- 1Key Laboratory of Plant Nutrition and Agri-environment in Northwest China, Ministry of Agriculture, College of Natural Resources and Environment, Northwest A&F University, Yangling, China
- 2School of Environment, The University of Auckland, Auckland, New Zealand
- 3School of Agriculture and Environment, The University of Western Australia, Crawley, WA, Australia
Soil microorganisms can be exposed to, and affected by, nanoparticles (NPs) that are either purposely released into the environment (e.g., nanoagrochemicals and NP-containing amendments) or reach soil as nanomaterial contaminants. It is crucial to evaluate the potential impact of NPs on key plant-microbe symbioses such as mycorrhizas and rhizobia, which are vital for health, functioning and sustainability of both natural and agricultural ecosystems. Our critical review of the literature indicates that NPs may have neutral, negative, or positive effects on development of mycorrhizal and rhizobial symbioses. The net effect of NPs on mycorrhizal development is driven by various factors including NPs type, speciation, size, concentration, fungal species, and soil physicochemical properties. As expected for potentially toxic substances, NPs concentration was found to be the most critical factor determining the toxicity of NPs against mycorrhizas, as even less toxic NPs such as ZnO NPs can be inhibitory at high concentrations, and highly toxic NPs such as Ag NPs can be stimulatory at low concentrations. Likewise, rhizobia show differential responses to NPs depending on the NPs concentration and the properties of NPs, rhizobia, and growth substrate, however, most rhizobial studies have been conducted in soil-less media, and the documented effects cannot be simply interpreted within soil systems in which complex interactions occur. Overall, most studies indicating adverse effects of NPs on mycorrhizas and rhizobia have been performed using either unrealistically high NP concentrations that are unlikely to occur in soil, or simple soil-less media (e.g., hydroponic cultures) that provide limited information about the processes occurring in the real environment/agrosystems. To safeguard these ecologically paramount associations, along with other ecotoxicological considerations, large-scale application of NPs in farming systems should be preceded by long-term field trials and requires an appropriate application rate and comprehensive (preferably case-specific) assessment of the context parameters i.e., the properties of NPs, microbial symbionts, and soil. Directions and priorities for future research are proposed based on the gaps and experimental restrictions identified.
Introduction
Nanotechnology is starting to affect virtually every single aspect of our life, nearly 60 years after Richard Feynman articulated the concept of nanotechnology in his seminal 1959 lecture entitled “There’s plenty of room at the bottom”(Feynman, 1960). Nanotechnology has the potential to truly revolutionize agricultural systems by offering diverse applications such as nanofertilizers; nanopesticides; NP-based plant growth stimulators such as TiO2 NPs, SiO2 NPs, and carbon nanotubes; nanocarriers for targeted delivery and controlled release of agrochemicals; nanosensors for detecting early symptoms of biotic/abiotic stresses in plants; improving soil quality; and instrumental implications in efficient genetic manipulation techniques (Pérez-de-Luque and Hermosín, 2013; Fraceto et al., 2016). Crop growth promotion and protection against stresses are the core benefits of nanoagrochemicals, which can also make significant contributions to precision agriculture by reducing the quantity of agrochemicals applied to crops, wastage, and environmental pollution (Fraceto et al., 2016), though the environmental impact might not necessarily be mitigated (Kah et al., 2018).
Large-scale and versatile applications of NPs have inevitably led to their increasing presence in the environment and consequent risks (Nowack, 2009), which are of utmost significance from health and environmental perspectives. Nanoparticles entry into soil can occur through application of nanoagrochemicals, NP-containing amendments (e.g., biosolids, sludge and manure), and contamination by industrial wastes, plant litter, animal feces, carcasses, exuviae, and atmospheric deposition (Nowack et al., 2012; Qiu, 2012; McKee and Filser, 2016; Bundschuh et al., 2018). Hence, development of NPs for specific applications needs to be accompanied by ecotoxicological and biosafety studies on the interactions between NPs and biological components of the potentially affected environments. In the context of global agriculture, the fundamental question is “whether nanotechnology revolution can help us overcome the major challenge that agriculture is facing today i.e., feeding the world’s booming population without compromising soil health and sustainability?” To partly address this challenge, we critically assess the research literature dealing with the effects of different NPs on key plant-microbe symbioses namely mycorrhizas and rhizobia, which are of major ecological significance and are vital for functionality, productivity, and resilience of terrestrial ecosystems.
