- School of Life Sciences, Northwestern Polytechnical University, Xi’an, China
Endogenous hydrogen sulfide (H2S), which is primarily generated by 3-mercaptopyruvate sulfurtransferase (3-MST) in Escherichia coli (E. coli) under aerobic conditions, renders bacteria highly resistant to oxidative stress. However, the biosynthetic pathway and physiological role of this gas under anaerobic conditions remains largely unknown. In the present study, we demonstrate that cysteine desulfurase (IscS), not 3-MST, is the primary source of endogenous H2S in E. coli under anaerobic conditions. A significant decrease in H2S production under anaerobic conditions was observed in E. coli upon deletion of IscS, but not in 3-MST-deficient bacteria (ΔmstA). Furthermore, the H2S-producing activity of recombinant IscS using L-cysteine as a substrate exhibited an approximately 2.6-fold increase in the presence of dithiothreitol (DTT), indicating that H2S production catalyzed by IscS was greatly increased under reducing conditions. The activity of IscS was regulated under the different redox conditions and the midpoint redox potential was determined to be −329 ± 1.6 mV. Moreover, in E. coli cells H2S production from IscS is regulated under oxidative and reductive stress. A mutant E. coli (ΔiscS) strain lacking a chromosomal copy of the IscS-encoding gene iscS showed significant growth defects and low levels of ATP under both aerobic and anaerobic conditions. The growth defects could be fully restored after addition of 500 μM Na2S (an H2S donor) under anaerobic conditions, but not by the addition of cysteine, sodium sulfite or sodium sulfate. We also showed that the addition of 500 μM Na2S to culture medium stimulates ATP synthesis in the mutant E. coli (ΔiscS) strain in the logarithmic growth phase but suppresses ATP synthesis in wild-type E. coli. Our results reveal a new H2S-producing pathway in E. coli under anaerobic conditions and show that hydrogen sulfide from IscS contributes to sustaining cell growth and bioenergetics under oxygen-deficient conditions.
Introduction
The noxious gas hydrogen sulfide (H2S) is now recognized as a third gas signaling molecule together with nitric oxide (NO) and carbon monoxide (CO), which play important roles in mammals, including in inflammation, vascular tone, angiogenesis, cancer, and protection against oxidative stress (Chen et al., 2012; Kimura, 2014; Niu et al., 2018a). In addition, H2S can donate electrons to the mitochondrial electron transport chain through sulfide:quinone oxidoreductase, consequently promoting oxidative phosphorylation and increasing mitochondrial ATP production (Fu et al., 2012; Módis et al., 2016). In mammalian cells, the enzymatic process for endogenous H2S production from cysteine is primarily associated with cystathionine β-synthase (CBS, EC 4.2.1.22), cystathionine γ-lyase (CSE, EC 4.4.1.1) and 3-mercaptopyruvate sulfurtransferase (3-MST, EC 2.8.1.2) in combination with cysteine aminotransferase (CAT, EC 2.6.1.3) (Kabil and Banerjee, 2014; Niu et al., 2018b).
Compared with H2S production derived from mammalian cells, the bacterial production of H2S was described in the 19th century but was considered to be a byproduct of sulfur metabolism that lacked physiological roles (Shatalin et al., 2011; Kimura, 2014). Consequently, few studies have been published on the H2S-associated metabolic pathways in bacteria. Recently, Evgeny Nudler et al. reported that most bacterial genomes, if not all, have orthologs of mammalian CSE, CBS or 3-MST, suggesting that these genes may have had important biological functions throughout bacterial evolution (Shatalin et al., 2011). Indeed, in Escherichia coli (E. coli) grown in Luria-Bertani (LB) broth, H2S is primarily generated by 3-MST under aerobic culture conditions and can defend against oxidative stress induced by antibiotics (Shatalin et al., 2011; Mironov et al., 2017). However, most recently, Sergey Korshunov et al. reported that H2S production in 3-MST-deficient E. coli (ΔmstA) grown in minimal medium was not significantly altered compared with that in wild-type E. coli (Korshunov et al., 2016). The basis for the discrepancy between these two results is not known but could result from different components of the culture medium. Since microorganisms that colonize mammalian intestines grow under the oxygen-deficient conditions, the H2S biosynthetic pathway in E. coli and its physiological functions under anaerobic conditions should be characterized.
Cysteine desulfurase (IscS, EC 2.8.1.7) is a pyridoxal phosphate (PLP)-containing enzyme that catalyzes the conversion of cysteine to alanine and sulfane sulfur via the formation of a protein-bound cysteine persulfide intermediate on a conserved cysteine residue, and this enzyme is highly conserved throughout all kingdoms of life (Zheng et al., 1993; Mihara and Esaki, 2002; Li et al., 2006). Cysteine desulfurase acts as a sulfur donor and is involved in biological sulfur trafficking and the assembly of iron-sulfur clusters, which are essential prosthetic groups required for enzymatic catalysis and respiratory chain complexes (Rouault, 2012). In addition, IscS is capable of donating the persulfide sulfur atoms to a variety of biosynthetic pathways for sulfur-containing biofactors, such as thiamin, transfer RNA thionucleosides, biotin and lipoic acid (Hidese et al., 2011). However, a previous study showed that a cysteine desulfurase from Azotobacter vinelandii catalyzes the synthesis of H2S and alanine in the presence of dithiothreitol (Zheng et al., 1993). In the in vitro studies in which sulfur transfer from IscS to acceptors has been reported, reaction mixtures contained levels of reducing agent (1–5 mM DTT) sufficient to release persulfide-bound sulfur from IscS as H2S (Zheng et al., 1993, 1994; Urbina et al., 2001). In plants, cysteine desulfurase is also one of the key enzymes involved in H2S biogenesis (Scuffi et al., 2014). Based on these results, we hypothesize that cysteine desulfurase in E. coli is probably involved in the synthesis of H2S under anaerobic conditions and that endogenous H2S production may sustain cellular bioenergetics under oxygen-deficient conditions.
In this study, we report that endogenous H2S production in E. coli under anaerobic conditions was primarily generated from cysteine desulfurase but not 3-mercaptopyruvate sulfurtransferase. A mutant E. coli (ΔiscS) strain lacking IscS activity was observed to display a remarkable decrease in H2S production under anaerobic conditions, but not under aerobic conditions. H2S generated by purified recombinant IscS exhibited an approximately 2.6-fold increase in the presence of DTT. Furthermore, the addition of exogenous Na2S (an H2S donor), but not cysteine, sodium sulfite or sodium sulfate can stimulate ATP synthesis in the mutant E. coli (ΔiscS) strain under anaerobic conditions, indicating that H2S from cysteine desulfurase in E. coli contributes to sustaining cell growth and bioenergetics.
Materials and Methods
Strains, Plasmids and Chemicals
The plasmid pET-28a (Novagen, United States) was used for IscS expression. E. coli DH5α (Tiangen, China) was used to amplify recombinant plasmids, and E. coli BL21(DE3) pLysS (Tiangen) was the host strain for IscS expression. E. coli BW25113 and the mutant strain BW25113 (ΔiscS) were kindly provided by Jingdan Liang from Shanghai Jiao Tong University (An et al., 2012). The mutant strain BW25113 (ΔcyuA) was kindly provided by Sheng Yang from Chinese academy of science. Sodium phenylpyruvate (PPNa) and diamide were obtained from TCI (Shanghai, China). Dithiothreitol (DTT), trans-4,5-dihydroxy-1,2-dithiane (DTToxi), 7-Azido-4-methylcoumarin, lead nitrate and L-cysteine were obtained from Sigma-Aldrich (St. Louis, United States). The other materials used in this study were purchased from Sangon (Shanghai, China). Unless otherwise specified, all chemicals were used as they were received.
Expression and Purification of IscS
The E. coli cysteine desulfurase iscS gene GenBank (Gene ID: 947004) was cloned using E. coli DH5α genomic DNA as template for PCR. All the primers used in this study are listed in Supplementary Table S1. To express IscS in E. coli, the iscS gene was inserted into the BamHI and XhoI sites of the expression vector pET-28a in the correct reading frame and was transformed into E. coli BL21(DE3) pLysS.
Recombinant E. coli cells with the IscS expression constructs were grown in LB medium containing kanamycin (50 μg/ml) and chloramphenicol (50 μg/ml) until the optical density at 600 nm (OD600) reached 0.6–0.8. The expression of IscS was induced by adding 0.2 mM isopropyl β-D-1-thiogalactopyranoside (IPTG), and the cells were further cultured for 16 h at 30°C. The harvested cells were resuspended in lysis buffer containing 50 mM phosphate buffered saline (PBS; pH 7.4), 300 mM NaCl and 30 mM imidazole. The resuspended cells were then sonicated using an Ultrasonic Cell Disruption System (Scientz, China). Subsequently, the supernatant was collected by centrifugation at 12,000 rpm for 30 min at 4°C and was loaded onto a HisTrap FF column (GE Healthcare, United States) pre-equilibrated with lysis buffer. The recombinant IscS was eluted with 300 mM imidazole in 50 mM PBS, pH 7.4, and the collected fractions were desalted using a HiTrap Desalting column (GE Healthcare) that had been equilibrated with 50 mM Tris-HCl, pH 8.0. The fractions containing IscS protein were pooled and stored at −80°C. Protein concentration was determined by the BCA protein assay reagent kit (TransGen Biotech, China), and bovine serum albumin was used as a standard.
