- 1Department of Microbiology, College of Resources, Sichuan Agricultural University, Chengdu, China
- 2Soil and Fertilizer Institute, Sichuan Academy of Agricultural Sciences, Chengdu, China
In the artificial cultivation of truffles, ectomycorrhizal colonization level, host plant quality, and the associated microbes in the rhizosphere soil are vitally important. To explore the effects of nitric oxide (NO) and phosphorus (P) stress on the early symbiosis of truffles and host plants, different concentrations of exogenous NO donor sodium nitroprusside (SNP) and P were applied to Carya illinoinensis seedlings inoculated with the Chinese black truffle (Tuber indicum). The growth of T. indicum-mycorrhized seedlings and their mycorrhizal colonization rate were investigated. Additionally, the denitrifying bacterial community harboring NO reductase (norB) genes and the fungal community in the rhizosphere of the host were analyzed by high-throughput sequencing. The results showed that the colonization rate of T. indicum was significantly influenced by SNP treatments and P stress, with the highest level being obtained when the SNP was 100 μmol/L under low P stress (5 μmol/L). Treatment with 100 μmol/L SNP alone also increased the colonization rate of T. indicum and had positive effects on the plant height, stem circumference, biomass, root-shoot ratio and root POD activity of the seedlings at different times after inoculation. Under low P stress, the 100 μmol/L SNP increased the richness of the norB-type denitrifying bacterial community. Interestingly, the diversity and richness of norB-type denitrifying bacteria were significantly positively correlated with the colonization rate of T. indicum. SNP treatments under low P stress altered the abundance of some dominant taxa such as Alphaproteobacteria, Gammaproteobacteria, Pseudomonas, Ensifer, and Sulfitobacter. Evaluation of the fungal community in the rhizosphere revealed that 100 μmol/L SNP treatment alone had no noticeable effect on their richness and diversity, but it did shape the abundance of some fungi. Buellia, Podospora, Phaeoisaria, Ascotaiwania, and Lophiostoma were more abundant following exogenous NO application, while the abundance of Acremonium, Monographella, and Penicillium were decreased. Network analysis indicated that T. indicum was positively and negatively correlated with some fungal genera when treated with 100 μmol/L SNP. Overall, these results revealed how exogenous NO and P stress influence the symbiosis of truffles and host plants, and indicate that application of SNP treatments has the potential for ectomycorrhizal synthesis and truffle cultivation.
Introduction
Tuber spp., commonly known as truffles, are ascomycete fungi that form ectomycorrhizae in a symbiotic relationship with plant roots and are prized for their hypogeous, edible fruiting bodies which adds a unique flavor to dishes (Kues and Martin, 2011; Vahdatzadeh et al., 2015). The interest in artificial cultivation of truffles has increased because of both the scarcity of truffle resources and reports of them being a source of polysaccharides with antitumor activity (Luo et al., 2011; Pereira et al., 2013; Zhao et al., 2014; Schmidberger and Schieberle, 2017). At present, synthesis of truffle-colonized seedlings and establishment of truffle plantations are the main methods of truffle cultivation (Deng et al., 2014). Tuber indicum, which is morphologically and phylogenetically similar to T. melanosporum, is the major commercial species of black truffle in China (Geng et al., 2009; Liu et al., 2011). Carya illinoinensis is an economically important nut tree native to North America, which is now cultivated worldwide (Benucci et al., 2012; Marozzi et al., 2017). Accordingly, utilizing C. illinoinensis as the host plant for T. indicum would have many practical and economic benefits and it has been shown that the ectomycorrhizae of T. indicum have now been successfully cultivated with C. illinoinensis (Bonito et al., 2011). Although the mycorrhization of these two organisms has been successfully accomplished, determining how to increase the ectomycorrhizal colonization levels and physiological and molecular mechanisms in response to this symbiont formation requires further exploration.
Ectomycorrhizae play an important role in P cycling of the rhizosphere (Cumming et al., 2015; Liu et al., 2018). Many studies have shown that ectomycorrhizae can contribute to the P absorption of plant roots, especially when P is scarce in the rhizosphere of the soil (Núñez et al., 2008; Bortier et al., 2018; Köhler et al., 2018). The content of P in the environment has been verified to impact the growth of ectomycorrhizal fungi and their colonization (Jones et al., 1990; Xue et al., 2008; Kluber et al., 2012). NO can affect the growth and development of plants as a signaling molecule that participates in many physiological processes, including seed germination, leaf growth, lateral root growth, stomatal movement and response to various biotic and abiotic stresses (Baudouin, 2011; Corpas and Barroso, 2015). Some reports have shown that NO can be produced in much higher levels in plants roots after colonization by arbuscular mycorrhizal fungi (Calcagno et al., 2012; Corpas and Barroso, 2015), which indicates that NO is important during the process of mycorrhizal colonization. In addition, evidence of the involvement of NO in plant responses to low P stress has been obtained (Simontacchi et al., 2015). As an exogenous NO donor, sodium nitroprusside (SNP) has commonly been used to explore the effects of NO on the physiology of many plants. However, it is still not known if SNP can affect the colonization levels of ectomycorrhizal fungi such as truffles on host plant root systems. Moreover, the effects of exogenous NO on the ectomycorrhizal synthesis of T. indicum and on the symbiotic system of C. illinoinensis with T. indicum under P stress conditions have not yet been reported.
In terrestrial ecosystems, ectomycorrhizal fungi including truffles have an important ecological function. A variety of microbial communities are involved in the lifecycle of truffles, and these play important roles in the truffle ectomycorrhizae and ascocarp formation, while also contributing to their aroma (Splivallo et al., 2015; Vahdatzadeh et al., 2015). Moreover, truffles have been predicted to influence soil microbial communities because of the formation of a brûlé (an area devoid of herbaceous cover) (Streiblova et al., 2012; Mello et al., 2013; Li et al., 2018). Our previous studies have also indicated that T. indicum shapes the bacterial and fungal communities in the ectomycorrhizosphere of P. armandii and Q. aliena (Li et al., 2017, 2018). Denitrification is a key nitrogen removal process that can produce NO, N2O, and N2, and different bacteria including bacteria harboring NO reductase (norB) genes can perform this process (Yunfu et al., 2017). Considering that NO may play an important role in the mycorrhizal colonization process of truffles and exogenous NO treatment was provided in this study, bacteria harboring norB-genes were selected for analysis, rather than 16S rRNA genes. Although many previous studies have investigated the microbial communities associated with truffles (Antony-Babu et al., 2014; Benucci and Bonito, 2016; Deveau et al., 2016; Fu et al., 2016), the specific roles of these microbial communities and the interaction between these microbes and truffle ectomycorrhizae is unclear, as are the conditions that occur under exogenous NO and P stress.
In this study, different concentrations of exogenous NO and P were provided to the C. illinoinensis seedlings inoculated and uninoculated with T. indicum. The colonization rate and the host plant growth and physiology were assessed. Additionally, high-throughput sequencing was used to analyze the norB-type denitrifying bacterial community of the rhizosphere soil. Next, to further explore the effects of NO alone on this symbiotic system, a suitable concentration of NO was selected for application to the colonized seedlings. The colonization rate, seedling growth and fungal communities of the rhizosphere soil were subsequently investigated from month 1 to 6 after inoculation. To our knowledge, this is the first study to explore the effects of exogenous NO and P stress on the ectomycorrhizal colonization of truffles and on the symbiotic system of host plants with truffles, with the goal of learning more about the physiological and molecular mechanism response to this symbiont formation under different conditions to improve the artificial cultivation of truffles.
Materials and Methods
C. illinoinensis Seedling Cultivation and T. indicum Inoculation
Carya illinoinensis seeds obtained from Yangbi County, China were first sterilized by soaking in 0.1% potassium permanganate solution for 2 h. Next, washed seeds were sown in sterilized nursery substrate composed of vermiculite, perlite, and water (volume ratio of 1:1:1) (Li et al., 2017). After 3 months, seedlings that were growing well were selected for transplantation into separate plastic containers filled with 1 L of sterilized cultivation substrate. There were two kinds of cultivation substrate prepared, Substrate I and Substrate II. Substrate I was composed of nutrient-poor sand, and Substrate II consisted of organic soil, vermiculite and water (volume ratio 1:1:0.5). The two cultivation substrates were autoclaved for 90 min at 121°C before use (Li et al., 2017, 2018). Truffle inoculation was performed when the seedlings were transplanted.
Tuber indicum was from Yanbian County, China. The truffle inoculum was prepared as previously described (Li et al., 2018). Briefly, 75% alcohol was used to disinfect the surface of the truffle ascocarps, after which they were pulverized and blended to spore powder. Next, 2 and 1 g of spore powder was inoculated into Substrate I and Substrate II, respectively, surrounding the roots of each C. illinoinensis seedling. There were 36 and 42 inoculated C. illinoinensis seedlings cultivated in Substrate I and Substrate II, respectively. Additionally, 3 uninoculated seedlings were cultivated in Substrate I. All seedlings were cultivated in a greenhouse under the same conditions with a clean environment and appropriate temperature and moisture content of the substrate.
Experimental Design
Exogenous NO Treatment Combined With P Stress
Seedlings cultivated in Substrate I were subjected to exogenous NO treatment combined with P stress (Supplementary Table S1a).
After T. indicum inoculation, the exogenous NO donor SNP with four different concentrations (0, 10, 100, and 1000 μmol/L) was applied to the inoculated C. illinoinensis seedlings every 15 days, while uninoculated seedlings were treated with 0 μmol/L SNP. Samples were treated with 100 mL SNP per pot at each treatment time, half of which was applied to the cultivation substrate, while the remainder was sprayed on the leaf surface. The inoculated seedlings treated with 0, 10, 100, and 1000 μmol/L SNP were denoted S0, S1, S2, and S3, respectively.
When the seedlings were treated with different concentrations of SNP, they were also treated with different levels of P. Briefly, modified Hoagland nutrient solution containing three different concentrations of P was prepared (0, 5, and 2000 μmol/L). The P originated from KH2PO4 and the final concentration of each element in the nutrient solution except P is shown in Supplementary Table S2. The inoculated C. illinoinensis seedlings that were irrigated with 0, 5, and 2000 μmol/L P nutrient solution were denoted as the no P treatment (P0), low P treatment (P5), and high P treatment group (P2000), respectively. Uninoculated seedlings treated with 0 μmol/L SNP were only irrigated with 0 μmol/L P nutrient solution and were assigned to CK group. Overall, there are 12 treatments (excluding CK): P0S0, P0S1, P0S2, P0S3, P5S0, P5S1, P5S2, P5S3, P2000S0, P2000S1, P2000S2, and P2000S3. Each treatment contained at least three C. illinoinensis seedlings, all of which were timely irrigated with corresponding modified Hoagland nutrient solution, while sterile water was periodically applied to keep the cultivation substrate moist.
Exogenous NO Treatment Only
Exogenous treatment of only NO was applied to seedlings that were cultivated in Substrate II (Supplementary Table S1b). To further investigate the effects of only NO on the growth of C. illinoinensis seedlings with T. indicum colonization in the early symbiotic stage, the appropriate concentration (100 μmol/L) of SNP was applied alone as described above. Overall, half of the inoculated seedlings cultivated in Substrate II were treated with 100 mL of 100 μmol/L SNP every 15 days after inoculation of T. indicum until day 90 (SNP treatment), while the remaining inoculated seedlings were treated with an equal amount of water at the same time (Control-M treatment). The seedlings in these two treatments were irrigated with water every 2–3 days to keep the cultivation substrate moist.
Sampling Strategy and Analysis
After 4 months from inoculation, seedlings cultivated in Substrate I were observed, and samples were collected. The ectomycorrhizae of C. illinoinensis seedlings colonized by T. indicum were successfully detected by morphological analysis using a microscope. In each treatment, seedlings and their root system were harvested. Moreover, the rhizospheres soil of seedlings in CK and low P treatments (P5S0, P5S1, P5S2, and P5S3) were also collected aseptically. The mycorrhizal colonization rate was determined by counting the number of root segments colonized by T. indicum under a stereomicroscope based on the mycorrhizal fungal structures, with 30 root segments randomly selected in total for each seedling, which was finally expressed as: (root segments colonized by T. indicum/total observed root segments) × 100% (Andres-Alpuente et al., 2014). The plant morphology and physiology was determined immediately after the collection of seedlings and their roots. The rhizosphere soil samples were stored at −80°C prior to high-throughput sequencing of the norB-type denitrifying bacterial community.
