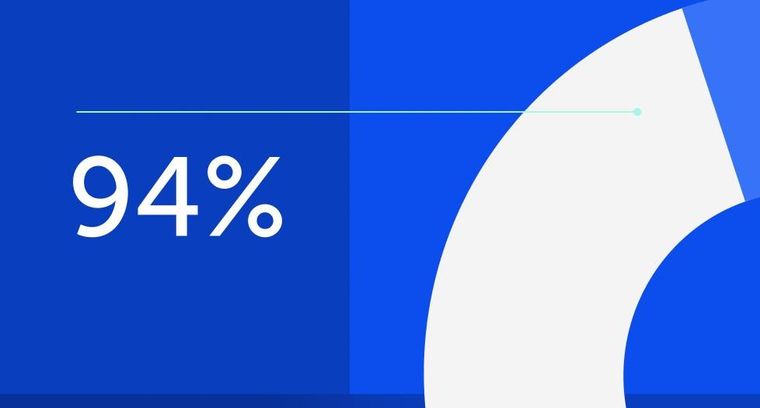
94% of researchers rate our articles as excellent or good
Learn more about the work of our research integrity team to safeguard the quality of each article we publish.
Find out more
REVIEW article
Front. Microbiol., 24 October 2019
Sec. Infectious Agents and Disease
Volume 10 - 2019 | https://doi.org/10.3389/fmicb.2019.02351
Elongation factor thermal unstable Tu (EF-Tu) is a G protein that catalyzes the binding of aminoacyl-tRNA to the A-site of the ribosome inside living cells. Structural and biochemical studies have described the complex interactions needed to effect canonical function. However, EF-Tu has evolved the capacity to execute diverse functions on the extracellular surface of both eukaryote and prokaryote cells. EF-Tu can traffic to, and is retained on, cell surfaces where can interact with membrane receptors and with extracellular matrix on the surface of plant and animal cells. Our structural studies indicate that short linear motifs (SLiMs) in surface exposed, non-conserved regions of the molecule may play a key role in the moonlighting functions ascribed to this ancient, highly abundant protein. Here we explore the diverse moonlighting functions relating to pathogenesis of EF-Tu in bacteria and examine putative SLiMs on surface-exposed regions of the molecule.
Elongation Factor Thermo Unstable (EF-Tu) is one the most abundant proteins found in bacteria, comprising up to 6% of the total protein expressed in Escherichia coli (Furano, 1975) and as high as 10% of the total protein expressed in the genome reduced pathogen Mycoplasma pneumoniae (Dallo et al., 2002). The primary, canonical function of EF-Tu is to transport aminoacylated tRNAs to the ribosome (Sprinzl, 1994). Ef-Tu has been a therapeutic target for antibiotics (elfamycins) since the 1970s (Wolf et al., 1974; Prezioso et al., 2017). However, current issues with elfamycins’ poor pharmacokinetics and solubility has prevented their commercialization as therapeutic agents (Prezioso et al., 2017).
Diverse functions have been ascribed to EF-Tu many of which include important virulence traits in Gram positive and Gram-negative pathogenic bacteria. To effect alternate virulence-associated functions, including adhesion to host extracellular matrix components, EF-Tu must gain access and be retained on the extracellular surface. This poses a challenge as signal secretion motifs are absent in this highly structured protein, and motifs required for binding diverse host cell surface receptor and matrix molecules must evolve without jeopardizing structural constraints needed to execute canonical function as a G protein. Here we refer to secondary functions as “moonlighting” functions. The concept of protein moonlighting is well established in eukaryotes (Jeffery, 1999; Huberts and van der Klei, 2010; Petit et al., 2014; Min et al., 2016; Yoon et al., 2018), and is rapidly gaining traction in prokaryotes (Henderson and Martin, 2011, 2013; Wang et al., 2013b; Kainulainen and Korhonen, 2014; Jeffery, 2018; Ebner and Götz, 2019) indicating that it is an ancient and evolutionally conserved phenomenon. Although EF-Tu executes various functions in eukaryotes, a review of the moonlighting roles of EF-Tu in bacteria is lacking. Therefore, this review has a focus to discuss the ever-expanding moonlighting roles of EF-Tu in prokaryotes, and how these roles relate to pathogenesis.
Elongation factors (Table 1) in bacteria (e.g., EF-Tu also known as EF1A) and in eukaryotes (e.g., the eukaryotic Elongation Factor 1 Complex [eEF1A]) all have the same primary and critical function to shuttle aminoacylated tRNAs to the ribosome during protein translation. A codon–anticodon system ensures that the correct amino acid is added to the growing protein chain, a process that consumes guanosine triphosphate (GTP) prior to releasing the elongation factor from the aminoacyl tRNA. However, bacteria and eukaryotes differ in the mechanism by which they recharge the elongation factor/guanosine diphosphate (GDP) complex. This recharging function is executed by the Elongation Factor Thermo stable (EF-Ts) in prokaryotes and by eukaryotic Elongation Factor 1B (eEF1B) in eukaryotes (Cacan et al., 2013) (Figure 1).
Figure 1. The canonical role of EF-Tu in translation. Structures sourced from protein databank (PDB), accession numbers 1DG1, 5OPD, 1B23, 4PC7, 1EFT, 1EFT. EF-Ts monomer structure obtained using Phyre2.
EF-Tu is comprised of three domains known as domains i, ii and iii which have evolved a high degree of molecular flexibility. To perform its canonical function, EF-Tu must form a functional binding pocket for an aminoacyl-tRNA, and to achieve this, domain i must become aligned more closely to domains ii and iii (i.e., they must move by around 90°) (Kjeldgaard et al., 1993). The extent of intramolecular movement needed to accommodate the aminoacyl-tRNA is about one third of the protein’s total diameter, indicating how significant this conformational change is (Sprinzl, 1994). Once the incoming aminoacyl-tRNA has docked with the mRNA, GTPase activity induces a reverse conformational change enabling the release of EF-Tu from the ribosome (Polekhina et al., 1996). The structural and functional constraints needed to execute these critical molecular interactions ensure that key domains within EF-Tu evolve slowly compared to molecules that perform their functions on the cell surface, where they face constant challenge from the host’s immunological defenses and undergo diversifying selection. As such, EF-Tu is considered to be an ancient molecule that is comprised of domains that are highly conserved in phylogenetically diverse prokaryotes (Filer and Furano, 1980). This sequence conservation extends to EF-Tu homologs in eukaryotes, which have also evolved a similar overall protein synthesis pathway (Ejiri, 2002).
In bacteria, EF-Tu is encoded by the tuf gene. tuf has a highly conserved genomic location and amino acid sequence, and has been used in the construction of phylogenetic trees for species discrimination (Iwabe et al., 1989; Baldauf et al., 1996; Mignard and Flandrois, 2007; Shin et al., 2009; Li et al., 2012; Caamano-Antelo et al., 2015). Amongst different bacterial species the EF-Tu sequences have less than 30% sequence divergence (Lathe and Bork, 2001). Low G + C Gram positive bacteria carry only a single copy of tuf (Ke et al., 2000). In contrast, many enteric bacteria have two copies (tufA and tufB) while three tuf-like genes have been identified in Streptomyces ramocissimus (Filer and Furano, 1981; Vijgenboom et al., 1994). In species with two copies of the gene, the two genes differ by less than 1.4%, based on nucleotide comparison (Lathe and Bork, 2001). In some bacteria with two copies of tuf, deletion of one copy of the gene is not lethal to the bacterium (Hughes, 1990; Zuurmond et al., 1999). It has been postulated that a second copy of this gene (which is mainly conserved in Gram negative bacteria) evolved before the branching of eubacteria (Lathe and Bork, 2001). The cause for the intermittent presence of the second copy of tuf within eubacteria has been debated. It has been proposed that the second copy arose by lateral gene transfer, at least within Enterococci (Ke et al., 2000), whilst others argue that lateral gene transfer is unlikely in translation factors and attribute the discontinuous observation of a second tuf gene to the theory that it had been randomly lost in some lineages (Lathe and Bork, 2001).
Eukaryotes have two isoforms of EF-Tu known as eEF1A1 and eEF1A2 (Table 1), with each sharing 96% amino acid similarity (Abbas et al., 2015). Both isoforms are also highly expressed representing 1–11% of the total protein expressed (Slobin, 1980; Abbas et al., 2015). Some cells express just one of the eEF1A isoforms, while both are expressed after muscle trauma and in some tumor cell types (Bosutti et al., 2007; Abbas et al., 2015). The number of genes encoding eEF1A varies widely within eukaryotes, from ten in maize to four in rice (Ejiri, 2002).
There is now overwhelming evidence that proteins with canonical functions in the bacterial cytosol also perform important tasks on the bacterial cell surface (Sanchez et al., 2008; Kainulainen and Korhonen, 2014; Ebner and Götz, 2019). EF-Tu features prominently in many of these studies. Many moonlighting proteins are ancient, highly expressed enzymes, or are proteins that are perform essential roles in glycolysis, respiration and respond to stress (Henderson and Martin, 2011). There is evidence that only a subset of cytosolic proteins can traffic onto the cell because other highly expressed cytosolic proteins are not observed on the cell surface or in extracellular secretions (Vanden Bergh et al., 2013). Mass spectrometry studies have been instrumental in revealing the identities of surface accessible proteins that are not predicted to reside on the cell surface that have canonical functions in the bacterial cytosol (Jeffery, 2005; Robinson et al., 2013; Jarocki et al., 2015; Tacchi et al., 2016; Wang and Jeffery, 2016; Widjaja et al., 2017). The presence of surface-associated moonlighting proteins has been confirmed using florescence and electron microscopy (Bergmann et al., 2001; Candela et al., 2010; Yamaguchi et al., 2010; Robinson et al., 2013; Grundel et al., 2015; Jarocki et al., 2015). It is notable that purified, soluble moonlighting protein fails to associate with the surface when exogenously incubated with bacterial cells (Saad et al., 2009) suggesting that posttranslational modification(s) that occur in the host bacteria and/or passage through the cell membrane may be important events in a proteins ability to moonlight on the cell surface. Another unusual feature of protein moonlighting is that not all strains belonging to the same species present moonlighting proteins on their cell surface. For example, only a subset of pathogenic E. coli express surface GAPDH which binds host molecules (Egea et al., 2007). Finally, it is now known that moonlighting proteins are processed on the surface of bacterial pathogens. Processing is expected to increase protein disorder and alter function compared to the full length proteoform (Tacchi et al., 2016). Here we present key studies that describe the salient features that define the diverse moonlighting functions of EF-Tu related to pathogenesis (Figure 2 and Table 2).
Figure 2. Moonlighting functions of prokaryote EF-Tu. (A) (1) EF-Tu binds immune system regulators such as Factor H, substance P and plasminogen (and enhancing its conversion to plasmin), increasing virulence and immune system evasion. (2) EF-Tu stimulates both host innate and humoral immune responses. (3) Antibodies against EF-Tu decrease bacterial load and offer at least partial protection against some bacterial infections. (B) (4) EF-Tu binding to fibronectin facilitates invasion into host cells. EF-Tu also binds to other extracellular matrix (ECM) proteins, such as glycosaminoglycans, facilitating adhesion. (C) (5) EF-Tu binds MreB and facilitates production of MreB filaments that regulate cell shape. (D) (6) EF-Tu undergoes proteolytic processing and EF-Tu fragments also bind ECM proteins. Furthermore, these fragments may act as molecular decoys to help evade immune detection.
EF-Tu was first described as having a moonlighting function on the cell surface of M. pneumoniae (Dallo et al., 2002). As EF-Tu has now been found on the surface of a wide range of prokaryotes (Table 3), the potential mechanisms behind its extracellular locale shall be summarized here.
Typically, newly synthesized proteins destined for the cell surface possess either signal peptides or signal motifs that are recognized by transport machinery and translocated through the cytoplasmic membrane (Green and Mecsas, 2016). However, conventionally cytosolic proteins lacking signal sequences, like EF-Tu, have also been identified extracellularly. These proteins, termed non-classically secreted proteins (Wang et al., 2016), can be differentiated from other cytosolic proteins by assessing properties such as amino acid composition and structurally disordered regions (Bendtsen et al., 2005). Indeed, EF-Tu was one protein used to construct the feature-based non-classically secreted protein prediction software SecretomeP (Bendtsen et al., 2005). However, the actual mechanisms behind non-classical protein secretion remain a topic for debate. While there are translocation systems that do not require signal peptides, such as the Holin–Antiholin system, ABC transporters, and a type seven secretion system in Gram-positive bacteria (Götz et al., 2015), these only account for a small portion of non-classically secreted proteins (Wang et al., 2016). Therefore, the presence of cytosolic proteins in extracellular locations is often linked with cell lysis.
There is evidence supporting cytosolic protein excretion through cell lysis, and there is evidence supporting excretion through alternative secretion pathways (reviewed in Wang et al., 2013a). In addition to specific secretion pathways, cytosolic protein excretion can also occur through compromised membrane integrity, translational and osmotic stress, the protein’s biochemical and structural properties, and via membrane vesicles (MVs) (Outer Membrane Vesicles [OMVs] in Gram-negative bacteria) (reviewed in Ebner and Götz, 2019).
Multiple mechanism may contribute to the surface location of EF-Tu; however, there are substantial reports of MVs carrying EF-Tu. Bacterial MVs are nanoparticles produced through processes such as membrane blebbing and endolysin-triggered cell death and contain various membrane and cytosolic proteins, as well as lipopolysaccharides, peptidoglycan and DNA (Turnbull et al., 2016; Toyofuku et al., 2019). MVs are involved in diverse biological processes, including virulence, biofilm development, quorum sensing, horizontal gene transfer, and exportation of cellular components (Toyofuku et al., 2019). EF-Tu is present within MVs derived from Gram-positive bacteria including Listeria monocytogenes (Coelho et al., 2019), Mycobacterium bovis, Mycobacterium smegmatis, Mycobacterium tuberculosis (Prados-Rosales et al., 2011), Staphylococcus aureus (Lee et al., 2009; Wang et al., 2018), Streptococcus agalactiae (Surve et al., 2016), Streptococcus pnuemoniae (Olaya-Abril et al., 2014), and Streptococcus pyogenes (Resch et al., 2016); Gram-negative bacteria including Acinetobacter baumannii (Kwon et al., 2009), Bacteroides fragilis (Zakharzhevskaya et al., 2017), Cronobacter sakazakii (Alzahrani et al., 2015), Escherichia coli (Lee et al., 2007), Francisella novicida (Pierson et al., 2011), Haemophilus influenzae (Sharpe et al., 2011), Klebsiella pneumoniae (Lee et al., 2012), Neisseria gonorrhoeae (Pérez-Cruz et al., 2015), Neisseria meningitidis (Vipond et al., 2006), and Pseudomonas aeruginosa (Choi et al., 2011); and in six Mycoplasma species (Gaurivaud et al., 2018). Indeed, EF-Tu was reported as one of the most abundant protein in some of these studies (Lee et al., 2009; Pérez-Cruz et al., 2015; Gaurivaud et al., 2018). Interestingly, several MVs that contain EF-Tu have been reported to increase virulence (Surve et al., 2016), modulate immune responses (Prados-Rosales et al., 2011; Sharpe et al., 2011; Alzahrani et al., 2015), and offer protection to infection via immunization (Vipond et al., 2006; Pierson et al., 2011; Olaya-Abril et al., 2014). As the number of MV-encapsulated proteins varied dramatically within this subset of studies, ranging from 8 in S. agalactiae to 416 in F. novicida, it is not possible to determine the exact role EF-Tu plays in these processes in most instances. However, in the case of S. pneumoniae MVs, which were shown to have high immunogenic capacity and induce protective responses in mice, EF-Tu was one of 15/161 MV proteins that were immunogenic and one of two proteins from which antibodies were generated against in immunized mice (Olaya-Abril et al., 2014).