Characterization of Purified IscS
The activity of IscS was quantified using the methylene blue method as previously described, with the following modifications (Urbina et al., 2001). First, H2S production in the reaction is trapped by Pb(NO3)2 to produce lead sulfide (PbS). Subsequently, in acid solution PbS reacts with N, N-dimethyl-p-phenylenediamine to produce methylene blue in the presence of FeCl3. The reaction mixture (total volume of 200 μl) contained 50 mM Tris, 1 mM DTT, 0.4 mM [Pb(NO3)2], pH 8.0, and 5 μg IscS. The reaction was initiated by the addition of 1 mM L-cysteine and was incubated for 30 min at 37°C, after which the reaction was terminated by the addition of 25 μl of 20 mM N, N-dimethyl-p-phenylenediamine in 7.2 M HCl and 25 μl of 30 mM FeCl3 in 1.2 M HCl. After incubating for 15 min, the samples were centrifuged at 12,000 rpm for 10 min. The supernatants were then transferred to 96-well plates, and the absorbance was determined at 670 nm in a multifunctional microplate reader. The H2S concentration was calculated using a standard curve that was prepared using different concentrations of sodium sulfide.
The effect of the redox potential on the activity of IscS was determined in solutions with various redox potentials as previously described (Niu et al., 2018b). Redox buffers were prepared in a 50 mM Hepes buffer containing various concentrations of DTT (9.9–0.025 mM) and DTToxi (0.1–9.975 mM), pH 7.4. The total DTT concentration in the redox buffers was 10 mM, and all solutions were de-oxygenated by bubbling with nitrogen for 30 min. The DTT/DTToxi redox potential was calculated according to the Nernst equation (Eq. 1),
Where E0 = −352 mV at pH 7.4, R is the gas constant, T is the absolute temperature, and F is Faraday’s constant, n = 2, and [DTToxi] and [DTT] are molar concentrations of oxidized and reduced DTT, respectively. Subsequently, recombinant IscS (5 μg) was preincubated in solutions with different redox potentials for 1 h at 37°C, and the reaction was initiated by the addition of 10 mM S-methylcysteine. After incubation for 30 min at 37°C, the H2S-producing activity of IscS was measured using the methylene blue method.
The effect of pH on the activity of IscS was determined using two buffer systems, sodium phosphate (pH 6.0–7.5) and Tris-HCl (pH 8.0–10.5) in the presence of 1 mM DTT. The kinetics of IscS activity was investigated using varying concentrations of L-cysteine in the presence 1 mM DTT, and the kinetic constant Km was calculated using non-linear regression in GraphPad Prism version 7 (Martin, 1997).
H2S Production Detection
A lead nitrate detection method was used to monitor H2S production in the wild-type and mutant E. coli cells as previously described (Mustafa et al., 2009). Filter paper (Whatman, United Kingdom) saturated with 2% [Pb(NO3)2] was affixed to the culture bottle mouth above the level of the liquid culture. Overnight cultures were diluted 1:100 in LB medium and incubated for 8 h at 37°C. To anaerobically culture the wild-type and mutant E. coli strains, the culture medium was deoxygenated by nitrogen bubbling for 10 min. Stained paper was scanned and quantified using Image-Pro Plus 6.0, and the results were normalized by OD600.
A commercially available fluorescent H2S probe 7-azido-4-methylcoumarin (AzMC) was used to measure H2S production in cells (Chen et al., 2013). The mutant E. coli strains (ΔmstA and ΔiscS) were cultured in a sealed culture bottle at 37°C under anaerobic conditions. After 3 h of culture, H2O2 and DTT were injected into the sealed culture bottles at a final concentration of 1 mM and 5 mM respectively followed by incubation for 1 h at 37°C. Subsequently, the cultures were collected by centrifugation at 4,000 rpm at 4°C for 10 min followed by washing 3 times with ice-cold PBS. The precipitate was resuspended in PBS containing 10 μM 7-Azido-4-methylcoumarin (AzMC). The cell suspension was lysed by five cycles of repetitive rapid freezing in liquid nitrogen and thawing in a 37°C water bath. The supernatant was collected by centrifugation at 15,000 rpm at 4°C for 5 min, and the fluorescent intensity was measured with a F-4500 fluorescence spectrophotometer (λex = 365 nm and λem = 450 nm).
Gene Knockouts of mstA and sufS
The ΔmstA, ΔiscSΔmstA and ΔsufS mutant derivatives of strain BW25113 were created using the gene knockout method described by Sheng Yang (Jiang et al., 2015), and the plasmid pCas and pTargetF were gifts from Sheng Yang (Addgene plasmid # 62225; Addgene plasmid # 62226) for use in generating the gene knockout strain. All the primers used in this study are listed in Supplementary Table S1. The mutant E. coli strains were confirmed by PCR and DNA sequencing.
Measurement of Growth Curves
Fifty microliters of overnight culture (1%, v/v) was inoculated into a culture bottle containing 5 ml of LB medium with the appropriate chemicals as described in the text or figure legends. For aerobic conditions, E. coli was grown at 37°C with shaking (250 rpm). For anaerobic conditions, all the cultures were deoxygenated by nitrogen bubbling for 10 min, and E. coli was cultured in a sealed culture bottle at 37°C with shaking (100 rpm). The OD600 values were determined using a spectrophotometer at specific times. To determine the effect of Na2S on the growth of the wild-type and mutant E. coli strains, different concentrations of Na2S were injected into the sealed culture bottles.
Succinate Dehydrogenase (SDH) and Aconitase (ACO) Activity Assays
The activity of succinate dehydrogenase (SDH) in the wild-type and mutant E. coli (ΔiscS) grown under anaerobic conditions with or without 500 μM Na2S was determined using an SDH activity assay kit (Solarbio, Beijing, China). One unit of SDH activity was defined as the amount of enzyme catalyzing the removal of 1 nmol 2,6-dichlorophenol indophenol per minute at 37°C under the specified conditions. The activity of aconitase (ACO) was measured according to the method described by Yarian et al. (2006). One unit of ACO activity was defined as the amount of enzyme catalyzing the generation of 1 nmol NADPH per minute at 37°C.
Detection of ATP Content in E. coli Cells
The ATP content in E. coli cells was determined using the luciferin/luciferase reaction with an ATP Assay Kit (Beyotime, China) following the manufacturer’s instructions. The wild-type E. coli, mutant E. coli (ΔiscS) and E. coli (ΔmstA) strains were growth at 37°C under aerobic or anaerobic conditions as mentioned above. Next, 50 μl of overnight culture was inoculated into a culture bottle containing 5 ml of LB medium. To determine the effect of Na2S on the synthesis of ATP in the wild-type and mutant E. coli strains, and Na2S solution was injected into the sealed culture bottle at a final concentration of 500 μM. After incubation for 2.5, 5, 7.5, and 10 h, the cultures were centrifuged at 4,000 rpm at 4°C for 10 min to collect the precipitate, which was followed by washing 3 times with ice-cold PBS. The precipitate was resuspended in 500 μl of a 50 mM HEPES buffer containing 500 mM NaCl, pH 7.4. The cell suspension was lysed by 5 cycles of a rapid freeze-thaw procedure that involved freezing in liquid nitrogen followed by thawing in a water bath at 37°C. Subsequently, the supernatant was collected by centrifugation at 15,000 rpm at 4°C for 5 min, and 20 μl of the lysate was added to the reaction solution. Luminescence was measured using a bioluminometer, and the results are presented as nmol ATP/mg protein (Zhang et al., 2014).
Results
3-MST Is Not the Primary Source of H2S in E. coli Under Anaerobic Conditions
To investigate whether 3-MST is the primary source of H2S in E. coli under anaerobic conditions, PPNa was used to inhibit the activity of 3-MST (Wing and Baskin, 1992). The growth of E. coli was not significantly inhibited in the presence of 50 mM PPNa (Supplementary Figure S1). H2S production in E. coli cultures was monitored using [Pb(NO3)2]-soaked paper, which specifically reacts with H2S to form a brown lead sulfide precipitate, where the H2S concentration is directly proportional to the change in the rate at which the paper is stained (Mustafa et al., 2009). Under aerobic conditions, 50 mM PPNa remarkably inhibited the biogenesis of H2S by 3-MST in wild-type E. coli (Figure 1A), indicating that 3-MST is the primary source of H2S in this bacterium. This result is consistent with those reported by Evgeny Nudler (Shatalin et al., 2011; Mironov et al., 2017). Surprisingly, 50 mM PPNa did not significantly affect the H2S production in E. coli under anaerobic conditions (Figure 1B). These results suggested that 3-MST is not the primary source of H2S in E. coli under anaerobic conditions.
