conditions (pH 7).
Intriguingly, some acetogens can use solid electron donors, including a cathode (Nevin et al., 2010, 2011), metallic iron [Fe(0)] (Kato et al., 2015; Philips et al., 2019) and possibly reduced minerals. The use of a cathode as electron donor is of high interest for the development of innovative bioelectrochemical technologies. Microbial electrosynthesis, for instance, is a promising process for the conversion of excess renewable electricity and CO2 into biofuels or other valuable organic compounds using acetogenic bacteria as biocatalysts (Rabaey and Rozendal, 2010; Lovley and Nevin, 2013). In contrast, microorganisms using Fe(0) as electron donor cause microbial induced corrosion, resulting in severe damage to steel infrastructure (Enning and Garrelfs, 2014). Finally, the oxidation of reduced minerals by acetogens could have a still unknown impact on global biogeochemical cycles.
Not all tested acetogens are capable of using a cathode or Fe(0) as electron donor (Table 1). The highest electron uptake rates from cathodes have been reported for Sporomusa ovata strains (Nevin et al., 2010; Aryal et al., 2017). In contrast, the well-studied strain Acetobacterium woodii is not capable of withdrawing electrons from cathodes poised at a potential of −0.4 V vs. Standard Hydrogen Electrode (SHE) (potential slightly more positive than the standard potential for H2 evolution at pH 7, see calculations below). At more negative cathode potentials (< −0.6 V vs. SHE), almost all tested acetogenic strains withdraw cathodic electrons, with the exception of Sporomusa aerivorans (Table 1). Acetogenic communities enriched on cathodes (potentials usually ≤ −0.6 V vs. SHE) are often dominated by Acetobacterium species (Table 2). Only one study has performed a metagenome analysis to identify their cathode-dominating acetogen and found a Acetobacterium wieringae strain (Marshall et al., 2017). Interestingly, similar acetogenic genera are found in enrichments using Fe(0) as electron donor (Table 2) and acetogenic strains related to Sporomusa sphaeroides and A. wieringae have been isolated with Fe(0) (Kato et al., 2015; Philips et al., 2019; Table 1). Moreover, a limited to no Fe(0) corrosion enhancement was found for A. woodii (Table 1). Consequently, acetogenic strains likely use a similar mechanism to withdraw extracellular electrons from a cathode as from Fe(0), while not all acetogens have such a mechanism.
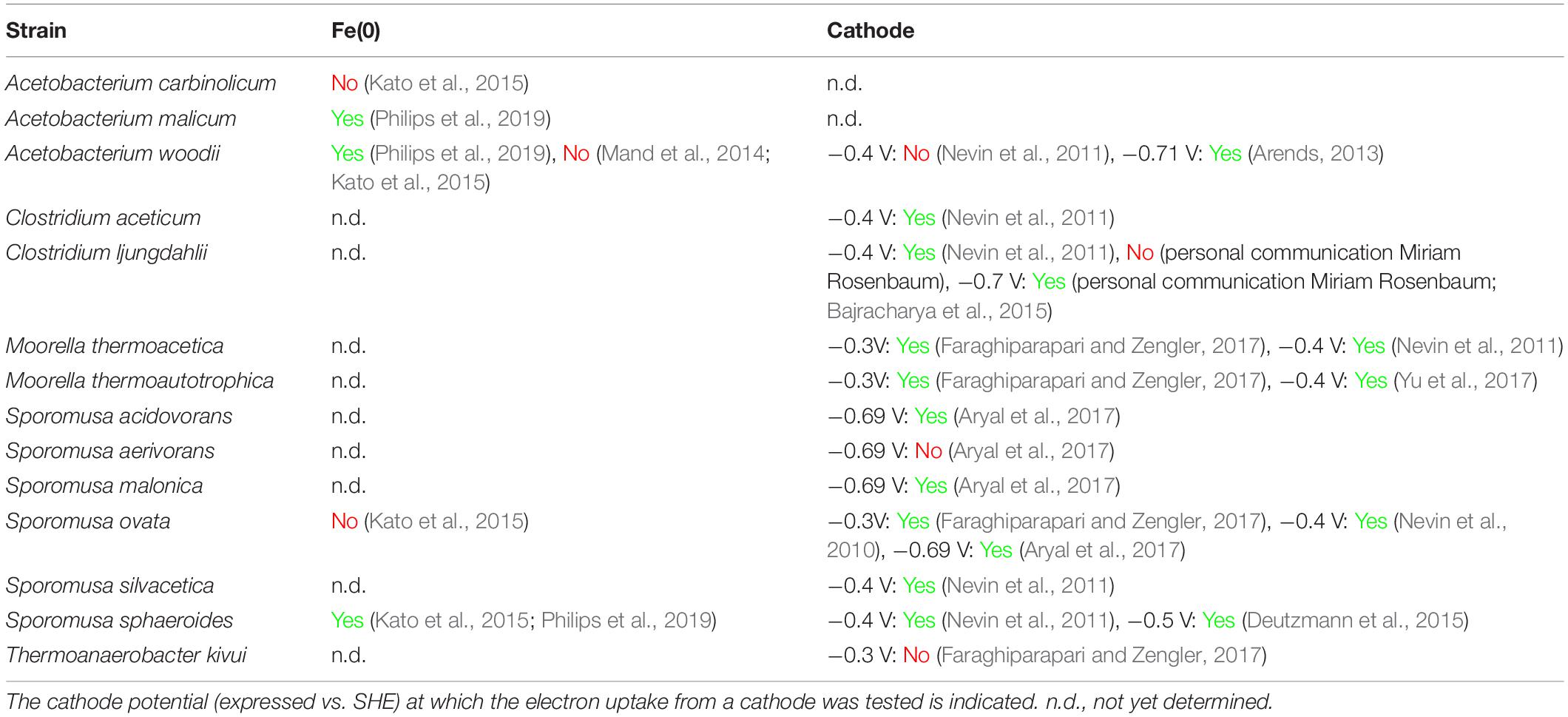
Table 1. Overview of acetogenic strains and their capacity to withdraw electrons from Fe(0) or a cathode.
This work first reviews the different extracellular electron transfer (EET) mechanisms that have been proposed for acetogenic bacteria. Next, an additional EET mechanism is proposed: the maintenance of low H2 partial pressures by H2 consumption, favoring H2 evolution by the cathode or Fe(0) surface. The H2 consumption characteristics of acetogens are further described using thermodynamic and kinetic calculations and a literature review. Finally, this work hypothesizes that the different capacities of acetogenic bacteria to use solid electron donors are related to differences in their H2 consumption characteristics. This work mainly focuses on acetogenic bacteria, but similar considerations are likely also valid for other hydrogenotrophic microorganisms.
Extracellular Electron Transfer Mechanisms of Acetogens
An overview of the different EET mechanisms that have been proposed for acetogenic bacteria is shown in Figure 1. Direct EET (Figure 1A) is a mechanism that is well-studied in microorganisms using solid electron acceptors, as for instance Geobacter spp. (Philips et al., 2016). Other microorganisms, e.g., Acidithiobacillus ferrooxidans, use a direct EET mechanism to withdraw electrons from solid electron donors (Valdes et al., 2008). A direct EET mechanism typically involves outer-membrane bound cytochromes, transporting extracellular electrons from the inside to the outside of the cell or the other way around (Philips et al., 2016). A direct extracellular electron uptake has been proposed for acetogenic bacteria (Nevin et al., 2011; Kato et al., 2015), but clear evidence is still lacking. Moreover, Moorella and Sporomusa spp. have cytochromes, but most other acetogens have not (Moller et al., 1984; Schuchmann and Müller, 2014).
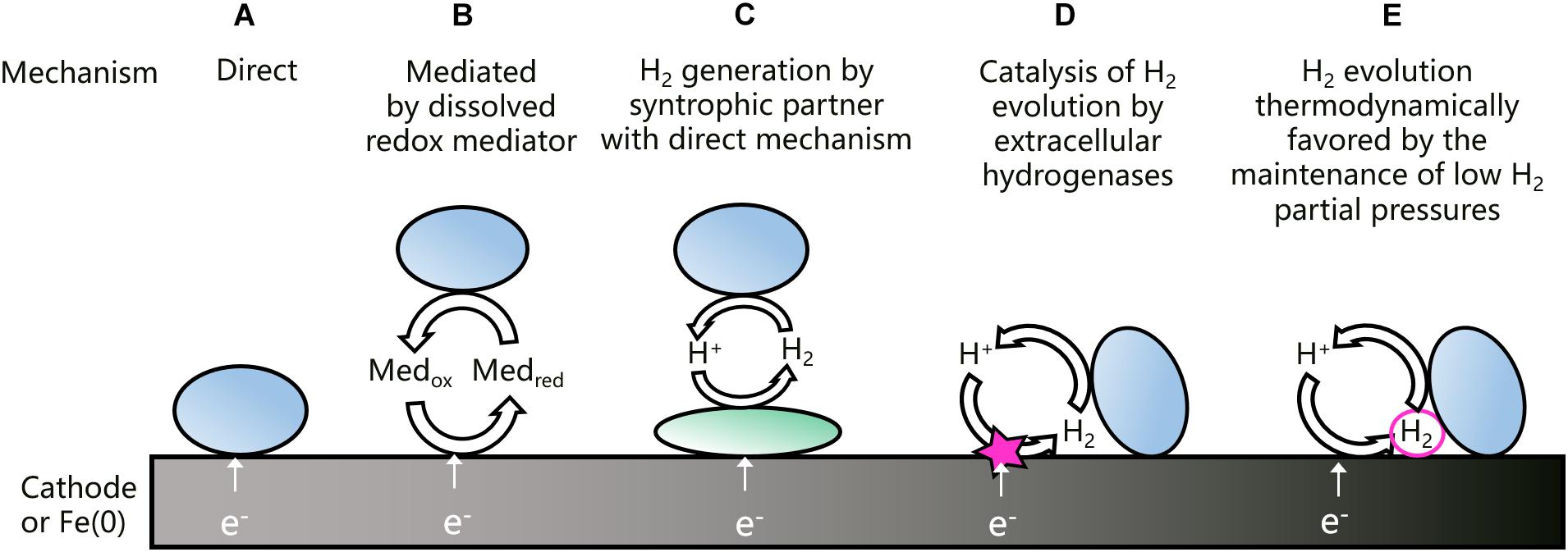
Figure 1. Schematic overview of the different EET mechanisms that have been proposed for the uptake of electrons from a cathode or Fe(0) by acetogenic bacteria (adjusted from Philips et al., 2019). Previously proposed EET mechanisms are direct (A), mediated by dissolved redox mediators (B), depended on H2 generation by a syntrophic partner with a direct mechanism (C), or depended on H2 generation catalyzed by extracellular hydrogenases (D). The last mechanism (E) is proposed and elaborated in this work.