Antibodies against EF-Tu have also been detected in a range of natural infections, including those caused by Mycoplasma hyopneumoniae (Pinto et al., 2007), Chlamydia trachomatis (Sanchez-Campillo et al., 1999) and K. pneumonia (Liu et al., 2014). Recombinant EF-Tu (rEF-Tu) from Mycoplasma ovipneumoniae induces an immune response in mice, increasing levels of IgG, TNF-α, IFN-γ, IL-12(p70), IL-4, IL-5, and IL-6. Sera from mice immunized with rEF-Tu also reduced M. ovipneumoniae growth (Jiang et al., 2016). In Mycoplasma fermentans, EF-Tu interacts specifically with the C-terminal 34 amino acids of CD21 in human B lymphoma cells (Balbo et al., 2005). CD21 receptors on the B cells enable the complement system to influence B-cell activation and maturation. The implication is that Mycoplasma species are involved in malignancies (reviewed in Vande Voorde et al., 2014) and the interaction between EF-Tu and CD21 may be significant in this regard. EF-Tu from L. monocytogenes is the main activator of host dendritic cells (DC) (Mirzaei et al., 2016), antigen presenting cells (APCs) that play a key role in host immune modulation. Activation of DCs is achieved when L. monocytogenes interacts with the pattern recognition receptors (PRRs) on the surface of DCs (Mirzaei et al., 2016). These PRRs recognize bacterial proteins known as PAMPS (pathogen-associated molecular pattern). In L. monocytogenes EF-Tu was identified as a potent immuno-stimulatory effector in this process indicating that EF-Tu is a candidate for DC maturation-based therapies (Mirzaei et al., 2016).
Recombinant EF-Tu (rEF-Tu) has recently shown promising results as a vaccine candidate against bacterial pathogens. Immunization with rEF-Tu elicited both Th1 and Th2-type responses against Streptococcus suis and anti-rEF-Tu sera reduced viable load detection in porcine blood (Feng et al., 2018). Mice immunized with rEF-Tu demonstrated significant protection against lethal challenges with S. pneumoniae and increased cytokine, IgG1 and IgG2a, and CD4+ T-cell production (Nagai et al., 2019). rEF-Tu mediated protection against S. pneumoniae has also been demonstrated in fish (Yang et al., 2018), and partial protection against H. influenzae was achieved in mice (Thofte et al., 2018).
Besides stimulating an immune response in mammals (Figure 2A), EF-Tu is also a recognized PAMP in plants (Kunze et al., 2004; Furukawa et al., 2014). EF-Tu is secreted by via unknown mechanisms in soil dwelling, plant–pathogenic bacteria and is recognized by membrane-associated PRRs found on the extracellular surface of root epithelial cells in different plant species. The interaction between PRRs and PAMPS identifies the bacteria as an infectious threat, triggering a signal transduction cascade, that elicits an innate immune response (Zipfel, 2008) that includes the production of reactive-oxygen species and programed cell death (Zipfel, 2008).
Both monocots and dicots use EF-Tu to notify their immune system of an infection. There is however, another level of sophistication to this interaction (Furukawa et al., 2014). Different plant species are known to have evolved recognition mechanisms in their respective PRRs that interact with different regions in the EF-Tu molecule. Rice PRRs recognize the amino acids (aa) 175-225 of EF-Tu, termed EFa50, from the plant pathogenic bacteria Acidovorax citrulli (formerly Acidovorax avenae) (Furukawa et al., 2014) whereas Arabidopsis thaliana recognizes the first 18 aa of EF-Tu from E. coli (termed elf18) (Kunze et al., 2004). Although elf18 does not illicit a response to this pathogen in rice plants, engineering the Arabidopsis PRR for elf18 into the rice plant enabled rice to recognize elf18 and respond by increasing resistance to bacterial attack (Lu et al., 2015). This proof-of-concept experiment demonstrated that PRRs can to be engineered into the genomes of different crop species and may be beneficial to the farming and food production industry. Transfer of PRRs has already been demonstrated on food crops such as tomatoes and wheat (Lacombe et al., 2010; Schoonbeek et al., 2015). Earlier structural studies of EF-Tu suggested that the first 12 amino acids of EF-Tu are exposed on the surface and the dodecapeptide can act as a competitive inhibitor of the elf18 elicitor (Kunze et al., 2004). The first 12aa of EF-Tu can also suppress the apoptotic response in plant cells allowing bacterial pathogens sufficient time and nutrient resources to colonize and replicate within plant cells (Igarashi et al., 2013). elf18 and EFa50 do not seem to have similar properties, but more recent structural studies have shown that they do appear to be (at least partially) surface exposed on the EF-Tu molecule (Furukawa et al., 2014). These data indicate that these two PAMPS can interact with PRRs on plant cell surfaces.
EF-Tu has also been shown to bind selectively to neuropeptide hormone substance P (SP) (Mijouin et al., 2013; N’Diaye et al., 2016). SP belongs to the tachykinin family of neuropeptides released by nerve and inflammatory cells (Datar et al., 2004) and is linked to many inflammatory diseases because it binds to the neurokinin 1 receptor (NK-1R) which stimulates pro-inflammatory responses (O’Connor et al., 2004). The inability to trigger NK-1R decreases bacterial clearance and increases death rates in mouse models of infection (Verdrengh and Tarkowski, 2008). SP and/or NK-1R have been linked to disease caused by infectious agents (Douglas et al., 2001; Schwartz et al., 2013), autoimmune disorders (Mantyh et al., 1988), psychological disturbances (Fehder et al., 1997; Herpfer and Lieb, 2005; McLean, 2005; Ebner and Singewald, 2006; Carpenter et al., 2008), cancer (Esteban et al., 2006), atopic dermatitis (Toyoda et al., 2002), and cell proliferation (Goode et al., 2003). EF-Tu from S. aureus, S. epidermidis, and B. cereus has been shown to bind SP, with an associated increase in virulence and biofilm formation (Mijouin et al., 2013; N’Diaye et al., 2016).
rEF-Tu derived from Lactobacillus johnsonii triggers a pro-inflammatory response in HT29 cells and increased IL-8 secretion in the presence of CD14 (Granato et al., 2004). IL-8 is known to increase levels of calcium ions within the cell in which it is expressed (Tuschil et al., 1992; Schorr et al., 1999). In Bacillus subtilis EF-Tu is a calcium binding protein (Dominguez et al., 2009). However, the link between calcium and EF-Tu in prokaryotes remains tenuous and further studies are needed to investigate the voracity of this association and its implication in the inflammatory response.
Adhesion to host cells and molecules is fundamental to pathogenesis in many bacterial species as it facilitates colonization, invasion, and host immune subversion (Stones and Krachler, 2016). In many instances, moonlighting functions for EF-Tu are associated with a role in adherence to a range of host molecules and host cells (Figure 2B). This does not appear to be a trend in specific phylogenetic groups of prokaryotes, as it extends through a range of bacteria. EF-Tu resides on the surface of Francisella tularensis where it binds to the RGG domain of nucleolin on the surface of the human monocytic cell line THP-1 (Barel et al., 2008). The HB-19 pseudopeptide irreversibly binds to the RGG domain in the C-terminus of nucleolin (Nisole et al., 1999, 2002) and effectively blocks attachment of Francisella tularensis (Barel et al., 2008). Consistent with these experiments, EF-Tu and a 32 kDa cleavage fragment of EF-Tu were recovered during affinity chromatography pull-down experiments using nucleolin as bait (Barel et al., 2008). It is notable that cleavage fragments of EF-Tu have been previously described in the cytoplasm and membrane fraction of L. monocytogenes (Archambaud et al., 2005) and more recently on the extracellular surfaces of S. aureus, Mycoplasma hyopneumoniae and M. pneumoniae (Widjaja et al., 2015, 2017). Despite this finding, data describing the cleavage products in L. monocytogenes has not been reported but is an interesting observation nonetheless that warrants further investigation.
Fibronectin (Fn) is a key component of the extracellular matrix. It is a glycoprotein that binds to integrins embedded in eukaryote cell membranes and provides support and anchors cells to substrata. Many bacterial pathogens and commensals express adhesins that bind Fn and these interactions can trigger cytoskeletal rearrangements that promote host cell invasion (Massey et al., 2001; Deutscher et al., 2010; Seymour et al., 2010, 2012; Henderson et al., 2011; Bogema et al., 2012; Raymond et al., 2015, 2018). Interestingly, Fn also plays a role as a signaling molecule so, binding Fn may serve other functions for the bacteria, compounding their infectivity (Sandig et al., 2009). Indeed, many bacteria have a repertoire of dedicated, secreted adhesins that target Fn (Henderson et al., 2011).
EF-Tu localizes to both the outer membrane (OM) and outer membrane vesicles (OMV) of A. baumannii and binds to DsbA (Premkumar et al., 2014), a protein important in protein folding and maturation (Heras et al., 2009). In its external location in A. baumannii EF-Tu directly binds Fn (Dallo et al., 2012). The genome-reduced, human pathogen, M. pneumoniae also displays EF-Tu on its cell surface and plays an important role in interactions with Fn (Dallo et al., 2002; Widjaja et al., 2017). Anti-EF-Tu antibodies are able to prevent the binding of M. pneumoniae to immobilized Fn demonstrating the specificity of this interaction (Dallo et al., 2002). Binding of EF-Tu is confined to the C-terminal region of EF-Tu, with two Fn-binding regions being identified at amino acid positions 192-292 and 314-394 (Balasubramanian et al., 2008). The specificity of the Fn binding domain between amino acids 314-394 was confirmed when peptides spanning this region blocked the binding capacity of EF-Tu to Fn by 62% (Balasubramanian et al., 2009). Notably, EF-Tu from Mycoplasma genitalium, which shares 96% identity with EF-Tu from M. pneumoniae, does not bind Fn (Balasubramanian et al., 2009). Experimental comparison between the two sequences identified the residues S343, P345 and T357 to be key in the interaction with Fn (Balasubramanian et al., 2009). However, the Fn-binding A. baumannii EF-Tu does not possess these key binding residues identified in M. pneumoniae (see Supplementary File), suggesting an alternative Fn-binding mechanism. More recently, we have shown that EF-Tu from M. pneumoniae is a multifunctional, adhesive moonlighting protein that can bind fetuin, heparin, actin, as well as to plasminogen, vitronectin, lactoferrin, laminin, and fibrinogen (Widjaja et al., 2017).
The exogenous addition of soluble Fn is known to promote the ability of Mycobacterium avium subsp. paratuberculosis to attach and invade two epithelial cell lines (Secott et al., 2002) but the identity of bacterial cell surface receptor(s) for Fn were not known. EF-Tu is a surface exposed cell wall protein in Mycobacterium avium subspecies paratuberculosis (MAP) (Viale et al., 2014). With the importance of Fn to MAP adhesion and invasion (Secott et al., 2002), and as EF-Tu is known to bind Fn in M. pneumoniae (Dallo et al., 2002; Balasubramanian et al., 2008) and A. baumannii (Dallo et al., 2012), it was investigated for its role as a Fn-binding protein. Knowledge of the Fn-binding regions of EF-Tu from M. pneumoniae was used to map putative Fn-binding regions in EF-Tu from A. baumannii (Dallo et al., 2012). Although the two previously identified Fn-binding regions (Balasubramanian et al., 2008) only showed 73 and 69% identity respectively in the EF-Tu homolog from MAP, ELISA assays showed that MAP EF-Tu binds Fn in a dose-dependent manner (Viale et al., 2014).
EF-Tu binds to human intestinal cells and mucins in a pH-dependent manner in the probiotic bacterium, Lactobacillus johnsonii suggesting a role for EF-Tu in gut colonization (Granato et al., 2004). At pH 5, EF-Tu derived from L. johnsonii was able to bind to the human colorectal adenocarcinoma cell line HT29 cells, undifferentiated CACO-2 cells and mucins isolated from HT29-MTX cells (Granato et al., 2004). The ability of EF-Tu to bind mucins extends to other anatomical locations, including the saliva mucin, MUC7 (Kesimer et al., 2009). EF-Tu was identified as one of six proteins to bind this mucin in Streptococcus gordonii, a major oral colonizer (Kesimer et al., 2009).
When H. pylori is co-cultured with THP-1 cells, expression of EF-Tu is upregulated and secreted and shown to localize to the surface of the human monocytic cell line THP-1 implicating it in host adhesion (Chiu et al., 2016).
While there are currently no universal moonlighting motifs (Babady et al., 2007), basic residues, both singularly and in clusters, are known to be binding anchors for anionic glycosaminoglycans (Jayaraman et al., 2000), are key in plasminogen (Jarocki et al., 2015), DNA (Ruyechan and Olson, 1992) and actin-binding (Peng et al., 2018), and have been linked with biofilm formation (Shanks et al., 2005; Green et al., 2013). Such basic residue clusters are found throughout the sequences of EF-Tus with reported moonlighting functions (Figure 3 and Supplementary File).
Figure 3. Basic amino acid residue clusters that reside within EF-Tu molecules that moonlight in prokaryotes. An exposed basic amino acid cluster (blue bar) with a putative P:P interaction sites (green circle) is present at the N-termini of moonlighting EF-Tu examples from both Gram positive and Gram-negative bacteria. However, only the Gram-positive bacteria appear to have a consensus heparin binding motif (red bar) and only in B. subtilis is this motif predicted to be solvent accessible (solid red bar). The remaining basic residue clusters are predicted to be at least partial buried.
A basic residue cluster is located at the N-termini of EF-Tu molecules with known moonlighting functions (Figure 3 and Table 2). This short linear motif (SLiM) has at least three surface exposed basic residues, resides within a region of protein disorder, and possesses predicted protein:protein interaction sites. The arginine and lysine residues in positions 7 and 9 are unconserved in some bacterial species, thus may have arisen from advantageous point mutations (Figure 4).