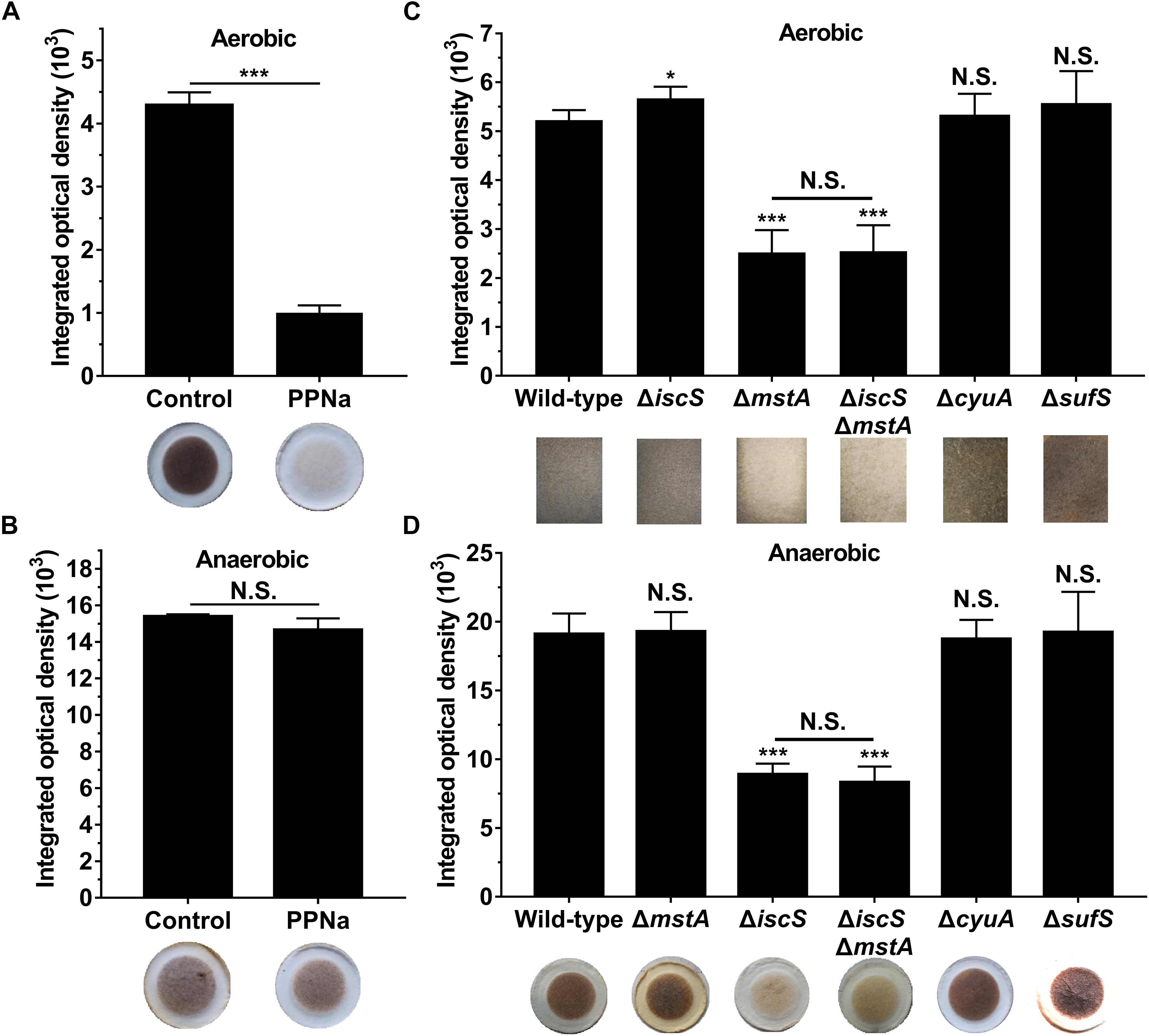
Figure 1. IscS, not 3-MST, is the primary source of H2S in E. coli under anaerobic conditions. (A) H2S production in E. coli cultured under aerobic conditions. (B) H2S production in E. coli cultured under anaerobic conditions. 3-MST activity was inhibited using 50 mM PPNa. H2S production in E. coli cultured without PPNa was used as control group. (C) H2S production in E. coli cultures under aerobic conditions. (D) H2S production in E. coli cultures under anaerobic conditions. H2S production in E. coli cultures was monitored using [Pb(NO3)2]-soaked paper. Saturated papers were quantified using Image Pro Plus 6.0, and the integrated optical density (IOD) value was normalized to the OD600 value of the culture. Each bar represents the mean ± SD of four independent experiments. ∗p < 0.1, ∗∗∗p < 0.001, N.S., not significant, versus the control or wild-type group.
IscS Is the Primary Source of H2S in E. coli Under Anaerobic Conditions
To further verify that 3-MST is not the primary source of H2S in E. coli under anaerobic conditions, a 3-MST-deficient E. coli (ΔmstA) strain and a double mutant (ΔiscSΔmstA) strain were constructed. These mutant E. coli strains were confirmed by agarose gel electrophoresis and sequencing (Supplementary Figure S2a). Additionally, a recent research showed that an iron-sulfur-containing cysteine desulfidase (CyuA) modulates intracellular cysteine concentrations and is the major anaerobic cysteine-catabolizing enzyme in E. coli under the conditions tested (Loddeke et al., 2017). To identify the endogenous source of H2S under anaerobic conditions, a CyuA-deficient E. coli (ΔcyuA) strain was constructed. The mutant E. coli (ΔcyuA) strain was confirmed by agarose gel electrophoresis and sequencing (Supplementary Figure S2b). In addition, SufS is another cysteine desulfurase in E. coli and can also abstract sulfur from cysteine, resulting in the production of alanine and persulfide (Kim et al., 2018; Dunkle et al., 2019). We generated an E. coli mutant (ΔsufS) strain which was verified by agarose gel electrophoresis and sequencing (Supplementary Figure S2c).
First, we investigated the effect of deleting the mstA, iscS, cyuA and sufS genes on H2S biosynthesis in E. coli under both aerobic and anaerobic conditions. Similar to the results obtained for the wild-type E. coli cells treated with the 3-MST inhibitor PPNa, endogenous H2S production in the E. coli (ΔmstA) strain and the double mutant (ΔiscSΔmstA) strain greatly decreased under aerobic conditions, but not under anaerobic conditions. Conversely, H2S biogenesis in the E. coli (ΔiscS) mutant and (ΔiscSΔmstA) mutant dramatically decreased under anaerobic conditions, but not under aerobic conditions (Figures 1C,D). Additionally, the double mutant (ΔiscSΔmstA) strain produced the same level of H2S as the mutant E. coli (ΔmstA) under aerobic conditions and the same level of H2S as the mutant E. coli (ΔiscS) under anaerobic conditions. However, the mutant E. coli (ΔcyuA) strain grown in LB medium generated wild-type levels of H2S both aerobically and anaerobically (Figures 1C,D), which is inconsistent with a previous report that mutant E. coli (ΔcyuA) grown with cysteine generated substantially less H2S under anaerobic conditions (Loddeke et al., 2017). The basis for the discrepancy between these two studies could result from different culture medium and culture conditions. There was no significant difference in endogenous H2S product between the mutant (sufS) strain and a wild type strain both aerobically and anaerobically (Figures 1C,D). Collectively, these results clearly indicated that IscS is the primary source of H2S in E. coli under anaerobic conditions, at least under the conditions tested.
We next investigated the effect of the deletion of the mstA, iscS, cyuA or sufS genes on the growth of E. coli. As is shown in Figure 2 and Supplementary Figure S3, the deletion of iscS significantly decreased the growth rate of E. coli under both aerobic and anaerobic conditions, whereas the deletion of mstA or cyuA or sufS did not.
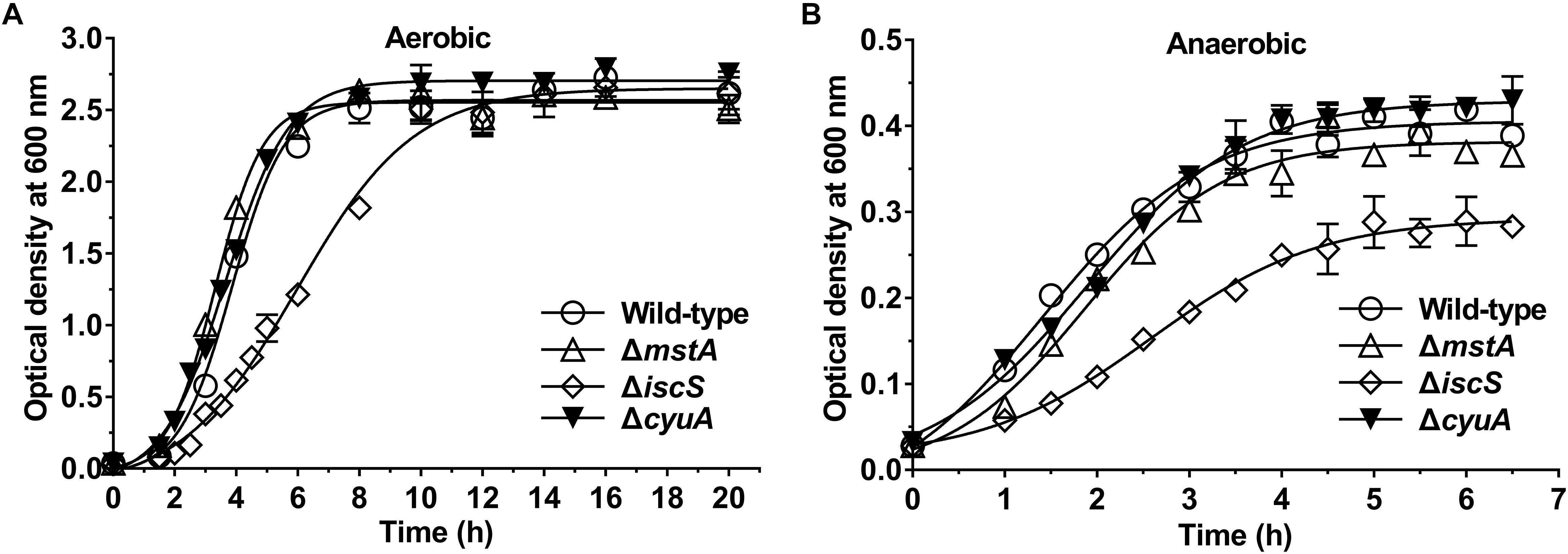
Figure 2. Deletion of the iscS gene, but not the mstA and ΔcyuA gene, significantly decreases the growth rate of E. coli. (A) Growth curves of the wild-type, ΔmstA, ΔiscS and ΔcyuA E. coli strains under aerobic conditions. (B) Growth curves of the wild-type, ΔmstA, ΔiscS and ΔcyuA strains under anaerobic conditions. Each bar represents the mean ± SD of four independent experiments.