Some microorganisms excrete redox shuttles to mediate EET (Figure 1B). Shewanella oneidensis and Pseudomonas aeruginosa, for instance, use respectively flavins and phenazines to mediate the transport of electrons to an anode (Philips et al., 2016). Artificial mediators have been applied to improve the EET of acetogens from cathodes (Song et al., 2011), but acetogens were not found to excrete redox mediators to mediate EET from Fe(0) (Philips et al., 2019).
Another possibility is an indirect EET mechanism relying on the evolution of H2 on the cathode or Fe(0). All acetogens are capable of using H2 as electron donor. In addition, a cathode at a sufficiently low potential (calculated in detail below) generates H2 through proton reduction:
Moreover, the presence of Fe(0) in anoxic conditions always leads to H2 generation through the anoxic corrosion reaction:
Nevertheless, an indirect EET mechanism depending on H2 has often been disregarded, because the acetate levels in biological treatments are often higher than can be explained by the H2 levels in abiotic controls. For instance, no H2 evolution was recorded for an abiotic cathode poised at −0.4 V vs. SHE, while several acetogenic strains consumed current and produced acetate at the same potential (Nevin et al., 2011). Similarly, some acetogens have a higher acetate production rate with Fe(0) as electron donor than can be explained just by chemically generated H2 (Kato et al., 2015; Philips et al., 2019). Consequently, an H2-dependent EET mechanism can only explain the extracellular electron uptake by acetogens, if the microorganisms somehow increase the H2 evolution on the cathode or Fe(0) surface.
Acetogens could increase H2 evolution on a cathode or Fe(0) through a syntrophic association with an electrotrophic microorganism, which produces H2 using a direct EET mechanism (Figure 1C). Such a mechanism was proposed for a cathodic microbial community dominated by Acetobacterium and Desulfovibrio spp. (Marshall et al., 2017). In addition, acetate production by A. woodii on a cathode poised at −0.4 V vs. SHE was facilitated through H2 generation by strain IS4 (Deutzmann and Spormann, 2017), i.e., a sulfate reducer isolated with Fe(0) as electron donor (Dinh et al., 2004) and possibly using cytochromes for a direct EET (Beese-Vasbender et al., 2015b).
Interestingly, some acetogenic strains can increase H2 evolution on a cathode or Fe(0), also without a syntrophic partner. Deutzmann et al. (2015) found that cell-free spent medium of S. sphaeroides increased the H2 evolution rate on a cathode poised at −0.5 V vs. SHE, while similar results were reported with Fe(0) for Sporomusa and Acetobacterium strains (Philips et al., 2019). Tremblay et al. (2019) detected H2 already at a cathode potential of −0.3 V vs. SHE with cell-free spent medium of S. ovata, while H2 could only be detected at −0.5 V vs. SHE in fresh medium. Deutzmann et al. (2015) suggested that spent medium contains extracellular enzymes, such as hydrogenases, that absorb on the cathode or Fe(0) surface and catalyze the H2 evolution reaction (Figure 1D). For the methanogen Methanococcus maripaludis, a heterodisulfide reductase supercomplex was isolated, which catalyzes the reduction of CO2 to formate at a cathode and Fe(0) surface (Lienemann et al., 2018). In addition, this methanogen excretes a [NiFe] hydrogenase to stimulate the anoxic corrosion reaction (Reaction 3) (Tsurumaru et al., 2018). So far, the H2 catalyzing components in the spent medium of acetogens have not yet been identified.
The recent evidence discussed above (Deutzmann et al., 2015; Philips et al., 2019; Tremblay et al., 2019), suggests that H2 plays an important role in the EET mechanism of acetogenic bacteria. Nevertheless, H2 can often not be detected during acetogenesis with a cathode or Fe(0) as electron donor (Jourdin et al., 2016; Philips et al., 2019). For that reason, this work proposes that the maintenance of low H2 partial pressures is an additional mechanism by which acetogens favor H2 evolution on a cathode or Fe(0) surface (Figure 1E). The importance of the H2 partial pressure for the H2 evolution reaction on a cathode or Fe(0) is elaborated next.
Low H2 Partial Pressures Favor the H2 Evolution Reaction on a Cathode and Fe(0)
Effect of the H2 Partial Pressure on Cathodic H2 Evolution
The cathode potential below which H2 evolution (Reaction 2) is thermodynamically favorable, i.e., the H2 evolution onset potential (EH^+ /H_2) (V), is given by the Nernst equation:
With R the ideal gas constant (8.314 10–3 kJ⋅mol–1⋅K–1), T the temperature (K), F the Faraday constant (96.485 kJ⋅V–1) and pH_2 the H2 partial pressure (atm) and [H+] is the proton concentration (M). Equation 1 further neglects activity coefficients, assuming that activities can be approached by concentrations. The H2 partial pressure (atm) is used throughout this work even for conditions in solution (such as at the cathode or Fe(0) surface), but can be related to the dissolved H2 concentration using the Henri constant. E°H + /H2 is the standard potential (pH 0, 1 atm H2) for H2 evolution, which is 0 V (i.e., the potential of the SHE), while E°’H^+ /H_2 is −0.414 V (pH 7, 1 atm H2).
Equation 1 demonstrates that the H2 evolution onset potential depends on the H2 partial pressure and the pH at the cathode surface (Figure 2A; Vincent et al., 2007; May et al., 2016). For instance, at a H2 partial pressure of 50 Pa (5 × 10−4 atm), the H2 evolution onset potential becomes – 0.316 V (pH 7), while at pH 5.5 [optimal pH for some acetogens (Liew et al., 2016)], the H2 evolution onset potential is – 0.325 V (1 atm H2). Consequently, H2 evolution can thermodynamically be favorable at cathode potentials less negative than −0.4 V vs. SHE, even though this potential is often used in bioelectrochemical studies to avoid H2 evolution.
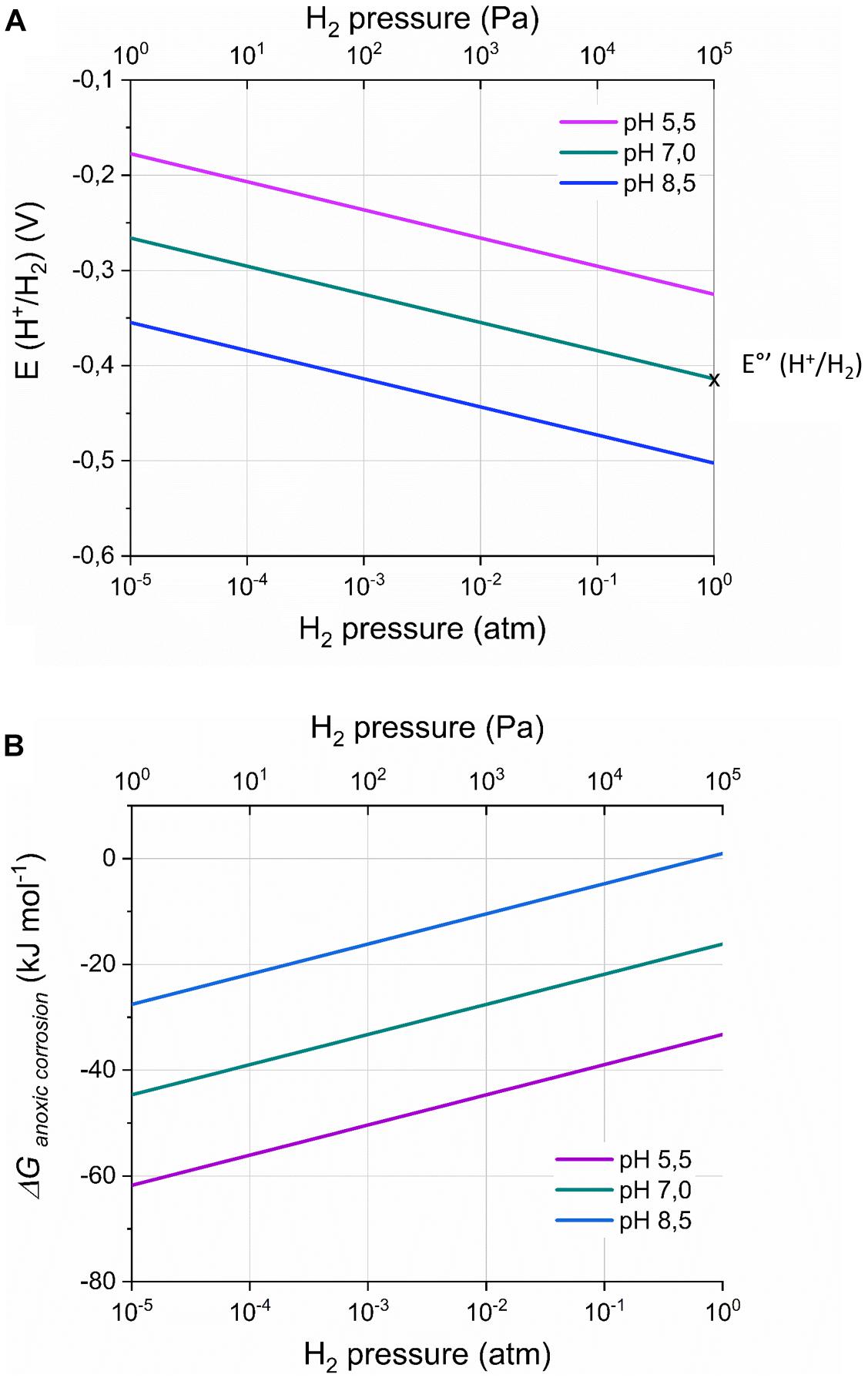
Figure 2. The onset potential for H2 evolution by a cathode E(H+/H2) (V) (A) and the Gibbs free energy change (ΔGanoxic corrosion; kJ mol– 1) of the anoxic corrosion reaction (B) in function of the H2 partial pressure and the pH, according to respectively Equations 1 and 3. The standard H2 potential at pH 7 [E°’(H+/H2)] is indicated on the graph. ΔGanoxic corrosion was calculated assuming a Fe2+ concentration of 1 mM. Remark the logarithmic scale for the H2 partial pressures.
The H2 evolution onset potential, and thus the H2 partial pressure (Equation 1), also affects the kinetics of the cathodic H2 evolution (Rheinlander et al., 2014), which can be described by the Butler–Volmer equation (Bard and Faulkner, 2001):
With j the current density (A⋅cm–2), j0 the exchange current density (A⋅cm–2), α the transfer coefficient (-) (usually approximated by 0.5) (Bard and Faulkner, 2001) and Eelectrode the potential at which the electrode is poised. The left exponential expresses the cathodic reaction (H+ to H2 reduction), while the right exponential expresses the anodic reaction (H2 to H+ oxidation). When Eelectrode is EH^+ /H_2, (thermodynamic equilibrium) both the anodic and the cathodic current density become j0, hence the net current density is zero. Remark that Equation 2 is only valid if mass transfer is not limiting, e.g., when the concentrations at the electrode surface are the same as in the bulk liquid, which is only true at electrode potentials close to EH^+ /H_2 or, in other words, at very low currents.