For the seedlings of two treatments cultivated in Substrate II, their root systems and rhizosphere soil were collected every month after inoculation and used to determine the plant morphology and physiology, as well as for high-throughput sequencing of the fungal communities. Samples harvested at 0, 1, 2, 3, 4, 5, and 6 months after inoculation were denoted M0, M1, M2, M3, M4, M5, and M6, respectively. The ectomycorrhizae in samples from each month were detected and the colonization rate of T. indicum was calculated at month 6.
The whole experimental design and sampling strategy in this study can be seen in Supplementary Table S1. Three biological samples in each treatment were used for analysis, including the analysis of plant physiology, colonization rate and microbial communities.
Determination of Plant Morphology and Physiology
The measured plant morphological and physiological indices included the plant height, stem circumference, root-shoot ratio, biomass, root activity, and superoxide dismutase (SOD) activity in roots and peroxidase (POD) activity in roots.
The plant height and stem circumference of C. illinoinensis seedlings were measured using a ruler and vernier caliper. Next, the seedlings were put in 100°C water for 20 min to halt respiration, then they were oven-dried at 75°C until constant weight for determination of the dry-weight, which was taken as the biomass. Next, the seedlings were divided into their underground and aboveground parts and the dry weights of the two parts were determined. The root-shoot ratio was expressed as the ratio of the dry weight of underground to aboveground parts of the seedlings (Maunoury-Danger et al., 2010).
The root activity was determined by the triphenyl tetrazolium chloride method as previously described (Zhang et al., 2012). The root SOD activity was determined by their ability to inhibit the photochemical reduction of nitro-blue tetrazolium (NBT) under light, and a unit of enzyme activity (U) was expressed as 50% inhibition of the NBT photoreduction (Fridovich, 2011). The determination of root POD activity was based on the theory that H2O2 can oxidize guaiacol under the catalysis of POD and form a tawny substance that can be detected using a spectrophotometer (Meloni et al., 2003). A unit of POD activity (U) was expressed as an absorbance change of 0.01 per minute at 470 nm.
Soil DNA Extraction and PCR Amplification of norB Genes and ITS Genes
Total genomic DNA of the rhizosphere soil samples was extracted using a Power Soil® DNA Isolation Kit (MoBio, Carlsbad, CA, United States) according to the manufacturer’s instructions. The extracted DNA was detected by 0.8% agarose gel electrophoresis and quantified by ultraviolet spectrophotometry.
For the DNA extracted from the soil samples of the P5S0, P5S1, P5S2, P5S3, and CK treatments (each treatment was performed in triplicate), the norB gene was amplified with the universal primers cnorB2F (5′-GACAAGNNNTACTGGTGGT-3′) and cnorB6R (5′-GAANCCCCANACNCCN GC-3′). The PCR reaction mix was 25 μL, which included the DNA template (2 μL), reaction buffer (5 μL), GC buffer (5 μL), 2 μL dNTPs (2.5 mmol L–1), 1 μL forward primer (10 μmol L–1), 1 μL reverse primer (10 μmol L–1), 0.25 μL Q5 DNA polymerase, and 8.75 μL ddH2O. The cycling conditions were as follows: initial denaturation at 98°C for 2 min, followed by denaturation at 98°C for 15 s, and annealing at 55°C for 30 s, extension at 72°C for 30 s, after which samples were subjected to final extension at 72°C for 5 min. For the DNA extracted from the soil samples in Substrate II, the ITS1 region was amplified using primers ITS1 (5′-GGAAGTAAAAGTCGTA ACAAGG-3′) and ITS2 (5′-GCTGCGTTCTTCATCGATGC-3′). The PCR products were checked by 2% agarose gel electrophoresis and the target fragments were recovered using an Axygen Axy Prep DNA Gel Extraction kit (AxyGen Biosystems, United States). The recovered PCR products obtained from three technical replicates were combined in equidense ratios for each sample and purified with a Qiagen Gel Extraction Kit (Qiagen, Hilden, Germany). The PCR products were then quantified using a Quant-iT PicoGreen dsDNA Assay Kit (P7589, Invitrogen). An Illumina TruSeq Nano DNA LT Sample Prep Kit (Illumina, San Diego, CA, United States) was used to generate PCR amplicon libraries, after which the library quality was assessed with Agilent High Sensitivity DNA Kit (Agilent Technologies, Inc., United States).
Illumina MiSeq High-Throughput Sequencing and Data Analysis
High throughput sequencing was conducted by Personal Biotechnology, Co., Ltd. (Shanghai, China) on an Illumina MiSeq sequencing platform. The overlapping paired-end reads were assembled using PEAR software and poor-quality sequences were removed using QIIME (v1.8.0) and USEARCH (v5.2.236) (Caporaso et al., 2010; Luo et al., 2017). High-quality sequences with 97% similarity were assigned to operational taxonomic units (OTUs) using UCLUST. The taxonomic information of norB-denitrifying bacteria was obtained using Ribosomal Database Project (RDP) (Release 11.11) and the fungal sequences were taxonomically classed using UNITE database (Release 5.02) (Wang et al., 2007; Edgar, 2010). The alpha and beta diversity of norB-type denitrifying bacterial and fungal communities were respectively analyzed using QIIME (v1.8.0). The alpha diversity of the species complexity of each sample was determined using the Chao1, ACE, Shannon, and Simpson indices. The beta diversity was determined by non-metric multidimensional scaling (NMDS) using R software, which reflects the differences in microbial communities among groups. Permutational multivariate analysis of variance (PERMANOVA) was performed by QIIME accompanied with NMDS. Linear discriminant analysis effect size analysis was used to respectively reveal the bacterial and fungal taxa at all taxonomic levels with significantly differential abundance between groups, which was carried out by Galaxy online analysis platform3. Network analysis for investigation of the interactions between the dominant genera was also performed using Mothur software (Schloss et al., 2009).
All of the raw sequencing data used in this study were submitted to the NCBI Sequence Read Archive (SRA) database with the accession number PRJNA544895/SRP199549.
Statistical Analyses
Statistical analyses were performed using SPSS v22.0 (IBM, Inc., Armonk, NY, United States). The data were analyzed by one-way analyses of variance (ANOVAs) and independent t-tests, and the results reported were the means ± standard deviation (SD) of three biological replicates for each treatment. The least significant difference (LSD) test was performed using P < 0.05 as the threshold. Spearman’s correlation coefficient (rho) was calculated using SPSS 22.0.
Results
Effects of T. indicum Colonization on C. illinoinensis Seedlings
Four months after inoculation, mycorrhization was successfully detected on the inoculated C. illinoinensis seedlings, while other uninoculated seedlings (CK) had not been colonized by truffles based on morphological evidence (Figure 1). Comparison of the P0S0 treatment and CK revealed that T. indicum inoculation significantly increased the plant height and POD activity in roots, but significantly decreased the root SOD activity (P < 0.05) (Table 1). The root activity and stem circumference were higher in the P0S0 treatment than in CK, but not significantly. T. indicum inoculation had no noticeable effect on the root-shoot ratio and biomass.
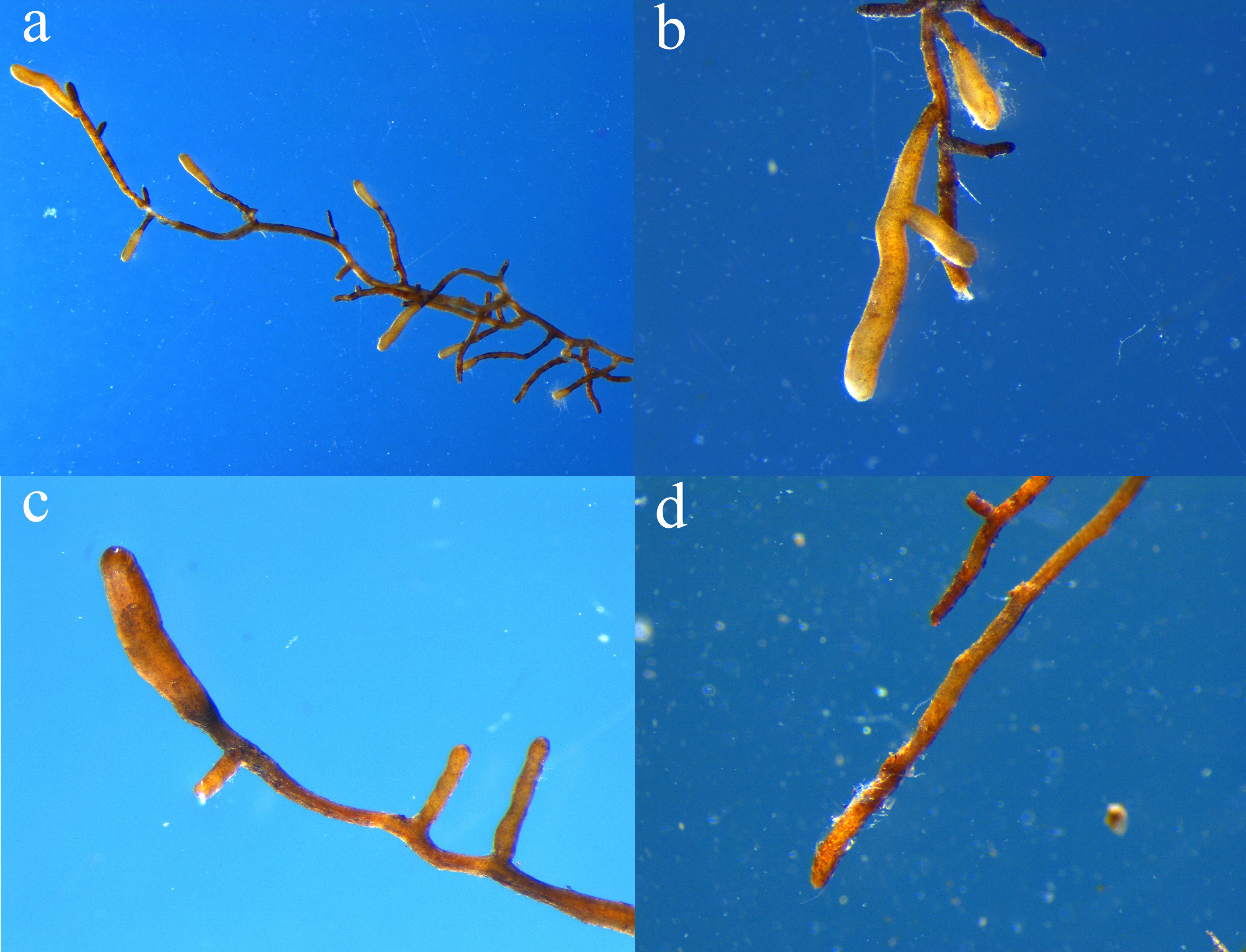
Figure 1. Ectomycorrhizae of Carya illinoinensis seedlings with Tuber indicum (a–c) and the roots of C. illinoinensis seedlings that were not colonized by T. indicum (d).