The Activity of IscS Is Redox Regulated in vitro and in E. coli Cell
To assess the H2S-producing activity of IscS, the recombinant IscS was purified and the protein purity was judged to be >95% by SDS-PAGE analysis (Figure 3A). The optimum pH of the purified IscS enzyme was 8.0∼8.5 (Supplementary Figure S4a) with a Michaelis constant (Km) of 0.8 ± 0.05 mM at pH 8.0 (Supplementary Figure S4b), similar to the characteristics of the human cysteine desulfurase NFS1 (Marelja et al., 2008), but the Km value is much higher than that observed for E. coli IscS (Urbina et al., 2001). The discrepancy between these two studies could result from different reaction mixtures and reaction conditions. As IscS is a pyridoxal phosphate (PLP)-containing enzyme that generates H2S using L-cysteine as a substrate, the purified protein appears yellow, which has been observed for other PLP-dependent proteins, and it exhibited a maximum absorbance at 385 nm. When 20 mM cysteine was added to the purified IscS enzyme, the absorbance at 385 nm decreased and a concomitant increase at 350 nm was observed (Supplementary Figure S4c).
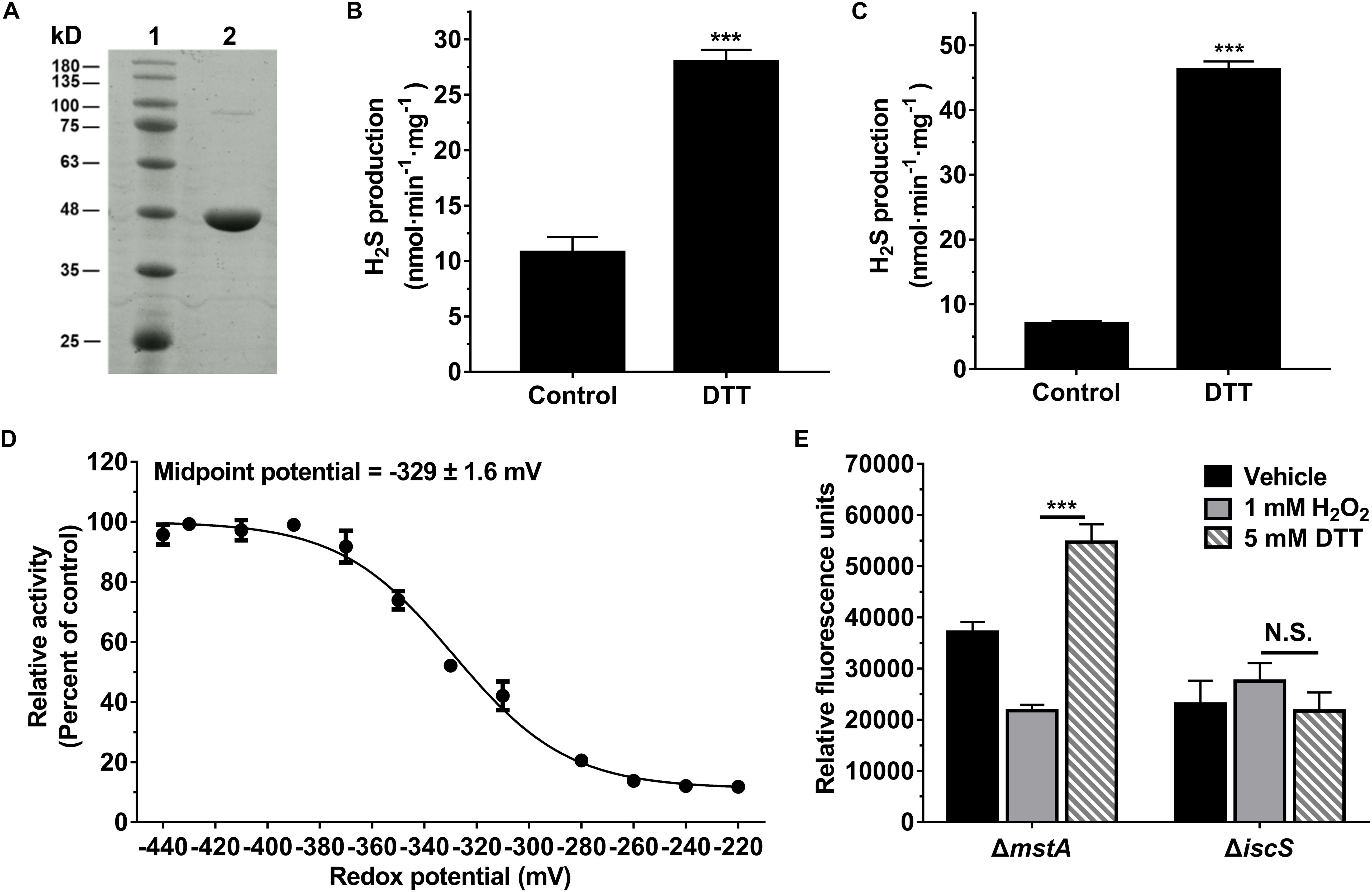
Figure 3. The activity of IscS was redox regulated in vitro and in E. coli cell. (A) SDS-PAGE (12%) analysis of the purified IscS protein: Lane 1 - Molecular mass standards; Lane 2 - Purified IscS protein. (B) H2S-producing activity of the purified IscS protein using L-cysteine as a substrate. Samples without DTT were used as control, and samples without IscS protein were used as blank control, and all values are subtracted by the mean value of blank control. Each bar represents the mean ± SD of four independent experiments. ∗∗∗P < 0.001, versus the control group. (C) H2S-producing activity of purified IscS protein using S-methylcysteine as a substrate. Samples without DTT were used as control, and samples without IscS protein were used as blank control. ∗∗∗P < 0.001, versus the control group. (D) H2S-producing activity of the purified IscS protein was regulated under the different redox conditions. The graph represents the relative activity of the samples compared with control group (samples with 10 mM DTT) and shows the means ± SD (n = 4). The H2S-producing activity of the control group was 59 nmol⋅min– 1⋅mg protein– 1. (E) H2S-producing activity of IscS in E. coli cells was redox-regulated under anaerobic conditions. H2S production in E. coli cell lysates was determined using a fluorescent H2S probe AzMC (10 μM). The fluorescent intensity was measured with a fluorescence spectrophotometer (λex = 365 nm and λem = 450 nm). Relative fluorescence units were normalized to the total protein content of each sample. Each bar represents the mean ± SD of four independent experiments.
The H2S-producing activity of the recombinant IscS was assessed in 50 mM Tris-HCl buffer (pH 8.0) using 1 mM L-cysteine as a substrate in the absence or presence of DTT. The activity of IscS was determined to be 28 nmol⋅min–1⋅mg protein–1 in the presence of 1 mM DTT, 2.6-fold higher than that of IscS in the absence of DTT (Figure 3B). IscS can catalyze the formation of a protein-bound persulfide group using L-cysteine as a substrate (Lauhon et al., 2004). As L-cysteine was used as a substrate and could reduce the persulfide group, a modified assay in which S-methylcysteine, a substrate analog of cysteine, served as the substrate was developed. The H2S-producing activity of IscS in the presence of DTT (46 nmol⋅min–1⋅mg protein–1) was 6.3-fold higher than that of IscS in the absence of DTT (Figure 3C). Collectively, these results showed that recombinant IscS has higher specific activity for H2S biogenesis under reducing conditions.
We next investigated the effect of the redox potential on the activity of IscS. The activity of IscS in solutions with various redox potentials was determined using the methylene blue method. As shown in Figure 3D, the activity of recombinant IscS for H2S generation was regulated under the different redox conditions. The midpoint redox potential was determined to be −329 ± 1.6 mV. Additionally, we determined the H2S content of E. coli cells using a fluorescent probe under oxidative and reductive stress, which were induced by the addition of 1 mM H2O2 or 5 mM DTT. As shown in Figure 3E, the H2S content of the mutant (ΔmstA) strain under reductive stress exhibits a 2.5-fold higher than that under oxidative stress. However, H2S production in an IscS-deficient E. coli (ΔiscS) strain was not affected by the addition of 1 mM or 5 mM DTT. These results indicated that H2S production from IscS is redox regulated in E. coli cells under anaerobic conditions.