Figure 4. N-termini basic residue cluster logo. The logo is representative of EF-Tu molecules derived from 17 prokaryote species.
Interestingly, the moonlighting EF-Tu from Mycoplasma fermentans has a unique, highly surface exposed, N-terminal extension that is 39 amino acids in length and possess an additional basic residue cluster at 31nKmKgKy38 (see Supplementary File). Whether M. fermentans EF-Tu harbors moonlighting adhesive capabilities is currently unknown and may warrant future investigation.
Apart from the N-termini, the remaining basic residue clusters observed in EF-Tu molecules that moonlight are at least partially buried, which may impede their ability to bind host molecules. However selective cell surface proteolysis, described in detail in Section “Post-translational Modifications of EF-Tu,” can overcome some of the structural impediments.
Many bacterial pathogens of medical (Sanderson-Smith et al., 2012) and veterinary (Raymond and Djordjevic, 2015) significance have the ability to bind and activate plasminogen, a process that relies on interactions between basic amino acid residues on surface-accessible bacterial adhesins and kringle domains in plasminogen (Figure 2A). EF-Tu is also utilized by bacteria to dampen the host immune response. The ability of EF-Tu to bind to host complement factors and plasminogen has been demonstrated in Pseudomonas aeruginosa (Kunert et al., 2007), Leptospira sp. (Wolff et al., 2013), Streptococcus pneumoniae (Mohan et al., 2014) M. pneumoniae (plasminogen only) (Widjaja et al., 2017) and Acinetobacter baumannii (plasminogen only) (Koenigs et al., 2015). The complement system is part of the innate immune system which non-specifically acts to clear the body of infection by lysing bacteria (Lambris et al., 2008). Complement factors bound on the bacterial surface remain active, and plasminogen can be converted to plasmin in its bound state (Kunert et al., 2007; Wolff et al., 2013; Mohan et al., 2014; Raymond and Djordjevic, 2015). This allows the bacteria to regulate and utilize these components for their own gain. By recruiting FH, FH1 and plasminogen the bacteria are able to inactivate C3b via a cleavage event mediated by FH, FH1 and plasmin (Lambris et al., 2008). The C3b complement factor is an important opsonization trigger that binds and labels bacteria ready for opsonization by the host immune system (Lambris et al., 2008). By degrading C3b, the bacteria are able to overcome this aspect of the innate immune system (Lambris et al., 2008). Additionally, the recruitment of plasmin to the bacterial cell surface plays an important role in degrading ECM and facilitating tissue invasion (Lahteenmaki et al., 2000; Bhattacharya et al., 2012). By binding complement factors such as Factor-H, FHL-1 and CFHR1 the bacterium is able to suppress this process and evade the host innate immune response.
Bacterial infections can also lead to a reduction in white blood cell (WBC) counts in infected hosts leading to leukopenia. Isolates of K. pneumoniae from patients with leukopenia express higher levels of EF-Tu compared with K. pneumoniae isolates from patients with leucocytosis (Liu et al., 2014) suggesting that EF-Tu may be a pathogenicity factor in K. pneumonia-based leukopenia (Liu et al., 2014). Interestingly, EF-Tu is upregulated in Mycobacterium species that have been phagocytosed by macrophages. The purpose of this upregulation has yet to be determined, but may further support the idea of a role for EF-Tu in bacterial evasion of the host immune system (Monahan et al., 2001).
Not only do bacteria have to defend themselves against the host immune response, but often they must defend themselves against each other. Due to the competitive environment in which bacteria live, they have developed toxins that target other bacterial cells. The Type VI secretion system (T6SS) can deliver toxin molecules into the cytosol of competing bacteria inhabiting the same niche (Pukatzki et al., 2006; Coulthurst, 2013; Cianfanelli et al., 2016). For the T6SS effector molecule in P. aeruginosa to enact its toxic effect, it must interact with EF-Tu prior to delivery into the recipient’s cytoplasm (Whitney et al., 2015). The effector molecule and EF-Tu directly bind to each other in members of the Pseudomonas genus (Whitney et al., 2015). These observations suggest that EF-Tu has a wide variety of moonlighting functions relating to pathogenesis in different prokaryote species.
Some bacteria can change cell shape as a protective strategy against the immune system. By changing shape, bacteria can become less easily engulfed by phagocytes, and can enhance biofilm formation thereby increasing persistence in the host (van Teeseling et al., 2017). Moreover, major actin-like cytoskeletal proteins, such as MreB, have a role in virulence. For example, in P. aeruginosa MreB regulates the type IV pili assemblage (Cowles and Gitai, 2010); in H. pylori MreB regulates the secretion of virulence factors (Waidner et al., 2009); and in Salmonella enterica serovar Typhimurium a disruption in the mreB gene led to downregulation of genes involved in pathogenicity (Bulmer et al., 2012; Doble et al., 2012).
The hypothesis that EF-Tu may interact with the cytoskeleton in prokaryotes is not a new idea, with the concept being introduced as early as the 1970s when EF-Tu was shown to form filaments (Beck, 1979). More recently, the formation of amyloid-like filaments by Gallibacterium anatis EF-Tu has been linked to biofilm formation (López-Ochoa et al., 2017). EF-Tu also interacts with MreB in E. coli (Butland et al., 2005). MreB forms helical filaments beneath the cell membrane and is essential for regulating cell shape (Jones et al., 2001). As it is well known that eukaryotic EF-Tu (eEF1A) interacts with actin, and influences cell shape, it is conceivable that this moonlighting function also occurs in prokaryotes. In B. subtilis and E. coli (Defeu Soufo et al., 2010, 2015), EF-Tu modulates the formation of MreB filaments by binding MreB in a ratio of 1:1 (Defeu Soufo et al., 2015) (Figure 2C). One hypothesis suggests a link between EF-Tu cell concentration and cytoskeletal function. By reducing the expression of EF-Tu in the cell, cell shape can be modulated from the typical rod-like appearance to an abnormal cell shape (Defeu Soufo et al., 2010). Alteration of cell shape is due to disruption of the process that places MreB in helical structures beneath the membrane (Defeu Soufo et al., 2010). Further studies investigated whether EF-Tu’s role in the translation mechanism was directly related to the population of EF-Tu molecules that interact with MreB, or whether separate populations of EF-Tu are generated for these alternate roles. Treatment of bacterial cells with kirromycin, which inhibits the release of EF-Tu from the ribosome, failed to interfere with EF-Tu localization in the cytoskeleton or its interactions with MreB filaments (Defeu Soufo et al., 2010). As previous studies have only revealed an interaction between tufB with mreB (not tufA) (Butland et al., 2005), it is tantalizing to consider whether only one of the tuf genes is responsible for the alternate function of cytoskeletal integrity. This might suggest that evolution of two tuf genes is useful in the delegation of EF-Tu moonlighting roles.
Proteolytic processing is an irreversible post-translational modification (PTM) that can result in a loss, gain or change of function in a protein, as well as in degradation (Turk, 2006). Processing may be a mechanism to unlock moonlighting functions that are inherent in the newly created cleavage fragments via a mechanism similar to ectodomain shedding (Raymond et al., 2013; Tacchi et al., 2016) (Figure 2D). Furthermore, cleavage fragments may serve as competitive inhibitors to host immune cells. Host cytokines, chemokines, enzymes, antimicrobial peptides and growth factors all bind ECM components, including heparin and fibronectin, to control immune responses such as leukocyte emigration through tissue (Gill et al., 2010). By binding to the same ligands as host effector molecules (Kaneider et al., 2007; Krachler and Orth, 2013), bacterial proteins and their cleavage fragments may dampen an immune response. Additionally, cleavage may result in the loss of antigenic epitopes in surface proteins thereby circumventing host immune detection.
Cell surface EF-Tu is proteolytically processed in M. hyopneumoniae (Tacchi et al., 2016; Berry et al., 2017; Widjaja et al., 2017). Cleavage fragments of EF-Tu are retained on the cell surface and recovered during affinity chromatography using different host molecules as bait (Tacchi et al., 2016). The cleavage of EF-Tu has recently been demonstrated to be more widespread than previously appreciated with cleavage sites now also mapped in the M. pneumoniae and S. aureus (Scherl et al., 2005; Plikat et al., 2007; Widjaja et al., 2017). Processing generates fragments that are predicted to be more structural disordered and exposes regions of EF-Tu that are normally inaccessible to the aqueous environment. In particular we have shown that novel SLiMs enriched in positive charges are exposed allowing them to bind to a range of host molecules (Widjaja et al., 2017). Processing has so far been described in bacteria that belong to the low G + C Firmicutes. Many protein:protein and protein:nucleic acid interactions require correctly spaced positive charges derived from lysine, arginine and histidine side chains in short regions of peptide sequence. Amino acids with positively charged side chain residues are encoded by A:T rich triplet codons and members of the low G + C Firmicutes are well suited to employ this as a strategy to expand their functional proteome. Single amino acid substitutions caused by single nucleotide polymorphisms (SNPs) have previously been described as pathogenicity-enhancing (Weissman et al., 2003). Specifically, SNPs in E. coli and S. typhimurium adhesin genes have led to distinctive pathogenicity-enhanced phenotypes (Sokurenko et al., 1998; Pouttu et al., 1999; Boddicker et al., 2002). SNPs can provide bacteria a selective advantage, leading to niche expansion and ultimately, novel species (Weissman et al., 2003). We propose that the accumulation of positively charged residues via SNPs in SLiMs facilitates binding interactions with diverse host molecules (Widjaja et al., 2017). Processing presents a mechanism to release fragments, each with the potential to expose a different repertoire of SLiMS to the aqueous environment compared with the parent molecule, and generate protein multifunctionality (Widjaja et al., 2017).
Bacterial EF-Tus are also the target for reversible PTMs such as phosphorylation, methylation and acetylation. Phosphorylation of EF-Tu has been identified in E. coli (Lippmann et al., 1993), Thermus thermophiles (Lippmann et al., 1993), B. subtilis (Levine et al., 2006), Corynebacterium glutamicum (Bendt et al., 2003), Streptomyces collinus (Mikulik and Zhulanova, 1995), Thiobacillus ferrooxidans (Seeger et al., 1996), S. pneumoniae (Sun et al., 2010), M. genitalium (Su et al., 2007), and M. pneumoniae (Su et al., 2007). While phosphorylation of EF-Tu lowers its binding affinity to GTP, subsequently reducing protein synthesis, the PTM also inhibits binding by the antibiotic kirromycin, a specific inhibitor of EF-Tu (Archambaud et al., 2005; Sajid et al., 2011). Furthermore, the associated decreased bacterial growth facilitated by EF-Tu phosphorylation has been implicated as an acclimation measure to stress conditions during infection (Archambaud et al., 2005). Similarly, Van Noort et al. (1986) demonstrated that EF-Tu methylation in E. coli lowers GTP hydrolysis and suggest a more accurate translation process as a result.
Lysine acetylation and lysine glutarylation has been described in EF-Tu from Mycobacterium tuberculosis (Xie et al., 2015, 2016). These modifications can affect protein–protein and protein:nucleic acid interactions and it may be important to the pathogenicity of M. tuberculosis, although this is yet to be determined. Evidence for the role of PTMs in EF-Tu moonlighting functions have been described in P. aeruginosa, where the trimethylation of the lysine at residue 5, allows EF-Tu to structurally mimic phosphorylcholine (Barbier et al., 2013). This modification means that EF-Tu can specifically bind a platelet-activating receptor, resulting in successful bacterial adhesion (Barbier et al., 2013).
EF-Tu has evolved to be a multifunctional protein in a wide variety of pathogenic bacteria. While moonlighting functions vary among microbial species there is a common theme for roles in adherence and in immune regulation. The understanding of how this essential and highly expressed protein evolved moonlighting functions is an active area of research and is likely that more diverse and important roles are yet to be discovered.
KH and SD conceived and co-wrote the first draft with input from all authors for the final draft. VJ created the figures and contributed significantly to the revised manuscript. All authors contributed to revision, read and approved the submitted version.
This work was supported by funds provided to SD from the University of Technology Sydney. Some of the authors were partly supported by funds from the Australian Centre for Genomic Epidemiological Microbiology (Ausgem), a collaborative partnership between the NSW Department of Primary Industries and the University of Technology Sydney.
The authors declare that the research was conducted in the absence of any commercial or financial relationships that could be construed as a potential conflict of interest.
The authors would like to thank Judith Pell for providing editorial assistance during the writing of this manuscript.
The Supplementary Material for this article can be found online at: https://www.frontiersin.org/articles/10.3389/fmicb.2019.02351/full#supplementary-material
FILE S1 | Amino acid sequence analysis of EF-Tus with moonlighting functions.