The importance of the H2 evolution onset potential for the current density (equivalent to the H2 evolution rate) is illustrated in Figure 3A. A less negative EH^+ /H_2 value, for instance due to a lower H2 partial pressure, allows cathodic current at less negative potentials.
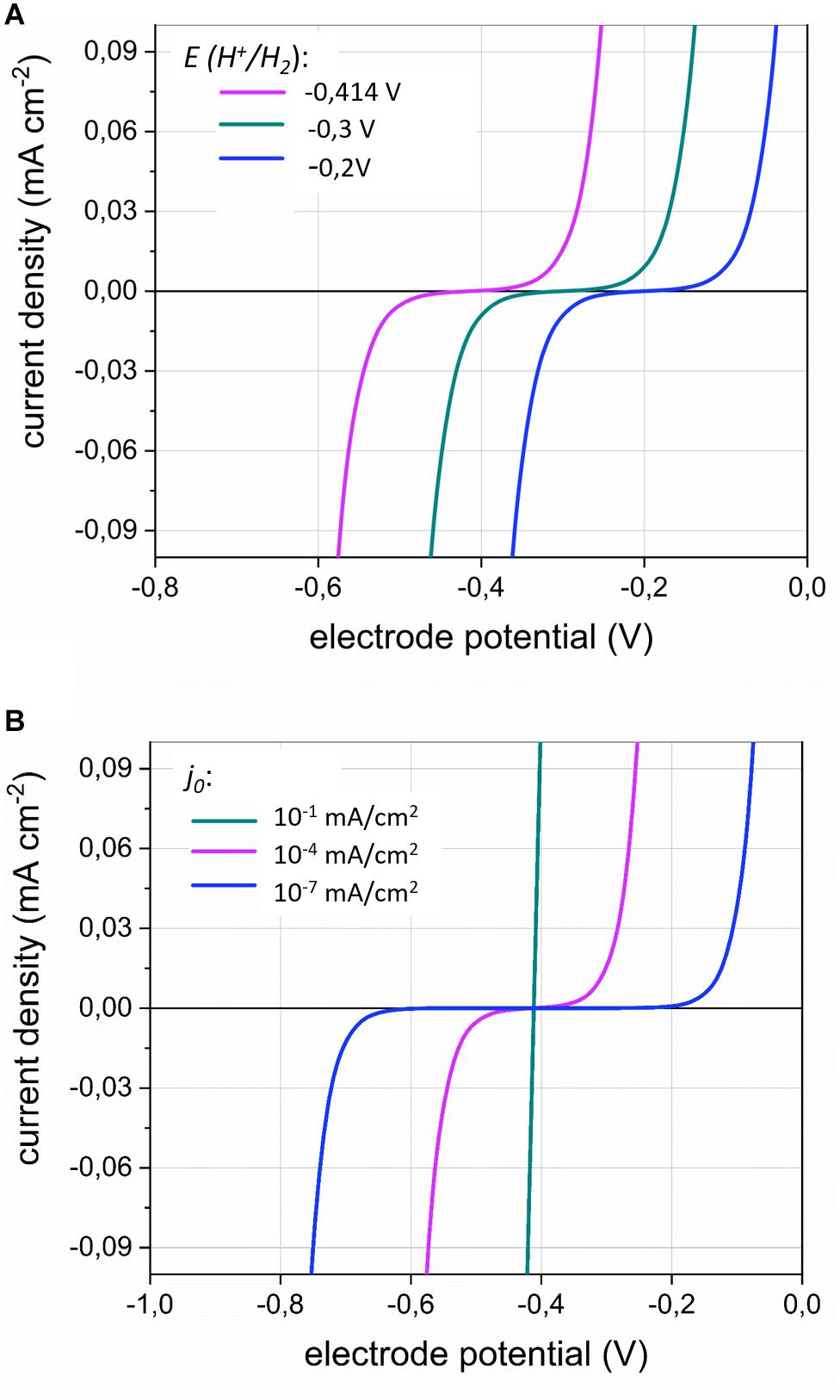
Figure 3. The current density j (mA⋅cm–2) in function of the electrode potential (V) according to the Butler–Volmer Equation (Equation 2) for (A) different values of the H2 evolution onset potential E(H+/H2); (with j0 = 1⋅10–7 mA⋅cm–2) and (B) different values of the exchange current density j0 (with E(H+/H2) = −0.414V). The transfer coefficient α was set to 0.5. The inflection point of the curves represents E(H+/H2).
In addition, the effect the exchange current density j0 is illustrated in Figure 3B. The exchange current density inversely relates to the activation overpotential (Bard and Faulkner, 2001), as a smaller exchange current density entails that a more negative electrode potential is needed to enable a substantial cathodic current (Figure 3B). In bioelectrochemical studies, the overpotentials for cathodic H2 evolution are often high (0.2 V more negative than E°’H^+ /H_2), due to the low reactivity of the often used carbon-based electrode materials. Choosing for more reactive cathode materials (materials with high j0) strongly reduces the activation overpotential of the H2 evolution reaction (Jeremiasse, 2011). Such materials facilitate the cathodic electron uptake by acetogens at less negative cathode potentials than are required with unreactive electrode materials (Kracke et al., 2019; Tian et al., 2019). Moreover, cell-free spent medium of S. ovata was also found to decrease the overpotential for cathodic H2 evolution (Tremblay et al., 2019), likely because it contains hydrogenase enzymes or other components catalyzing the H2 evolution (Figure 1D).
In summary, Equation 2 demonstrates that the current density (H2 evolution rate) depends both on the H2 evolution onset potential (and thus the H2 partial pressure; thermodynamic effect) and on the exchange current density (kinetic effect, related to the electrode material and catalysis by enzymes), when mass transfer is not limiting.
Effect of the H2 Partial Pressure on Anoxic Fe(0) Corrosion
The H2 partial pressure also affects the anoxic chemical corrosion reaction, as the Gibbs free energy change of Reaction 3 (ΔGanoxic corrosion) depends on the H2 partial pressure and pH at the Fe(0) surface (Figure 2B):
With [Fe2+] the dissolved Fe2+ concentration (M) and ΔG°anoxic corrosion the standard Gibbs free energy change (−78.9 kJ⋅mol–1, pH 0). Consequently, hydrogenotrophic microorganisms can thermodynamically favor H2 evolution on Fe(0) by maintaining low H2 partial pressures on the Fe(0) surface. For instance, A. woodii maintained a H2 partial pressure on Fe(0) of 150 Pa (1.5 × 10−3 atm) (Philips et al., 2019), leading to ΔGanoxic corrosion of −32 kJ⋅mol–1, while this is only −22 kJ⋅mol–1 in abiotic conditions (0.1 atm or 10.000 Pa H2; assuming [Fe2+] of 1 mM) (Philips et al., 2019). All other strains tested in the same study maintained lower H2 partial pressures on Fe(0) than A. woodii [below the detection limit of a TCD detector (40 Pa)] (Philips et al., 2019), thus leading to a ΔGanoxic corrosion value at least as negative as −36 kJ⋅mol–1.
Also the rate of the anoxic chemical corrosion reaction likely depends on the H2 partial pressure. In addition, Reaction 3 was found to be catalyzed by hydrogenase enzymes (Bryant and Laishley, 1990; Da Silva et al., 2004; Rouvre and Basseguy, 2016). Consequently, the rate of the anoxic corrosion reaction likely depends both on the H2 partial pressure (thermodynamic effect) and on enzymatic catalysis (kinetic effect), similar as for a cathode.
In general, the H2 partial pressure at the cathode or Fe(0) surface results from the balance (steady-state) between the H2 evolution rate on the cathode or Fe(0) and the H2 consumption rate by the microorganisms. For that reason, the H2 consumption characteristics of acetogenic bacteria are discussed next.
H2 Consumption Characteristics of Acetogenic Bacteria
The consumption of H2 by any microorganism is described by its H2 threshold (the thermodynamic limit of H2 consumption) and its H2 consumption kinetics. Below, the theoretical H2 threshold is calculated for acetogens and experimentally determined values for the H2 threshold and H2 consumption kinetic parameters of acetogens are reviewed.
The Theoretical H2 Threshold of Acetogens
Microorganisms do not completely consume their substrates due to bioenergetic constraints. The theoretical limit for H2 consumption by acetogenic bacteria is the H2 partial pressure at which Reaction 1 reaches its thermodynamic equilibrium:
With [CH3COO–] and [H+] respectively the acetate and proton concentrations (M) and pCO_2 and pH_2 respectively the CO2 and H2 partial pressures (atm). The minimum H2 partial pressure at which a reaction is thermodynamically feasible is called the H2 threshold (θH_2; atm) (Cord-Ruwisch et al., 1988) and can for acetogens be derived as:
Using pH 7, a temperature of 298 K, a CO2 partial pressure of 0.2 atm and an acetate concentration of 2 mM [i.e., relevant physiological conditions for acetogens using a cathode or Fe(0) as electron donor (Nevin et al., 2010; Kato et al., 2015; Aryal et al., 2017; Philips et al., 2019)], the H2 threshold becomes 3⋅10–5 atm or 3 Pa (30 ppm or 0.003%, assuming 1 atm total pressure). Experimental H2 thresholds for acetogens are always higher than this value (discussed below). This is likely because Reaction 1 does not account for the coupling of acetogenesis to energy conservation. Indeed, calculations of the Gibbs free energy change at experimentally derived H2 thresholds found a critical Gibbs free energy change, which was not zero but slightly negative (Seitz et al., 1990). This critical Gibbs free energy change likely reflects the energy needed for the microbial metabolism. Accordingly, Reaction 1 should be written as (Poehlein et al., 2012):
With n the number of ATP molecules gained per molecule of acetate (i.e., the ATP gain). Consequently, the expression for the H2 threshold becomes:
with ΔGADP/ATP the Gibbs free energy change for the phosphorylation of ADP to ATP in physiological conditions (also called the phosphorylation potential). Reported values for ΔGADP/ATP range between 30 and 80 kJ⋅mol–1 (Thauer et al., 1977; Atkins and De Paula, 2011). For A. woodii, a phosphorylation potential of only 32 kJ⋅mol–1 has been measured (Spahn et al., 2015), but for other acetogens this value is unknown. For the calculation of the H2 threshold of different acetogens described here, a value for ΔG°ADP/ATP of 50 kJ⋅mol–1 (Poehlein et al., 2012) was used, in order not to underestimate the H2 threshold.