Nanoparticles are atomic or molecular aggregates of which a single unit measures from 1 to <1000 nm (in at least one dimension), but they usually are sized between 1 and 100 nm (Buzea et al., 2007). Due to their ultrafine size, NPs possess unique properties that are normally different from their respective bulk counterparts, which include large specific surface area (very high surface-to-volume ratio), high surface energy, and quantum confinement (Auffan et al., 2009). Nanoagrochemicals, including nanofertilizers, nanopesticides, nanocarriers, and NP-based growth stimulators, that are potentially more efficient and less contaminant than their conventional analogs have been synthesized and researched worldwide (Fraceto et al., 2016; Dimkpa and Bindraban, 2018; Kah et al., 2018). Positive, neutral, or negative responses have been documented in plants exposed to NPs, driven by the NPs type, size, dose, application methods, the target plant species, and experimental conditions (Hatami et al., 2016; Kah et al., 2018). Antimicrobial properties of certain NPs (such as Ag NPs, TiO2 NPs, and ZnO NPs) against bacteria and fungi are well acknowledged. Nanoparticles can deform and damage fungal hyphae and bacterial cells (Figure 1). Hence, NPs presence in soil might lead to reduced diversity and function of soil microorganisms (Ge et al., 2011; Kumar et al., 2011; Navale et al., 2015; Asadishad et al., 2018). However, there are also studies indicating limited (Tong et al., 2007; Johansen et al., 2008), or even positive effects of NPs on soil microbial communities and functioning (He et al., 2011; Karunakaran et al., 2013), proposing that NP-microbe interactions are context-driven. The root-microbe symbioses reside in the rhizosphere where factors such as interactions among the soil biota, resource complexity and availability, and biophysical heterogeneities may influence the magnitude of the NP effects on both free-living and root-colonizing microbes (Saleem et al., 2015; Raffi and Husen, 2019). Figure 2 illustrates the NPs exposure to roots and root microbial symbionts.