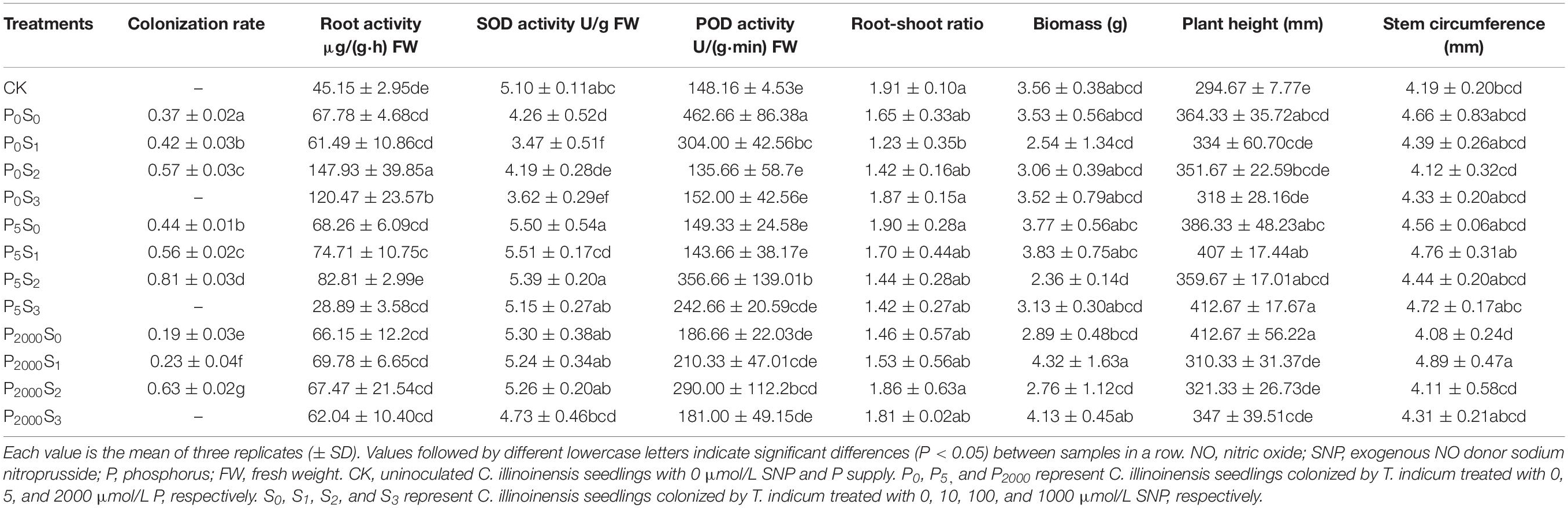
Table 1. The colonization rate of T. indicum and the growth of C. illinoinensis seedlings under different concentrations of exogenous NO donor SNP and P treatments.
Effects of Exogenous NO Combined With P Stress on C. illinoinensis Seedlings Colonized by T. indicum
The colonization rate of T. indicum and the physiological indices of C. illinoinensis seedlings were significantly affected by different concentrations of exogenous NO and P (Table 1). Under the same P concentration, the colonization rate of T. indicum on C. illinoinensis seedlings significantly increased as the SNP concentration increased from 0 to 100 μmol/L (P < 0.05), but when the SNP concentration increased to 1000 μmol/L, there were no ectomycorrhizae successfully detected. Under the same concentration of SNP (except 1000 μmol/L), the colonization rate was significantly higher when the P concentration was 5 μmol/L (P < 0.05) and reached a maximum of 81 ± 3% in P5S2 treatment.
The different concentrations of P had no noticeable effect on the plant height, stem circumference, root-shoot ratio or biomass of seedlings (Table 1). In the P0 and P5 treatments, no differences in these four indices under different SNP concentrations were observed, while in the P2000 treatments, the stem circumference and biomass were significantly higher when the SNP concentration was 10 μmol/L compared to when SNP was 0 and 100 μmol/L (P < 0.05).
The highest root activity was observed in the P0S2 treatment. Specifically, no significant differences were observed among the three different P levels when the SNP concentration was 0 or 10 μmol/L, but at 100 μmol/L, the root activity significantly decreased as the P concentration increased (P < 0.05). In the P0 and P5 treatments, root activity was significant higher when SNP was 100 μmol/L (P < 0.05), and there were no significant differences in P2000 treatments. The SOD activity of roots in the P0 treatments were significantly lower than in the P5 and P2000 treatments (P < 0.05). There was no noticeable effect of different SNP concentrations on root SOD activity. The maximum POD activity was observed in the P0S0 treatment, followed by the P5S2 treatment. In the P5 treatments, POD activity was significantly higher when the SNP was 100 μmol/L (P < 0.05) and there were no significant differences between P2000 treatments. Overall, the colonization rate and the physiological indices of the root system were higher when the SNP concentration was 100 μmol/L and the P content was low.
Effects of Solely Exogenous NO (100 μmol/L SNP) on the Growth of C. illinoinensis Seedlings Colonized by T. indicum
The morphology of the inoculated seedlings and their root systems are shown in Figure 2. The seedlings supplied by SNP grew better and had more lateral roots. The ectomycorrhizae of the two different treatments both occurred on the third month after inoculation, but the indicators of T. indicum colonization were more noticeable in the SNP treatment. On the sixth month after inoculation, the colonization rate of T. indicum reached 88 ± 2% on the seedlings to which 100 μmol/L SNP were applied, which was significantly higher than that of the seedlings in the Control-M treatment (62 ± 3%) (P < 0.05).
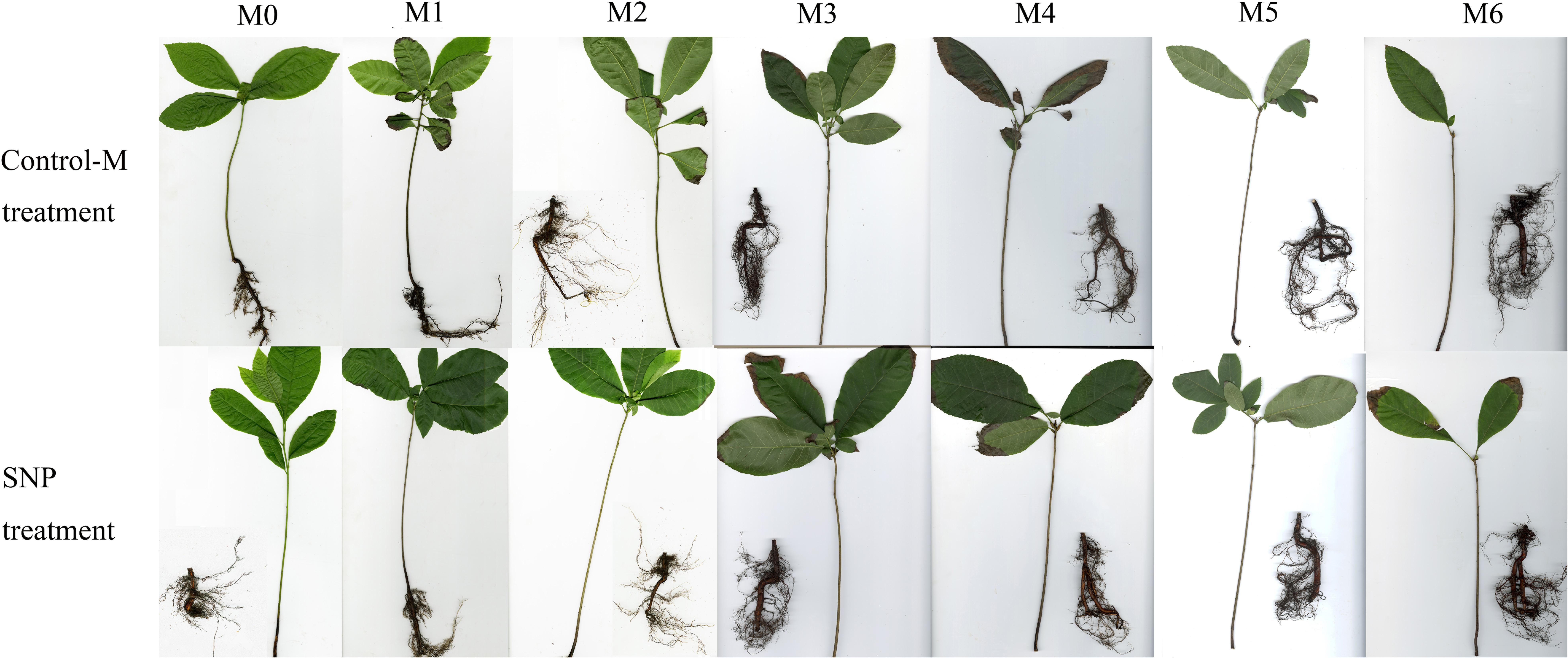
Figure 2. Morphology of inoculated C. illinoinensis seedlings and their root system with or without exogenous NO (100 μmol/L SNP). Control-M treatment, inoculated seedlings that did not receive the 100 μmol/L SNP treatment; SNP treatment, inoculated seedlings treated with 100 μmol/L SNP. M0, M1, M2, M3, M4, M5, and M6 represent seedlings harvested at month 0, 1, 2, 3, 4, 5, and 6, respectively, after T. indicum inoculation.
Sodium nitroprusside treatment significantly increased the plant height from the second month to the sixth month compared with the Control-M (P < 0.05), and the stem circumference became significantly thicker in the SNP treatment from the third to the sixth month (P < 0.05) (Figure 3). The biomass was higher in the SNP treatment, and significant differences were observed between the treatments on the third and fourth month (P < 0.05). SNP treatment also significantly increased the root-shoot ratio on the third, fifth and sixth month (P < 0.05). The POD activity in roots differed significantly between the two treatments on the first and second month, and was also higher in response to SNP treatment (P < 0.05). However, SNP treatment had no noticeable effect on the SOD activity in roots because there were no significant differences between the two treatments after inoculation. Root activity was significantly lower in response to SNP treatment during the fourth and fifth month (P < 0.05).
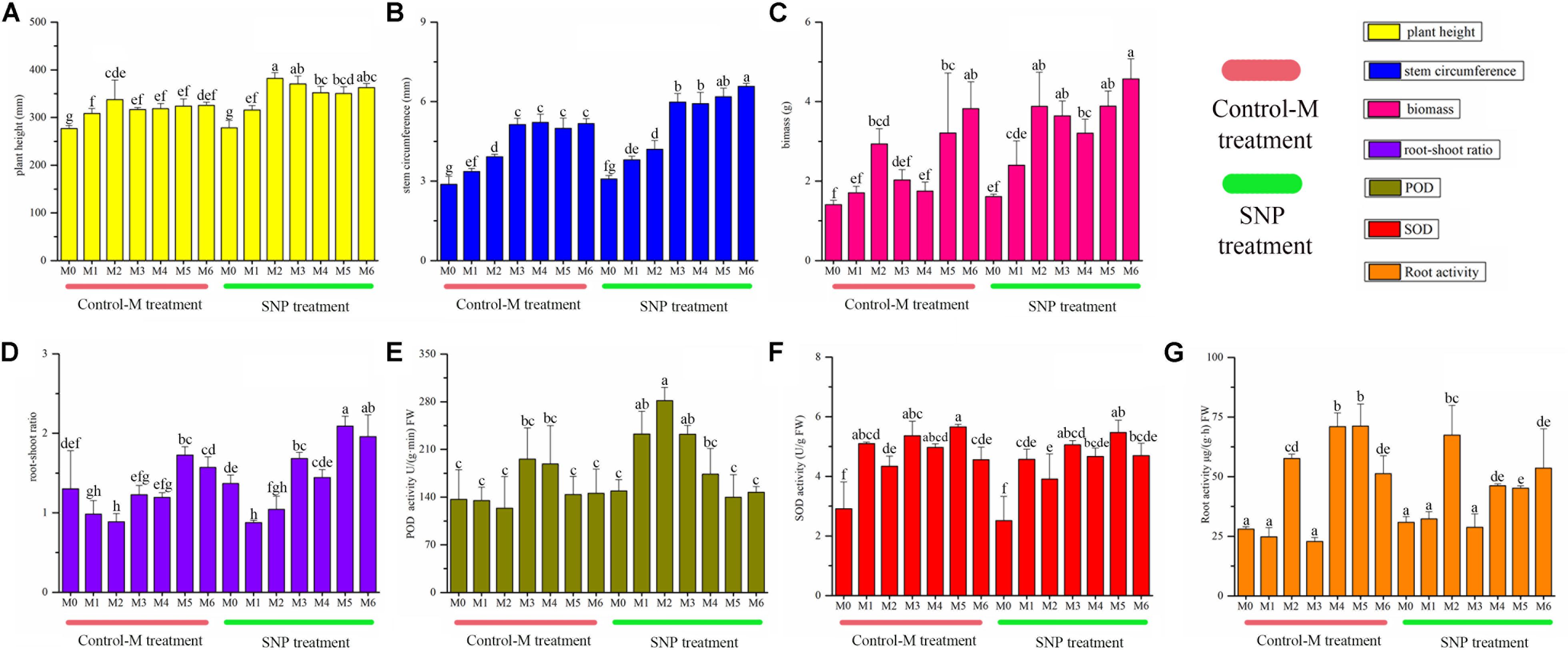
Figure 3. Growth indicators of inoculated C. illinoinensis seedlings with or without exogenous NO (100 μmol/L SNP) at different growth months after inoculation. (A) plant height, (B) stem circumference, (C) biomass, (D) root-shoot ratio, (E) POD activity in roots, (F) SOD activity in roots, (G) root activity. Each value is the mean of three replicates (±SD) in a treatment. Different lowercase letters indicate significant differences between all of the treatments in different months after inoculation (P < 0.05 in the LSD test of ANOVA). Control-M treatment: inoculated seedlings that did not receive 100 μmol/L SNP; SNP treatment: inoculated seedlings treated with 100 μmol/L SNP. M0, M1, M2, M3, M4, M5, and M6 represent seedlings harvested at month 0, 1, 2, 3, 4, 5, and 6, respectively, after T. indicum inoculation.