Equation 6 shows that the H2 threshold depends on the ATP gain n, which is maximally 1.9 (ΔG0’acetogenesis divided by ΔGADP/ATP), but depends on the energy conservation mechanism of the acetogenic strain (Schuchmann and Müller, 2014).
All acetogenic bacteria share the Wood–Ljungdahl pathway, as the enzymes forming this pathway are highly conserved among acetogens (Schuchmann and Müller, 2014). However, the carbon flow through the Wood–Ljungdahl pathway does not lead to energy conservation. Acetogens conserve energy using chemiosmotic ion gradient-driven phosphorylation. The cation generating this gradient (Na+ or H+), the energy-conserving module (Rnf or Ech complex), as well as other components (electron bifurcating enzymes) creating the electron flow, differ between acetogenic bacteria (Schuchmann and Müller, 2014). So far, only for few model acetogenic strains the energy conservation machinery is (almost) fully unraveled and the theoretical ATP gain n has become available (Table 3). This ATP gain ranges between 0.3 for A. woodii to 1 for Clostridium autoethanogenum. Based on Equation 6, this entails that the H2 thresholds for these model strains range from 14 to 1160 Pa (including different optimal temperatures and pH) (Table 3). Moreover, based on recently sequenced genomes, it is plausible that a wide variability in the energy conservation mechanism of acetogenic strains exists (Poehlein et al., 2016). In theory, the ATP gain n could range from 0.15 (Mock et al., 2015) to maximally 1.9, entailing H2 thresholds ranging over five order of magnitudes (Figure 4). Consequently, acetogenic bacteria strongly differ in the lowest H2 partial pressure they can use. Strains with a high H2 threshold (high ATP gain) obtain high energy by performing acetogenesis, but cannot grow at low H2 partial pressures. In contrast, strains with a low H2 threshold (low ATP gain) gain low energy from acetogenesis, but have the advantage of being able to grow at low H2 partial pressures.
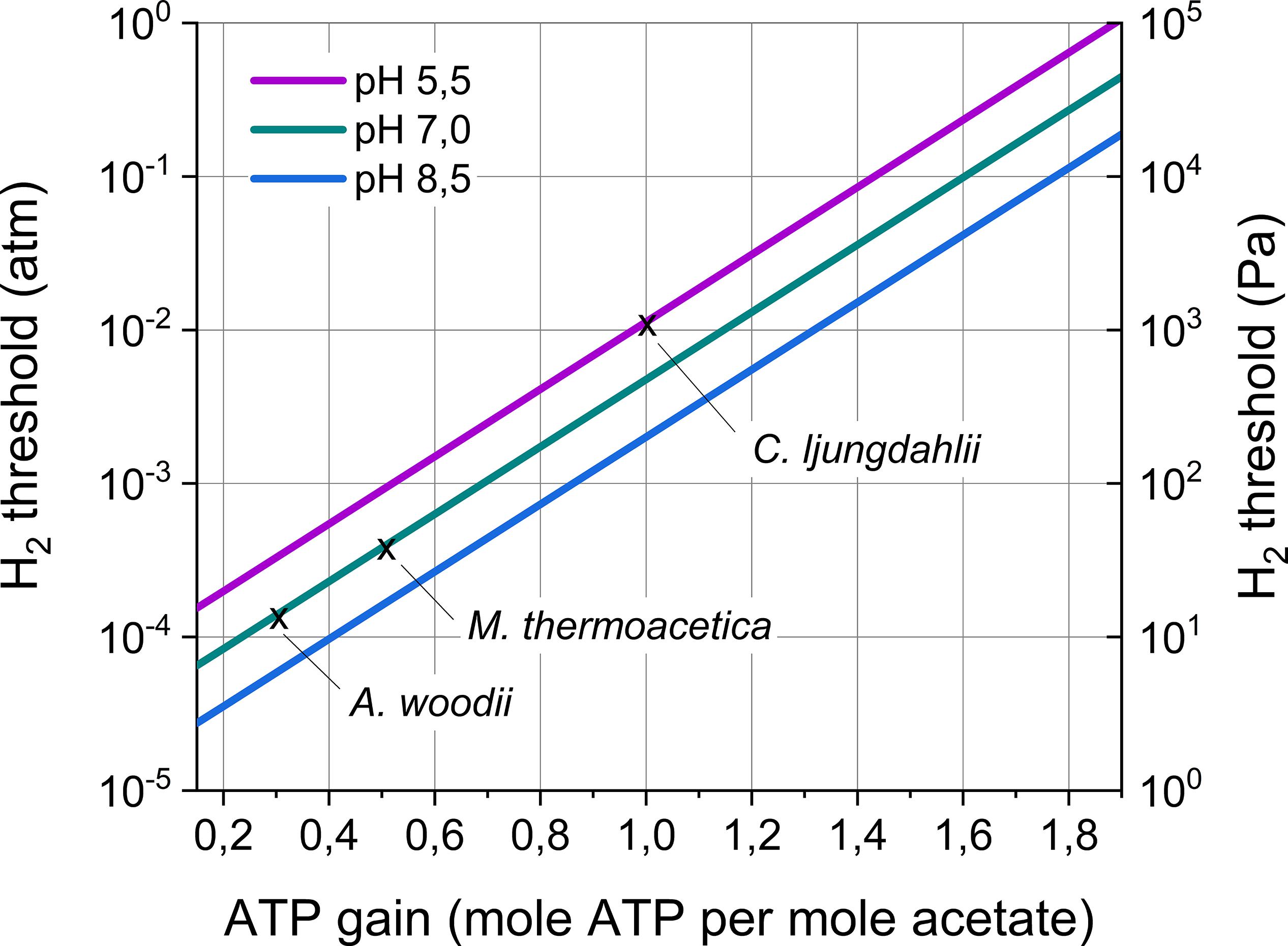
Figure 4. H2 threshold in function of the ATP gain of acetogenesis and the pH. The H2 threshold was calculated according to Equation 6 assuming an acetate concentration of 2 mM and a CO2 partial pressure of 0.2 atm. Temperature effects were not included in this graph. The H2 thresholds for three model acetogens (as in Table 3) are indicated. Remark the logarithmic scale for the H2 threshold.
Previously, differences in the H2 threshold of methanogens were similarly linked to different ATP gains (Thauer et al., 2008). The above analysis, however, only holds as long as the metabolism is coupled to energy generation, as it does not incorporate the possibility that acetogenesis continues decoupled from energy generation (Schuchmann and Müller, 2014). Moreover, the above reactions do not incorporate the consumption of H2 and CO2 for biomass formation. Furthermore, different energy conservation strategies could exist in a single strain (Mock et al., 2015) and be expressed depending on the H2 partial pressure. Consequently, further investigations of the energy conservation mechanisms of acetogenic bacteria will be highly important to better understand their H2 threshold.
Experimental H2 Thresholds of Acetogens
Experimental H2 thresholds have been reported for several acetogenic strains (Table 4). These values are usually measured as the constant H2 partial pressure that remains after H2 depletion (other nutrients not limiting) (Cord-Ruwisch et al., 1988; Poehlein et al., 2012). Reported H2 thresholds range over two orders of magnitudes (Table 4), but strong value variability was reported even for the same strain, as experimental H2 thresholds for A. woodii for instance range from 14 to 250 Pa (Table 4). This variability is likely due to varying experimental conditions, as the H2 threshold depends in theory on the CO2 partial pressure, the acetate concentration, the pH, the total pressure and the temperature (Equations 5 and 6). Conrad and Wetter (1990) and Kotsyurbenko et al. (2001) nicely demonstrated that experimental H2 thresholds followed the theoretical temperature dependence (Equation 5), as long as the temperature remained in the strain’s optimal temperature range. The effect of the other parameters on the experimental H2 thresholds has much less been studied and often these parameters are not reported together with the experimental H2 threshold values. Fortunately, some studies have used the same experimental conditions to determine the H2 threshold of different acetogenic strains and demonstrated significant differences in experimental H2 thresholds between strains (Cord-Ruwisch et al., 1988; Leclerc et al., 1997; Le Van et al., 1998).
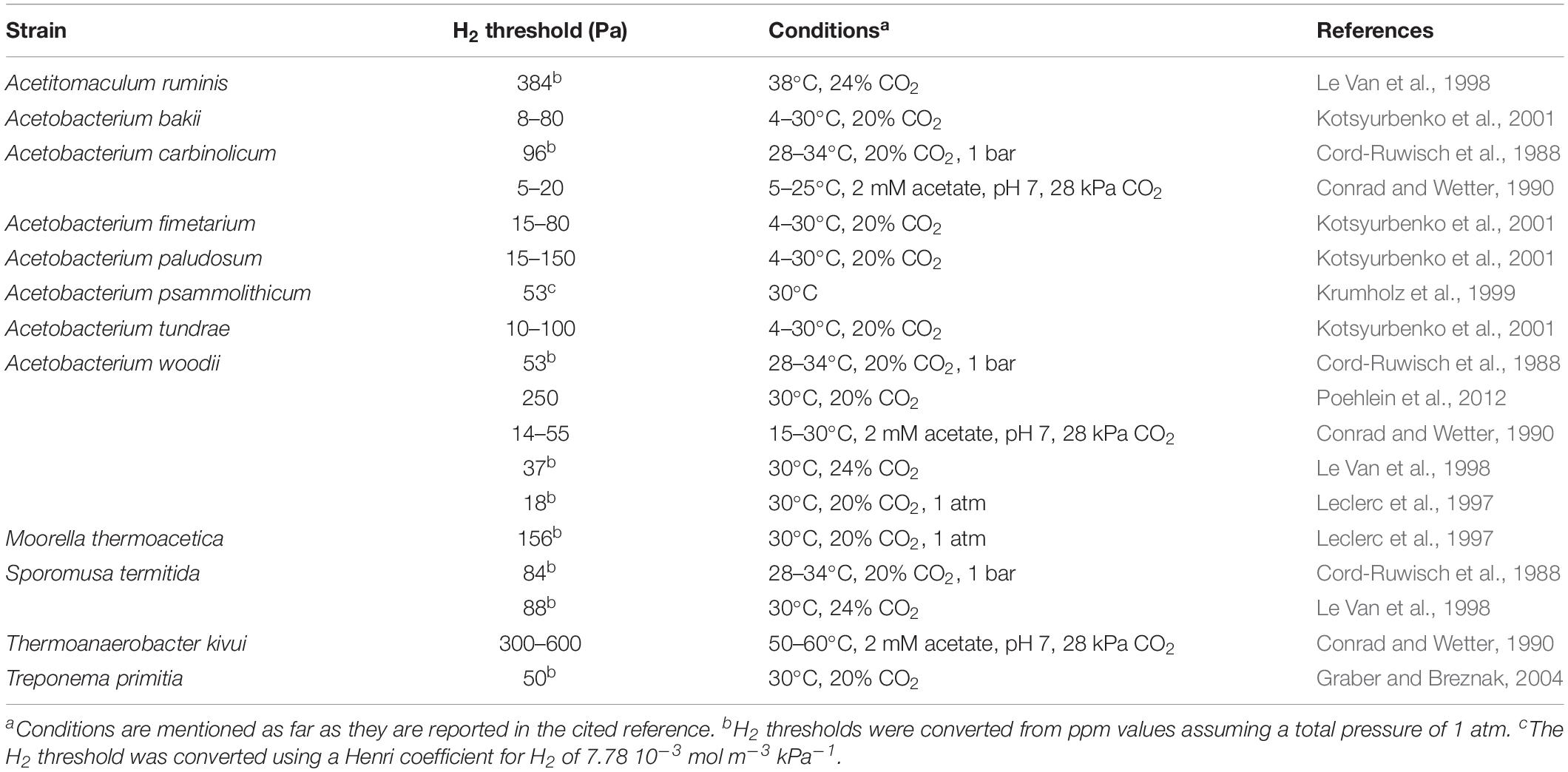
Table 4. Experimental H2 thresholds for different acetogens performing acetogenesis from CO2 and H2.