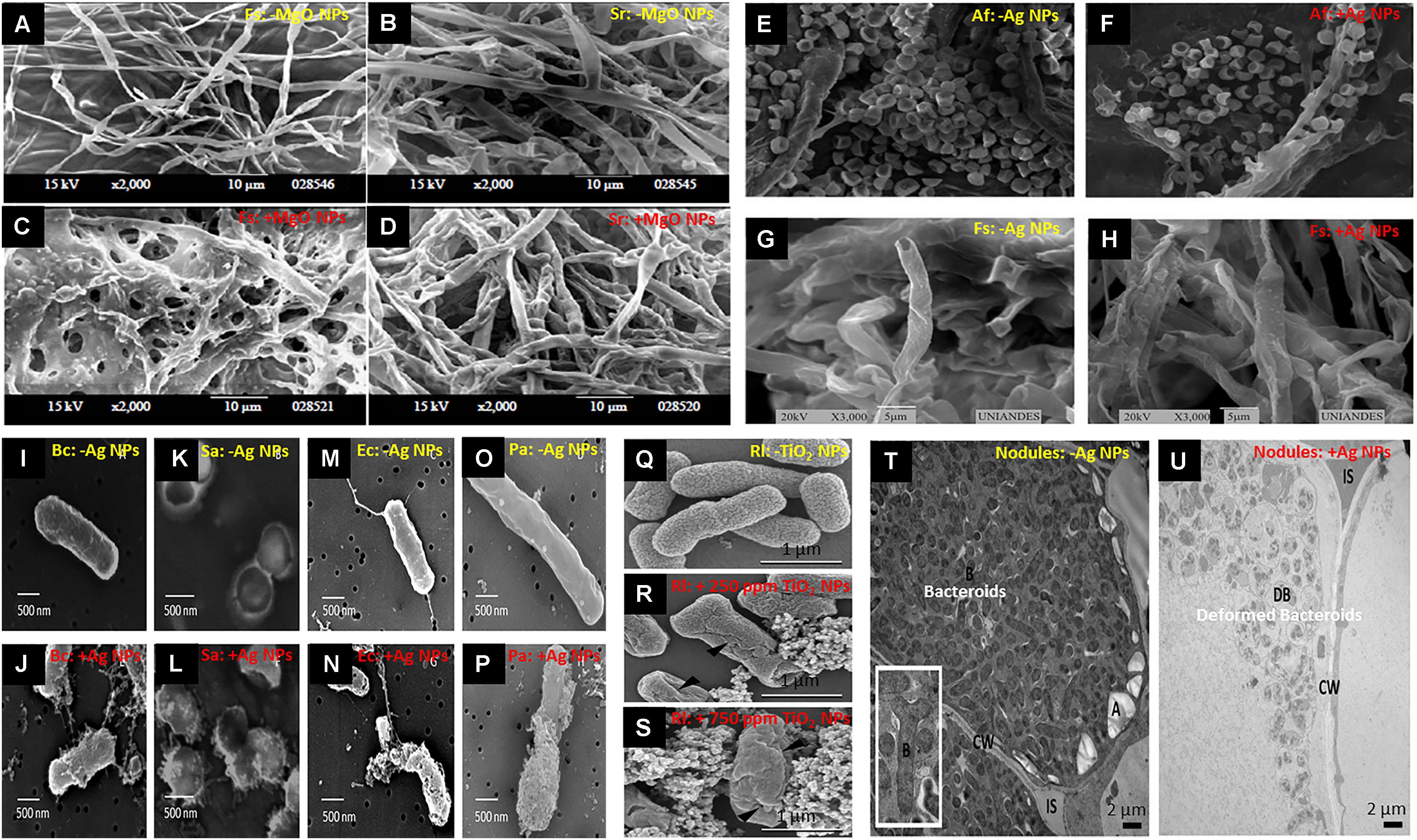
Figure 1. Deformation and damage in fungal hyphae and bacterial cells upon exposure to nanoparticles. (A–D) Scanning electron microscopy (SEM) of hyphae of Fusarium solani and Sclerotium rolfsii, untreated (A,B) or treated (C,D) with 100 ppm of magnesium oxide nanoparticles (MgO NPs), respectively. Some hyphae disintegrated and unusual bulges formed on the surface of fungal hyphae [adapted from El-Argawy et al. (2017)]. (E,F) SEM images of Aspergillus flavus before and after treatment with 50 ppm of silver nanoparticles (Ag NPs), showing dwindling of conidia; (G,H) SEM images of Fusarium solani before and after treatment with 50 ppm of Ag NPs, showing hyphal deformation [adapted from Villamizar-Gallardo et al. (2016)]. (I–P) SEM images of different bacteria, untreated or treated with bactericidal concentrations of Ag NPs: Bacillus cereus (I,J), Staphylococcus aureus (K,L), Escherichia coli (M,N), and Pseudomonas aeruginosa (O,P), indicating membrane damage [adapted from Gopinath et al. (2012)]. (Q–S) Cell surface structure of Rhizobium leguminosarum bv. viciae 3841 shown by SEM micrograph in untreated control, and following exposure to 250 or 750 mg L–1 of titanium oxide (TiO2) NPs, respectively. (▲) indicates cracks and wrinkles caused by TiO2 NPs [adapted from Fan et al. (2014)]. (T,U) Transmission electron micrographs (TEM) of the infected zone of untreated and Ag NP-treated nodules, respectively, indicating digestion of peribacteroid membrane and deformed bacteroids (DB) in nodules of treated plants [adapted from Abd-Alla et al. (2016)].