Abbas, W., Kumar, A., and Herbein, G. (2015). The eEF1A proteins: at the crossroads of oncogenesis, apoptosis, and viral infections. Front. Oncol. 5:75. doi: 10.3389/fonc.2015.00075
Altindis, E., Dong, T., Catalano, C., and Mekalanos, J. (2015). Secretome analysis of Vibrio cholerae type VI secretion system reveals a new effector-immunity pair. mBio 6:e00075-15. doi: 10.1128/mBio.00075-15
Alzahrani, H., Winter, J., Boocock, D., De Girolamo, L., and Forsythe, S. J. (2015). Characterization of outer membrane vesicles from a neonatal meningitic strain of Cronobacter sakazakii. FEMS Microbiol. Lett. 362:fnv085. doi: 10.1093/femsle/fnv085
Archambaud, C., Gouin, E., Pizarro-Cerda, J., Cossart, P., and Dussurget, O. (2005). Translation elongation factor EF-Tu is a target for Stp, a serine-threonine phosphatase involved in virulence of Listeria monocytogenes. Mol. Microbiol. 56, 383–396. doi: 10.1111/j.1365-2958.2005.04551.x
Arntzen, M. O., Karlskas, I. L., Skaugen, M., Eijsink, V. G., and Mathiesen, G. (2015). Proteomic Investigation of the response of Enterococcus faecalis V583 when cultivated in urine. PLoS One 10:e0126694. doi: 10.1371/journal.pone.0126694
Babady, N. E., Pang, Y.-P., Elpeleg, O., and Isaya, G. (2007). Cryptic proteolytic activity of dihydrolipoamide dehydrogenase. Proc. Natl. Acad. Sci. U.S.A. 104, 6158–6163. doi: 10.1073/pnas.0610618104
Balasubramanian, S., Kannan, T. R., and Baseman, J. B. (2008). The surface-exposed carboxyl region of Mycoplasma pneumoniae elongation factor Tu interacts with fibronectin. Infect. Immun. 76, 3116–3123. doi: 10.1128/IAI.00173-08
Balasubramanian, S., Kannan, T. R., Hart, P. J., and Baseman, J. B. (2009). Amino acid changes in elongation factor Tu of Mycoplasma pneumoniae and Mycoplasma genitalium influence fibronectin binding. Infect. Immun. 77, 3533–3541. doi: 10.1128/IAI.00081-09
Balbo, M., Barel, M., Lottin-Divoux, S., Jean, D., and Frade, R. (2005). Infection of human B lymphoma cells by Mycoplasma fermentans induces interaction of its elongation factor with the intracytoplasmic domain of Epstein-Barr virus receptor (gp140, EBV/C3dR, CR2, CD21). FEMS Microbiol. Lett. 249, 359–366. doi: 10.1016/j.femsle.2005.06.052
Baldauf, S. L., Palmer, J. D., and Doolittle, W. F. (1996). The root of the universal tree and the origin of eukaryotes based on elongation factor phylogeny. Proc. Natl. Acad. Sci. U.S.A. 93, 7749–7754. doi: 10.1073/pnas.93.15.7749
Barbier, M., Owings, J. P., Martinez-Ramos, I., Damron, F. H., Gomila, R., Blazquez, J., et al. (2013). Lysine trimethylation of EF-Tu mimics platelet-activating factor to initiate Pseudomonas aeruginosa pneumonia. mBio 4:e00207-13. doi: 10.1128/mBio.00207-13
Barel, M., Hovanessian, A. G., Meibom, K., Briand, J. P., Dupuis, M., and Charbit, A. (2008). A novel receptor - ligand pathway for entry of Francisella tularensis in monocyte-like THP-1 cells: interaction between surface nucleolin and bacterial elongation factor Tu. BMC Microbiol. 8:145. doi: 10.1186/1471-2180-8-145
Beck, B. D. (1979). Polymerization of the bacterial elongation factor for protein synthesis, EF-Tu. Eur. J. Biochem. 97, 495–502. doi: 10.1111/j.1432-1033.1979.tb13137.x
Bendt, A. K., Burkovski, A., Schaffer, S., Bott, M., Farwick, M., and Hermann, T. (2003). Towards a phosphoproteome map of Corynebacterium glutamicum. Proteomics 3, 1637–1646. doi: 10.1002/pmic.200300494
Bendtsen, J. D., Kiemer, L., Fausbøll, A., and Brunak, S. (2005). Non-classical protein secretion in bacteria. BMC Microbiol. 5:58. doi: 10.1186/1471-2180-5-58
Bergmann, S., Rohde, M., Chhatwal, G. S., and Hammerschmidt, S. (2001). alpha-Enolase of Streptococcus pneumoniae is a plasmin(ogen)-binding protein displayed on the bacterial cell surface. Mol. Microbiol. 40, 1273–1287. doi: 10.1046/j.1365-2958.2001.02448.x
Berry, I. J., Jarocki, V. M., Tacchi, J. L., Raymond, B. B. A., Widjaja, M., Padula, M. P., et al. (2017). N-terminomics identifies widespread endoproteolysis and novel methionine excision in a genome-reduced bacterial pathogen. Sci. Rep. 7:11063. doi: 10.1038/s41598-017-11296-9
Bhattacharya, S., Ploplis, V. A., and Castellino, F. J. (2012). Bacterial plasminogen receptors utilize host plasminogen system for effective invasion and dissemination. J. Biomed. Biotechnol. 2012:482096. doi: 10.1155/2012/482096
Bingham, R., Ekunwe, S. I., Falk, S., Snyder, L., and Kleanthous, C. (2000). The major head protein of bacteriophage T4 binds specifically to elongation factor Tu. J. Biol. Chem. 275, 23219–23226. doi: 10.1074/jbc.M002546200
Boddicker, J. D., Ledeboer, N. A., Jagnow, J., Jones, B. D., and Clegg, S. (2002). Differential binding to and biofilm formation on, HEp-2 cells by Salmonella enterica serovar typhimurium is dependent upon allelic variation in the fimH gene of the fim gene cluster. Mol. Microbiol. 45, 1255–1265. doi: 10.1046/j.1365-2958.2002.03121.x
Bogema, D. R., Deutscher, A. T., Woolley, L. K., Seymour, L. M., Raymond, B. B. A., Tacchi, J. L., et al. (2012). Characterization of cleavage events in the multifunctional cilium adhesin Mhp684 (P146) reveals a mechanism by which Mycoplasma hyopneumoniae regulates surface topography. mBio 3:e00282-11. doi: 10.1128/mBio.00282-11
Bosutti, A., Scaggiante, B., Grassi, G., Guarnieri, G., and Biolo, G. (2007). Overexpression of the elongation factor 1A1 relates to muscle proteolysis and proapoptotic p66(ShcA) gene transcription in hypercatabolic trauma patients. Metabolism 56, 1629–1634. doi: 10.1016/j.metabol.2007.07.003
Boysen, A., Borch, J., Krogh, T. J., Hjerno, K., and Moller-Jensen, J. (2015). SILAC-based comparative analysis of pathogenic Escherichia coli secretomes. J. Microbiol. Methods 116, 66–79. doi: 10.1016/j.mimet.2015.06.015
Bulmer, D. M., Kharraz, L., Grant, A. J., Dean, P., Morgan, F. J. E., Karavolos, M. H., et al. (2012). The bacterial cytoskeleton modulates motility, type 3 secretion, and colonization in Salmonella. PLoS Pathog. 8:e1002500. doi: 10.1371/journal.ppat.1002500
Burtnick, M. N., Brett, P. J., and DeShazer, D. (2014). Proteomic analysis of the Burkholderia pseudomallei type II secretome reveals hydrolytic enzymes, novel proteins, and the deubiquitinase TssM. Infect. Immun. 82, 3214–3226. doi: 10.1128/IAI.01739-14
Butland, G., Peregrin-Alvarez, J. M., Li, J., Yang, W., Yang, X., Canadien, V., et al. (2005). Interaction network containing conserved and essential protein complexes in Escherichia coli. Nature 433, 531–537. doi: 10.1038/nature03239
Caamano-Antelo, S., Fernandez-No, I. C., Bohme, K., Ezzat-Alnakip, M., Quintela-Baluja, M., Barros-Velazquez, J., et al. (2015). Genetic discrimination of foodborne pathogenic and spoilage Bacillus spp. based on three housekeeping genes. Food Microbiol. 46, 288–298. doi: 10.1016/j.fm.2014.08.013
Cacan, E., Kratzer, J. T., Cole, M. F., and Gaucher, E. A. (2013). Interchanging functionality among homologous elongation factors using signatures of heterotachy. J. Mol. Evol. 76, 4–12. doi: 10.1007/s00239-013-9540-9
Candela, M., Centanni, M., Fiori, J., Biagi, E., Turroni, S., Orrico, C., et al. (2010). DnaK from Bifidobacterium animalis subsp. Lactis is a surface-exposed human plasminogen receptor upregulated in response to bile salts. Microbiology 156, 1609–1618. doi: 10.1099/mic.0.038307-0
Cao, Y., and Bazemore-Walker, C. R. (2014). Proteomic profiling of the surface-exposed cell envelope proteins of Caulobacter crescentus. J. Proteomics 97, 187–194. doi: 10.1016/j.jprot.2013.08.011
Carpenter, L. L., Bayat, L., Moreno, F., Kling, M. A., Price, L. H., Tyrka, A. R., et al. (2008). Decreased cerebrospinal fluid concentrations of substance P in treatment-resistant depression and lack of alteration after acute adjunct vagus nerve stimulation therapy. Psychiatry Res. 157, 123–129. doi: 10.1016/j.psychres.2007.04.016
Carrasco, S. E., Yang, Y., Troxell, B., Yang, X., Pal, U., and Yang, X. F. (2015). Borrelia burgdorferi elongation factor EF-Tu is an immunogenic protein during Lyme borreliosis. Emerg. Microbes Infect. 4:e54. doi: 10.1038/emi.2015.54
Chande, A. G., Siddiqui, Z., Midha, M. K., Sirohi, V., Ravichandran, S., and Rao, K. V. (2015). Selective enrichment of mycobacterial proteins from infected host macrophages. Sci. Rep. 5:13430. doi: 10.1038/srep13430
Chiu, K. H., Wang, L. H., Tsai, T. T., Lei, H. Y., and Liao, P. C. (2016). Secretomic analysis of host-pathogen interactions reveals that elongation Factor-Tu is a potential adherence factor of Helicobacter pylori during pathogenesis. J. Proteome Res. 16, 264–273. doi: 10.1021/acs.jproteome.6b00584
Choi, D. S., Kim, D. K., Choi, S. J., Lee, J., Choi, J. P., Rho, S., et al. (2011). Proteomic analysis of outer membrane vesicles derived from Pseudomonas aeruginosa. Proteomics 11, 3424–3429. doi: 10.1002/pmic.201000212
Christie-Oleza, J. A., Armengaud, J., Guerin, P., and Scanlan, D. J. (2015a). Functional distinctness in the exoproteomes of marine Synechococcus. Env. Microbiol. 17, 3781–3794. doi: 10.1111/1462-2920.12822
Christie-Oleza, J. A., Scanlan, D. J., and Armengaud, J. (2015b). “You produce while I clean up”, a strategy revealed by exoproteomics during Synechococcus-Roseobacter interactions. Proteomics 15, 3454–3462. doi: 10.1002/pmic.201400562
Chung, M.-C., Tonry, J. H., Narayanan, A., Manes, N. P., Mackie, R. S., Gutting, B., et al. (2011). Bacillus anthracis interacts with plasmin(ogen) to evade C3b-dependent innate immunity. PLoS One 6:e18119. doi: 10.1371/journal.pone.0018119
Churchward, C. P., Rosales, R. S., Gielbert, A., Dominguez, M., Nicholas, R. A., and Ayling, R. D. (2015). Immunoproteomic characterisation of Mycoplasma mycoides subspecies capri by mass spectrometry analysis of two-dimensional electrophoresis spots and western blot. J. Pharm. Pharmacol. 67, 364–371. doi: 10.1111/jphp.12344
Cianfanelli, F. R., Monlezun, L., and Coulthurst, S. J. (2016). Aim, load, fire: the type VI secretion system, a bacterial nanoweapon. Trends Microbiol. 24, 51–62. doi: 10.1016/j.tim.2015.10.005
Clair, G., Lorphelin, A., Armengaud, J., and Duport, C. (2013). OhrRA functions as a redox-responsive system controlling toxinogenesis in Bacillus cereus. J. Proteomics 94, 527–539. doi: 10.1016/j.jprot.2013.10.024
Coelho, C., Brown, L., Maryam, M., Vij, R., Smith, D. F. Q., Burnet, M. C., et al. (2019). Listeria monocytogenes virulence factors, including listeriolysin O, are secreted in biologically active extracellular vesicles. J. Biol. Chem. 294, 1202–1217. doi: 10.1074/jbc.RA118.006472
Coulthurst, S. J. (2013). The type VI secretion system - a widespread and versatile cell targeting system. Res. Microbiol. 164, 640–654. doi: 10.1016/j.resmic.2013.03.017
Cowles, K. N., and Gitai, Z. (2010). Surface association and the MreB cytoskeleton regulate pilus production, localization and function in Pseudomonas aeruginosa. Mol. Microbiol. 76, 1411–1426. doi: 10.1111/j.1365-2958.2010.07132.x
Dalla Vecchia, E., Shao, P. P., Suvorova, E., Chiappe, D., Hamelin, R., and Bernier-Latmani, R. (2014). Characterization of the surfaceome of the metal-reducing bacterium Desulfotomaculum reducens. Front. Microbiol. 5:432. doi: 10.3389/fmicb.2014.00432
Dallo, S. F., Kannan, T. R., Blaylock, M. W., and Baseman, J. B. (2002). Elongation factor Tu and E1 beta subunit of pyruvate dehydrogenase complex act as fibronectin binding proteins in Mycoplasma pneumoniae. Mol. Microbiol. 46, 1041–1051. doi: 10.1046/j.1365-2958.2002.03207.x
Dallo, S. F., Zhang, B., Denno, J., Hong, S., Tsai, A., Haskins, W., et al. (2012). Association of Acinetobacter baumannii EF-Tu with cell surface, outer membrane vesicles, and fibronectin. ScientificWorldJournal 2012:128705. doi: 10.1100/2012/128705
Datar, P., Srivastava, S., Coutinho, E., and Govil, G. (2004). Substance P: structure, function, and therapeutics. Curr. Top. Med. Chem 4, 75–103. doi: 10.2174/1568026043451636
Defeu Soufo, H. J., Reimold, C., Breddermann, H., Mannherz, H. G., and Graumann, P. L. (2015). Translation elongation factor EF-Tu modulates filament formation of actin-like MreB protein in vitro. J. Mol. Biol. 427, 1715–1727. doi: 10.1016/j.jmb.2015.01.025
Defeu Soufo, H. J., Reimold, C., Linne, U., Knust, T., Gescher, J., and Graumann, P. L. (2010). Bacterial translation elongation factor EF-Tu interacts and colocalizes with actin-like MreB protein. Proc. Natl. Acad. Sci. U.S.A. 107, 3163–3168. doi: 10.1073/pnas.0911979107
Deutscher, A. T., Jenkins, C., Minion, F. C., Seymour, L. M., Padula, M. P., Dixon, N. E., et al. (2010). Repeat regions R1 and R2 in the P97 paralogue Mhp271 of Mycoplasma hyopneumoniae bind heparin, fibronectin and porcine cilia. Mol. Microbiol. 78, 444–458. doi: 10.1111/j.1365-2958.2010.07345.x
Dhanani, A. S., and Bagchi, T. (2013). The expression of adhesin EF-Tu in response to mucin and its role in Lactobacillus adhesion and competitive inhibition of enteropathogens to mucin. J. Appl. Microbiol. 115, 546–554. doi: 10.1111/jam.12249
Dhanani, A. S., Gaudana, S. B., and Bagchi, T. (2011). The ability of Lactobacillus adhesin EF-Tu to interfere with pathogen adhesion. Eur. Food Res. Technol. 232, 777–785. doi: 10.1007/s00217-011-1443-7
Doble, A. C., Bulmer, D. M., Kharraz, L., Karavolos, M. H., and Khan, C. M. A. (2012). The function of the bacterial cytoskeleton in Salmonella pathogenesis. Virulence 3, 446–449. doi: 10.4161/viru.20993
Dominguez, D. C., Lopes, R., and Palomino, J. (2009). B. subtilis elongation factor Tu binds Calcium ions. FASEB J. 23:LB211.