Exogenous H2S Promotes the Growth of the E. coli (ΔiscS) Mutant Under Anaerobic Conditions
The energy metabolism pathway in E. coli is greatly altered under aerobic versus anaerobic conditions. Therefore, we speculated that H2S production from IscS would promote the growth of E. coli under anaerobic conditions. In the present study, Na2S was used as a source of exogenous H2S. Indeed, the addition of exogenous Na2S significantly increased the intracellular content of H2S in E. coli cells (Supplementary Figure S5), but did not significantly affect the intracellular content of cysteine (Supplementary Figure S6). First, we investigated the effect of different concentrations of Na2S on the growth rates of the wild-type and the mutant E. coli (ΔiscS) strains under anaerobic conditions. As is shown in Figure 4A, the addition of exogenous Na2S at final concentrations of 20–200 μM did not significantly affect the growth of wild-type E. coli, whereas 500–2000 μM Na2S greatly inhibited its growth (Figures 4A,C). Expectedly, the growth rate of the mutant E. coli (ΔiscS) strain was significantly promoted by the addition of exogenous Na2S at final concentrations of 100–500 μM (Figure 4B), and the cell biomass increased ∼1.6-fold in the presence of 500 μM Na2S (Figures 4B,D). Additionally, the effect of Na2S on the growth rates of the wild-type and the mutant E. coli (ΔiscS) strains under aerobic conditions was also determined. As shown in Figure 4E, 500–2000 μM Na2S significantly inhibited the growth of wild-type E. coli (Figures 4E,G). Interestingly, unlike under anaerobic conditions, 500 μM Na2S did not significantly promote the growth of the mutant E. coli (ΔiscS) strain under aerobic conditions (Figures 4F,H). These results showed that exogenous H2S promotes the growth of the E. coli (ΔiscS) mutant under anaerobic conditions, but not under aerobic conditions.
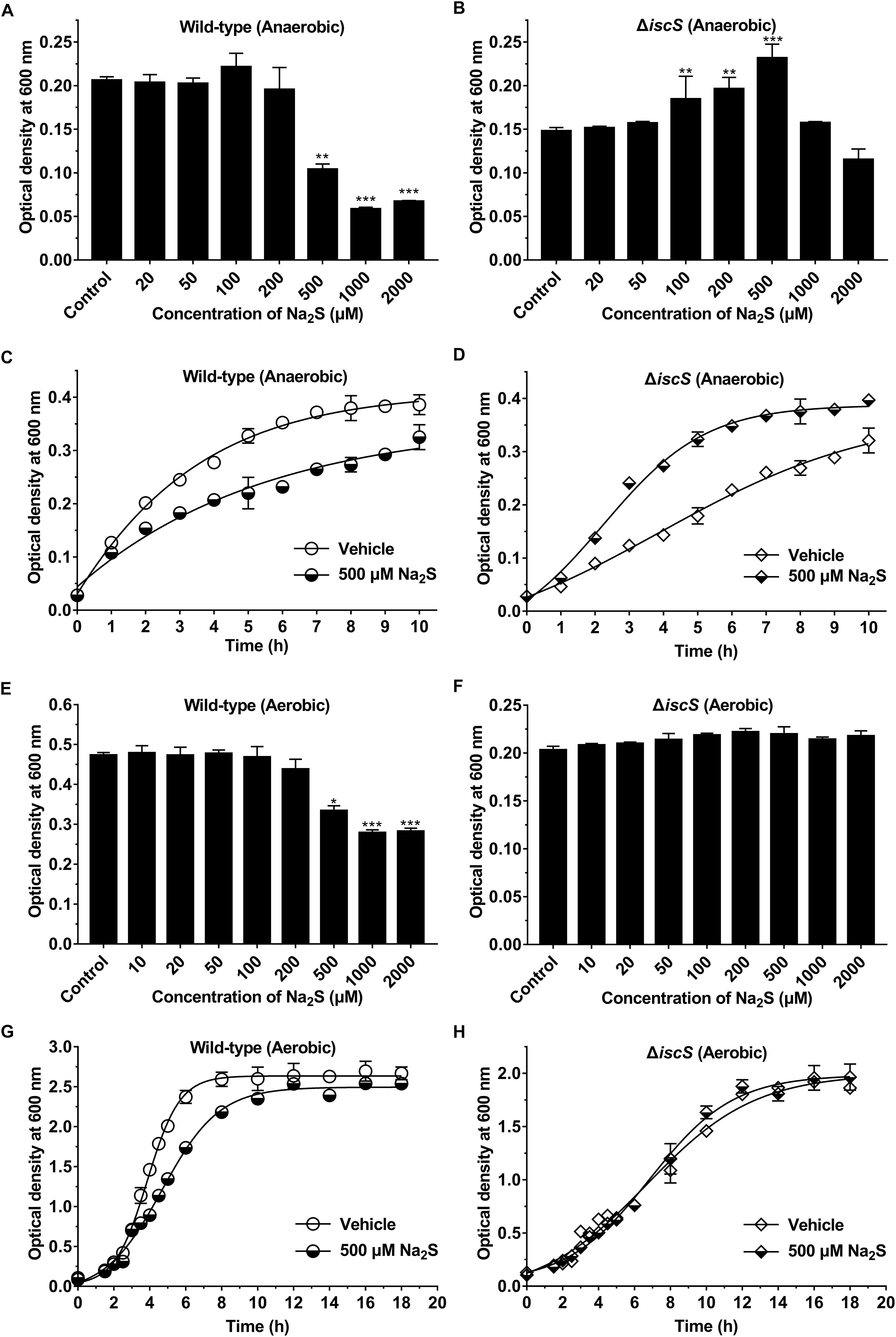
Figure 4. Exogenous H2S promotes the growth of the E. coli (ΔiscS) mutant under anaerobic conditions. (A) The effect of different concentrations of Na2S on the growth of wild-type E. coli under anaerobic conditions. (B) The effect of different concentrations of Na2S on the growth of the E. coli (ΔiscS) strain under anaerobic conditions. (C) The effect of 500 μM Na2S on the growth of wild-type E. coli under anaerobic conditions. (D) The effect of 500 μM Na2S on the growth of the E. coli (ΔiscS) mutant under anaerobic conditions. (E) The effect of different concentrations of Na2S on the growth of wild-type E. coli under aerobic conditions. (F) The effect of different concentrations of Na2S on the growth of the E. coli (ΔiscS) strain under aerobic conditions. (G) The effect of 500 μM Na2S on the growth of wild-type E. coli under aerobic conditions. (H) The effect of 500 μM Na2S on the growth of the E. coli (ΔiscS) mutant under aerobic conditions. The data points and errors show the means ± SD of four independent experiments. ∗p < 0.05, ∗∗p < 0.01, ∗∗∗p < 0.001, versus the control group. Samples without Na2S were used as a control.
Additionally, to assess whether Na2S specifically promotes the growth of E. coli, the effects of L-cysteine (0.1–50 mM), sodium sulfite (0.01–5 mM) and sodium sulfate (0.01–5 mM) on the growth of the wild-type and the mutant E. coli (ΔiscS) strains grown in LB medium and M9 minimal medium under anaerobic conditions were determined. The results indicated that none of the three sulfur compounds could significantly promote the growth of the mutant E. coli (ΔiscS) (Supplementary Figures S7, S8).
As IscS is involved in the biogenesis of iron-sulfur clusters in E. coli, the functions of various Fe-S cluster containing proteins in IscS-deficient mutant E. coli (ΔiscS) are impaired. To investigate whether exogenous H2S rescues Fe-S cluster synthesis in the E. coli (ΔiscS) mutant, the activities of two Fe-S cluster containing enzymes SDH and ACO in E. coli grown with or without 500 μM Na2S were determined. As shown in Figure 5, addition of 500 μM Na2S to culture medium did not significantly increase the activities of these two enzymes in the mutant E. coli (ΔiscS) lysate. These results indicated that growth promotion of the mutant E. coli (ΔiscS) by the addition of Na2S should not be attributed to the rescue of Fe-S cluster synthesis.