This work advocates the reporting of experimental H2 thresholds (as well as of the experimental conditions of the measurements) of H2 consuming anaerobic microorganisms, as this parameter can easily be determined and forms a highly valuable measure to assess bioenergetics, and possibly also the energy conservation mechanism, of new and already-known strains.
H2 Consumption Kinetics of Acetogens
Very limited information on the H2 consumption kinetics of acetogenic bacteria is available in literature, except for frequently reported doubling times (overview in Bengelsdorf et al., 2018). The kinetic parameters for H2 consumption were previously reported only for four acetogenic strains (Table 5). These strains strongly differ in their H2 consumption kinetics, as the maximum cell specific growth rate (μmax) differs one order of magnitude between these strains, while the Monod or half saturation constant (KH_2), i.e., a measure for the affinity of the strains for H2, ranges over more than two orders of magnitude. The importance of these different kinetic parameters becomes clear in Figure 5, plotting the cell specific growth rate (μ) in function of the H2 partial pressure according to the Monod Equation:

Table 5. Monod kinetic parameters for H2 consumption by acetogenic strains with KH_2 (Pa) the Monod or half saturation constant (i.e., a measure for the affinity of the strains for H2) and μmax the maximum cell specific growth rate (h–1).
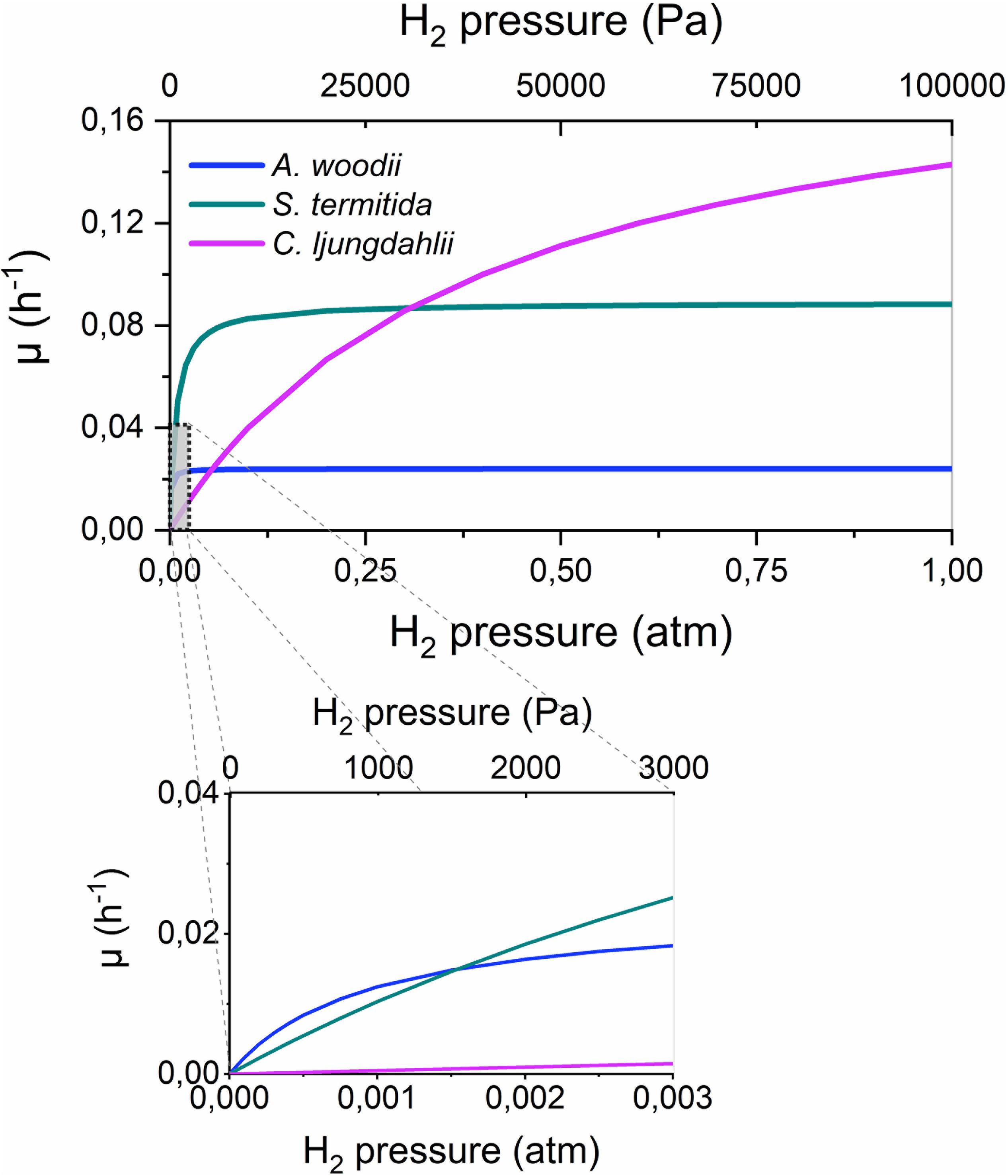
Figure 5. The cell specific growth rate (μ, h– 1) in function of the H2 partial pressure for three acetogens, according to Equation 7 and the kinetic parameters of Table 5. The H2 threshold was not incorporated in this graph.
This figure shows that strains with a high μmax, such as C. ljungdahlii, have the highest growth rate and thus a competitive advantage at high H2 partial pressures (> 0.25 atm). In contrast, at intermediate H2 partial pressures, strains with an intermediate KH_2 value, such as S. termitida, have a competitive advantage, while at very low H2 partial pressures (< 0.0015 atm), strains with a strong affinity for H2 (low KH_2), such as A. woodii, have the highest growth rate. Possibly, these differences explain why acetogenic Clostridium spp. are well suited for gas fermentations (Liew et al., 2016), while Acetobacterium and Sporomusa species are often found on cathodes or Fe(0), where low H2 partial pressures prevail (Table 2). Importantly, Figure 5 also shows that the highest growth rates are only obtained at high H2 partial pressures, possibly impeding the production rates attainable with microbial electrosynthesis in comparison to gas fermentation.
Equation 7 can further be extended to also include the H2 threshold (Kotsyurbenko et al., 2001):
This equation was not used for Figure 5, as for none of the strains, all three parameters (μmax, KH_2 and θH_2) are reported.
Discussion
Several acetogenic bacteria are capable of using solid electron donors (Tables 1, 2), implying that these strains have an extracellular electron uptake mechanism. Different EET mechanisms have been proposed for acetogenic bacteria (Figure 1). Recent evidence suggests that an H2-dependent indirect EET mechanism is combined with a mechanism to increase the H2 evolution reaction rate on the cathode or Fe(0) surface (Deutzmann et al., 2015; Jourdin et al., 2016; Tremblay et al., 2019). In addition, low H2 partial pressures often prevail during acetogenesis with a solid electron donor (< 50 Pa) (Nevin et al., 2010; Deutzmann et al., 2015; Jourdin et al., 2016; Philips et al., 2019). This work explained that the H2 partial pressure affects the H2 evolution onset potential (Equation 1 and Figure 2A) and the H2 evolution rate (current) (Equation 2 and Figure 3A) at a cathode, as well the Gibbs free energy change of the anoxic corrosion reaction (Equation 3 and Figure 2B) and likely also the rate of the corrosion reaction. Consequently, hydrogenotrophic microorganisms could favor the H2 evolution reaction by maintaining low H2 partial pressures at the cathode or Fe(0) surface (Figure 1E).
The steady-state H2 partial pressure at the material surface results from the balance between the H2 evolution reaction and microbial H2 consumption. Here, the H2 consumption characteristics of acetogenic bacteria were reviewed, which suggested that acetogens differ in their H2 threshold (thermodynamic limit for H2 consumption) (Figure 4 and Tables 3, 4) and their H2 consumption kinetics (Figure 5 and Table 5). This entails that different acetogens likely maintain different H2 partial pressures on the surface of a cathode or Fe(0). Therefore, I hypothesize that acetogens that maintain lower H2 pressures (strains with a lower H2 threshold and/or higher H2 affinity) more strongly increase the H2 evolution reaction on a cathode or Fe(0). Consequently, the differences in the capacities of acetogenic bacteria to use solid electron donors (Table 1) could be related to differences in their H2 consumption characteristics.
The lowest theoretical H2 threshold and highest H2 affinity (lowest KH_2) was reported for A. woodii (Tables 3, 5). This contradicts with my hypothesis, as A. woodii is not capable of withdrawing cathodic electrons at a cathode potential of −0.4 V vs. SHE and does not increase Fe(0) corrosion or just to a limited extent (Table 1). However, information on the H2 consumption characteristics of acetogens is limited to just a few strains (Tables 3–5), not including the acetogenic strains most capable of using a cathode or Fe(0) as electron donor, i.e., S. ovata, S. sphaeroides, and A. wieringae (Kato et al., 2015; Aryal et al., 2017; Marshall et al., 2017; Philips et al., 2019). These and other acetogenic strains could have a lower H2 threshold and/or a lower KH_2 than A. woodii (Figure 4). A. woodii maintained a H2 partial pressure on Fe(0) of 150 Pa, while all other strains tested in the same study maintained H2 partial pressures on Fe(0) below the detection limit (40 Pa) (Philips et al., 2019), indicating that the other strains have better H2 consumption characteristics to maintain low H2 partial pressures on the Fe(0) surface than A. woodii. Experimental studies are needed to determine the H2 consumption characteristics (H2 threshold and kinetic parameters) of more acetogenic strains and to investigate if those H2 consumption characteristics relate to the capacity of acetogens to use solid electron donors.