Douglas, S. D., Ho, W. Z., Gettes, D. R., Cnaan, A., Zhao, H., Leserman, J., et al. (2001). Elevated substance P levels in HIV-infected men. AIDS 15, 2043–2045. doi: 10.1097/00002030-200110190-00019
Ebner, K., and Singewald, N. (2006). The role of substance P in stress and anxiety responses. Amino Acids 31, 251–272. doi: 10.1007/s00726-006-0335-9
Ebner, P., and Götz, F. (2019). Bacterial excretion of cytoplasmic proteins (ECP): occurrence, mechanism, and function. Trends Microbiol. 27, 176–187. doi: 10.1016/j.tim.2018.10.006
Egea, L., Aguilera, L., Gimenez, R., Sorolla, M. A., Aguilar, J., Badia, J., et al. (2007). Role of secreted glyceraldehyde-3-phosphate dehydrogenase in the infection mechanism of enterohemorrhagic and enteropathogenic Escherichia coli: interaction of the extracellular enzyme with human plasminogen and fibrinogen. Int. J. Biochem. Cell Biol. 39, 1190–1203. doi: 10.1016/j.biocel.2007.03.008
Ejiri, S. (2002). Moonlighting functions of polypeptide elongation factor 1: from actin bundling to zinc finger protein R1-associated nuclear localization. Biosci. Biotechnol. Biochem. 66, 1–21. doi: 10.1271/bbb.66.1
Eshghi, A., Henderson, J., Trent, M. S., and Picardeau, M. (2015). Leptospira interrogans lpxD homologue is required for thermal acclimatization and virulence. Infect. Immun. 83, 4314–4321. doi: 10.1128/IAI.00897-15
Espino, E., Koskenniemi, K., Mato-Rodriguez, L., Nyman, T. A., Reunanen, J., Koponen, J., et al. (2015). Uncovering surface-exposed antigens of Lactobacillus rhamnosus by cell shaving proteomics and two-dimensional immunoblotting. J. Proteome Res. 14, 1010–1024. doi: 10.1021/pr501041a
Esteban, F., Munoz, M., Gonzalez-Moles, M. A., and Rosso, M. (2006). A role for substance P in cancer promotion and progression: a mechanism to counteract intracellular death signals following oncogene activation or DNA damage. Cancer Metastasis Rev. 25, 137–145. doi: 10.1007/s10555-006-8161-9
Fehder, W. P., Sachs, J., Uvaydova, M., and Douglas, S. D. (1997). Substance P as an immune modulator of anxiety. Neuroimmunomodulation 4, 42–48. doi: 10.1159/000097314
Feng, L., Niu, X., Mei, W., Li, W., Liu, Y., Willias, S. P., et al. (2018). Immunogenicity and protective capacity of EF-Tu and FtsZ of Streptococcus suis serotype 2 against lethal infection. Vaccine 36, 2581–2588. doi: 10.1016/j.vaccine.2018.03.079
Ferreira, R. M., Moreira, L. M., Ferro, J. A., Soares, M. R., Laia, M. L., Varani, A. M., et al. (2016). Unravelling potential virulence factor candidates in Xanthomonas citri. subsp. citri by secretome analysis. PeerJ 4:e1734. doi: 10.7717/peerj.1734
Filer, D., and Furano, A. V. (1980). Portions of the gene encoding elongation factor Tu are highly conserved in prokaryotes. J. Biol. Chem. 255, 728–734.
Filer, D., and Furano, A. V. (1981). Duplication of the tuf gene, which encodes peptide chain elongation factor Tu, is widespread in Gram-negative bacteria. J. Bacteriol. 148, 1006–1011.
Furano, A. V. (1975). Content of elongation factor Tu in Escherichia coli. Proc. Natl. Acad. Sci. U.S.A. 72, 4780–4784. doi: 10.1073/pnas.72.12.4780
Furukawa, T., Inagaki, H., Takai, R., Hirai, H., and Che, F. S. (2014). Two distinct EF-Tu epitopes induce immune responses in rice and Arabidopsis. Mol. Plant Microbe Interact. 27, 113–124. doi: 10.1094/MPMI-10-13-0304-R
Gaurivaud, P., Ganter, S., Villard, A., Manso-Silvan, L., Chevret, D., Boulé, C., et al. (2018). Mycoplasmas are no exception to extracellular vesicles release: revisiting old concepts. PLoS One 13:e0208160. doi: 10.1371/journal.pone.0208160
Georgiou, T., Yu, Y. N., Ekunwe, S., Buttner, M. J., Zuurmond, A., Kraal, B., et al. (1998). Specific peptide-activated proteolytic cleavage of Escherichia coli elongation factor Tu. Proc. Natl. Acad. Sci. U.S.A. 95, 2891–2895. doi: 10.1073/pnas.95.6.2891
Gill, S., Wight, T. N., and Frevert, C. W. (2010). Proteoglycans: key regulators of pulmonary inflammation and the innate immune response to lung infection. Anat. Rec. Hoboken N. J. 2007, 968–981. doi: 10.1002/ar.21094
Goode, T., O’Connor, T., Hopkins, A., Moriarty, D., O’Sullivan, G. C., Collins, J. K., et al. (2003). Neurokinin-1 receptor (NK-1R) expression is induced in human colonic epithelial cells by proinflammatory cytokines and mediates proliferation in response to substance P. J. Cell. Physiol. 197, 30–41. doi: 10.1002/jcp.10234
Götz, F., Yu, W., Dube, L., Prax, M., and Ebner, P. (2015). Excretion of cytosolic proteins (ECP) in bacteria. Int. J. Med. Microbiol. 305, 230–237. doi: 10.1016/j.ijmm.2014.12.021
Granato, D., Bergonzelli, G. E., Pridmore, R. D., Marvin, L., Rouvet, M., and Corthesy-Theulaz, I. E. (2004). Cell surface-associated elongation factor Tu mediates the attachment of Lactobacillus johnsonii NCC533 (La1) to human intestinal cells and mucins. Infect. Immun. 72, 2160–2169. doi: 10.1128/iai.72.4.2160-2169.2004
Green, E., and Mecsas, J. (2016). Bacterial secretion systems: an overview, in: Virulence Mechanisms of Bacterial Pathogens. Washington, DC: ASM Press, 215–240.
Green, J. V., Orsborn, K. I., Zhang, M., Tan, Q. K. G., Greis, K. D., Porollo, A., et al. (2013). Heparin-binding motifs and biofilm formation by Candida albicans. J. Infect. Dis. 208, 1695–1704. doi: 10.1093/infdis/jit391
Grundel, A., Friedrich, K., Pfeiffer, M., Jacobs, E., and Dumke, R. (2015). Subunits of the pyruvate dehydrogenase cluster of Mycoplasma pneumoniae are surface-displayed proteins that bind and activate human plasminogen. PLoS One 10:e0126600. doi: 10.1371/journal.pone.0126600
He, Y., Wang, H., and Chen, L. (2015). Comparative secretomics reveals novel virulence-associated factors of Vibrio parahaemolyticus. Front. Microbiol. 6:707. doi: 10.3389/fmicb.2015.00707
Henderson, B., and Martin, A. (2011). Bacterial virulence in the moonlight: multitasking bacterial moonlighting proteins are virulence determinants in infectious disease. Infect. Immun. 79, 3476–3491. doi: 10.1128/IAI.00179-11
Henderson, B., and Martin, A. (2013). Bacterial moonlighting proteins and bacterial virulence. Curr. Top. Microbiol. Immunol. 358, 155–213. doi: 10.1007/82_2011_188
Henderson, B., Nair, S., Pallas, J., and Williams, M. A. (2011). Fibronectin: a multidomain host adhesin targeted by bacterial fibronectin-binding proteins. FEMS Microbiol. Rev. 35, 147–200. doi: 10.1111/j.1574-6976.2010.00243.x
Heras, B., Shouldice, S. R., Totsika, M., Scanlon, M. J., Schembri, M. A., and Martin, J. L. (2009). DSB proteins and bacterial pathogenicity. Nat. Rev. Microbiol. 7, 215–225. doi: 10.1038/nrmicro2087
Herpfer, I., and Lieb, K. (2005). Substance P receptor antagonists in psychiatry: rationale for development and therapeutic potential. CNS Drugs 19, 275–293. doi: 10.2165/00023210-200519040-00001
Huberts, D. H. E. W., and van der Klei, I. J. (2010). Moonlighting proteins: An intriguing mode of multitasking. Biochim. Biophys. Acta 1803, 520–525. doi: 10.1016/j.bbamcr.2010.01.022
Hughes, D. (1990). Both genes for EF-Tu in Salmonella typhimurium are individually dispensable for growth. J. Mol. Biol. 215, 41–51. doi: 10.1016/S0022-2836(05)80093-2
Igarashi, D., Bethke, G., Xu, Y., Tsuda, K., Glazebrook, J., and Katagiri, F. (2013). Pattern-triggered immunity suppresses programmed cell death triggered by fumonisin b1. PLoS One 8:e60769. doi: 10.1371/journal.pone.0060769
Iwabe, N., Kuma, K., Hasegawa, M., Osawa, S., and Miyata, T. (1989). Evolutionary relationship of archaebacteria, eubacteria, and eukaryotes inferred from phylogenetic trees of duplicated genes. Proc. Natl. Acad. Sci. U.S.A. 86, 9355–9359. doi: 10.1073/pnas.86.23.9355
Jain, S., Kumar, S., Dohre, S., Afley, P., Sengupta, N., and Alam, S. I. (2014). Identification of a protective protein from stationary-phase exoproteome of Brucella abortus. Pathog. Dis. 70, 75–83. doi: 10.1111/2049-632X.12079
Jarocki, V. M., Santos, J., Tacchi, J. L., Raymond, B. B. A., Deutscher, A. T., Jenkins, C., et al. (2015). MHJ_0461 is a multifunctional leucine aminopeptidase on the surface of Mycoplasma hyopneumoniae. Open Biol. 5:140175. doi: 10.1098/rsob.140175
Jayaraman, G., Wu, C. W., Liu, Y. J., Chien, K. Y., Fang, J. C., and Lyu, P. C. (2000). Binding of a de novo designed peptide to specific glycosaminoglycans. FEBS Lett. 482, 154–158. doi: 10.1016/s0014-5793(00)01964-5
Jeffery, C. J. (1999). Moonlighting proteins. Trends Biochem. Sci. 24, 8–11. doi: 10.1016/S0968-0004(98)01335-8
Jeffery, C. J. (2005). Mass spectrometry and the search for moonlighting proteins. Mass Spectrom. Rev. 24, 772–782. doi: 10.1002/mas.20041
Jeffery, C. J. (2018). Protein moonlighting: what is it, and why is it important? Philos. Trans. R. Soc. Lond. B. Biol. Sci. 373:20160523. doi: 10.1098/rstb.2016.0523
Jiang, F., He, J., Navarro-Alvarez, N., Xu, J., Li, X., Li, P., et al. (2016). Elongation factor Tu and heat shock protein 70 are membrane-associated proteins from Mycoplasma ovipneumoniae capable of inducing strong immune response in mice. PLoS One 11:e0161170. doi: 10.1371/journal.pone.0161170
Jimenez-Munguia, I., van Wamel, W. J., Olaya-Abril, A., Garcia-Cabrera, E., Rodriguez-Ortega, M. J., and Obando, I. (2015). Proteomics-driven design of a multiplex bead-based platform to assess natural IgG antibodies to pneumococcal protein antigens in children. J. Proteomics 126, 228–233. doi: 10.1016/j.jprot.2015.06.011
Jones, L. J., Carballido-Lopez, R., and Errington, J. (2001). Control of cell shape in bacteria: helical, actin-like filaments in Bacillus subtilis. Cell 104, 913–922.