Analyses of norB-Type Denitrifying Bacterial Communities
Alpha Diversity of norB-Type Denitrifying Bacteria in Rhizosphere Soil
Sequencing of the rhizosphere soils of the CK treatment and those treated with different concentrations of SNP under low P stress were yielded 947,615 high-quality sequences from all 15 samples after quality control, which were clustered into 4,387 OTUs (Supplementary Figure S1a). The Venn diagram revealed 469 shared OTUs among the samples of the five different treatments (Figure 4), with the number of unique OTUs in treatment P5S2 being highest, followed by that in the P5S3 treatment. The unique number of OTUs in P5S0 and P5S1 was even lower than that in CK.
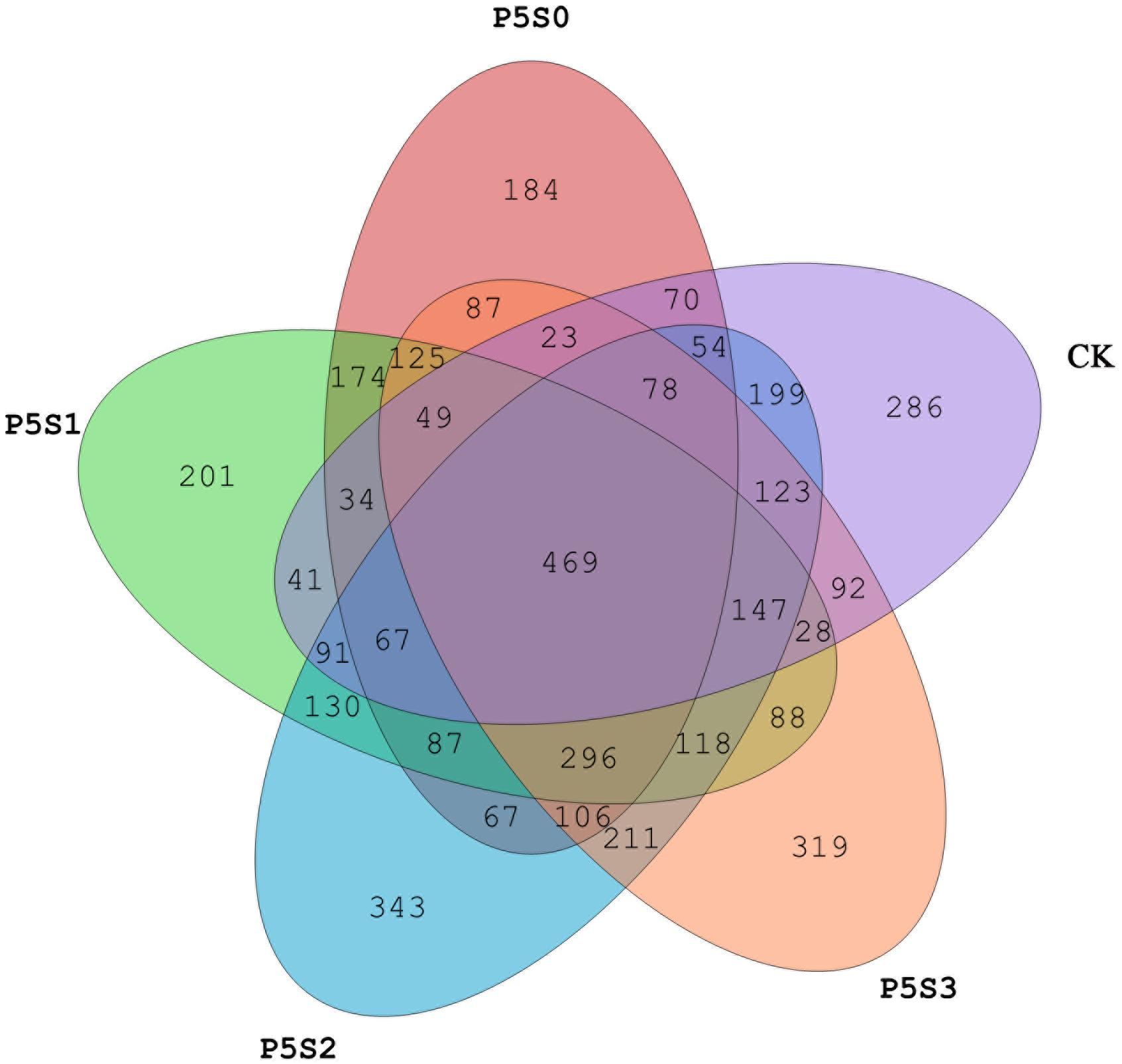
Figure 4. Shared and unique norB-type denitrifying bacterial operational taxonomic units (OTUs) among rhizosphere soil samples in CK treatment and in different SNP treatments under low P stress. CK, the rhizosphere soil of uninoculated C. illinoinensis seedlings that received 0 μmol/L SNP and P application. P5S0, P5S1, P5S2, and P5S3 represent the rhizosphere soil of C. illinoinensis seedlings colonized by T. indicum treated with 0, 10, 100, and 1000 μmol/L SNP, respectively, under low P stress (5 μmol/L).
The two diversity indices (Shannon and Simpson) showed no significant differences among the five treatments (Table 2), indicating that the effects of treatment with different concentrations of SNP on the diversity of norB-type denitrifying bacteria were not significant. The estimated richness indices (Chao1 and ACE) revealed that the norB-type denitrifying bacterial community richness was highest in the P5S2 treatment, and was significantly higher than that in the P5S0 and CK treatments (P < 0.05).
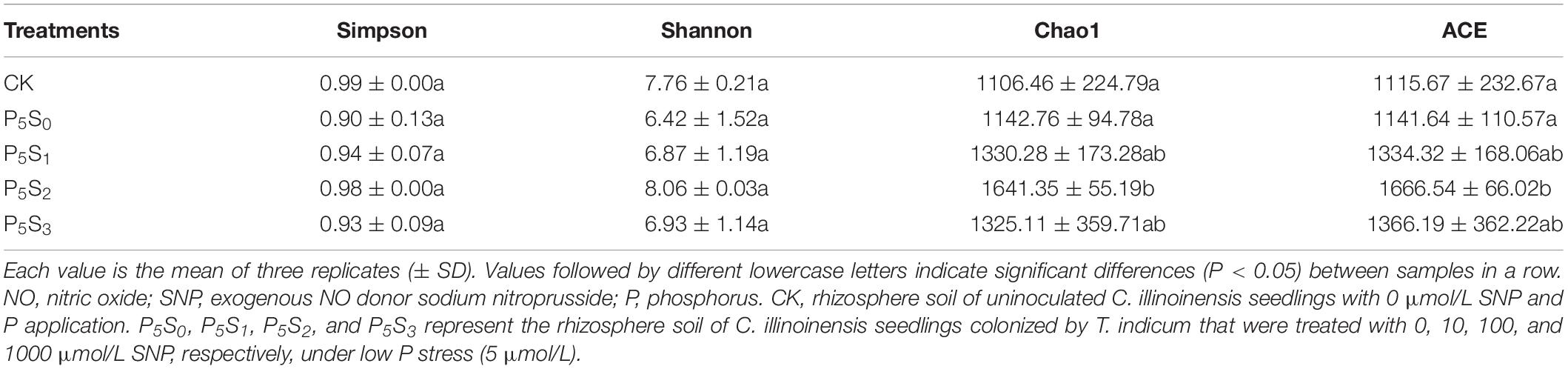
Table 2. The richness and diversity indices of norB-type denitrifying bacteria in rhizosphere soil of C. illinoinensis seedlings with different concentrations of exogenous NO donor SNP treatment under low P stress.
Taxonomic Composition of norB-Type Denitrifying Bacterial Communities
In the 15 samples from the five different treatments, a total of 10 phyla, 16 classes, 35 orders, 56 families, and 95 genera of norB-type denitrifying bacterial communities were detected. At the phylum level, Proteobacteria was the most abundant phylum, accounting for 98.33% (Supplementary Figure S2), followed by Actinobacteria (1.07%) and Acidobacteria (0.42%). The relative abundance of these three phyla showed no significant differences among the five treatments.
At the class level (Figure 5A), Alphaproteobacteria (75.70%), Gammaproteobacteria (20.07%), and Betaproteobacteria (2.50%) were the dominant taxa. Under low P stress, the abundance of Alphaproteobacteria gradually increased as the SNP concentrations increased from 0 to 100 μmol/L and then decreased when the SNP concentration was 1000 μmol/L. Alphaproteobacteria was significantly more abundant in the CK and P5S2 groups than in the P5S0 and P5S3 groups (P < 0.05). The abundance of Gammaproteobacteria was lowest in the CK and P5S2 groups, while the P5S3 treatment contained significantly more Gammaproteobacteria than the CK (P < 0.05). Betaproteobacteria was more abundant in the P5S1 group.
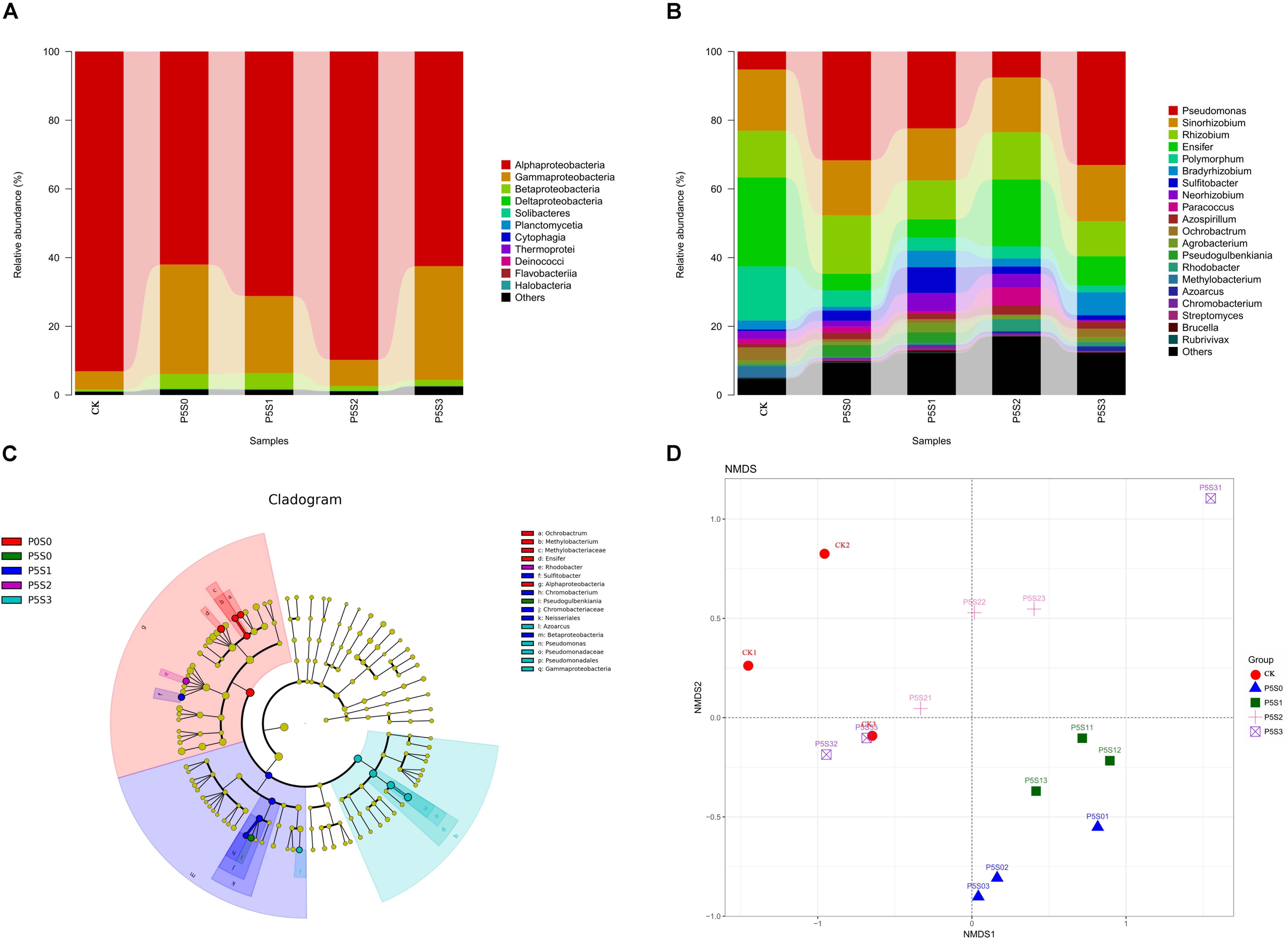
Figure 5. Taxonomic composition of norB-type denitrifying bacterial communities at the (A) class and (B) genus levels in rhizosphere soil of C. illinoinensis seedlings in CK treatment and in different SNP treatments under low P stress. (C) Cladogram based on linear discriminant analysis effect size (LEfSe) analysis (P < 0.05, LDA score > 2) showing significant differences in abundance of norB-type denitrifying bacterial taxa in different groups; (D) non-metric multidimensional scaling (NMDS) analysis of norB-type denitrifying bacterial communities in rhizosphere soil of C. illinoinensis seedlings with different treatments. All treatments were had three replicates. CK, rhizosphere soil of uninoculated C. illinoinensis seedlings that received 0 μmol/L SNP and P application. P5S0, P5S1, P5S2, and P5S3 represent the rhizosphere soil of C. illinoinensis seedlings colonized by T. indicum and treated with 0, 10, 100, and 1000 μmol/L SNP, respectively, under low P stress (5 μmol/L).