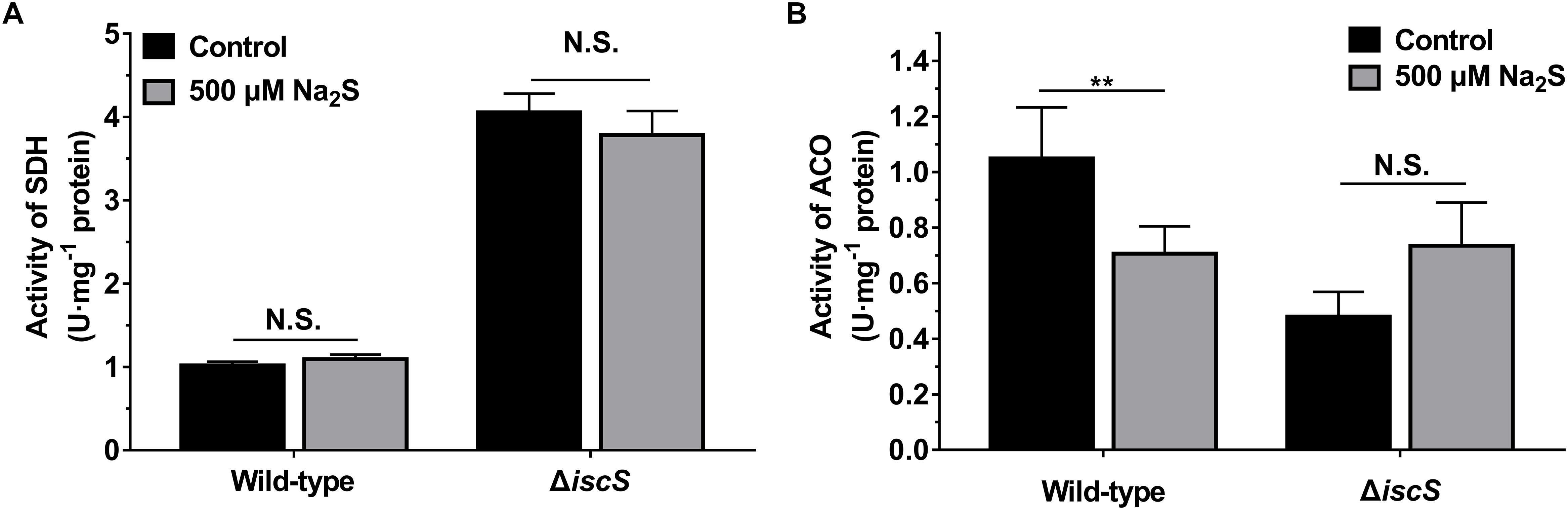
Figure 5. Effect of exogenous H2S on the activities of SDH and ACO in E. coli cells grown under anaerobic conditions. (A) The effect of 500 μM Na2S on the activity of SDH. (B) the effect of 500 μM Na2S on the activity of ACO. Samples without Na2S were used as control. Each bar represents the mean ± SD of four independent experiments. ∗∗p < 0.01, N.S., not significant, versus the control group.
Exogenous H2S Can Promote ATP Synthesis in the E. coli (ΔiscS) Strain Under Anaerobic Conditions
As exogenous H2S promoted the growth of the E. coli (ΔiscS) mutant, we hypothesized that H2S is involved in cellular energy metabolism by stimulating the synthesis of ATP. After a 2.5–10 h incubation of the wild-type E. coli, mutant E. coli (ΔmstA) and E. coli (ΔiscS) strains under anaerobic conditions, the ATP content in cell lysates was measured. As shown in Figure 6A, after incubation for 2.5 h the ATP content in the wild-type E. coli cells decreased from 10.1 to 4.8 nmol mg–1 protein after the addition of 500 μM Na2S to the culture, and the ATP content in the mutant E. coli (ΔmstA) cells decreased from 10.9 to 4.6 nmol mg–1 protein. These results suggested that 500 μM H2S is toxic to wild-type E. coli and mutant E. coli (ΔmstA) under anaerobic conditions. In contrast, the ATP content in the mutant E. coli (ΔiscS) cells increased ∼2.3-fold (from 2.6 to 5.9 nmol mg–1 protein.) in the presence of 500 μM Na2S. However, exogenous Na2S did not significantly affect ATP content in all three strains in the stable growth phase after incubation for 10 h (Figures 4C,D, 6A). These results showed that exogenous Na2S promotes ATP synthesis in the mutant E. coli (ΔiscS) during the logarithmic growth phase under anaerobic conditions.
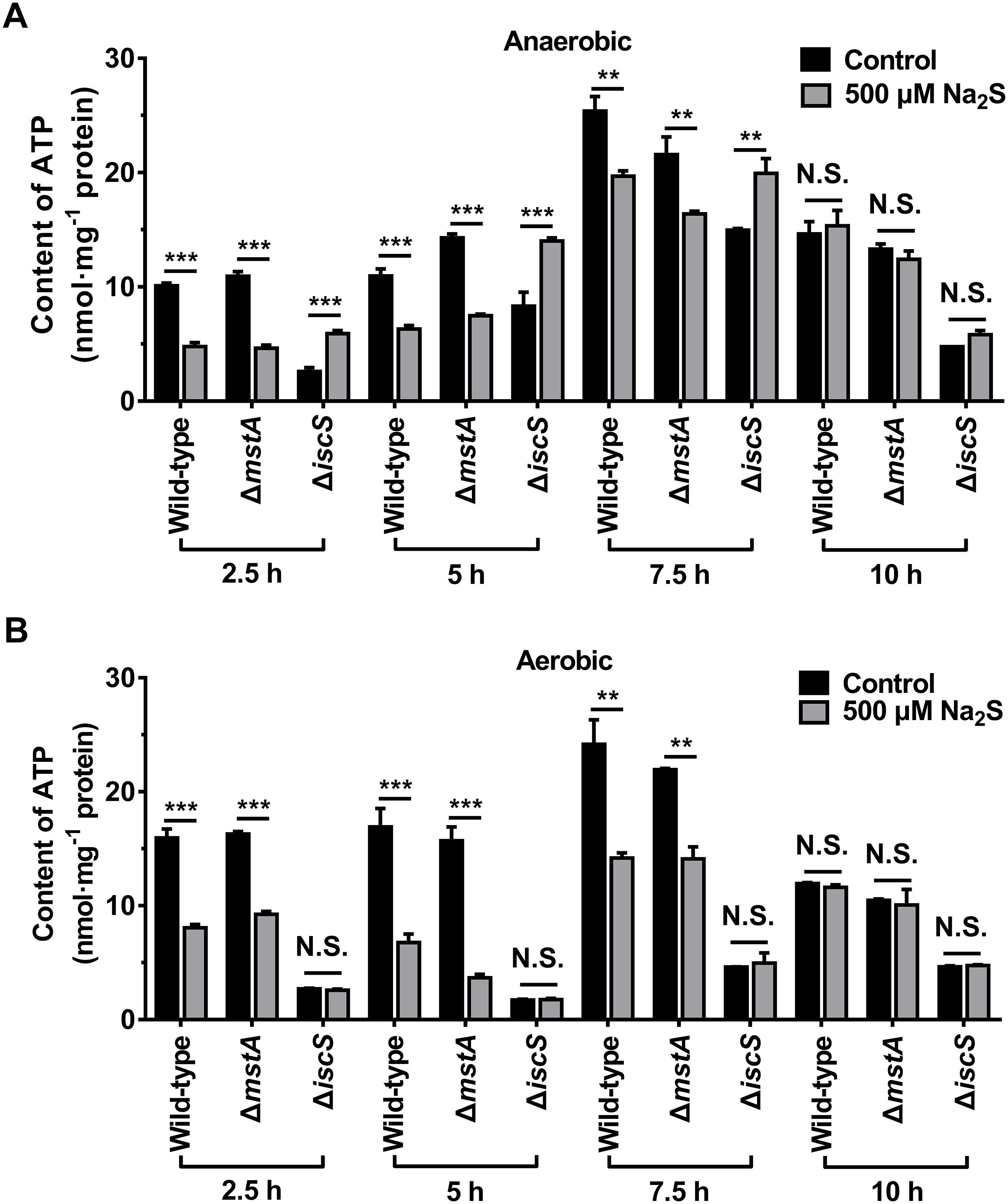
Figure 6. Exogenous H2S promotes ATP synthesis in the E. coli (ΔiscS) mutant strains in the logarithmic growth phase under anaerobic conditions. (A) The ATP content in cell lysates of E. coli under anaerobic conditions. (B) the ATP content in cell lysates of E. coli under aerobic conditions. Samples without Na2S treatment were used as control, and the results were normalized to the protein concentrations of the cell lysates. Each bar represents the mean ± SD of four independent experiments. ∗∗p < 0.01, ∗∗∗p < 0.001, N.S., not significant, versus the control group.
We next measured the ATP content in the wild-type and the mutant E. coli cells cultured under aerobic conditions. As shown in Figure 6B, The ATP content in the wild-type and the mutant E. coli (ΔmstA) cells was greatly decreased after the addition of 500 μM Na2S to the culture. Exogenous Na2S (500–2000 μM) significantly inhibited the growth of wild-type E. coli under aerobic conditions (Figures 4E,G). It is, therefore, not surprising that exogenous Na2S inhibited the biosynthesis of ATP in the wild-type and the mutant E. coli (ΔmstA) cells. Interestingly, unlike under anaerobic conditions, the ATP content in the mutant E. coli (ΔiscS) cells cultured under aerobic conditions was not significantly affected by the addition of 500 μM Na2S. These results indicated that hydrogen sulfide from IscS sustains cellular bioenergetics in E. coli under anaerobic conditions.
Finally, we explored the mechanism of hydrogen sulfide promoting ATP synthesis in the mutant E. coli (ΔiscS) strain under anaerobic conditions. A previous study indicated that incubation with NaHS (an H2S donor) markedly augmented the catalytic activity of glyceraldehyde-3-phosphate dehydrogenase (GAPDH) in both purified protein and HEK293 cells (Mustafa et al., 2009). As the amino acid sequence of E. coli GAPDH shared 79% identity with that of human beings, we speculated that S-sulfhydration of GAPDH in E. coli would stimulate the glycolytic pathway and enhance ATP synthesis under anaerobic conditions. Recombinant E. coli GAPDH was purified and protein purity was judged to be >95% by SDS-PAGE analysis (Supplementary Figure S9a). Recombinant GAPDH protein was S-sulfhydrated when incubated with Na2S combined with diamide as a strong thiol-specific oxidant. Unexpectedly, unlike GAPDH from mammalian cells, incubation with Na2S combined with diamide did not significantly increase the E. coli GAPDH activity (Supplementary Figure S9b). However, a recent study indicated that GAPDH was inactivated by S-sulfhydration in vitro, which is inconsistent with the study mentioned above (Jarosz et al., 2015). Further studies will be needed to elucidate the mechanism by which exogenous H2S enhances ATP synthesis in the mutant E. coli (ΔiscS) under anaerobic conditions.