Kainulainen, V., and Korhonen, T. K. (2014). Dancing to another tune-adhesive moonlighting proteins in bacteria. Biol. Basel 3, 178–204. doi: 10.3390/biology3010178
Kaneider, N. C., Djanani, A., and Wiedermann, C. J. (2007). Heparan sulfate proteoglycan-involving immunomodulation by cathelicidin antimicrobial peptides LL-37 and PR-39. ScientificWorldJournal 7, 1832–1838. doi: 10.1100/tsw.2007.285
Ke, D., Boissinot, M., Huletsky, A., Picard, F. J., Frenette, J., Ouellette, M., et al. (2000). Evidence for horizontal gene transfer in evolution of elongation factor Tu in enterococci. J. Bacteriol. 182, 6913–6920. doi: 10.1128/jb.182.24.6913-6920.2000
Kesimer, M., Kilic, N., Mehrotra, R., Thornton, D. J., and Sheehan, J. K. (2009). Identification of salivary mucin MUC7 binding proteins from Streptococcus gordonii. BMC Microbiol. 9:163. doi: 10.1186/1471-2180-9-163
Kim, S. K., Park, M. K., Kim, S. H., Oh, K. G., Jung, K. H., Hong, C. H., et al. (2014). Identification of stringent response-related and potential serological proteins released from Bacillus anthracis overexpressing the RelA/SpoT homolog, Rsh Bant. Curr. Microbiol. 69, 436–444. doi: 10.1007/s00284-014-0606-8
Kjeldgaard, M., Nissen, P., Thirup, S., and Nyborg, J. (1993). The crystal structure of elongation factor EF-Tu from Thermus aquaticus in the GTP conformation. Structure 1, 35–50. doi: 10.1016/0969-2126(93)90007-4
Kloppot, P., Selle, M., Kohler, C., Stentzel, S., Fuchs, S., Liebscher, V., et al. (2015). Microarray-based identification of human antibodies against Staphylococcus aureus antigens. Proteomics Clin. Appl. 9, 1003–1011. doi: 10.1002/prca.201400123
Koenigs, A., Zipfel, P. F., and Kraiczy, P. (2015). Translation elongation factor Tuf of Acinetobacter baumannii is a plasminogen-binding protein. PLoS One 10:e0134418. doi: 10.1371/journal.pone.0134418
Komeil, D., Padilla-Reynaud, R., Lerat, S., Simao-Beaunoir, A. M., and Beaulieu, C. (2014). Comparative secretome analysis of Streptomyces scabiei during growth in the presence or absence of potato suberin. Proteome Sci. 12:35. doi: 10.1186/1477-5956-12-35
Krachler, A. M., and Orth, K. (2013). Targeting the bacteria-host interface: strategies in anti-adhesion therapy. Virulence 4, 284–294. doi: 10.4161/viru.24606
Kudva, I. T., Krastins, B., Torres, A. G., Griffin, R. W., Sheng, H., Sarracino, D. A., et al. (2015). The Escherichia coli O157:H7 cattle immunoproteome includes outer membrane protein A (OmpA), a modulator of adherence to bovine rectoanal junction squamous epithelial (RSE) cells. Proteomics 15, 1829–1842. doi: 10.1002/pmic.201400432
Kunert, A., Losse, J., Gruszin, C., Huhn, M., Kaendler, K., Mikkat, S., et al. (2007). Immune evasion of the human pathogen Pseudomonas aeruginosa: elongation factor Tuf is a factor H and plasminogen binding protein. J. Immunol. 179, 2979–2988. doi: 10.4049/jimmunol.179.5.2979
Kunze, G., Zipfel, C., Robatzek, S., Niehaus, K., Boller, T., and Felix, G. (2004). The N terminus of bacterial elongation factor Tu elicits innate immunity in Arabidopsis plants. Plant Cell 16, 3496–3507. doi: 10.1105/tpc.104.026765
Kwon, S.-O., Gho, Y. S., Lee, J. C., and Kim, S. I. (2009). Proteome analysis of outer membrane vesicles from a clinical Acinetobacter baumannii isolate. FEMS Microbiol. Lett. 297, 150–156. doi: 10.1111/j.1574-6968.2009.01669.x
Lacombe, S., Rougon-Cardoso, A., Sherwood, E., Peeters, N., Dahlbeck, D., van Esse, H. P., et al. (2010). Interfamily transfer of a plant pattern-recognition receptor confers broad-spectrum bacterial resistance. Nat. Biotechnol. 28, 365–369. doi: 10.1038/nbt.1613
Lahteenmaki, K., Kuusela, P., and Korhonen, T. K. (2000). Plasminogen activation in degradation and penetration of extracellular matrices and basement membranes by invasive bacteria. Methods 21, 125–132. doi: 10.1006/meth.2000.0983
Lambris, J. D., Ricklin, D., and Geisbrecht, B. V. (2008). Complement evasion by human pathogens. Nat. Rev. Microbiol. 6, 132–142. doi: 10.1038/nrmicro1824
Laouami, S., Clair, G., Armengaud, J., and Duport, C. (2014). Proteomic evidences for rex regulation of metabolism in toxin-producing Bacillus cereus ATCC 14579. PLoS One 9:e107354. doi: 10.1371/journal.pone.0107354
Lathe, W. C., and Bork, P. (2001). Evolution of tuf genes: ancient duplication, differential loss and gene conversion. FEBS Lett. 502, 113–116. doi: 10.1016/s0014-5793(01)02639-4
Le Marechal, C., Peton, V., Ple, C., Vroland, C., Jardin, J., Briard-Bion, V., et al. (2015). Surface proteins of Propionibacterium freudenreichii are involved in its anti-inflammatory properties. J Proteomics 113, 447–461. doi: 10.1016/j.jprot.2014.07.018
Lecomte, X., Gagnaire, V., Briard-Bion, V., Jardin, J., Lortal, S., Dary, A., et al. (2014). The naturally competent strain Streptococcus thermophilus LMD-9 as a new tool to anchor heterologous proteins on the cell surface. Microb. Cell Fact. 13:82. doi: 10.1186/1475-2859-13-82
Lee, E.-Y., Bang, J. Y., Park, G. W., Choi, D.-S., Kang, J. S., Kim, H.-J., et al. (2007). Global proteomic profiling of native outer membrane vesicles derived from Escherichia coli. Proteomics 7, 3143–3153. doi: 10.1002/pmic.200700196
Lee, E.-Y., Choi, D.-Y., Kim, D.-K., Kim, J.-W., Park, J. O., Kim, S., et al. (2009). Gram-positive bacteria produce membrane vesicles: Proteomics-based characterization of Staphylococcus aureus-derived membrane vesicles. Proteomics 9, 5425–5436. doi: 10.1002/pmic.200900338
Lee, J. C., Lee, E. J., Lee, J. H., Jun, S. H., Choi, C. W., Kim, S. I., et al. (2012). Klebsiella pneumoniae secretes outer membrane vesicles that induce the innate immune response. FEMS Microbiol. Lett. 331, 17–24. doi: 10.1111/j.1574-6968.2012.02549.x
Levine, A., Vannier, F., Absalon, C., Kuhn, L., Jackson, P., Scrivener, E., et al. (2006). Analysis of the dynamic Bacillus subtilis Ser/Thr/Tyr phosphoproteome implicated in a wide variety of cellular processes. Proteomics 6, 2157–2173. doi: 10.1002/pmic.200500352
Li, X., Xing, J., Li, B., Wang, P., and Liu, J. (2012). Use of tuf as a target for sequence-based identification of Gram-positive cocci of the genus Enterococcus, Streptococcus, coagulase-negative Staphylococcus, and Lactococcus. Ann. Clin. Microbiol. Antimicrob. 11:31. doi: 10.1186/1476-0711-11-31
Liew, Y. K., Awang Hamat, R., van Belkum, A., Chong, P. P., and Neela, V. (2015). Comparative exoproteomics and host inflammatory response in Staphylococcus aureus skin and soft tissue infections, bacteremia, and subclinical colonization. Clin. Vaccine Immunol. 22, 593–603. doi: 10.1128/CVI.00493-14
Lippmann, C., Lindschau, C., Vijgenboom, E., Schroder, W., Bosch, L., and Erdmann, V. A. (1993). Prokaryotic elongation factor Tu is phosphorylated in vivo. J. Biol. Chem. 268, 601–607.
Liu, H., Cheng, Z., Song, W., Wu, W., and Zhou, Z. (2014). Immunoproteomic to analysis the pathogenicity factors in leukopenia caused by Klebsiella pneumonia bacteremia. PLoS One 9:e110011. doi: 10.1371/journal.pone.0110011
Liu, L., Wu, R., Zhang, J., Shang, N., and Li, P. (2017). D-ribose interferes with quorum sensing to inhibit biofilm formation of Lactobacillus paraplantarum L-ZS9. Front. Microbiol. 8:1860. doi: 10.3389/fmicb.2017.01860
López-Ochoa, J., Montes-García, J. F., Vázquez, C., Sánchez-Alonso, P., Pérez-Márquez, V. M., Blackall, P. J., et al. (2017). Gallibacterium elongation factor-Tu possesses amyloid-like protein characteristics, participates in cell adhesion, and is present in biofilms. J. Microbiol. 55, 745–752. doi: 10.1007/s12275-017-7077-0
Lu, F., Wang, H., Wang, S., Jiang, W., Shan, C., Li, B., et al. (2015). Enhancement of innate immune system in monocot rice by transferring the dicotyledonous elongation factor Tu receptor EFR. J. Integr. Plant Biol. 57, 641–652. doi: 10.1111/jipb.12306
Lylloff, J. E., Hansen, L. B., Jepsen, M., Sanggaard, K. W., Vester, J. K., Enghild, J. J., et al. (2016). Genomic and exoproteomic analyses of cold- and alkaline-adapted bacteria reveal an abundance of secreted subtilisin-like proteases. Microb. Biotechnol. 9, 245–256. doi: 10.1111/1751-7915.12343
Maddi, A., Haase, E., and Scannapieco, F. (2014). Mass spectrometric analysis of whole secretome and amylase-precipitated secretome proteins from Streptococcus gordonii. J. Proteomics Bioinform. 7, 287–295. doi: 10.4172/jpb.1000331
Madeira, J. P., Alpha-Bazin, B., Armengaud, J., and Duport, C. (2015). Time dynamics of the Bacillus cereus exoproteome are shaped by cellular oxidation. Front. Microbiol. 6:342. doi: 10.3389/fmicb.2015.00342
Mantyh, C. R., Gates, T. S., Zimmerman, R. P., Welton, M. L., Passaro, E. P., Vigna, S. R., et al. (1988). Receptor binding sites for substance P, but not substance K or neuromedin K, are expressed in high concentrations by arterioles, venules, and lymph nodules in surgical specimens obtained from patients with ulcerative colitis and Crohn disease. Proc. Natl. Acad. Sci. U.S.A. 85, 3235–3239. doi: 10.1073/pnas.85.9.3235
Massey, R. C., Kantzanou, M. N., Fowler, T., Day, N. P., Schofield, K., Wann, E. R., et al. (2001). Fibronectin-binding protein A of Staphylococcus aureus has multiple, substituting, binding regions that mediate adherence to fibronectin and invasion of endothelial cells. Cell Microbiol. 3, 839–851. doi: 10.1046/j.1462-5822.2001.00157.x
McLean, S. (2005). Do substance P and the NK1 receptor have a role in depression and anxiety? Curr. Pharm. Des. 11, 1529–1547. doi: 10.2174/1381612053764779
Mignard, S., and Flandrois, J. P. (2007). Identification of Mycobacterium using the EF-Tu encoding (tuf) gene and the tmRNA encoding (ssrA) gene. J. Med. Microbiol. 56, 1033–1041. doi: 10.1099/jmm.0.47105-0
Mijouin, L., Hillion, M., Ramdani, Y., Jaouen, T., Duclairoir-Poc, C., Follet-Gueye, M. L., et al. (2013). Effects of a skin neuropeptide (substance p) on cutaneous microflora. PLoS One 8:e78773. doi: 10.1371/journal.pone.0078773
Mikulik, K., and Zhulanova, E. (1995). Sequencing of the tuf1 gene and the phosphorylation pattern of EF-Tu1 during development and differentiation in Streptomyces collinus producing kirromycin. Biochem. Biophys. Res. Commun. 213, 454–461. doi: 10.1006/bbrc.1995.2153
Min, K.-W., Lee, S.-H., and Baek, S. J. (2016). Moonlighting proteins in cancer. Cancer Lett. 370, 108–116. doi: 10.1016/j.canlet.2015.09.022
Mirzaei, R., Saei, A., Torkashvand, F., Azarian, B., Jalili, A., Noorbakhsh, F., et al. (2016). Identification of proteins derived from Listeria monocytogenes inducing human dendritic cell maturation. Tumour Biol. 37, 10893–10907. doi: 10.1007/s13277-016-4933-1
Mishra, S., and Horswill, A. R. (2017). Heparin mimics extracellular DNA in binding to cell surface-localized proteins and promoting Staphylococcus aureus biofilm formation. mSphere 2:e00135-17. doi: 10.1128/mSphere.00135-17
Mohan, S., Hertweck, C., Dudda, A., Hammerschmidt, S., Skerka, C., Hallstrom, T., et al. (2014). Tuf of Streptococcus pneumoniae is a surface displayed human complement regulator binding protein. Mol. Immunol. 62, 249–264. doi: 10.1016/j.molimm.2014.06.029
Monahan, I. M., Betts, J., Banerjee, D. K., and Butcher, P. D. (2001). Differential expression of mycobacterial proteins following phagocytosis by macrophages. Microbiology 147, 459–471. doi: 10.1099/00221287-147-2-459
Montes-García, J. F., Chincoya Martinez, D. A., Vaca Pacheco, S., Vázquez Cruz, C., Sanchez Alonso, P., Xicohtencatl Cortes, J., et al. (2018). Identification of two adhesins of Actinobacillus seminis. Small Rumin. Res. 167, 100–103. doi: 10.1016/j.smallrumres.2018.08.013
Nagai, K., Domon, H., Maekawa, T., Hiyoshi, T., Tamura, H., Yonezawa, D., et al. (2019). Immunization with pneumococcal elongation factor Tu enhances serotype-independent protection against Streptococcus pneumoniae infection. Vaccine 37, 160–168. doi: 10.1016/j.vaccine.2018.11.015
Nascimento, R., Gouran, H., Chakraborty, S., Gillespie, H. W., Almeida-Souza, H. O., Tu, A., et al. (2016). Corrigendum: the type II secreted Lipase/Esterase LesA is a key virulence factor required for Xylella fastidiosa pathogenesis in grapevines. Sci. Rep. 6:21575. doi: 10.1038/srep21575
N’Diaye, A., Mijouin, L., Hillion, M., Diaz, S., Konto-Ghiorghi, Y., Percoco, G., et al. (2016). Effect of substance P in Staphylococcus aureus and Staphylococcus epidermidis virulence: implication for skin homeostasis. Front. Microbiol. 7:506. doi: 10.3389/fmicb.2016.00506
N’Diaye, A. R., Borrel, V., Racine, P.-J., Clamens, T., Depayras, S., Maillot, O., et al. (2019). Mechanism of action of the moonlighting protein EfTu as a substance P sensor in Bacillus cereus. Sci. Rep. 9:1304. doi: 10.1038/s41598-018-37506-6
Nega, M., Dube, L., Kull, M., Ziebandt, A. K., Ebner, P., Albrecht, D., et al. (2015). Secretome analysis revealed adaptive and non-adaptive responses of the Staphylococcus carnosus femB mutant. Proteomics 15, 1268–1279. doi: 10.1002/pmic.201400343
Newcombe, J., Mendum, T. A., Ren, C. P., and McFadden, J. (2014). Identification of the immunoproteome of the meningococcus by cell surface immunoprecipitation and MS. Microbiology 160, 429–438. doi: 10.1099/mic.0.071829-0
Nisole, S., Krust, B., Callebaut, C., Guichard, G., Muller, S., Briand, J. P., et al. (1999). The anti-HIV pseudopeptide HB-19 forms a complex with the cell-surface-expressed nucleolin independent of heparan sulfate proteoglycans. J. Biol. Chem. 274, 27875–27884. doi: 10.1074/jbc.274.39.27875
Nisole, S., Said, E. A., Mische, C., Prevost, M. C., Krust, B., Bouvet, P., et al. (2002). The anti-HIV pentameric pseudopeptide HB-19 binds the C-terminal end of nucleolin and prevents anchorage of virus particles in the plasma membrane of target cells. J. Biol. Chem. 277, 20877–20886. doi: 10.1074/jbc.M110024200