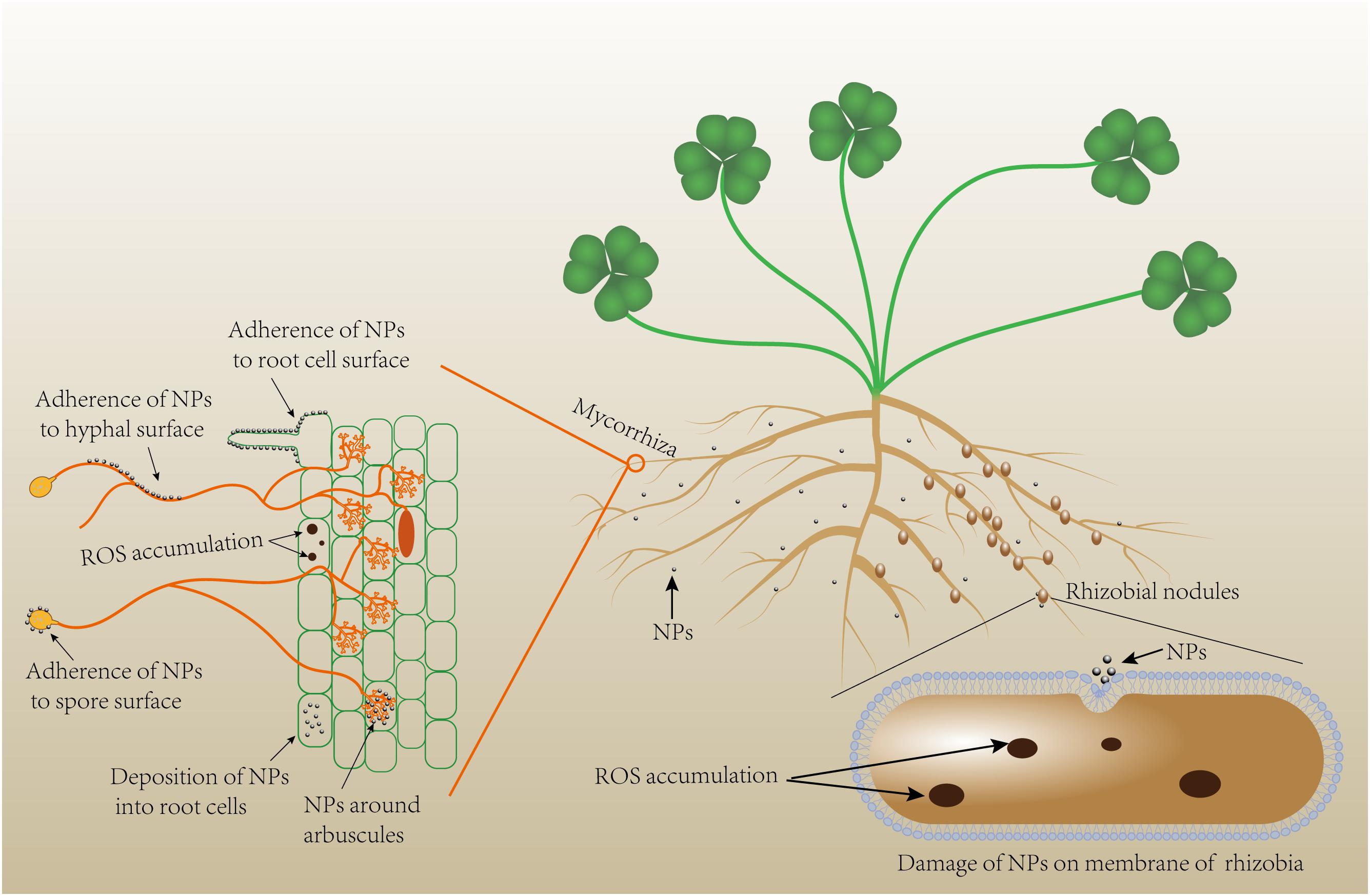
Figure 2. A theoretical demonstration of NPs exposure to roots, and root microbial symbionts. ROS, reactive oxygen species.