At the genus level (Figure 5B), the most abundant genera were Pseudomonas (19.97%), Sinorhizobium (16.27%), Rhizobium (13.20%), Ensifer (12.80%), Rhodobacteraceae_unidentified (8.70%), Polymorphum (5.96%), Bradyrhizobium (3.52%), and Sulfitobacter (2.88%). Under low P stress, Pseudomonas abundance gradually decreased as the SNP concentrations increased from 0 to 100 μmol/L, then increased to the maximum when the SNP concentration was 1000 μmol/L. The abundance of Pseudomonas in the P5S3 group was significantly higher than in the CK (P < 0.05) (Figure 5C). Sinorhizobium and Rhizobium showed no significant differences among the five treatments. Ensifer was significantly more abundant in the CK (P < 0.05) (Figure 5C), and the change in its abundance was contrary to that of Pseudomonas. Polymorphum was also significantly more abundant in CK (P < 0.05), while Sulfitobacter was significantly more abundant in the P5S1 group (P < 0.05) than in the other groups (Figure 5C).
Structural Differentiation and Network Associations of norB-Type Denitrifying Bacterial Communities
The differences in the norB-type denitrifying bacterial community structure among the five treatments were visualized by NMDS analysis (PERMANOVA, P = 0.001) (Figure 5D). The norB-type denitrifying bacterial community structures of the P5S0 and P5S1 treatment were similar and differed significantly from those of the other treatments. The community structure of the P5S2 treatment also differed from that of other treatments.
Among the top 50 genera of norB-type denitrifying bacterial communities, 36 showed correlations with others (Supplementary Figure S4a). Pseudomonas was negatively correlated with Ensifer and Paracoccus. Sulfitobacter was positively correlated with Pseudogulbenkiania, Chromobacterium, and Anaeromyxobacter, while it was negatively correlated with Methylobacterium.
Correlation Analysis Between norB-Type Denitrifying Bacterial Community and Colonization Rate of T. indicum
There were significant correlations between the colonization rate of T. indicum and the richness and diversity of norB-type denitrifying bacterial communities (P < 0.05) (Supplementary Table S3). Based on the Chao1 and ACE indices, colonization rate was positively correlated with the richness of the norB-type denitrifying bacterial communities. Additionally, the Shannon and Simpson indices indicated that colonization rate was positively correlated with the diversity of norB-type denitrifying bacterial communities.
Analyses of Fungal Communities
Fungal Alpha Diversity in Rhizosphere Soil
Overall, 1,824,064 high-quality sequences were obtained from the 42 samples collected during different months after quality control procedures. These sequences were clustered into 1,452 OTUs in all, and the rarefaction curves of the fungal OTUs in different samples are shown in Supplementary Figure S1b. The Venn diagram displays the degree of overlap of the fungal OTUs between the samples in the two treatments (Figure 6). The number of the unique OTUs in the SNP treatment was 166, which was twofold lower than that in the Control-M treatment.
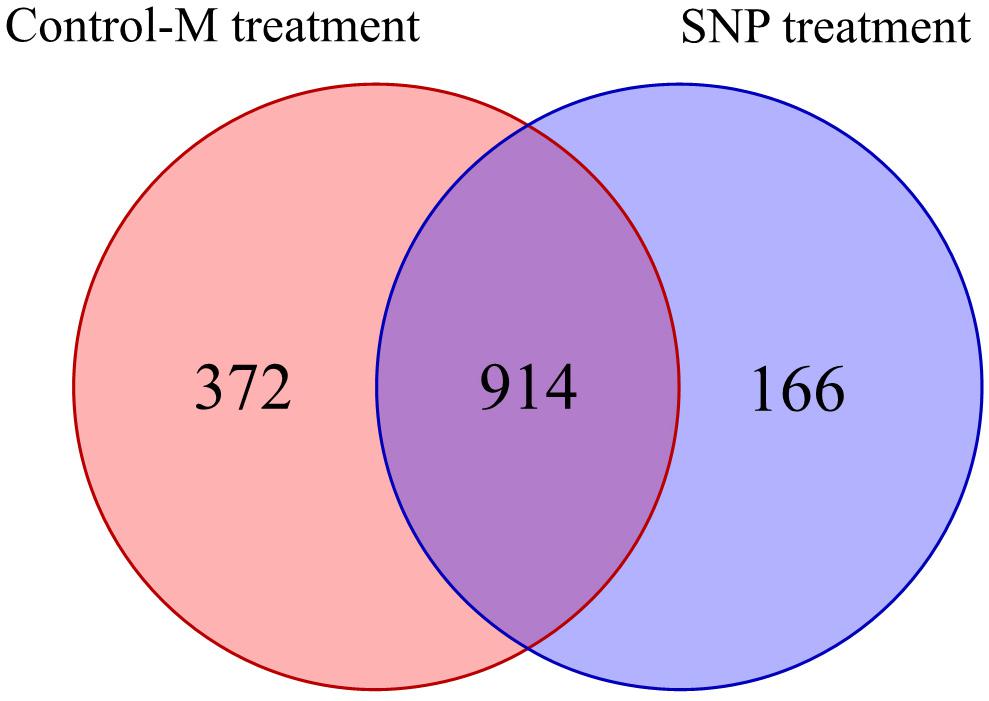
Figure 6. Shared and unique fungal operational taxonomic units among rhizosphere soil samples of inoculated C. illinoinensis seedlings with or without exogenous NO (100 μmol/L SNP) application. Control-M treatment, inoculated seedlings that did not receive 100 μmol/L SNP; SNP treatment, inoculated seedlings that received 100 μmol/L SNP.
Based on the Chao1 and ACE indices, fungal community richness of the rhizosphere soil did not differ significantly between the SNP treatments and Control-M treatments during each month (Table 3). Additionally, the Simpson index indicated that fungal diversity did not differ significantly between the two different treatments during each month. The Shannon index indicated that the fungal diversity was lowest in the fourth month in the two different treatments, but was significantly higher in the Control-M treatment in the third month compared with the SNP treatment (P < 0.05). In general, the SNP treatment did not have any noticeable effect on the diversity and richness of fungal communities in rhizosphere soil at different growth times.
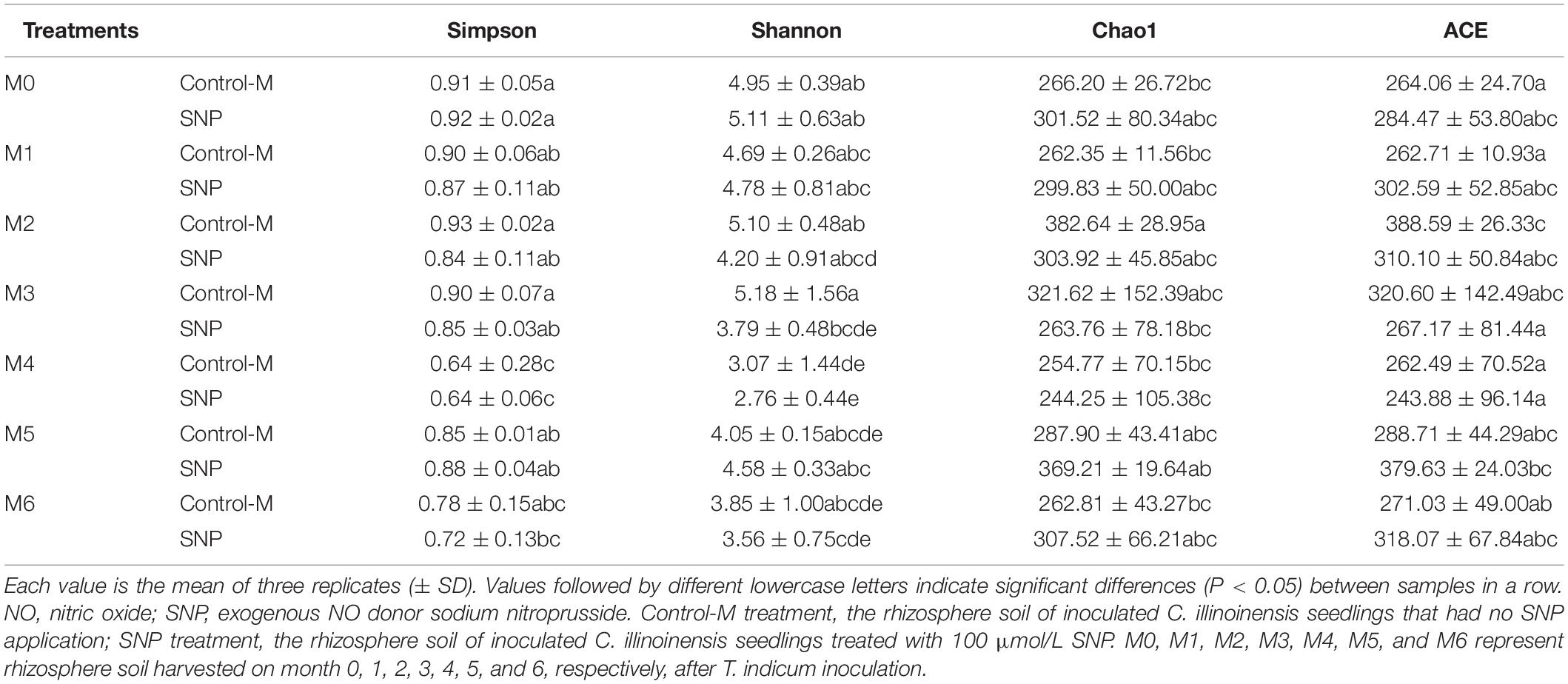
Table 3. The richness and diversity indices of fungal communities in rhizosphere soil of inoculated C. illinoinensis seedlings with or without exogenous NO (100 μmol/L SNP) application during different growth months.
Taxonomic Composition of Fungal Communities
Among the 42 samples of SNP and Control-M treatments, a total of 9 phyla, 30 classes, 84 orders, 177 families, and 291 genera were detected. At the phylum level, Ascomycota (80.26%) was the dominant fungal phylum, followed by Basidiomycota (10.41%) and Zygomycota (4.08%) (Figure 7A). The relative abundance of these three phyla showed no significant differences between the SNP treatments and Control-M treatments in each month.