It should be noted that H2 consumption depends on more factors than just the H2 threshold and the H2 consumption kinetic parameters. The number of cells on the surface also affects the H2 consumption rate, thus attachment and biofilm formation properties are important. In addition, several components, such as dissolved Fe(II), and a pH deviating from the optimal pH could inhibit H2 consumption. Future studies assessing also these factors will be important to fundamentally understand the role of H2 consumption in increasing the H2 evolution reaction.
This work suggests that microbial H2 consumption favors cathodic H2 evolution. Interestingly, the increase of the anoxic corrosion reaction by microbial H2 scavenging is a well-known theory, often referred to as “cathodic depolarization”, initially proposed in 1934 (von Wolzogen Kühr and van der Vlugt, 1934). This theory was thought to be disproven by studies showing that only microorganisms isolated with Fe(0) as sole electron donor were capable of increasing anoxic corrosion, while strains isolated with H2 as electron donor were not (Dinh et al., 2004; Mori et al., 2010; Uchiyama et al., 2010; Enning and Garrelfs, 2014; Kato et al., 2015). Those studies, however, did not consider that hydrogenotrophic microorganisms can differ strongly in their H2 consumption characteristics, as explained in this work for acetogens. Moreover, it is very likely that enrichments with Fe(0) as electron donor select for strains with a low H2 threshold and high H2 affinity, while isolations with H2 (often high H2 partial pressure) select for strains with a high growth yield and growth rate, but a high H2 threshold and low H2 affinity.
Previous studies demonstrated that acetogens stimulate H2 evolution on a cathode and Fe(0) through the excretion of hydrogenases or other components catalyzing the H2 evolution reaction (Deutzmann et al., 2015; Philips et al., 2019; Tremblay et al., 2019). This work used the Butler–Volmer Equation (Equation 2) to demonstrate that the H2 evolution rate (current) on a cathode depends both on the exchange current density (kinetic effect, related to catalysis by enzymes) (Figure 3B) and the H2 partial pressure (thermodynamic effect) (Figure 3A). Consequently, there are two mechanisms by which hydrogenotrophic microorganisms could increase the H2 evolution rate on a cathode or Fe(0) (Figure 6): (1) catalysis of the H2 evolution reaction by extracellular hydrogenases or other components; and (2) the maintenance of low H2 partial pressures by H2 consumption. These two mechanisms are not mutually exclusive, but likely reinforce each other. The relative importance of each mechanism possibly depends on the reactivity of the material (higher for Fe(0) than for carbon-based cathodes), as well as on the H2 consumption characteristics and enzyme secretion mechanisms of the involved strains.
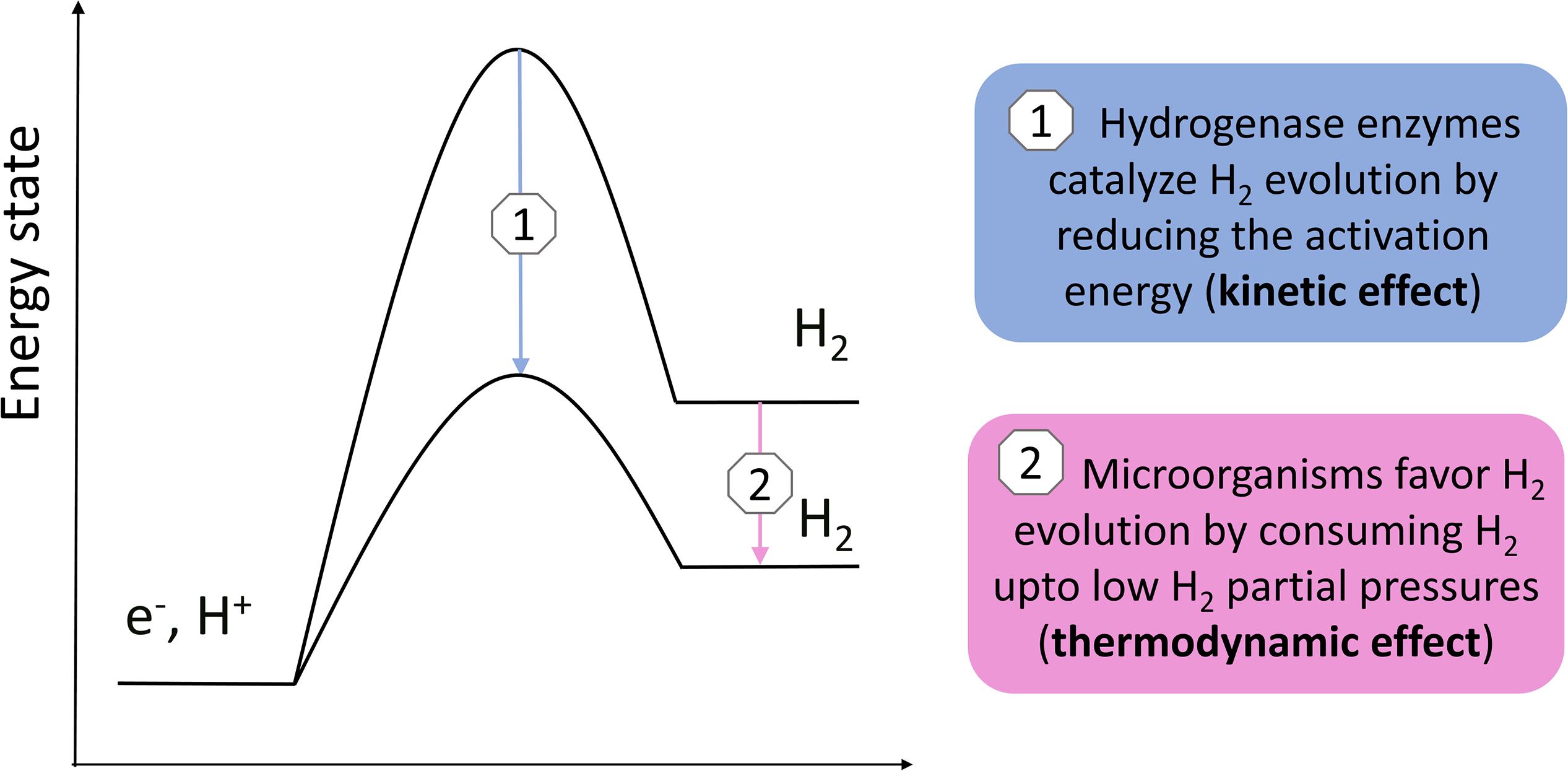
Figure 6. Simple scheme illustrating the two different mechanisms by which H2 consuming microorganisms possibly increase the H2 evolution reaction on a cathode or Fe(0).
In addition, more EET mechanism than presented in Figure 1 could exist, while strains could combine different EET mechanisms or adjust their EET mechanism depending on the conditions (for instance cathode potential). Moreover, the presence of cytochromes in the acetogenic Sporomusa and Moorella spp. definitely warrants further investigation of a possible direct EET mechanism.
The focus here was solely on acetogenic bacteria, but also other hydrogenotrophic microorganisms, e.g., methanogens and sulfate reducers, could favor the H2 evolution reaction on a cathode or Fe(0) by maintaining low H2 partial pressures. Methanogens differ in their H2 threshold and H2 affinity (Thauer et al., 2008) and a correlation between their H2 threshold and Fe(0) corrosion rate was already suggested (Palacios Jaramillo, 2019). In addition, some methanogens were found to excrete hydrogenase enzymes to catalyze the H2 evolution reaction (Deutzmann et al., 2015; Tsurumaru et al., 2018), while evidence exist that some methanogenic strains have a direct EET mechanism (Beese-Vasbender et al., 2015a; Rowe et al., 2019; Yee et al., 2019). Also the sulfate reducing IS4 strain likely has a direct EET mechanism (Beese-Vasbender et al., 2015b). Consequently, different strategies to obtain extracellular electrons from solid electron donors probably occur in the microbial world (Figure 1).
Acetogens and other hydrogenotrophic microorganisms capable of using a solid electron donors are of interest for biotechnological applications (e.g., microbial electrosynthesis), while they could also cause microbial induced corrosion and impact biogeochemical cycles. A good understanding of the role of microorganisms in those processes requires fundamental insights into their EET mechanism. I hypothesize here that the EET mechanism of acetogenic bacteria depends on their H2 consumption characteristics. Hence, assessment of the H2 consumption characteristics of various acetogenic strains could be valuable to select the optimal strain for microbial electrosynthesis applications. Genetic engineering cannot change the H2 consumption characteristics as easy as it changes the resulting end-products (Humphreys and Minton, 2018), so target strains should be chosen based on their H2 consumption characteristics. In addition, a good understanding of strain related differences in the EET mechanism will improve the assessment of microbial influenced corrosion based on microbial community compositions.
Conclusion
This work explained that the H2 partial pressure affects the H2 evolution reaction on a cathode or Fe(0) surface. This led to the assumption that the maintenance of low H2 partial pressures by hydrogenotrophic microorganisms is a mechanism to increase the H2 evolution reaction on a cathode or Fe(0), in addition to the catalysis by extracellular hydrogenases or other components (Figure 6). The H2 consumption characteristics of acetogenic bacteria were further discussed, which suggested that acetogens differ in their H2 threshold and H2 consumption kinetic parameters. Consequently, I hypothesize that the differences in the capacity of acetogens to use a solid electron donors, e.g., cathode and Fe(0), are related to the differences in their H2 consumption characteristics. The focus here was on acetogenic bacteria, but similar considerations are likely also relevant for other hydrogenotrophic microorganisms capable of using a cathode or Fe(0) as electron donor.
Data Availability Statement
The raw data supporting the conclusions of this article will be made available by the authors, without undue reservation, to any qualified researcher.
Author Contributions
The author confirms being the sole contributor of this work and has approved it for publication.
Funding
JP was supported by a Starting Grant of the Aarhus Universitets Forskningsfond.
Conflict of Interest
The authors declare that the research was conducted in the absence of any commercial or financial relationships that could be construed as a potential conflict of interest.
Acknowledgments
The initial idea for this work originates from the visit of Prof. Em. Rolf Thauer (Max Planck Institute for Terrestrial Microbiology, Marburg, Germany) to Ghent University (Belgium) in 2015. I also acknowledge Dr. Jan B.A. Arends and Prof. Korneel Rabaey (Ghent University) for their support in developing the ideas of this work. I thank Assis. Prof. Jacopo Catalano (Aarhus University) for his advice on the electrochemical aspects of this work. The reviewers and editor’s comments were very helpful to improve this work.
References
Arends, J. B. A. (2013). Optimizing the Plant Microbial Fuel Cell: Diversifying Applications and Product Outputs. Ph.D. thesis, Ghent University, Belgium.