O’Connor, T. M., O’Connell, J., O’Brien, D. I., Goode, T., Bredin, C. P., and Shanahan, F. (2004). The role of substance P in inflammatory disease. J. Cell. Physiol. 201, 167–180. doi: 10.1002/jcp.20061
Olaya-Abril, A., Prados-Rosales, R., McConnell, M. J., Martín-Peña, R., González-Reyes, J. A., Jiménez-Munguía, I., et al. (2014). Characterization of protective extracellular membrane-derived vesicles produced by Streptococcus pneumoniae. J. Proteomics 106, 46–60. doi: 10.1016/j.jprot.2014.04.023
Omer, H., Alpha-Bazin, B., Brunet, J. L., Armengaud, J., and Duport, C. (2015). Proteomics identifies Bacillus cereus EntD as a pivotal protein for the production of numerous virulence factors. Front. Microbiol. 6:1004. doi: 10.3389/fmicb.2015.01004
Padilla-Reynaud, R., Simao-Beaunoir, A. M., Lerat, S., Bernards, M. A., and Beaulieu, C. (2015). Suberin regulates the production of cellulolytic enzymes in Streptomyces scabiei, the causal agent of potato common scab. Microbes Env. 30, 245–253. doi: 10.1264/jsme2.ME15034
Peng, Z., Vogel, R. F., Ehrmann, M. A., and Xiong, T. (2018). Identification and characterization of adhesion proteins in lactobacilli targeting actin as receptor. Mol. Cell. Probes 37, 60–63. doi: 10.1016/j.mcp.2017.08.002
Pérez-Cruz, C., Delgado, L., López-Iglesias, C., and Mercade, E. (2015). Outer-inner membrane vesicles naturally secreted by gram-negative pathogenic bacteria. PLoS One 10:e0116896. doi: 10.1371/journal.pone.0116896
Petit, F. M., Serres, C., and Auer, J. (2014). Moonlighting proteins in sperm-egg interactions. Biochem. Soc. Trans. 42, 1740–1743. doi: 10.1042/BST20140218
Peton, V., Bouchard, D. S., Almeida, S., Rault, L., Falentin, H., Jardin, J., et al. (2014). Fine-tuned characterization of Staphylococcus aureus Newbould 305, a strain associated with mild and chronic mastitis in bovines. Vet. Res. 45:106. doi: 10.1186/s13567-014-0106-7
Pierson, T., Matrakas, D., Taylor, Y. U., Manyam, G., Morozov, V. N., Zhou, W., et al. (2011). Proteomic characterization and functional analysis of outer membrane vesicles of Francisella novicida suggests possible role in virulence and use as a vaccine. J. Proteome Res. 10, 954–967. doi: 10.1021/pr1009756
Pinto, P. M., Chemale, G., de Castro, L. A., Costa, A. P. M., Kich, J. D., Vainstein, M. H., et al. (2007). Proteomic survey of the pathogenic Mycoplasma hyopneumoniae strain 7448 and identification of novel post-translationally modified and antigenic proteins. Vet. Microbiol. 121, 83–93. doi: 10.1016/j.vetmic.2006.11.018
Plikat, U., Voshol, H., Dangendorf, Y., Wiedmann, B., Devay, P., Muller, D., et al. (2007). From proteomics to systems biology of bacterial pathogens: approaches, tools, and applications. Proteomics 7, 992–1003. doi: 10.1002/pmic.200600925
Polekhina, G., Thirup, S., Kjeldgaard, M., Nissen, P., Lippmann, C., and Nyborg, J. (1996). Helix unwinding in the effector region of elongation factor EF-Tu-GDP. Structure 4, 1141–1151. doi: 10.1016/s0969-2126(96)00122-0
Pouttu, R., Puustinen, T., Virkola, R., Hacker, J., Klemm, P., and Korhonen, T. K. (1999). Amino acid residue Ala-62 in the FimH fimbrial adhesin is critical for the adhesiveness of meningitis-associated Escherichia coli to collagens. Mol. Microbiol. 31, 1747–1757. doi: 10.1046/j.1365-2958.1999.01311.x
Prados-Rosales, R., Baena, A., Martinez, L. R., Luque-Garcia, J., Kalscheuer, R., Veeraraghavan, U., et al. (2011). Mycobacteria release active membrane vesicles that modulate immune responses in a TLR2-dependent manner in mice. J. Clin. Invest. 121, 1471–1483. doi: 10.1172/JCI44261
Premkumar, L., Kurth, F., Duprez, W., Groftehauge, M. K., King, G. J., Halili, M. A., et al. (2014). Structure of the Acinetobacter baumannii dithiol oxidase DsbA bound to elongation factor EF-Tu reveals a novel protein interaction site. J. Biol. Chem. 289, 19869–19880. doi: 10.1074/jbc.M114.571737
Prezioso, S. M., Brown, N. E., and Goldberg, J. B. (2017). Elfamycins: inhibitors of elongation factor-Tu. Mol. Microbiol. 106, 22–34. doi: 10.1111/mmi.13750
Pribyl, T., Moche, M., Dreisbach, A., Bijlsma, J. J., Saleh, M., Abdullah, M. R., et al. (2014). Influence of impaired lipoprotein biogenesis on surface and exoproteome of Streptococcus pneumoniae. J. Proteome Res. 13, 650–667. doi: 10.1021/pr400768v
Pukatzki, S., Ma, A. T., Sturtevant, D., Krastins, B., Sarracino, D., Nelson, W. C., et al. (2006). Identification of a conserved bacterial protein secretion system in Vibrio cholerae using the Dictyostelium host model system. Proc. Natl. Acad. Sci. U.S.A. 103, 1528–1533. doi: 10.1073/pnas.0510322103
Ramiah, K., van Reenen, C. A., and Dicks, L. M. T. (2008). Surface-bound proteins of Lactobacillus plantarum 423 that contribute to adhesion of Caco-2 cells and their role in competitive exclusion and displacement of Clostridium sporogenes and Enterococcus faecalis. Res. Microbiol. 159, 470–475. doi: 10.1016/j.resmic.2008.06.002
Raymond, B. B. A., and Djordjevic, S. (2015). Exploitation of plasmin(ogen) by bacterial pathogens of veterinary significance. Vet. Microbiol. 178, 1–13. doi: 10.1016/j.vetmic.2015.04.008
Raymond, B. B. A., Jenkins, C., Seymour, L. M., Tacchi, J. L., Widjaja, M., Jarocki, V. M., et al. (2015). Proteolytic processing of the cilium adhesin MHJ_0194 (P123J) in Mycoplasma hyopneumoniae generates a functionally diverse array of cleavage fragments that bind multiple host molecules. Cell. Microbiol. 17, 425–444. doi: 10.1111/cmi.12377
Raymond, B. B. A., Tacchi, J. L., Jarocki, V. M., Minion, F. C., Padula, M. P., and Djordjevic, S. P. (2013). P159 from Mycoplasma hyopneumoniae binds porcine cilia and heparin and is cleaved in a manner akin to ectodomain shedding. J. Proteome Res. 12, 5891–5903. doi: 10.1021/pr400903s
Raymond, B. B. A., Turnbull, L., Jenkins, C., Madhkoor, R., Schleicher, I., Uphoff, C. C., et al. (2018). Mycoplasma hyopneumoniae resides intracellularly within porcine epithelial cells. Sci. Rep. 8:17697. doi: 10.1038/s41598-018-36054-3
Reales-Calderon, J. A., Corona, F., Monteoliva, L., Gil, C., and Martinez, J. L. (2015). Quantitative proteomics unravels that the post-transcriptional regulator Crc modulates the generation of vesicles and secreted virulence determinants of Pseudomonas aeruginosa. Data Brief 4, 450–453. doi: 10.1016/j.dib.2015.07.002
Resch, U., Tsatsaronis, J. A., Le Rhun, A., Stübiger, G., Rohde, M., Kasvandik, S., et al. (2016). A two-component regulatory system impacts extracellular membrane-derived vesicle production in group A Streptococcus. mBio 7:e00207-16. doi: 10.1128/mBio.00207-16
Robinson, M. W., Buchtmann, K. A., Jenkins, C., Tacchi, J. L., Raymond, B. B. A., To, J., et al. (2013). MHJ_0125 is an M42 glutamyl aminopeptidase that moonlights as a multifunctional adhesin on the surface of Mycoplasma hyopneumoniae. Open Biol. 3:130017. doi: 10.1098/rsob.130017
Rubiano-Labrador, C., Bland, C., Miotello, G., Armengaud, J., and Baena, S. (2015). Salt stress induced changes in the exoproteome of the halotolerant bacterium tistlia consotensis deciphered by proteogenomics. PLoS One 10:e0135065. doi: 10.1371/journal.pone.0135065
Ruyechan, W. T., and Olson, J. W. (1992). Surface lysine and tyrosine residues are required for interaction of the major herpes simplex virus type 1 DNA-binding protein with single-stranded DNA. J. Virol. 66, 6273–6279.
Rychli, K., Grunert, T., Ciolacu, L., Zaiser, A., Razzazi-Fazeli, E., Schmitz-Esser, S., et al. (2016). Exoproteome analysis reveals higher abundance of proteins linked to alkaline stress in persistent Listeria monocytogenes strains. Int. J. Food Microbiol. 218, 17–26. doi: 10.1016/j.ijfoodmicro.2015.11.002
Saad, N., Urdaci, M., Vignoles, C., Chaignepain, S., Tallon, R., Schmitter, J. M., et al. (2009). Lactobacillus plantarum 299v surface-bound GAPDH: a new insight into enzyme cell walls location. J. Microbiol. Biotechnol. 19, 1635–1643.
Sajid, A., Arora, G., Gupta, M., Singhal, A., Chakraborty, K., Nandicoori, V. K., et al. (2011). Interaction of Mycobacterium tuberculosis elongation factor Tu with GTP Is regulated by phosphorylation? J. Bacteriol. 193, 5347–5358. doi: 10.1128/JB.05469-11
Sanchez, B., Bressollier, P., and Urdaci, M. C. (2008). Exported proteins in probiotic bacteria: adhesion to intestinal surfaces, host immunomodulation and molecular cross-talking with the host. FEMS Immunol. Med. Microbiol. 54, 1–17. doi: 10.1111/j.1574-695X.2008.00454.x
Sanchez-Campillo, M., Bini, L., Comanducci, M., Raggiaschi, R., Marzocchi, B., Pallini, V., et al. (1999). Identification of immunoreactive proteins of Chlamydia trachomatis by Western blot analysis of a two-dimensional electrophoresis map with patient sera. Electrophoresis 20, 2269–2279. doi: 10.1002/(SICI)1522-2683(19990801)20:11<2269::AID-ELPS2269>3.0.CO;2-D
Sanderson-Smith, M. L., De Oliveira, D. M. P., Ranson, M., and McArthur, J. D. (2012). Bacterial plasminogen receptors: mediators of a multifaceted relationship. J. Biomed. Biotechnol. 2012:272148. doi: 10.1155/2012/272148
Sandig, H., McDonald, J., Gilmour, J., Arno, M., Lee, T. H., and Cousins, D. J. (2009). Fibronectin is a TH1-specific molecule in human subjects. J. Allergy Clin. Immunol. 124, 528.e5–535.e5. doi: 10.1016/j.jaci.2009.04.036
Sasikumar, A. N., Perez, W. B., and Kinzy, T. G. (2012). The many roles of the eukaryotic elongation factor 1 complex. Wiley Interdiscip. Rev. RNA 3, 543–555. doi: 10.1002/wrna.1118
Schaumburg, J., Diekmann, O., Hagendorff, P., Bergmann, S., Rohde, M., Hammerschmidt, S., et al. (2004). The cell wall subproteome of Listeria monocytogenes. Proteomics 4, 2991–3006. doi: 10.1002/pmic.200400928
Scherl, A., Francois, P., Bento, M., Deshusses, J. M., Charbonnier, Y., Converset, V., et al. (2005). Correlation of proteomic and transcriptomic profiles of Staphylococcus aureus during the post-exponential phase of growth. J. Microbiol. Methods 60, 247–257. doi: 10.1016/j.mimet.2004.09.017
Schoonbeek, H.-J., Wang, H.-H., Stefanato, F. L., Craze, M., Bowden, S., Wallington, E., et al. (2015). Arabidopsis EF-Tu receptor enhances bacterial disease resistance in transgenic wheat. New Phytol. 206, 606–613. doi: 10.1111/nph.13356
Schorr, W., Swandulla, D., and Zeilhofer, H. U. (1999). Mechanisms of IL-8-induced Ca2+ signaling in human neutrophil granulocytes. Eur. J. Immunol. 29, 897–904.
Schumacher, J., Waite, C. J., Bennett, M. H., Perez, M. F., Shethi, K., and Buck, M. (2014). Differential secretome analysis of Pseudomonas syringae pv tomato using gel-free MS proteomics. Front. Plant Sci. 5:242. doi: 10.3389/fpls.2014.00242
Schwartz, L., Spitsin, S. V., Meshki, J., Tuluc, F., Douglas, S. D., and Wolfe, J. H. (2013). Substance P enhances HIV-1 infection in human fetal brain cell cultures expressing full-length neurokinin-1 receptor. J. Neurovirol. 19, 219–227. doi: 10.1007/s13365-013-0166-x
Schwarz, S., Singh, P., Robertson, J. D., LeRoux, M., Skerrett, S. J., Goodlett, D. R., et al. (2014). VgrG-5 is a Burkholderia type VI secretion system-exported protein required for multinucleated giant cell formation and virulence. Infect. Immun. 82, 1445–1452. doi: 10.1128/IAI.01368-13
Secott, T. E., Lin, T. L., and Wu, C. C. (2002). Fibronectin attachment protein is necessary for efficient attachment and invasion of epithelial cells by Mycobacterium avium subsp. paratuberculosis. Infect Immun 70, 2670–2675.
Seeger, M., Osorio, G., and Jerez, C. A. (1996). Phosphorylation of GroEL, DnaK and other proteins from Thiobacillus ferrooxidans grown under different conditions. FEMS Microbiol. Lett. 138, 129–134.
Seymour, L. M., Deutscher, A. T., Jenkins, C., Kuit, T. A., Falconer, L., Minion, F. C., et al. (2010). A processed multidomain Mycoplasma hyopneumoniae adhesin binds fibronectin, plasminogen, and swine respiratory cilia. J. Biol. Chem. 285, 33971–33978. doi: 10.1074/jbc.M110.104463
Seymour, L. M., Jenkins, C., Deutscher, A. T., Raymond, B. B. A., Padula, M. P., Tacchi, J. L., et al. (2012). Mhp182 (P102) binds fibronectin and contributes to the recruitment of plasmin(ogen) to the Mycoplasma hyopneumoniae cell surface. Cell. Microbiol. 14, 81–94. doi: 10.1111/j.1462-5822.2011.01702.x
Shanks, R. M. Q., Donegan, N. P., Graber, M. L., Buckingham, S. E., Zegans, M. E., Cheung, A. L., et al. (2005). Heparin stimulates Staphylococcus aureus biofilm formation. Infect. Immun. 73, 4596–4606. doi: 10.1128/IAI.73.8.4596-4606.2005
Sharpe, S. W., Kuehn, M. J., and Mason, K. M. (2011). Elicitation of epithelial cell-derived immune effectors by outer membrane vesicles of nontypeable Haemophilus influenzae? Infect. Immun. 79, 4361–4369. doi: 10.1128/IAI.05332-11
Shin, J. H., Cho, E. J., Lee, J. Y., Yu, J. Y., and Kang, Y. H. (2009). Novel diagnostic algorithm using tuf gene amplification and restriction fragment length polymorphism is promising tool for identification of nontuberculous mycobacteria. J. Microbiol. Biotechnol. 19, 323–330.
Siljamaki, P., Varmanen, P., Kankainen, M., Pyorala, S., Karonen, T., Iivanainen, A., et al. (2014). Comparative proteome profiling of bovine and human Staphylococcus epidermidis strains for screening specifically expressed virulence and adaptation proteins. Proteomics 14, 1890–1894. doi: 10.1002/pmic.201300275
Sinnige, J. C., de Been, M., Zhou, M., Bonten, M. J., Willems, R. J., and Top, J. (2015). Growth condition-dependent cell surface proteome analysis of Enterococcus faecium. Proteomics 15, 3806–3814. doi: 10.1002/pmic.201500138
Slobin, L. I. (1980). The role of eucaryotic factor Tu in protein synthesis. The measurement of the elongation factor Tu content of rabbit reticulocytes and other mammalian cells by a sensitive radioimmunoassay. Eur. J. Biochem. 110, 555–563.