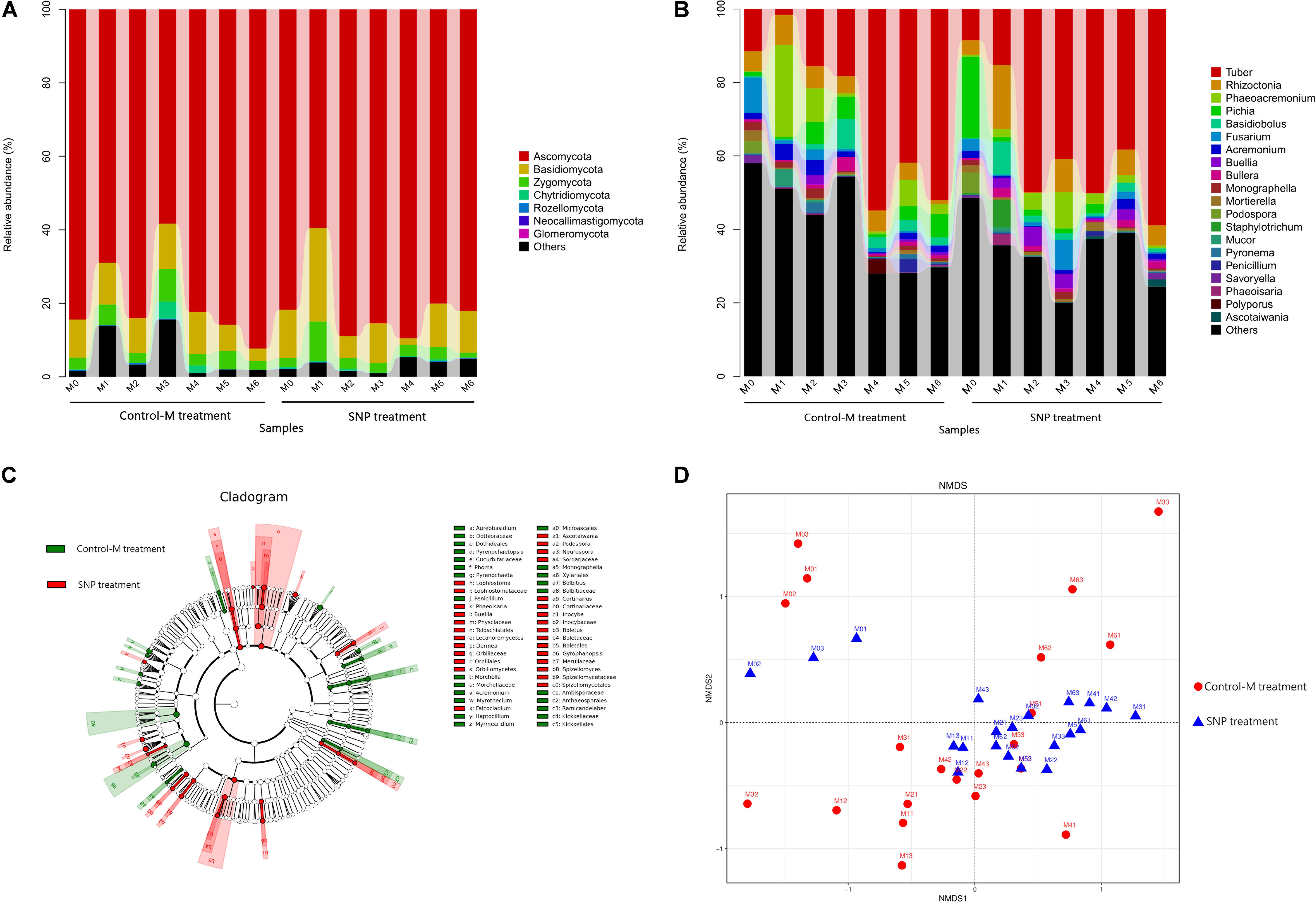
Figure 7. Taxonomic composition of fungal communities at the (A) phylum and (B) genus levels in rhizosphere soil of inoculated C. illinoinensis seedlings with or without 100 μmol/L SNP during different growth months. (C) Cladogram based on linear discriminant analysis effect size (LEfSe) analysis (P < 0.05, LDA score > 2) showing the significantly differentially abundant fungal taxa in the rhizosphere soil of inoculated C. illinoinensis seedlings with or without SNP application. (D) Non-metric multidimensional scaling analysis of fungal communities in rhizosphere soil of inoculated C. illinoinensis seedlings with or without SNP application during different growth months. All of the treatments were conducted with three replicates. Control-M treatment, inoculated seedlings that did not receive 100 μmol/L SNP application; SNP treatment, inoculated seedlings that received 100 μmol/L SNP. M0, M1, M2, M3, M4, M5, and M6 represent seedlings harvested at month 0, 1, 2, 3, 4, 5, and 6, respectively, after T. indicum inoculation.
At the class level, Pezizomycetes (41.06%), Sordariomycetes (18.32%), Agaricomycetes (10.53%), and Saccharomycetes (8.58%) were the dominant taxa (Supplementary Figure S3). The relative abundance of Pezizomycetes did not differ between the SNP treatments and Control-M treatments during each month. However, the SNP treatment significantly decreased the abundance of Sordariomycetes on the first month and decreased that of Saccharomycetes on the second month when compared with the Control-M treatment (P < 0.05).
At the genus level, the top 10 of the most abundant genera were Tuber (32.68%), Rhizoctonia (5.64%), Phaeoacremonium (4.93%), Pichia (3.81%), Basidiobolus (2.53%), Fusarium (2.27%), Acremonium (1.71%), Buellia (1.44%), Bullera (1.30%), and Monographella (0.98%) (Figure 7B). Tuber abundance was higher in the SNP treatment, but this difference was not significant. The relative abundance of Tuber, Rhizoctonia, Phaeoacremonium, and Basidiobolus did not differ significantly between the two treatments during each month. However, Pichia abundance was significantly lower in the SNP group than in the Control-M on the second month (P < 0.05). The abundance of Fusarium was also significantly lower in the SNP treatment on the first and second month (P < 0.05). In the SNP groups, Tuber showed significantly greater abundance from the second to the sixth month compared with month 0 and 1 (P < 0.05). However, in Control-M treatment, Tuber abundance increased from the fourth month, and was significantly more abundant compared with months 0, 1, 2, and 3 (P < 0.05).
Differentially Abundant Taxa and Network Associations of Fungal Communities
Linear discriminant analysis effect size (LEfSe) analysis was used to reveal the fungal taxa that showed significantly different abundance between the SNP and Control-M treatments (P < 0.05) (Figure 7C). At the phylum level, there were no differentially abundant phyla between treatments. At the class level, the samples of the SNP treatments contained significantly more Orbiliomycetes and Lecanoromycetes. At the family level, the relative abundances of Physciaceae, Orbiliaceae, Boletaceae, Lophiostomataceae, Inocybaceae, and Cortinariaceae were significantly higher in the SNP treatments, while the abundances of Dothioraceae and Kickxellaceae were significantly higher in the Control-M treatments. At the genus level, among the top 50 genera, Buellia, Podospora, Phaeoisaria, Ascotaiwania, and Lophiostoma were significantly more abundant in the SNP treatments while Acremonium, Monographella, and Penicillium were significantly more abundant in the Control-M treatments.
Among the top 50 genera, 39 showed correlations with others (Supplementary Figure S4b). Tuber was negatively correlated with Archaeorhizomyces, Podospora and Penicillium, but positively correlated with Tricholoma.
Structural Differentiation of Fungal Communities
Differences in fungal community structure among samples were visualized by NMDS analysis (PERMANOVA, P = 0.008) (Figure 7D). In SNP treatments, the fungal community structure of samples at month 0 differed obviously from those of other months. Analogously, in Control-M treatments, the fungal community structure of the samples at month 0, from month 0 to month 5, and at month 6 differed from each other. Comparison of the SNP treatments and Control-M treatments revealed that the fungal community structures of the two treatments differed in the same month, indicating that exogenous NO can shape the fungal community structure to a certain degree.
Discussion
As ectomycorrhizal fungi, the successful and efficient synthesis of ectomycorrhizae is the basis for the artificial cultivation of truffles (Li et al., 2017). The ability of truffles to colonize plant roots and successfully form ectomycorrhizae can be affected by various abiotic and biotic factors such as soil properties, soil fertility, soil microorganisms, and vegetation (Slankis, 1974). Thus, the surrounding environment and management measures are important to the symbiosis of truffles and host plants. In this study, different concentrations of exogenous NO donor SNP and P were provided to the C. illinoinensis seedlings. The shifts in the colonization levels of T. indicum, in the growth of host plants, and in the associated microbes of rhizosphere soil were then investigated during the early symbiotic stage.
The colonization rate of truffles reflects the degree of mycorrhization (García-Montero et al., 2008). In our study, different concentrations of exogenous NO and P had significant effects on the colonization rate of T. indicum with C. illinoinensis seedlings. The colonization rate reached a high level (81 ± 3%) when SNP was 100 μmol/L under low P stress (5 μmol/L). Previous research showed that plants could be less dependent on ectomycorrhizae for P absorption when more soil P is available, and that ectomycorrhizal colonization may be greater under P-limited conditions (Kluber et al., 2012). When compared with the high P treatments (2000 μmol/L) in the present study, the colonization levels of T. indicum significantly increased under low P stress. However, excessive P deficiency (no P treatments) did not contribute to colonization of T. indicum. The trend in colonization levels was P5 > P0 > P2000. These findings indicated that the plants could adjust their root architecture in response to low P conditions (Niu et al., 2013). The growth of primary roots was inhibited and the development of lateral roots, cluster roots and root hair was promoted to improve the P uptake. This adjustment seemed to provide more attached sites for ectomycorrhizal fungi, which was beneficial to the colonization of truffles. NO in plants was demonstrated to participate in the response to low P conditions (Niu et al., 2013; Simontacchi et al., 2015). P deficiency enhanced NO accumulation in primary and lateral roots. Previous studies confirmed that the appropriate concentration of SNP could promote cluster roots proliferation and lateral root development (Lira-Ruan et al., 2013; Corpas and Barroso, 2015; Sun et al., 2015). In the present study, 100 μmol/L SNP was found to be optimal for T. indicum colonization under different P concentrations, and high concentrations of SNP could completely inhibit T. indicum colonization. Treatment of C. illinoinensis seedlings with 100 μmol/L SNP alone also significantly increased the colonization rate of T. indicum (88 ± 2%). In previous studies, the colonization rate of truffles was between approximately 40 and 60%, depending on the truffle species species and host plant (García-Montero et al., 2008; Geng et al., 2009; Benucci et al., 2012; Li et al., 2018). Therefore, this increase in colonization rate caused by 100 μmol/L SNP could be applied to the ectomycorrhizal synthesis of truffles and material exchange between mycorrhizal fungi and host plants, which may be useful in the artificial cultivation of truffles.
In addition to the colonization rate, the quality of the host plant also contributes to the success or failure of truffle crops (Andres-Alpuente et al., 2014). If plant growth was improved while the ectomycorrhizal level was not affected, the truffle yields may be better or earlier (Bonet et al., 2006). T. indicum inoculation significantly increased the plant height and root POD activity of C. illinoinensis seedlings in this study, but had negative effect on the root SOD activity. Previous research showed that the growth of Pinus halepensis seedlings could be improved by T. melanosporum inoculation and that the nutrient uptake of the seedlings was also improved (Dominguez et al., 2012). T. indicum colonization on several Chinese indigenous trees could also lead to better growth of the host, showing higher ground diameter increases, plant height, and biomass compared with the uninoculated seedlings (Hu, 2004), which was similar with our results. However, further analysis is needed to explain the decrease in root SOD activity. Under low P stress, the maximum root and POD activity was obtained when the SNP concentration was 100 μmol/L, which was consistent with the colonization rate. Evaluation of various abiotic stresses revealed that SNP with appropriate concentration could enhance the activity of the antioxidant system in plants, such as SOD and POD (Yang et al., 2012; Arora and Bhatla, 2015). The increase in POD activity indicated that 100 μmol/L SNP improved the ability of the host plants to cope with stress. However, under low P stress, SOD activity was highest at 10 μmol/L SNP, and improvement of SOD activity in response to exogenous NO was not as great as the improvement of POD activity. Many studies have shown that exogenous NO application promoted plant growth under various stresses; however, the effects of exogenous NO on plants that formed symbiotic relationships with ectomycorrhizal fungi have rarely been reported (Dong et al., 2014; Liu et al., 2014; Kaya and Ashraf, 2015). In the present study, application of only 100 μmol/L SNP to inoculated C. illinoinensis seedlings induced positive effects on plant height, stem circumference, biomass, root-shoot ratio, and POD activity of seedlings, but the variations in these indicators were not synchronous during the 7 months after inoculation. Therefore, treatment with 100 μmol/L SNP could improve the growth of host plants colonized by truffles to a certain degree; however, the effect of SNP on the artificial cultivation of truffles and fructification requires further verification in the field.