Mycorrhizal and rhizobial symbioses, arguably the most important symbioses on earth, are indispensable functional guilds of terrestrial ecosystems and continue to play a vital role in soil nutrient cycling, mineralization of organic matter, shaping plant and microbial communities, and ultimately safeguarding the functionality and resilience of the ecosystems (Finlay, 2008; van der Heijden et al., 2008). To date, a multitude of beneficial root-fungal symbioses have been described based on the properties of interface structures and the plant-fungus species involved (Smith and Read, 2008; Kariman et al., 2018). In the nanotechnology context, however, most research has primarily focused on the ubiquitous mycorrhiza i.e., arbuscular mycorrhiza, with a few studies partially dealing with other associations. Approximately, 80% of all vascular plant species establish arbuscular mycorrhizal (AM) symbiosis including bryophytes, pteridophytes, gymnosperms and angiosperms (Brundrett and Tedersoo, 2018) with fungi belonging to Glomeromycotina, a subphylum within Mucoromycota (Spatafora et al., 2016). Assorted benefits of AM symbiosis for host plants include access to the soil nutrients that are otherwise unavailable to roots (in particular, those with low mobility such as P and Zn), improved plant growth, and resistance against biotic/abiotic stresses such as pathogens, drought, salinity and heavy metals toxicity (Hildebrandt et al., 2007; Smith and Read, 2008; Miransari, 2010). Plant growth and reproduction can be gravely restricted by shortage of biologically active forms of N in soil. Certain plants have developed an efficient strategy to cope with N deficiency in soil. Plants from Fabaceae family enter into a symbiotic relationship with soil bacteria (including Rhizobium, Bradyrhizobium, Sinorhizobium, and Burkholderia), which converts the atmospheric N2 to ammonia, referred to as rhizobial symbiosis (Oldroyd et al., 2011). Rhizobial symbioses make significant contributions to N nutrition of Fabaceae (the third largest plant family), legume crops and non-legume crops grown in rotation, and tolerance against environmental stresses such as heavy metals, organic pollutants and acidity (Crews and Peoples, 2004; Teng et al., 2015; Ramirez et al., 2018). At the symbiotic interface, roots form nodules to accommodate N2-fixing rhizobia. To initiate the legume-rhizobia symbiosis, nodule organogenesis and bacterial infection must be coordinated spatially and temporally, and need to be preceded by recognition of the rhizobial signaling molecule (a decorated lipochitooligosaccharide) called NOD Factor (Oldroyd and Downie, 2008). Rhizobial symbioses are sustainable N providers for legumes, and non-legume crops involved in intercropping systems or sequential rotations, and it is crucial to monitor their performance when exposed to NP-containing agrochemicals, amendments, or pollutants.
The net effect of NPs on development of mycorrhizal and rhizobial symbioses appear to be highly context-driven. While several studies indicated detrimental effects of NPs on mycorrhizal and rhizobial associations (Huang et al., 2014; Abd-Alla et al., 2016; Jing et al., 2016; Wang et al., 2016; Noori et al., 2017), there are also reports on the stimulatory impact of NPs (Feng et al., 2013; Chen et al., 2017), suggesting potentials to be harnessed for these beneficial root-microbe symbioses. Figure 3 displays routes by which mycorrhizal fungi and rhizobia may encounter NPs in soil, and the possible context-dependent consequences. In this review, we critically evaluate the existing literature dealing with the effects of NPs on development of mycorrhizal and rhizobial symbioses, identify the potential drivers of the interactions, and direct the future research toward minimizing the risks and harnessing the potential opportunities.