Arends, J. B. A., Patil, S. A., Roume, H., and Rabaey, K. (2017). Continuous long-term electricity-driven bioproduction of carboxylates and isopropanol from CO2 with a mixed microbial community. J. CO2 Util. 20, 141–149. doi: 10.1016/j.jcou.2017.04.014
Aryal, N., Tremblay, P. L., Lizak, D. M., and Zhang, T. (2017). Performance of different Sporomusa species for the microbial electrosynthesis of acetate from carbon dioxide. Bioresour. Technol. 233, 184–190. doi: 10.1016/j.biortech.2017.02.128
Atkins, P., and De Paula, J. (2011). Physical Chemistry for the Life Sciences. Oxford: Oxford University Press.
Bajracharya, S., ter Heijne, A., Benetton, X. D., Vanbroekhoven, K., Buisman, C. J. N., Strik, D., et al. (2015). Carbon dioxide reduction by mixed and pure cultures in microbial electrosynthesis using an assembly of graphite felt and stainless steel as a cathode. Bioresour. Technol. 195, 14–24. doi: 10.1016/j.biortech.2015.05.081
Bard, A. J., and Faulkner, L. R. (2001). Electrochemical Methods: Fundamentals and Applications. Hoboken, NJ: John Wiley & Sons.
Basen, M., and Müller, V. (2017). “Hot” acetogenesis. Extremophiles 21, 15–26. doi: 10.1007/s00792-016-0873-3
Beese-Vasbender, P. F., Grote, J. P., Garrelfs, J., Stratmann, M., and Mayrhofer, K. J. J. (2015a). Selective microbial electrosynthesis of methane by a pure culture of a marine lithoautotrophic archaeon. Bioelectrochemistry 102, 50–55. doi: 10.1016/j.bioelechem.2014.11.004
Beese-Vasbender, P. F., Nayak, S., Erbe, A., Stratmann, M., and Mayrhofer, K. J. J. (2015b). Electrochemical characterization of direct electron uptake in electrical microbially influenced corrosion of iron by the lithoautotrophic SRB Desulfopila corrodens strain IS4. Electrochim. Acta 167, 321–329. doi: 10.1016/j.electacta.2015.03.184
Bengelsdorf, F. R., Beck, M. H., Erz, C., Hoffmeister, S., Karl, M. M., Riegler, P., et al. (2018). “Bacterial anaerobic synthesis gas (syngas) and CO2+H2 fermentation,” in Advances in Applied Microbiology, Vol. 103, eds S. Sariaslani and G. M. Gadd (San Diego, CA: Elsevier Academic Press Inc), 143–221. doi: 10.1016/bs.aambs.2018.01.002
Bertsch, J., and Müller, V. (2015). Bioenergetic constraints for conversion of syngas to biofuels in acetogenic bacteria. Biotechnol. Biofuels 8:210. doi: 10.1186/s13068-015-0393-x
Breznak, J. A., Switzer, J. M., and Seitz, H. J. (1988). Sporomusa termitida sp. nov., an H2/CO2-utilizing acetogen isolated from termites. Arch. Microbiol. 150, 282–288. doi: 10.1007/bf00407793
Bryant, R. D., and Laishley, E. J. (1990). The role of hydrogenase in anaerobic biocorrosion. Can. J. Microbiol. 36, 259–264. doi: 10.1139/m90-045
Conrad, R., and Wetter, B. (1990). Influence of temperature on energetics of hydrogen metabolism in homoacetogenic, methanogenic and other anaerobic bacteria. Arch. Microbiol. 155, 94–98. doi: 10.1007/bf00291281
Cord-Ruwisch, R., Seitz, H.-J., and Conrad, R. (1988). The capacity of hydrogenotrophic anaerobic bacteria to compete for traces of hydrogen depends on the redox potential of the terminal electron acceptor. Arch. Microbiol. 149, 350–357. doi: 10.1007/bf00411655
Da Silva, S., Basseguy, R., and Bergel, A. (2004). Electron transfer between hydrogenase and 316L stainless steel: identification of a hydrogenase-catalyzed cathodic reaction in anaerobic mic. J. Electroanal. Chem. 561, 93–102. doi: 10.1016/j.jelechem.2003.07.005
Deutzmann, J. S., Merve, S., and Spormann, A. M. (2015). Extracellular enzymes facilitate electron uptake in biocorrosion and bioelectrosynthesis. mBio 6:e00496-15. doi: 10.1128/mBio.00496-15
Deutzmann, J. S., and Spormann, A. M. (2017). Enhanced microbial electrosynthesis by using defined co-cultures. ISME J. 11, 704–714. doi: 10.1038/ismej.2016.149
Dinh, H. T., Kuever, J., Mussmann, M., Hassel, A. W., Stratmann, M., and Widdel, F. (2004). Iron corrosion by novel anaerobic microorganisms. Nature 427, 829–832. doi: 10.1038/nature02321
Drake, H. L., Gossner, A. S., and Daniel, S. L. (2008). “Old acetogens, new light,” in Incredible Anaerobes: from Physiology to Genomics to Fuels, Vol. 1125, eds J. Wiegel, R. J. Maier, and M. W. W. Adams (Oxford: Blackwell Publishing), 100–128. doi: 10.1196/annals.1419.016
Enning, D., and Garrelfs, J. (2014). Corrosion of iron by sulfate-reducing bacteria: new views of an old problem. Appl. Environ. Microbiol. 80, 1226–1236. doi: 10.1128/AEM.02848-13
Faraghiparapari, N., and Zengler, K. (2017). Production of organics from CO2 by microbial electrosynthesis (MES) at high temperature. J. Chem. Technol. Biotechnol. 92, 375–381. doi: 10.1002/jctb.5015
Graber, J. R., and Breznak, J. A. (2004). Physiology and nutrition of Treponema primitia, an H2/CO2-acetogenic spirochete from termite hindguts. Appl. Environ. Microbiol. 70, 1307–1314. doi: 10.1128/aem.70.3.1307-1314.2004
Humphreys, C. M., and Minton, N. P. (2018). Advances in metabolic engineering in the microbial production of fuels and chemicals from C1 gas. Curr. Opin. Biotechnol. 50, 174–181. doi: 10.1016/j.copbio.2017.12.023
Jeremiasse, A. W. (2011). Cathode Innovations for Enhanced H2 Production through Microbial Electrolysis. Ph.D. thesis, Wageningen University, Wageningen.
Jourdin, L., Yang, L., Flexer, V., Keller, J., and Freguia, S. (2016). Biologically induced hydrogen production drives high rate/high efficiency microbial electrosynthesis of acetate from carbon dioxide. Chemelectrochem 3, 581–591. doi: 10.1002/celc.201500530
Kato, S., Yumoto, I., and Kamagata, Y. (2015). Isolation of acetogenic bacteria that induce biocorrosion by utilizing metallic iron as the sole electron donor. Appl. Environ. Microbiol. 81, 67–73. doi: 10.1128/AEM.02767-14
Kotsyurbenko, O. R., Glagolev, M. V., Nozhevnikova, A. N., and Conrad, R. (2001). Competition between homoacetogenic bacteria and methanogenic Archaea for hydrogen at low temperature. FEMS Microbiol. Ecol. 38, 153–159. doi: 10.1016/s0168-6496(01)00179-9
Kracke, F., Wong, A. B., Maegaard, K., Deutzmann, J. S., Hubert, M. A., Hahn, C., et al. (2019). Robust and biocompatible catalysts for efficient hydrogen-driven microbial electrosynthesis. Commun. Chem. 2:45.
Krumholz, L. R., Harris, S. H., Tay, S. T., and Suflita, J. M. (1999). Characterization of two subsurface H2-utilizing bacteria, Desulfomicrobium hypogeium sp nov and Acetobacterium psammolithicum sp nov., and their ecological roles. Appl. Environ. Microbiol. 65, 2300–2306.
LaBelle, E. V., Marshall, C. W., Gilbert, J. A., and May, H. D. (2014). Influence of acidic pH on hydrogen and acetate production by an electrosynthetic microbiome. PLoS One 9:e109935. doi: 10.1371/journal.pone.0109935
Le Van, T. D., Robinson, J. A., Ralph, J., Greening, R. C., Smolenski, W. J., Leedle, J. A. Z., et al. (1998). Assessment of reductive acetogenesis with indigenous ruminal bacterium populations and Acetitomaculum ruminis. Appl. Environ. Microbiol. 64, 3429–3436.
Leclerc, M., Bernalier, A., Donadille, G., and Lelait, M. (1997). H2/CO2 metabolism in acetogenic bacteria isolated from the human colon. Anaerobe 3, 307–315. doi: 10.1006/anae.1997.0117
Lienemann, M., Deutzmann, J. S., Sahin, M., and Spormann, A. M. (2018). Mediator-free enzymatic electrosynthesis of formate by the Methanococcus maripaludis heterodisulfide reductase supercomplex. Bioresour. Technol. 254, 278–283. doi: 10.1016/j.biortech.2018.01.036
Liew, F., Martin, M. E., Tappel, R. C., Heijstra, B. D., Mihalcea, C., and Kopke, M. (2016). Gas fermentation - A flexible platform for commercial scale production of low-carbon-fuels and chemicals from waste and Renewable feedstocks. Front. Microbiol. 7:694. doi: 10.3389/fmicb.2016.00694
Lovley, D. R., and Nevin, K. P. (2013). Electrobiocommodities: powering microbial production of fuels and commodity chemicals from carbon dioxide with electricity. Curr. Opin. Biotechnol. 24, 385–390. doi: 10.1016/j.copbio.2013.02.012
Mand, J., Park, H. S., Jack, T. R., and Voordouw, G. (2014). The role of acetogens in microbially influenced corrosion of steel. Front. Microbiol. 5:268. doi: 10.3389/fmicb.2014.00268
Marshall, C. W., Ross, D. E., Fichot, E. B., Norman, R. S., and May, H. D. (2012). Electrosynthesis of commodity chemicals by an autotrophic microbial community. Appl. Environ. Microbiol. 78, 8412–8420. doi: 10.1128/AEM.02401-12
Marshall, C. W., Ross, D. E., Fichot, E. B., Norman, R. S., and May, H. D. (2013). Long-term operation of microbial electrosynthesis systems improves acetate production by autotrophic microbiomes. Environ. Sci. Technol. 47, 6023–6029. doi: 10.1021/es400341b
Marshall, C. W., Ross, D. E., Handley, K. M., Weisenhorn, P. B., Edirisinghe, J. N., Henry, C. S., et al. (2017). Metabolic reconstruction and modeling microbial electrosynthesis. Sci. Rep. 7:8391. doi: 10.1038/s41598-017-08877-z
May, H. D., Evans, P. J., and LaBelle, E. V. (2016). The bioelectrosynthesis of acetate. Curr. Opin. Biotechnol. 42, 225–233. doi: 10.1016/j.copbio.2016.09.004
Mock, J., Zheng, Y. N., Mueller, A. P., Ly, S., Tran, L., Segovia, S., et al. (2015). Energy conservation associated with ethanol formation from H2 and CO2 in Clostridium autoethanogenum involving electron bifurcation. J. Bacteriol. 197, 2965–2980. doi: 10.1128/JB.00399-15
Mohammadi, M., Mohamed, A. R., Najafpour, G. D., Younesi, H., and Uzir, M. H. (2014). Kinetic studies on fermentative production of biofuel from synthesis gas using Clostridium ljungdahlii. Sci. World J. 2014:910590. doi: 10.1155/2014/910590
Moller, B., Ossmer, R., Howard, B. H., Gottschalk, G., and Hippe, H. (1984). Sporomusa, a new genus of gram-negative anaerobic bacteria including Sporomusa sphaeroides spec-nov and Sporomusa ovata spec-nov. Arch. Microbiol. 139, 388–396. doi: 10.1007/bf00408385
Mori, K., Tsurumaru, H., and Harayama, S. (2010). Iron corrosion activity of anaerobic hydrogen-consuming microorganisms isolated from oil facilities. J. Biosci. Bioeng. 110, 426–430. doi: 10.1016/j.jbiosc.2010.04.012
Nevin, K. P., Hensley, S. A., Franks, A. E., Summers, Z. M., Ou, J., Woodard, T. L., et al. (2011). Electrosynthesis of organic compounds from carbon dioxide is catalyzed by a diversity of acetogenic microorganisms. Appl. Environ. Microbiol. 77, 2882–2886. doi: 10.1128/AEM.02642-10
Nevin, K. P., Woodard, T. L., Franks, A. E., Summers, Z. M., and Lovley, D. R. (2010). Microbial electrosynthesis: feeding microbes electricity to convert carbon dioxide and water to multicarbon extracellular organic compounds. mBio 1:e00103-10. doi: 10.1128/mBio.00103-10
Palacios Jaramillo, P. A. (2019). Microbial Induced Corrosion by Methanogens and other Associated Microbial Groups. Ph.D. thesis, University of Southern Denmark, Odense.