Discussion
IscS is highly conserved in all living cells, from bacteria to humans, catalyzing the formation of a protein-bound persulfide and L-alanine using L-cysteine as a substrate (Lauhon et al., 2004). Obviously, a persulfide intermediate can produce either elemental sulfur or H2S depending on the redox state of the reaction medium. H2S production catalyzed by purified IscS greatly increased in the presence of DTT (Figures 3B–D). The activity of IscS was regulated under the different redox conditions, and the midpoint redox potential was determined to be −329 ± 1.6 mV. Additionally, in E. coli cells H2S production from IscS is regulated under oxidative and reductive stress (Figure 3E). Notably, the redox state of the bacterial cytoplasm is different under aerobic versus anaerobic conditions (Li et al., 2004). A recent study indicated that the intracellular redox potential undergoes reductive changes associated with the induction of hypoxia (Jiang et al., 2014). These results suggest that H2S generation by IscS would be regulated by the redox state of the bacterial cytoplasm under aerobic and anaerobic conditions, which is consistent with our observations in the present study.
IscS acts as a sulfur donor and is involved in the biogenesis of iron-sulfur clusters in the model bacterium E. coli. Iron-sulfur clusters are of great importance in the function of proteins involved in energy metabolism, including in electron transport in respiratory chain complexes, the Krebs cycle and photosynthesis (Rouault, 2012). Under oxygen-rich conditions, the Krebs cycle is a major energy-producing metabolic pathway, and a large amount of elemental sulfur generated by IscS is required for the assembly of iron-sulfur proteins. However, under oxygen-deficient conditions, we speculate that H2S production from IscS would promote cellular energy metabolism and ATP synthesis through an increase in substrate-level phosphorylation. Actually, exogenous Na2S (100–500 μM) significantly promoted the growth of the mutant E. coli (ΔiscS) in the logarithmic growth phase under anaerobic conditions, but not under aerobic conditions (Figure 4). Accordingly, the ATP content in the mutant E. coli (ΔiscS) increased ∼2.3-fold in the presence of 500 μM Na2S under anaerobic conditions, but was not altered under aerobic conditions (Figure 6). It should be noted, however, that elimination of iscS results in a viable organism that displays complex nutritional requirements and severely reduced activity of Fe-S cluster enzymes (Lauhon and Kambampati, 2000; Schwartz et al., 2000; Tokumoto and Takahashi, 2001; Lauhon et al., 2004). Exogenous H2S might promote the growth of the mutant E. coli (ΔiscS) by directly or indirectly rescuing these defects.
Additionally, in the present study it was observed that 500 μM Na2S is toxic to wild-type E. coli under both aerobic and anaerobic conditions, whereas it promotes the growth of the mutant E. coli (ΔiscS) under anaerobic conditions. The mechanism of the opposite effects of Na2S on the wild-type and the mutant E. coli (ΔiscS) is not known but could result from altered energy metabolism pathways. Previous studies showed that the toxicity of H2S has been attributed to its ability to inhibit cytochrome oxidase of the electron transport chain, resulting in the inhibition of ATP production and metabolic suppression (Cooper and Brown, 2008). In the present study, high concentrations of Na2S (500 μM) would inhibit cytochrome oxidase of wild-type E. coli, and consequently inhibited cell growth and ATP production (Korshunov et al., 2016). In the case of the mutant E. coli (ΔiscS), however, the function of electron transport in respiratory chain complexes was severely impaired due to defects in iron-sulfur cluster synthesis. Therefore, dysfunctional cytochrome oxidase or respiratory chain complexes may be insensitive to H2S treatment, resulting in enhanced H2S tolerance in the mutant E. coli (ΔiscS).
Previous studies demonstrated that elevated levels of H2S in mammalian mitochondria stimulates the production of ATP, which is based on the mechanism by which H2S donates electrons to the electron transport chain through H2S oxidation catalyzed by sulphide:quinone oxidoreductase (SQR) (Fu et al., 2012; Módis et al., 2016). Additionally, when sulfide levels rose, E. coli became strictly dependent upon cytochrome bd oxidase for continued respiration. The sulfide resistance of cytochrome bd oxidase is a key trait that permits respiration in low oxygen conditions (Forte et al., 2016; Korshunov et al., 2016). However, these mechanisms do not provide a reasonable explanation for the results obtained in the present study. The first reason is because sulfide:quinone oxidoreductase or one of its orthologs is not present in E. coli. Second, our results clearly indicated that exogenous H2S do not promote ATP synthesis in the wild-type and the mutant E. coli (ΔiscS) under aerobic conditions (Figure 6). Third, the cytochrome oxidase in E. coli catalyzes the transfer of electrons from reduced ubiquinone to molecular oxygen. This electron transfer contributes to the energy yield of respiration. However, molecular oxygen cannot be the terminal electron acceptor of E. coli respiratory chain under anaerobic conditions.
In summary, our results clearly demonstrated that IscS, not 3-MST, is the primary source of H2S in E. coli under anaerobic conditions, as evidenced by assays using chemical inhibitors and iscS, mstA, cyuA and sufS knockout strains. Hydrogen sulfide from IscS sustains cell growth and bioenergetics in E. coli under anaerobic conditions.
Data Availability Statement
All datasets generated/analyzed for this study are included in the manuscript/Supplementary Files.
Author Contributions
WN conceived and designed the experiments. JW, XG, HL, HQ, JQ, SY, and WN performed the experiments. WN, JW, and JS analyzed the data. JW and WN wrote the manuscript.
Funding
This study was funded by the National Natural Science Foundation of China (Nos. 31871145 and 31471086), the Key Science and Technology Program of Shaanxi Province, China (No. 2017GY-143), the Seed Foundation of Innovation and Creation for Graduate Students in Northwestern Polytechnical University (No. ZZ2019270), and the Fundamental Research Funds for the Central Universities of China (No. G2017KY0001).
Conflict of Interest
The authors declare that the research was conducted in the absence of any commercial or financial relationships that could be construed as a potential conflict of interest.
Supplementary Material
The Supplementary Material for this article can be found online at: https://www.frontiersin.org/articles/10.3389/fmicb.2019.02357/full#supplementary-material
References
An, X., Xiong, W., Yang, Y., Li, F., Zhou, X., Wang, Z., et al. (2012). A novel target of IscS in Escherichia coli: participating in DNA phosphorothioation. PLoS One 7:e51265. doi: 10.1371/journal.pone.0051265
Chen, B., Li, W., Lv, C., Zhao, M., Jin, H., Jin, H., et al. (2013). Fluorescent probe for highly selective and sensitive detection of hydrogen sulfide in living cells and cardiac tissues. Analyst 138, 946–951. doi: 10.1039/C2AN36113B
Chen, S., Chen, Z., Ren, W., and Ai, H. (2012). Reaction-based genetically encoded fluorescent hydrogen sulfide sensors. J. Am. Chem. Soc. 134, 9589–9592. doi: 10.1021/ja303261d
Cooper, C. E., and Brown, G. C. (2008). The inhibition of mitochondrial cytochrome oxidase by the gases carbon monoxide, nitric oxide, hydrogen cyanide and hydrogen sulfide: chemical mechanism and physiological significance. J. Bioenerg. Biomembr. 40, 533–539. doi: 10.1007/s10863-008-9166-6
Dunkle, J. A., Bruno, M. R., Outten, F. W., and Frantom, P. A. (2019). Structural evidence for dimer-interface-driven regulation of the type II cysteine desulfurase, SufS. Biochemistry 58, 687–696. doi: 10.1021/acs.biochem.8b01122
Forte, E., Borisov, V. B., Falabella, M., Colaço, H. G., Tinajero-Trejo, M., Poole, R. K., et al. (2016). The terminal oxidase cytochrome bd promotes sulfide-resistant bacterial respiration and growth. Sci. Rep. 6:23788. doi: 10.1038/srep23788