Rhizosphere soil microbes play important roles in ecological environments associated with truffles, contributing to ectomycorrhizae synthesis and truffle production, as well as the formation of truffle aroma (Splivallo et al., 2015; Vahdatzadeh et al., 2015). Moreover, microbes in rhizosphere soil participate in plant growth as well as the plant tolerance to disease and abiotic stress (Choudhary, 2012). Using high-throughput sequencing, the effects of exogenous NO at different concentrations under low P stress on bacteria harboring norB-type genes in rhizosphere soil were analyzed in this study. Some studies have reported that NO was frequently involved in the early basal signaling of interactions between plant roots and bacteria, which greatly influenced the root growth patterns and the accumulation of major nutrients (Simontacchi et al., 2015; Vaishnav et al., 2018). NO was also found to promote the formation of biofilms in bacteria (Vaishnav et al., 2016). Under low P stress, the exogenous NO did not influence the diversity of norB-type denitrifying bacteria in the present study, but did increase their richness when 100 μmol/L SNP was applied. Interestingly, the diversity and richness of norB-type denitrifying bacteria were significantly correlated with the colonization rate of T. indicum. This indicated that an interactive network may exist among the NO, norB-type denitrifying bacteria community and the colonization of truffles. Many studies have investigated the role of NO in symbiotic interactions, and exogenous NO has been reported to promote the establishment of the PGPR, i.e., Pseudomonas simiae, strain, which contributed to better colonization and plant growth under saline conditions (Vaishnav et al., 2016). However, the role of NO in symbiotic interactions of ectomycorrhizal fungi is not clear. In the present study, exogenous NO affected some dominant populations of norB-type denitrifying bacteria under low P stress. Alphaproteobacteria was more abundant while Gammaproteobacteria was less abundant when SNP was applied at 100 μmol/L. Alphaproteobacteria and Gammaproteobacteria comprised the predominant components of the bacterial communities of truffles (Barbieri et al., 2007; Li et al., 2017). The abundance of the Pseudomonas genus was P5S3 > P5S0 > P5S1 > P5S2, which was contrary to the colonization rate. However, Pseudomonas was reported to play a role in ectomycorrhizal symbiosis and P. fluorescens is believed to be important to growth and truffle mycorrhizal synthesis (Dominguez et al., 2012; Li et al., 2017). However, further study is needed to explain these phenomena and the interactions of exogenous NO and the denitrifying bacteria associated with truffles.
To date, the effects of exogenous NO on fungal communities in rhizosphere soil have rarely been reported to date. In this study, the effects of only exogenous NO (100 μmol/L SNP) on soil rhizosphere fungi associated with truffles were investigated. Not surprisingly, Tuber was the dominate genus, accounting for 32.36%. These results indicated that exogenous NO did not significantly influence the abundance of T. indicum mycelia during the first 7 months after inoculation. However, the significant increase in Tuber abundance occurred earlier in exogenous NO treatments, which seems to be beneficial to truffle colonization. Previous studies showed that truffle inoculation reduced fungal richness and diversity in the roots and surrounding soil (Li et al., 2017, 2018). No significant effects of exogenous NO on the fungal richness and diversity in rhizosphere soil were observed in this study. NO has been shown to protect roots against further aggression from phytopathogens (Compant et al., 2010). In addition, Buellia, Podospora, Phaeoisaria, Ascotaiwania, and Lophiostoma were found to be more abundant because of exogenous NO application, while the abundance of Acremonium, Monographella, and Penicillium decreased. Network analysis provides an understanding of the potential interactions in microbial communities, and may identify keystone populations (Gu et al., 2018). During the early symbiotic stage, Tuber was positively correlated with Tricholoma, but negatively correlated with Archaeorhizomyces, Podospora, and Penicillium when 100 μmol/L SNP was provided. These fungal communities may be closely related to the growth of truffles under NO application.
Conclusion
Both exogenous NO and P stress affected the ectomycorrhizal synthesis of T. indicum and the growth of host seedlings, with the shift of colonization rate, plant physiology, and some microbial communities in the rhizosphere, which could have potential application in the artificial cultivation of truffles in the future. Also, the mechanism of how exogenous NO and P stress affect the symbionts of truffles and the host also needs to be further explored.
Data Availability Statement
The datasets generated for this study can be found in the NCBI Sequence Read Archive (SRA) database with the accession number PRJNA544895/SRP199549.
Author Contributions
XL, XZ (first author), and XZ (last author) conceived and designed the experiments. CW, ZK, and LY performed the experiments. XZ (first author) and XL wrote and revised the manuscript. All of the authors approved the final version of the manuscript.
Funding
This work was supported by the National Natural Science Foundation of China (No. 31900079), the Project of Sichuan Academy of Agricultural Sciences (2018LWJJ-012), the Science and Technology Support Project in Sichuan Province (2016NYZ0040), and the Sichuan Mushroom Innovation Team.
Conflict of Interest
The authors declare that the research was conducted in the absence of any commercial or financial relationships that could be construed as a potential conflict of interest.
Acknowledgments
We thank LetPub (www.letpub.com) for providing linguistic assistance during the preparation of this manuscript.
Supplementary Material
The Supplementary Material for this article can be found online at: https://www.frontiersin.org/articles/10.3389/fmicb.2019.02634/full#supplementary-material
Footnotes
References
Andres-Alpuente, A., Sanchez, S., Martin, M., Javier Aguirre, A., and Barriuso, J. J. (2014). Comparative analysis of different methods for evaluating quality of Quercus ilex seedlings inoculated with Tuber melanosporum. Mycorrhiza 24, S29–S37. doi: 10.1007/s00572-014-0563-x
Antony-Babu, S., Deveau, A., Van Nostrand, J., Zhou, J., Le Tacon, F., Robin, C., et al. (2014). Black truffle-associated bacterial communities during the development and maturation of Tuber melanosporum ascocarps and putative functional roles. Environ. Microbiol. 16, 2831–2847. doi: 10.1111/1462-2920.12294
Arora, D., and Bhatla, S. C. (2015). Nitric oxide triggers a concentration-dependent differential modulation of superoxide dismutase (FeSOD and Cu/ZnSOD) activity in sunflower seedling roots and cotyledons as an early and long distance signaling response to NaCl stress. Plant Signal. Behav. 10:e1071753. doi: 10.1080/15592324.2015.1071753
Barbieri, E., Guidi, C., Bertaux, J., Frey-Klett, P., Garbaye, J., Ceccaroli, P., et al. (2007). Occurrence and diversity of bacterial communities in Tuber magnatum during truffle maturation. Environ. Microbiol. 9, 2234–2246. doi: 10.1111/j.1462-2920.2007.01338.x
Baudouin, E. (2011). The language of nitric oxide signalling. Plant Biol. 13, 233–242. doi: 10.1111/j.1438-8677.2010.00403.x
Benucci, G. M., Bonito, G., Baciarelli Falini, L., and Bencivenga, M. (2012). Mycorrhization of pecan trees (Carya illinoinensis) with commercial truffle species: Tuber aestivum Vittad. and Tuber borchii Vittad. Mycorrhiza 22, 383–392. doi: 10.1007/s00572-011-0413-z
Benucci, G. M. N., and Bonito, G. M. (2016). The truffle microbiome: species and geography effects on bacteria associated with fruiting bodies of hypogeous pezizales. Microb. Ecol. 72, 4–8. doi: 10.1007/s00248-016-0755-3
Bonet, J. A., Fischer, C. R., and Colinas, C. (2006). Cultivation of black truffle to promote reforestation and land-use stability. Agron. Sustain. Dev. 26, 69–76. doi: 10.1051/agro:2005059
Bonito, G., Trappe, J. M., Donovan, S., and Vilgalys, R. (2011). The Asian black truffle Tuber indicum can form ectomycorrhizas with North American host plants and complete its life cycle in non-native soils. Fungal Ecol. 4, 83–93. doi: 10.1016/j.funeco.2010.08.003
Bortier, M. F., Andivia, E., Genon, J. G., Grebenc, T., and Deckmyn, G. (2018). Towards understanding the role of ectomycorrhizal fungi in forest phosphorus cycling : a modelling approach. Cent. Eur. For. J. 64, 79–95. doi: 10.1515/forj-2017-0037
Calcagno, C., Novero, M., Genre, A., Bonfante, P., and Lanfranco, L. (2012). The exudate from an arbuscular mycorrhizal fungus induces nitric oxide accumulation in Medicago truncatula roots. Mycorrhiza 22, 259–269. doi: 10.1007/s00572-011-0400-4
Caporaso, J. G., Kuczynski, J., Stombaugh, J., Bittinger, K., Bushman, F. D., Costello, E. K., et al. (2010). QIIME allows analysis of high-throughput community sequencing data. Nat. Methods 7, 335–336. doi: 10.1038/nmeth.f.303
Choudhary, D. K. (2012). Microbial rescue to plant under habitat-imposed abiotic and biotic stresses. Appl. Microbiol. Biotechnol. 96, 1137–1155. doi: 10.1007/s00253-012-4429-x
Compant, S., Clément, C., and Sessitsch, A. (2010). Plant growth-promoting bacteria in the rhizo- and endosphere of plants: their role, colonization, mechanisms involved and prospects for utilization. Soil Biol. Biochem. 42, 669–678. doi: 10.1016/j.soilbio.2009.11.024
Corpas, F. J., and Barroso, J. B. (2015). Functions of Nitric Oxide (NO) in roots during development and under adverse stress conditions. Plants 4, 240–252. doi: 10.3390/plants4020240
Cumming, J. R., Zawaski, C., Desai, S., and Collart, F. (2015). Phosphorus disequilibrium in the tripartite plant-ectomycorrhiza-plant growth promoting rhizobacterial association. Soil Sci. Plant. Nutr. 15, 464–485.
Deng, X., Yu, F., and Liu, P. (2014). Contribution to Confirmed and Synthesized on Mycorrhizae of Tuber indicum s.l. with two dominated and subalpine broadleaf trees in Southwestern China. Am. J. Plant Sci. 05, 3269–3279. doi: 10.4236/ajps.2014.521341
Deveau, A., Antony-Babu, S., Le, T. F., Robin, C., Frey-Klett, P., and Uroz, S. J. M. (2016). Temporal changes of bacterial communities in the Tuber melanosporum ectomycorrhizosphere during ascocarp development. Mycorrhiza 26, 389–399. doi: 10.1007/s00572-015-0679-7
Dominguez, J. A., Martin, A., Anriquez, A., and Albanesi, A. (2012). The combined effects of Pseudomonas fluorescens and Tuber melanosporum on the quality of Pinus halepensis seedlings. Mycorrhiza 22, 429–436. doi: 10.1007/s00572-011-0420-0
Dong, Y. J., Jinc, S. S., Liu, S., Xu, L. L., and Kong, J. (2014). Effects of exogenous nitric oxide on growth of cotton seedlings under NaCl stress. J. Plant Nutr. Soil Sci. 14, 1–13.
Edgar, R. C. (2010). Search and clustering orders of magnitude faster than BLAST. Bioinformatics 26, 2460–2461. doi: 10.1093/bioinformatics/btq461
Fridovich, I. (2011). Superoxide dismutases: anti- versus pro- oxidants? Anticancer Agents Med. Chem. 11, 175–177. doi: 10.2174/187152011795255966
Fu, Y., Li, X., Li, Q., Wu, H., Xiong, C., Geng, Q., et al. (2016). Soil microbial communities of three major Chinese truffles in southwest China. Can. J. Microbiol. 62, 970–979. doi: 10.1139/cjm-2016-0139
García-Montero, L. G., Di Massimo, G., Manjón, J. L., and García-Abril, A. (2008). New data on ectomycorrhizae and soils of the Chinese truffles Tuber pseudoexcavatum and Tuber indicum, and their impact on truffle cultivation. Mycorrhiza 19, 7–14. doi: 10.1007/s00572-008-0198-x
Geng, L. Y., Wang, X. H., Yu, F. Q., Deng, X. J., Tian, X. F., Shi, X. F., et al. (2009). Mycorrhizal synthesis of Tuber indicum with two indigenous hosts, Castanea mollissima and Pinus armandii. Mycorrhiza 19, 461–467. doi: 10.1007/s00572-009-0247-0
Gu, Y., Bai, Y., Xiang, Q., Yu, X., Zhao, K., Zhang, X., et al. (2018). Degradation shaped bacterial and archaeal communities with predictable taxa and their association patterns in Zoige wetland at Tibet plateau. Sci. Rep. 8:3884. doi: 10.1038/s41598-018-21874-0
Hu, B. (2004). The mycorrhizal synthesis of Tuber indicum and its affect for growth and against disease on the seedlings. Guizhou Forestry Sci. Technol. 32, 19–24.