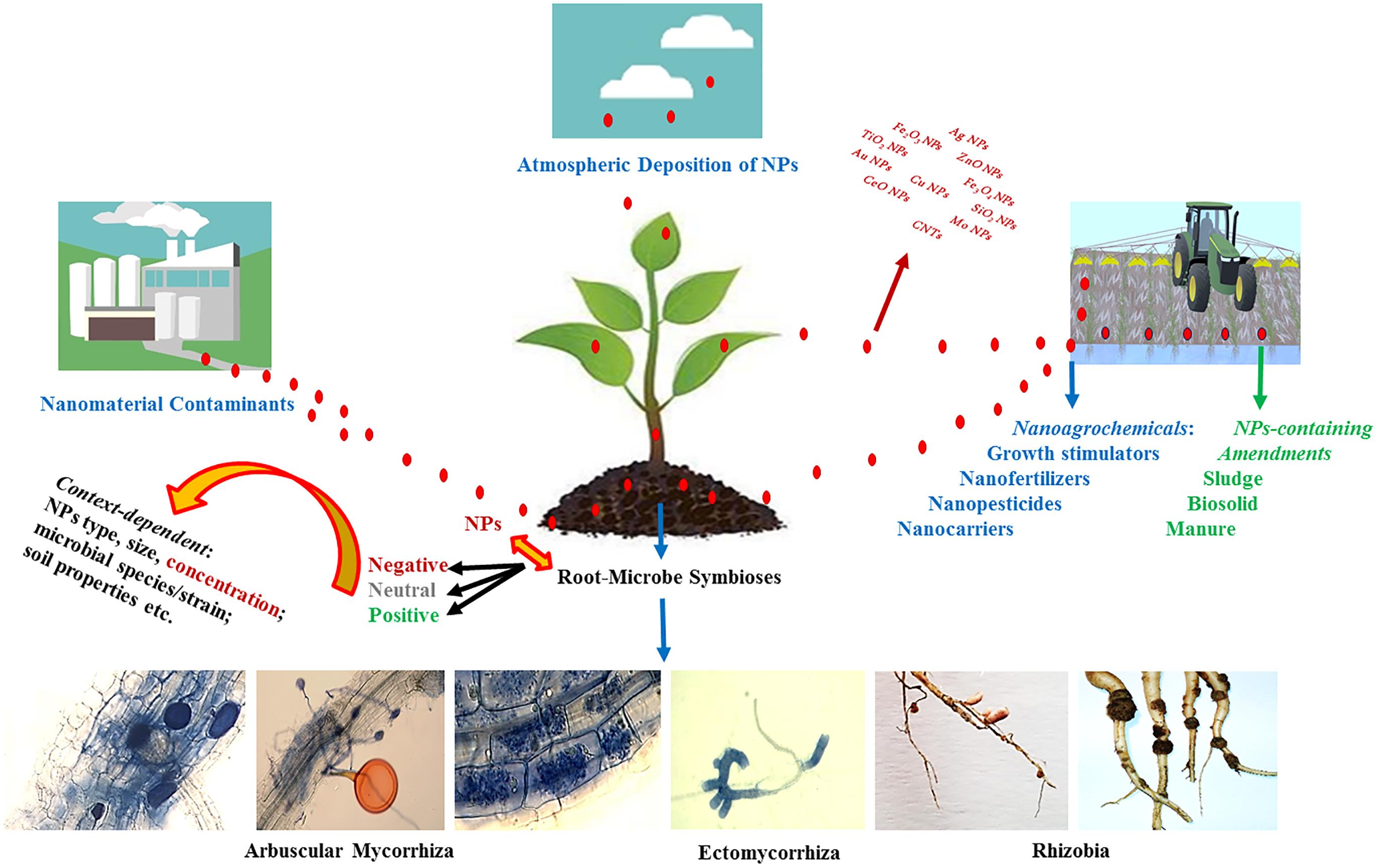
Figure 3. A schematic diagram showing the routes by which mycorrhizas and rhizobia may encounter nanoparticles (NPs) in soil, and the possible context-dependent consequences.