Fu, M., Zhang, W., Wu, L., Yang, G., Li, H., and Wang, R. (2012). Hydrogen sulfide (H2S) metabolism in mitochondria and its regulatory role in energy production. Proc. Natl. Acad. Sci. U.S.A. 109, 2943–2948. doi: 10.1073/pnas.1115634109
Hidese, R., Mihara, H., and Esaki, N. (2011). Bacterial cysteine desulfurases: versatile key players in biosynthetic pathways of sulfur-containing biofactors. Appl. Microbiol. Biotechnol. 91, 47–61. doi: 10.1007/s00253-011-3336-x
Jarosz, A. P., Wei, W. L., Gauld, J. W., Auld, J., Özcan, F., Aslan, M., et al. (2015). Glyceraldehyde 3-phosphate dehydrogenase (GAPDH) is inactivated by S-sulfuration in vitro. Free Radic. Biol. Med. 89, 512–521. doi: 10.1016/j.freeradbiomed.2015.09.007
Jiang, J., Auchinvole, C., Fisher, K., and Campbell, C. J. (2014). Quantitative measurement of redox potential in hypoxic cells using SERS nanosensors. Nanoscale 6, 12104–12110. doi: 10.1039/c4nr01263a
Jiang, Y., Chen, B., Duan, C., Sun, B., Yang, J., and Yang, S. (2015). Multigene editing in the Escherichia coli genome via the CRISPR-Cas9 system. Appl. Environ. Microbiol. 81, 2506–2514. doi: 10.1128/AEM.04023-14
Kabil, O., and Banerjee, R. (2014). Enzymology of H2S biogenesis, decay and signaling. Antioxid. Redox Signal. 20, 770–782. doi: 10.1089/ars.2013.5339
Kim, D., Singh, H., Dai, Y., Dong, G., Busenlehner, L. S., Outten, F. W., et al. (2018). Changes in protein dynamics in Escherichia coli SufS reveal a possible conserved regulatory mechanism in type ? cysteine desulfurase systems. Biochemistry 57, 5210–5217. doi: 10.1021/acs.biochem.7b01275
Kimura, H. (2014). Production and physiological effects of hydrogen sulfide. Antioxid. Redox Signal. 20, 783–793. doi: 10.1089/ars.2013.5309
Korshunov, S., Imlay, K. R., and Imlay, J. A. (2016). The cytochrome bd oxidase of Escherichia coli prevents respiratory inhibition by endogenous and exogenous hydrogen sulfide. Mol. Microbiol. 101, 62–77. doi: 10.1111/mmi.13372
Lauhon, C. T., and Kambampati, R. (2000). The iscS gene in Escherichia coli is required for the biosynthesis of 4-thiouridine, thiamin, and NAD. J. Biol. Chem. 275, 20096–20103. doi: 10.1074/jbc.M002680200
Lauhon, C. T., Skovran, E., Urbina, H. D., Downs, D. M., and Vickery, L. E. (2004). Substitutions in an active site loop of Escherichia coli IscS result in specific defects in Fe-S cluster and thionucleoside biosynthesis in vivo. J. Biol. Chem. 279, 19551–19558. doi: 10.1074/jbc.M401261200
Li, K., Pasternak, C., Härtig, E., Haberzettl, K., Maxwell, A., and Klug, G. (2004). Thioredoxin can influence gene expression by affecting gyrase activity. Nucleic Acids Res. 32, 4563–4575. doi: 10.1093/nar/gkh794
Li, K., Tong, W. H., Hughes, R. M., and Rouault, T. A. (2006). Roles of the mammalian cytosolic cysteine desulfurase, ISCS, and scaffold protein, ISCU, in iron-sulfur cluster assembly. J. Biol. Chem. 281, 12344–12351. doi: 10.1074/jbc.M600582200
Loddeke, M., Schneider, B., Oguri, T., Mehta, I., Xuan, Z., Reitzer, L., et al. (2017). Anaerobic cysteine degradation and potential metabolic coordination in Salmonella enterica and Escherichia coli. J. Bacteriol. 199:e117. doi: 10.1128/JB.00117-17
Marelja, Z., Stöcklein, W., Nimtz, M., and Leimkühler, S. (2008). A novel role for human Nfs1 in the cytoplasm: Nfs1 acts as a sulfur donor for MOCS3, a protein involved in molybdenum cofactor biosynthesis. J. Biol. Chem. 283, 25178–25185. doi: 10.1074/jbc.M804064200
Martin, R. B. (1997). Disadvantages of double reciprocal plots. J. Chem. Educ. 74, 1238–1240. doi: 10.1021/ed074p1238
Mihara, H., and Esaki, N. (2002). Bacterial cysteine desulfurases: their function and mechanisms. Appl. Microbiol. Biotechnol. 60, 12–23. doi: 10.1007/s00253-002-1107-4
Mironov, A., Seregina, T., Nagornykh, M., Luhachack, L. G., Korolkova, N., Lopes, L. E., et al. (2017). Mechanism of H2S-mediated protection against oxidative stress in Escherichia coli. Proc. Natl. Acad. Sci. U.S.A. 114, 6022–6027. doi: 10.1073/pnas.1703576114
Módis, K., Ju, Y., Ahmad, A., Untereiner, A. A., Altaany, Z., Wu, L., et al. (2016). S-Sulfhydration of ATP synthase by hydrogen sulfide stimulates mitochondrial bioenergetics. Pharmacol. Res. 113, 116–124. doi: 10.1016/j.phrs.2016.08.023
Mustafa, A. K., Gadalla, M. M., Sen, N., Kim, S., Mu, W., Gazi, S. K., et al. (2009). H2S signals through protein S-sulfhydration. Sci. Signal. 2:ra72. doi: 10.1126/scisignal.2000464
Niu, W., Chen, F., Wang, J., Qian, J., and Yan, S. (2018a). Antitumor effect of sikokianin C, a selective cystathionine β-synthase inhibitor, against human colon cancer in vitro and in vivo. Medchemcomm 9, 113–120. doi: 10.1039/c7md00484b
Niu, W., Wang, J., Qian, J., Wang, M., Wu, P., Chen, F., et al. (2018b). Allosteric control of human cystathionine β-synthase activity by a redox active disulfide bond. J. Biol. Chem. 293, 2523–2533. doi: 10.1074/jbc.RA117.000103
Rouault, T. (2012). Biogenesis of iron-sulfur clusters in mammalian cells: new insights and relevance to human disease. Dis. Model. Mech. 5, 155–164. doi: 10.1242/dmm.009019
Schwartz, C. J., Djaman, O., Imlay, J. A., and Kiley, P. J. (2000). The cysteine desulfurase, IscS, has a major role in in vivo Fe-S cluster formation in Escherichia coli. Proc. Natl. Acad. Sci. U.S.A. 97, 9009–9014. doi: 10.1073/pnas.160261497
Scuffi, D., Álvarez, C., Laspina, N., Gotor, C., Lamattina, L., and García-Mata, C. (2014). Hydrogen sulfide generated by L-cysteine desulfhydrase acts upstream of nitric oxide to modulate Abscisic acid-dependent stomatal closure. Plant Physiol. 166, 2065–2076. doi: 10.1104/pp.114.245373
Shatalin, K., Shatalina, E., Mironov, A., and Nudler, E. (2011). H2S: a universal defense against antibiotics in bacteria. Science 334, 986–990. doi: 10.1126/science.1209855
Tokumoto, U., and Takahashi, Y. (2001). Genetic analysis of the isc operon in Escherichia coli involved in the biogenesis of cellular iron-sulfur proteins. J. Biochem. 130, 63–71. doi: 10.1093/oxfordjournals.jbchem.a002963
Urbina, H. D., Silberg, J. J., Hoff, K. G., and Vickery, L. E. (2001). Transfer of sulfur from IscS to IscU during Fe/S cluster assembly. J. Biol. Chem. 276, 44521–44526. doi: 10.1074/jbc.M106907200
Wing, D. A., and Baskin, S. I. (1992). Modifiers of mercaptopyruvate sulfurtransferase catalyzed conversion of cyanide to thiocyanate in vitro. J. Biochem. Toxicol. 7, 65–72. doi: 10.1002/jbt.2570070203
Yarian, C. S., Toroser, D., and Sohal, R. S. (2006). Aconitase is the main functional target of aging in the citric acid cycle of kidney mitochondria from mice. Mech. Ageing Dev. 127, 79–84. doi: 10.1016/j.mad.2005.09.028
Zhang, X., Zuo, X., Yang, B., Li, Z., Xue, Y., Zhou, Y., et al. (2014). MicroRNA directly enhances mitochondrial translation during muscle differentiation. Cell 158, 607–619. doi: 10.1016/j.cell.2014.05.047
Zheng, L., White, R. H., Cash, V. L., and Dean, D. R. (1994). Mechanism for the desulfurization of L-cysteine catalyzed by the nifS gene product. Biochemistry 33, 4714–4720. doi: 10.1021/bi00181a031
Keywords: hydrogen sulfide, cysteine desulfurase, bioenergetics, anaerobic conditions, 3-mercaptopyruvate sulfurtransferase
Citation: Wang J, Guo X, Li H, Qi H, Qian J, Yan S, Shi J and Niu W (2019) Hydrogen Sulfide From Cysteine Desulfurase, Not 3-Mercaptopyruvate Sulfurtransferase, Contributes to Sustaining Cell Growth and Bioenergetics in E. coli Under Anaerobic Conditions. Front. Microbiol. 10:2357. doi: 10.3389/fmicb.2019.02357
Received: 07 July 2019; Accepted: 27 September 2019;
Published: 11 October 2019.
Edited by:
Patricia Coutinho Dos Santos, Wake Forest University, United StatesReviewed by:
Timothy J. Larson, Virginia Tech, United StatesSilke Leimkuehler, University of Potsdam, Germany
Copyright © 2019 Wang, Guo, Li, Qi, Qian, Yan, Shi and Niu. This is an open-access article distributed under the terms of the Creative Commons Attribution License (CC BY). The use, distribution or reproduction in other forums is permitted, provided the original author(s) and the copyright owner(s) are credited and that the original publication in this journal is cited, in accordance with accepted academic practice. No use, distribution or reproduction is permitted which does not comply with these terms.
*Correspondence: Weining Niu, bml1d2VpbmluZ0Bud3B1LmVkdS5jbg==