Jones, M. D., Durall, D., and Tinker, P. J. N. P. (1990). Phosphorus relationships and production of extrametrical hyphae by two types of willow ectomycorrhizas at different soil phosphorus levels. New Phytol. 115, 259–267. doi: 10.1111/j.1469-8137.1990.tb00451.x
Kaya, C., and Ashraf, M. (2015). Exogenous application of nitric oxide promotes growth and oxidative defense system in highly boron stressed tomato plants bearing fruit. Sci. Hortic. 185, 43–47. doi: 10.1016/j.scienta.2015.01.009
Kluber, L. A., Carrino-Kyker, S. R., Coyle, K. P., DeForest, J. L., Hewins, C. R., Shaw, A. N., et al. (2012). Mycorrhizal response to experimental pH and P manipulation in acidic hardwood forests. PLoS One 7:e48946. doi: 10.1371/journal.pone.0048946
Köhler, J., Yang, N., Pena, R., Raghavan, V., Polle, A., and Meier, I. C. (2018). Ectomycorrhizal fungal diversity increases phosphorus uptake efficiency of European beech. New Phytol. 220, 1200–1210. doi: 10.1111/nph.15208
Kues, U., and Martin, F. (2011). On the road to understanding truffles in the underground. Fungal Genet. Biol. 48, 555–560. doi: 10.1016/j.fgb.2011.02.002
Li, Q., Yan, L., Ye, L., Zhou, J., Zhang, B., Peng, W., et al. (2018). Chinese black truffle (Tuber indicum) alters the Ectomycorrhizosphere and Endoectomycosphere microbiome and metabolic profiles of the host tree Quercus aliena. Front. Microbiol. 9:2202. doi: 10.3389/fmicb.2018.02202
Li, Q., Zhao, J., Xiong, C., Li, X., Chen, Z., Li, P., et al. (2017). Tuber indicum shapes the microbial communities of ectomycorhizosphere soil and ectomycorrhizae of an indigenous tree (Pinus armandii). PLoS One 12:e0175720. doi: 10.1371/journal.pone.0175720
Lira-Ruan, V., Mendivil, S. N., and Dubrovsky, J. G. (2013). Heuristic aspect of the lateral root initiation index: A case study of the role of nitric oxide in root branching. Appl. Plant Sci. 1:1300029. doi: 10.3732/apps.1300029
Liu, P. G., Wang, Y., Wang, X. H., Chen, J., Zheng, H. D., Deng, X. J., et al. (2011). Outline of Chinese truffles and their conservational strategies. J. Fungal Res. 9, 232–243.
Liu, S. L., Yang, R. J., Ma, M. D., Dan, F., Zhao, Y., Jiang, P., et al. (2014). Effects of exogenous NO on the growth, mineral nutrient content, antioxidant system, and ATPase activities of Trifoliumrepens L. plants under cadmium stress. Acta Physiol. Plant 37, e1721. doi: 10.1007/s11738-014-1721-7
Liu, X., Burslem, D. F., Taylor, J. D., Taylor, A. F., Khoo, E., Majalap-Lee, N., et al. (2018). Partitioning of soil phosphorus among arbuscular and ectomycorrhizal trees in tropical and subtropical forests. Ecol. Lett. 21, 713–723. doi: 10.1111/ele.12939
Luo, G., Ling, N., Nannipieri, P., Chen, H., Raza, W., Wang, M., et al. (2017). Long-term fertilisation regimes affect the composition of the alkaline phosphomonoesterase encoding microbial community of a vertisol and its derivative soil fractions. Biol. Fertil. Soils 53, 375–388. doi: 10.1007/s00374-017-1183-3
Luo, Q., Zhang, J., Yan, L., Tang, Y., Ding, X., Yang, Z., et al. (2011). Composition and antioxidant activity of water-soluble polysaccharides from Tuber indicum. J. Med. Food. 14, 1609–1616. doi: 10.1089/jmf.2011.1659
Marozzi, G., Sã, S., Benucci, G. M., Bonito, G., Falini, L. B., Albertini, E., et al. (2017). Mycorrhization of pecan (Carya illinoinensis) with black truffles: Tuber melanosporum and Tuber brumale. Mycorrhiza 27, 303–309. doi: 10.1007/s00572-016-0743-y
Maunoury-Danger, F., Fresneau, C., Eglin, T., Berveiller, D., Francois, C., Lelarge-Trouverie, C., et al. (2010). Impact of carbohydrate supply on stem growth, wood and respired CO2 delta C13: assessment by experimental girdling. Tree Physiol. 30, 818–830. doi: 10.1093/treephys/tpq039
Mello, A., Ding, G. C., Piceno, Y. M., Napoli, C., Tom, L. M., DeSantis, T. Z., et al. (2013). Truffle brules have an impact on the diversity of soil bacterial communities. PLoS One 8:e61945. doi: 10.1371/journal.pone.0061945
Meloni, D. A., Oliva, M. A., Martinez, C. A., and Cambraia, J. (2003). Photosynthesis and activity of superoxide dismutase, peroxidase and glutathione reductase in cotton under salt stress. Environ. Exp. Bot. 49, 69–76. doi: 10.1016/s0098-8472(02)00058-8
Niu, Y. F., Chai, R. S., Jin, G. L., Wang, H., Tang, C. X., and Zhang, Y. S. (2013). Responses of root architecture development to low phosphorus availability: a review. Ann. Bot. 112, 391–408. doi: 10.1093/aob/mcs285
Núñez, J. A. D., González, R. P., and Barreal, J. A. R. (2008). The effect of Tuber melanosporum Vitt. mycorrhization on growth, nutrition, and water relations of Quercus petraea Liebl., Quercus faginea lamk., and Pinus halepensis Mill. seedlings. New For. 35, 159–171. doi: 10.1007/s11056-007-9069-0
Pereira, G., Palfner, G., Chavez, D., Suz, L. M., Machuca, A., and Honrubia, M. (2013). Using common mycorrhizal networks for controlled inoculation of Quercus spp. with Tuber melanosporum: the nurse plant method. Mycorrhiza 23, 373–380. doi: 10.1007/s00572-013-0480-4
Schloss, P. D., Westcott, S. L., Ryabin, T., Hall, J. R., Hartmann, M., Hollister, E. B., et al. (2009). Introducing mothur: open-source, platform-independent, community-supported software for describing and comparing microbial communities. Appl. Environ. Microbiol. 75, 7537–7541. doi: 10.1128/aem.01541-09
Schmidberger, P., and Schieberle, P. (2017). Characterization of the key aroma compounds in white alba truffle (Tuber magnatum pico) and burgundy truffle (Tuber uncinatum) by means of the sensomics approach. J. Agric. Food. Chem. 65, 9287–9296. doi: 10.1021/acs.jafc.7b04073
Simontacchi, M., Galatro, A., Ramos-Artuso, F., and Santa-María, G. E. (2015). Plant survival in a changing environment: the role of nitric oxide in plant responses to abiotic stress. Front. Plant Sci. 6:977. doi: 10.3389/fpls.2015.00977
Slankis, V. (1974). Soil factors influencing formation of mycorrhizae. Annu. Rev. Phytopathol. 12, 437–457. doi: 10.1146/annurev.py.12.090174.002253
Splivallo, R., Deveau, A., Valdez, N., Kirchhoff, N., Freyklett, P., and Karlovsky, P. (2015). Bacteria associated with truffle-fruiting bodies contribute to truffle aroma. Environ. Microbiol. 17, 2647–2660. doi: 10.1111/1462-2920.12521
Streiblova, E., Gryndlerova, H., and Gryndler, M. (2012). Truffle brule: an efficient fungal life strategy. FEMS Microbiol. Ecol. 80, 1–8. doi: 10.1111/j.1574-6941.2011.01283.x
Sun, H., Li, J., Song, W., Tao, J., Huang, S., Chen, S., et al. (2015). Nitric oxide generated by nitrate reductase increases nitrogen uptake capacity by inducing lateral root formation and inorganic nitrogen uptake under partial nitrate nutrition in rice. J. Exp. Bot. 66, 2449–2459. doi: 10.1093/jxb/erv030
Vahdatzadeh, M., Deveau, A., and Splivallo, R. (2015). The role of the microbiome of truffles in aroma formation: a meta-analysis approach. Appl. Environ. Microbiol. 81, 6946–6952. doi: 10.1128/AEM.01098-15
Vaishnav, A., Kumari, S., Jain, S., Varma, A., Tuteja, N., and Choudhary, D. K. (2016). PGPR-mediated expression of salt tolerance gene in soybean through volatiles under sodium nitroprusside. J. Basic Microbiol. 56, 1274–1288. doi: 10.1002/jobm.201600188
Vaishnav, A., Sharma, S. K., Choudhary, D. K., Sharma, K. P., Ahmad, E., Sharma, M. P., et al. (2018). “Nitric oxide as a signaling molecule in plant-bacterial interactions, 183-199,” in Plant Microbiome: Stress Response, Microorganisms for Sustainability, eds D. Egamberdieva, and P. Ahmad (Singapore: Springer Nature).
Wang, Q., Garrity, G. M., Tiedje, J. M., and Cole, J. R. (2007). Naive Bayesian classifier for rapid assignment of rRNA sequences into the new bacterial taxonomy. Appl. Environ. Microbiol. 73, 5261–5267. doi: 10.1128/aem.00062-07
Xue, X., Zhang, S., Li, H., Chen, J., and Huang, J. (2008). Effects of phosphorus on the excretion of oxalate, hydrion and phosphatase by ectomycorrhizal fungi Lactarius deliciosus and Laccaria bicolor. Mycosystema 27, 193–200.
Yang, M. S., Wang, Y. F., Gan, X. X., Luo, H. H., Zhang, Y. L., and Zhang, W. F. (2012). Effects of exogenous nitric oxide on growth, antioxidant system and photosynthetic characteristics in seedling of cotton cultivar under chilling injury stress. J. Scientia Agricultura Sinica 15, 58–67.
Yunfu, G., Wang, Y., Xiang, Q., Yu, X., Zhao, K., Zhang, X., et al. (2017). Implications of wetland degradation for the potential denitrifying activity and bacterial populations with nirS genes as found in a succession in Qinghai-Tibet plateau, China. Eur. J. Soil Biol. 80, 19–26. doi: 10.1016/j.ejsobi.2017.03.005
Zhang, X. Q., Huang, G. Q., Bian, X. M., and Zhao, Q. G. (2012). Effects of nitrogen fertilization and root separation on the plant growth and grain yield of maize and its rhizosphere microorganisms. The journal of applied ecology 23, 3369–3376.
Keywords: Tuber indicum, ectomycorrhizae, nitric oxide, phosphorus stress, truffle
Citation: Zhang X, Li X, Wu C, Ye L, Kang Z and Zhang X (2019) Exogenous Nitric Oxide and Phosphorus Stress Affect the Mycorrhization, Plant Growth, and Associated Microbes of Carya illinoinensis Seedlings Colonized by Tuber indicum. Front. Microbiol. 10:2634. doi: 10.3389/fmicb.2019.02634
Received: 13 August 2019; Accepted: 29 October 2019;
Published: 13 November 2019.
Edited by:
Fred Asiegbu, University of Helsinki, FinlandReviewed by:
Gian Maria Niccolò Benucci, Michigan State University, United StatesQiang Li, Sichuan University, China
Copyright © 2019 Zhang, Li, Wu, Ye, Kang and Zhang. This is an open-access article distributed under the terms of the Creative Commons Attribution License (CC BY). The use, distribution or reproduction in other forums is permitted, provided the original author(s) and the copyright owner(s) are credited and that the original publication in this journal is cited, in accordance with accepted academic practice. No use, distribution or reproduction is permitted which does not comply with these terms.
*Correspondence: Xiaolin Li, a2VycnlsZWVfdHdAc2luYS5jb20=; Xiaoping Zhang, emhhbmd4aWFvcGluZ3BoZEAxMjYuY29t
†These authors have contributed equally to this work