Patil, S. A., Arends, J. B. A., Vanwonterghem, I., van Meerbergen, J., Guo, K., Tyson, G. W., et al. (2015). Selective enrichment establishes a stable performing community for microbial electrosynthesis of acetate from CO2. Environ. Sci. Technol. 49, 8833–8843. doi: 10.1021/es506149d
Peters, V., Janssen, P. H., and Conrad, R. (1998). Efficiency of hydrogen utilization during unitrophic and mixotrophic growth of Acetobacterium woodii on hydrogen and lactate in the chemostat. FEMS Microbiol. Ecol. 26, 317–324. doi: 10.1016/s0168-6496(98)00047-6
Philips, J., Monballyu, E., Georg, S., De Paepe, K., Prévoteau, A., Rabaey, K., et al. (2019). An Acetobacterium strain isolated with metallic iron as electron donor enhances iron corrosion by a similar mechanism as Sporomusa sphaeroides. FEMS Microbiol. Ecol. 95:fiy222. doi: 10.1093/femsec/fiy222
Philips, J., Verbeeck, K., Rabaey, K., and Arends, J. B. A. (2016). “Electron transfer mechanisms in biofilms,” in Microbial Electrochemical and Fuel Cells: Fundamentals and Applications, eds K. Scott and E. Yu (Amsterdam: Elsevier), 67–113. doi: 10.1016/b978-1-78242-375-1.00003-4
Poehlein, A., Bengelsdorf, F. R., Schiel-Bengelsdorf, B., Daniel, R., and Dürre, P. (2016). Genome sequence of the acetogenic bacterium Acetobacterium wieringae DSM 1911(T). Genome Announc. 4:e01430-16. doi: 10.1128/genomeA.01430-16
Poehlein, A., Schmidt, S., Kaster, A. K., Goenrich, M., Vollmers, J., Thürme, A., et al. (2012). An ancient pathway combining carbon dioxide fixation with the generation and utilization of a sodium ion gradient for ATP synthesis. PLoS One 7:e33439. doi: 10.1371/journal.pone.0033439
Rabaey, K., and Rozendal, R. A. (2010). Microbial electrosynthesis - revisiting the electrical route for microbial production. Nat. Rev. Microbiol. 8, 706–716. doi: 10.1038/nrmicro2422
Rheinlander, P. J., Herranz, J., Durst, J., and Gasteiger, H. A. (2014). Kinetics of the hydrogen oxidation/evolution reaction on polycrystalline platinum in alkaline electrolyte reaction order with respect to hydrogen pressure. J. Electrochem. Soc. 161, F1448–F1457.
Rouvre, I., and Basseguy, R. (2016). Exacerbation of the mild steel corrosion process by direct electron transfer between Fe-Fe -hydrogenase and material surface. Corros. Sci. 111, 199–211. doi: 10.1016/j.corsci.2016.05.005
Rowe, A. R., Xu, S., Gardel, E., Bose, A., Girguis, P., Amend, J. P., et al. (2019). Methane-linked mechanisms of electron uptake from cathodes by Methanosarcina barkeri. mBio 10:e02448-18. doi: 10.1128/mBio.02448-18
Saheb-Alam, S., Singh, A., Hermansson, M., Persson, F., Schnurer, A., Wilen, B. M., et al. (2018). Effect of start-up strategies and electrode materials on carbon dioxide reduction on biocathodes. Appl. Environ. Microbiol. 84:e02242-17. doi: 10.1128/AEM.02242-17
Schuchmann, K., and Müller, V. (2014). Autotrophy at the thermodynamic limit of life: a model for energy conservation in acetogenic bacteria. Nat. Rev. Microbiol. 12, 809–821. doi: 10.1038/nrmicro3365
Seitz, H. J., Schink, B., Pfennig, N., and Conrad, R. (1990). Energetics of syntrophic ethanol oxidation in defined chemostat cocultures: 1. Energy requirement of H2 production and H2 oxidation. Arch. Microbiol. 155, 82–88. doi: 10.1007/bf00291279
Song, J., Kim, Y., Lim, M., Lee, H., Lee, J. I., and Shin, W. (2011). Microbes as electrochemical CO2 conversion catalysts. Chemsuschem 4, 587–590. doi: 10.1002/cssc.201100107
Spahn, S., Brandt, K., and Müller, V. (2015). A low phosphorylation potential in the acetogen Acetobacterium woodii reflects its lifestyle at the thermodynamic edge of life. Arch. Microbiol. 197, 745–751. doi: 10.1007/s00203-015-1107-2
Su, M., Jiang, Y., and Li, D. (2013). Production of acetate from carbon dioxide in bioelectrochemical systems based on autotrophic mixed culture. J. Microbiol. Biotechnol. 23, 1140–1146. doi: 10.4014/jmb.1304.04039
Thauer, R. K., Jungermann, K., and Decker, K. (1977). Energy conservation in chemotrophic anaerobic bacteria. Bacteriol. Rev. 41, 100–180.
Thauer, R. K., Kaster, A. K., Seedorf, H., Buckel, W., and Hedderich, R. (2008). Methanogenic archaea: ecologically relevant differences in energy conservation. Nat. Rev. Microbiol. 6, 579–591. doi: 10.1038/nrmicro1931
Tian, S. H., Wang, H. Q., Dong, Z. W., Yang, Y., Yuan, H., Huang, Q., et al. (2019). Mo2C-induced hydrogen production enhances microbial electrosynthesis of acetate from CO2 reduction. Biotechnol. Biofuels 12:71. doi: 10.1186/s13068-019-1413-z
Tremblay, P. L., Faraghiparapari, N., and Zhang, T. (2019). Accelerated H2 evolution during microbial electrosynthesis with Sporomusa ovata. Catalysts 9:166. doi: 10.3390/catal9020166
Tsurumaru, H., Ito, N., Mori, K., Wakai, S., Uchiyama, T., Iino, T., et al. (2018). An extracellular NiFe hydrogenase mediating iron corrosion is encoded in a genetically unstable genomic island in Methanococcus maripaludis. Sci. Rep. 8:15149. doi: 10.1038/s41598-018-33541-5
Uchiyama, T., Ito, K., Mori, K., Tsurumaru, H., and Harayama, S. (2010). Iron-corroding methanogen isolated from a crude-oil storage tank. Appl. Environ. Microbiol. 76, 1783–1788. doi: 10.1128/AEM.00668-09
Valdes, J., Pedroso, I., Quatrini, R., Dodson, R. J., Tettelin, H., Blake, R., et al. (2008). Acidithiobacillus ferrooxidans metabolism: from genome sequence to industrial applications. BMC Genomics 9:597. doi: 10.1186/1471-2164-9-597
Vincent, K. A., Parkin, A., and Armstrong, F. A. (2007). Investigating and exploiting the electrocatalytic properties of hydrogenases. Chem. Rev. 107, 4366–4413. doi: 10.1021/cr050191u
von Wolzogen Kühr, C. A. H., and van der Vlugt, L. S. (1934). The graphitization of cast iron as an electrobiochemical process in anaerobic soil. Water 18, 147–165.
Xafenias, N., and Mapelli, V. (2014). Performance and bacterial enrichment of bioelectrochemical systems during methane and acetate production. Int. J. Hydrogen Energy 39, 21864–21875. doi: 10.1016/j.ijhydene.2014.05.038
Yee, M. O., Snoeyenbos-West, O. L., Thamdrup, B., Ottosen, L. D. M., and Rotaru, A. E. (2019). Extracellular electron uptake by two Methanosarcina species. Front. Energy Res. 7:29. doi: 10.3389/fenrg.2019.00029
Keywords: acetogenesis, extracellular electron transfer mechanisms, energy conservation, ATP gain, Butler-Volmer equation, zero valent iron, biocathode, HER reaction
Citation: Philips J (2020) Extracellular Electron Uptake by Acetogenic Bacteria: Does H2 Consumption Favor the H2 Evolution Reaction on a Cathode or Metallic Iron? Front. Microbiol. 10:2997. doi: 10.3389/fmicb.2019.02997
Received: 05 July 2019; Accepted: 11 December 2019;
Published: 10 January 2020.
Edited by:
Mirko Basen, University of Rostock, GermanyReviewed by:
Rashmi Chandra, Monterrey Institute of Technology and Higher Education (ITESM), MexicoAmelia-Elena Rotaru, University of Southern Denmark, Denmark
Copyright © 2020 Philips. This is an open-access article distributed under the terms of the Creative Commons Attribution License (CC BY). The use, distribution or reproduction in other forums is permitted, provided the original author(s) and the copyright owner(s) are credited and that the original publication in this journal is cited, in accordance with accepted academic practice. No use, distribution or reproduction is permitted which does not comply with these terms.
*Correspondence: Jo Philips, am8ucGhpbGlwc0BlbmcuYXUuZGs=