Snider, C. A., Voss, B. J., McDonald, W. H., and Cover, T. L. (2016). Growth phase-dependent composition of the Helicobacter pylori exoproteome. J. Proteomics 130, 94–107. doi: 10.1016/j.jprot.2015.08.025
Sokurenko, E. V., Chesnokova, V., Dykhuizen, D. E., Ofek, I., Wu, X.-R., Krogfelt, K. A., et al. (1998). Pathogenic adaptation of Escherichia coli by natural variation of the FimH adhesin. Proc. Natl. Acad. Sci. U.S.A. 95, 8922–8926. doi: 10.1073/pnas.95.15.8922
Sprinzl, M. (1994). Elongation factor Tu: a regulatory GTPase with an integrated effector. Trends Biochem. Sci. 19, 245–250.
Stewart, P. E., Carroll, J. A., Olano, L. R., Sturdevant, D. E., and Rosa, P. A. (2015). Multiple posttranslational modifications of Leptospira biflexa proteins as revealed by proteomic analysis. Appl. Environ. Microbiol. 82, 1183–1195. doi: 10.1128/AEM.03056-15
Stones, D. H., and Krachler, A. M. (2016). Against the tide: the role of bacterial adhesion in host colonization. Biochem. Soc. Trans. 44, 1571–1580. doi: 10.1042/BST20160186
Su, H. C., Hutchison, C. A., and Giddings, M. C. (2007). Mapping phosphoproteins in Mycoplasma genitalium and Mycoplasma pneumoniae. BMC Microbiol. 7:63. doi: 10.1186/1471-2180-7-63
Sun, X., Ge, F., Xiao, C. L., Yin, X. F., Ge, R., Zhang, L. H., et al. (2010). Phosphoproteomic analysis reveals the multiple roles of phosphorylation in pathogenic bacterium Streptococcus pneumoniae. J. Proteome Res. 9, 275–282. doi: 10.1021/pr900612v
Surve, M. V., Anil, A., Kamath, K. G., Bhutda, S., Sthanam, L. K., Pradhan, A., et al. (2016). Membrane vesicles of Group B Streptococcus disrupt feto-maternal barrier leading to preterm birth. PLoS Pathog. 12:e1005816. doi: 10.1371/journal.ppat.1005816
Tacchi, J. L., Raymond, B. B. A., Haynes, P. A., Berry, I. J., Widjaja, M., Bogema, D. R., et al. (2016). Post-translational processing targets functionally diverse proteins in Mycoplasma hyopneumoniae. Open Biol. 6:150210. doi: 10.1098/rsob.150210
Thofte, O., Su, Y.-C., Brant, M., Littorin, N., Duell, B. L., Alvarado, V., et al. (2018). EF-Tu From Non-typeable Haemophilus influenzae is an immunogenic surface-exposed protein targeted by bactericidal antibodies. Front. Immunol. 9:2910. doi: 10.3389/fimmu.2018.02910
Tiong, H. K., Hartson, S., and Muriana, P. M. (2015). Comparison of five methods for direct extraction of surface proteins from Listeria monocytogenes for proteomic analysis by orbitrap mass spectrometry. J. Microbiol. Methods 110, 54–60. doi: 10.1016/j.mimet.2015.01.004
Toyoda, M., Nakamura, M., Makino, T., Hino, T., Kagoura, M., and Morohashi, M. (2002). Nerve growth factor and substance P are useful plasma markers of disease activity in atopic dermatitis. Br. J. Dermatol. 147, 71–79.
Toyofuku, M., Nomura, N., and Eberl, L. (2019). Types and origins of bacterial membrane vesicles. Nat. Rev. Microbiol. 17, 13–24. doi: 10.1038/s41579-018-0112-2
Turk, B. (2006). Targeting proteases: successes, failures and future prospects. Nat. Rev. Drug Discov. 5, 785–799. doi: 10.1038/nrd2092
Turnbull, L., Toyofuku, M., Hynen, A. L., Kurosawa, M., Pessi, G., Petty, N. K., et al. (2016). Explosive cell lysis as a mechanism for the biogenesis of bacterial membrane vesicles and biofilms. Nat. Commun. 7:11220. doi: 10.1038/ncomms11220
Tuschil, A., Lam, C., Haslberger, A., and Lindley, I. (1992). Interleukin-8 stimulates calcium transients and promotes epidermal cell proliferation. J. Invest. Dermatol. 99, 294–298.
Van Noort, J. M. V., Kraal, B., Sinjorgo, K. M. C., Persoon, N. L. M., Johanns, E. S. D., and Bosch, L. (1986). Methylation in vivo of elongation factor EF-Tu at lysine-56 decreases the rate of tRNA-dependent GTP hydrolysis. Eur. J. Biochem. 160, 557–561. doi: 10.1111/j.1432-1033.1986.tb10074.x
van Teeseling, M. C. F., de Pedro, M. A., and Cava, F. (2017). Determinants of bacterial morphology: from fundamentals to possibilities for antimicrobial targeting. Front. Microbiol. 8:1264. doi: 10.3389/fmicb.2017.01264
Vande Voorde, J., Balzarini, J., and Liekens, S. (2014). Mycoplasmas and cancer: focus on nucleoside metabolism. EXCLI J 13, 300–322.
Vanden Bergh, P., Heller, M., Braga-Lagache, S., and Frey, J. (2013). The Aeromonas salmonicida subsp. salmonicida exoproteome: global analysis, moonlighting proteins and putative antigens for vaccination against furunculosis. Proteome Sci. 11, 1–12. doi: 10.1186/1477-5956-11-44
Verdrengh, M., and Tarkowski, A. (2008). The impact of substance P signalling on the development of experimental staphylococcal sepsis and arthritis. Scand. J. Immunol. 67, 253–259. doi: 10.1111/j.1365-3083.2007.02065.x
Viale, M. N., Echeverria-Valencia, G., Romasanta, P., Mon, M. L., Fernandez, M., Malchiodi, E., et al. (2014). Description of a novel adhesin of Mycobacterium avium subsp. paratuberculosis. Biomed. Res. Int. 2014:729618. doi: 10.1155/2014/729618
Vijgenboom, E., Woudt, L. P., Heinstra, P. W., Rietveld, K., van Haarlem, J., van Wezel, G. P., et al. (1994). Three tuf-like genes in the kirromycin producer Streptomyces ramocissimus. Microbiology 140(Pt 4), 983–998.
Vipond, C., Suker, J., Jones, C., Tang, C., Feavers, I. M., and Wheeler, J. X. (2006). Proteomic analysis of a meningococcal outer membrane vesicle vaccine prepared from the group B strain NZ98/254. Proteomics 6, 3400–3413. doi: 10.1002/pmic.200500821
Voros, A., Simm, R., Slamti, L., McKay, M. J., Hegna, I. K., Nielsen-LeRoux, C., et al. (2014). SecDF as part of the Sec-translocase facilitates efficient secretion of Bacillus cereus toxins and cell wall-associated proteins. PLoS One 9:e103326. doi: 10.1371/journal.pone.0103326
Waidner, B., Specht, M., Dempwolff, F., Haeberer, K., Schaetzle, S., Speth, V., et al. (2009). A Novel system of cytoskeletal elements in the human pathogen Helicobacter pylori. PLoS Pathog. 5:e1000669. doi: 10.1371/journal.ppat.1000669
Wakarchuk, W. W., Brochu, D., Foote, S., Robotham, A., Saxena, H., Erak, T., et al. (2016). Proteomic analysis of the secretome of Cellulomonas fimi ATCC 484 and Cellulomonas flavigena ATCC 482. PLoS One 11:e0151186. doi: 10.1371/journal.pone.0151186
Wang, G., Chen, H., Xia, Y., Cui, J., Gu, Z., Song, Y., et al. (2013a). How are the non-classically secreted bacterial proteins released into the extracellular milieu? Curr. Microbiol. 67, 688–695. doi: 10.1007/s00284-013-0422-6
Wang, G., Xia, Y., Cui, J., Gu, Z., Song, Y., Chen, Y. Q., et al. (2013b). The roles of moonlighting proteins in bacteria. Curr. Issues Mol. Biol. 16, 15–22.
Wang, G., Xia, Y., Song, X., and Ai, L. (2016). Common non-classically secreted bacterial proteins with experimental evidence. Curr. Microbiol. 72, 102–111. doi: 10.1007/s00284-015-0915-6
Wang, W., and Jeffery, C. J. (2016). An analysis of surface proteomics results reveals novel candidates for intracellular/surface moonlighting proteins in bacteria. Mol. Biosyst. 2, 1420–1431. doi: 10.1039/c5mb00550g
Wang, X., Thompson, C. D., Weidenmaier, C., and Lee, J. C. (2018). Release of Staphylococcus aureus extracellular vesicles and their application as a vaccine platform. Nat. Commun. 9:1379. doi: 10.1038/s41467-018-03847-z
Weissman, S. J., Moseley, S. L., Dykhuizen, D. E., and Sokurenko, E. V. (2003). Enterobacterial adhesins and the case for studying SNPs in bacteria. Trends Microbiol. 11, 115–117.
Whitney, J. C., Quentin, D., Sawai, S., LeRoux, M., Harding, B. N., Ledvina, H. E., et al. (2015). An interbacterial NAD(P)(+) glycohydrolase toxin requires elongation factor Tu for delivery to target cells. Cell 163, 607–619. doi: 10.1016/j.cell.2015.09.027
Widjaja, M., Berry, I. J., Pont, E. J., Padula, M. P., and Djordjevic, S. P. (2015). P40 and P90 from Mpn142 are targets of multiple processing events on the surface of Mycoplasma pneumoniae. Proteomes 3, 512–537. doi: 10.3390/proteomes3040512
Widjaja, M., Harvey, K. L., Hagemann, L., Berry, I. J., Jarocki, V. M., Raymond, B. B. A., et al. (2017). Elongation factor Tu is a multifunctional and processed moonlighting protein. Sci. Rep. 7:11227. doi: 10.1038/s41598-017-10644-z
Wilson, M. M., Anderson, D. E., and Bernstein, H. D. (2015). Analysis of the outer membrane proteome and secretome of Bacteroides fragilis reveals a multiplicity of secretion mechanisms. PLoS One 10:e0117732. doi: 10.1371/journal.pone.0117732
Wolf, H., Chinali, G., and Parmeggiani, A. (1974). Kirromycin, an inhibitor of protein biosynthesis that acts on elongation factor Tu. Proc. Natl. Acad. Sci. U.S.A. 71, 4910–4914.
Wolff, D. G., Castiblanco-Valencia, M. M., Abe, C. M., Monaris, D., Morais, Z. M., Souza, G. O., et al. (2013). Interaction of Leptospira elongation factor Tu with plasminogen and complement factor H: a metabolic leptospiral protein with moonlighting activities. PLoS One 8:e81818. doi: 10.1371/journal.pone.0081818
Xie, L., Wang, G., Yu, Z., Zhou, M., Li, Q., Huang, H., et al. (2016). Proteome-wide lysine glutarylation profiling of the Mycobacterium tuberculosis H37Rv. J. Proteome Res. 15, 1379–1385. doi: 10.1021/acs.jproteome.5b00917
Xie, L., Wang, X., Zeng, J., Zhou, M., Duan, X., Li, Q., et al. (2015). Proteome-wide lysine acetylation profiling of the human pathogen Mycobacterium tuberculosis. Int. J. Biochem. Cell Biol. 59, 193–202. doi: 10.1016/j.biocel.2014.11.010
Yamaguchi, M., Ikeda, R., Nishimura, M., and Kawamoto, S. (2010). Localization by scanning immunoelectron microscopy of triosephosphate isomerase, the molecules responsible for contact-mediated killing of Cryptococcus, on the surface of Staphylococcus. Microbiol. Immunol. 54, 368–370. doi: 10.1111/j.1348-0421.2010.00225.x
Yang, Q., Liu, J., Wang, K., Liu, T., Zhu, L., He, S., et al. (2018). Evaluation of immunogenicity and protective efficacy of the elongation factor Tu against Streptococcus agalactiae in tilapia. Aquaculture 492, 184–189. doi: 10.1016/j.aquaculture.2018.03.056
Yoon, J.-H., Ryu, J., and Baek, S. J. (2018). Moonlighting activity of secreted inflammation-regulatory proteins. Yonsei Med. J. 59, 463–469. doi: 10.3349/ymj.2018.59.4.463
Yu, Y., Wang, H., Wang, J., Feng, Z., Wu, M., Liu, B., et al. (2018). Elongation factor thermo unstable (EF-Tu) moonlights as an adhesin on the surface of Mycoplasma hyopneumoniae by binding to fibronectin. Front. Microbiol. 9:974. doi: 10.3389/fmicb.2018.00974
Yu, Y. T., and Snyder, L. (1994). Translation elongation factor Tu cleaved by a phage-exclusion system. Proc. Natl. Acad. Sci. U.S.A. 91, 802–806.
Zakharzhevskaya, N. B., Vanyushkina, A. A., Altukhov, I. A., Shavarda, A. L., Butenko, I. O., Rakitina, D. V., et al. (2017). Outer membrane vesicles secreted by pathogenic and nonpathogenic Bacteroides fragilis represent different metabolic activities. Sci. Rep. 7:5008. doi: 10.1038/s41598-017-05264-6
Zipfel, C. (2008). Pattern-recognition receptors in plant innate immunity. Curr. Opin. Immunol. 20, 10–16. doi: 10.1016/j.coi.2007.11.003
Keywords: EF-Tu, moonlighting, bacteria, adhesion, chaperone activities
Citation: Harvey KL, Jarocki VM, Charles IG and Djordjevic SP (2019) The Diverse Functional Roles of Elongation Factor Tu (EF-Tu) in Microbial Pathogenesis. Front. Microbiol. 10:2351. doi: 10.3389/fmicb.2019.02351
Received: 18 July 2019; Accepted: 27 September 2019;
Published: 24 October 2019.
Edited by:
Sunil Kumar Lal, Monash University Malaysia, MalaysiaReviewed by:
Jared Schrader, Wayne State University, United StatesCopyright © 2019 Harvey, Jarocki, Charles and Djordjevic. This is an open-access article distributed under the terms of the Creative Commons Attribution License (CC BY). The use, distribution or reproduction in other forums is permitted, provided the original author(s) and the copyright owner(s) are credited and that the original publication in this journal is cited, in accordance with accepted academic practice. No use, distribution or reproduction is permitted which does not comply with these terms.
*Correspondence: Steven P. Djordjevic, U3RldmVuLkRqb3JkamV2aWNAdXRzLmVkdS5hdQ==
Disclaimer: All claims expressed in this article are solely those of the authors and do not necessarily represent those of their affiliated organizations, or those of the publisher, the editors and the reviewers. Any product that may be evaluated in this article or claim that may be made by its manufacturer is not guaranteed or endorsed by the publisher.
Research integrity at Frontiers
Learn more about the work of our research integrity team to safeguard the quality of each article we publish.