Effects of Nanoparticles on Mycorrhizal/Rhizobial Symbioses, and the Influencing Factors
While there is great variability in the responses of plant associations with AM fungi and rhizobia to NPs, there are also clear patterns in what drives this variability. Our detailed assessment of the experimental conditions suggests that the net outcome of NPs on mycorrhizal colonization or nodule development is highly context-dependent and vary according to the NP properties, concentration, fungal/bacterial species, and characteristics of the interaction matrix (mostly soil) in which mycorrhizas/rhizobia live and interact with plant roots. There are very few studies on potential impact of NPs on the functioning of these symbiotic associations, so here we focus on the structure (i.e., the proportion of root length colonized by mycorrhizal fungi, or nodule formation on roots). The existing evidence, however, suggests that a balanced focus on both structural and functional traits is required in studies dealing with toxicology of NPs against these beneficial root-microbe symbioses. For instance, exposure of soybean (Glycine max) plants to a range of CeO NP concentrations (10−100 g kg–1 soil) did not affect nodulation, however, N fixation was shut down at medium and high concentrations (50 and 100 g kg–1 soil) (Priester et al., 2012). This highlights the fact that rhizobial (and perhaps mycorrhizal) development might appear to be unaffected by NPs, but they might not necessarily be functional. Below, we explore the potential factors driving the effects of NPs on mycorrhizal/rhizobial development by stating the supporting evidence. The reviewed studies have been conducted in controlled environments (e.g., glasshouse, lab, etc.), hence, we also highlight the experimental considerations that would direct future research closer to real-world scenarios.
Nanoparticles Properties
Physicochemical properties of NPs (e.g., type, speciation, and size) strongly affect their impact on root mycorrhizal fungal colonization or nodulation. For example, Ag NPs seem to exhibit greater toxicity against mycorrhizas (Abd-Alla et al., 2016; Noori et al., 2017) compared with ZnO NPs (Li et al., 2015; Jing et al., 2016; Wang et al., 2016), considering their negative impact on root colonization at approximately 5−600 times lower concentrations in soil. Exposure to Fe2O3 NPs at 6 g L–1 did not affect nodulation in a symbiosis between pea and Rhizobium leguminosarum. 35 days after treatment, whereas the similar concentration and exposure time for ZnO NPs and TiO2 NPs led to negative effects on nodule development (Sarabia-Castillo and Fernández-Luqueño, 2016). CeO NPs application at 50 g kg–1 did not affect nodulation in soybean-Bradyrhizobium japonicum symbiosis, while the same concentration of ZnO NPs improved nodulation (Priester et al., 2012; Table 1). Bioavailability and potential effects of NPs on mycorrhizas/rhizobia might differ according to the NPs speciation and coating. For instance, PVP-Ag NPs (functionalized Ag NPs prepared via coating Ag NPs with polyvinyl pyrrolidone) were shown to reduce AM fungal colonization in tomato roots, while no significant effect was recorded for Ag2S NPs (silver sulfide NPs) at the same application rate of 100 mg kg–1 soil (Judy et al., 2015). Burke et al. (2015) used amine- and carboxylic acid-functionalized Fe3O4 NPs (carrying positive and negative surface charges, respectively) to investigate NP-rhizobia interactions, and observed that positively charged Fe3O4 NPs enhanced nodulation in soybean, compared to the negatively charged Fe3O4 NPs. This suggests that surface modification and coating of the NPs prior to exposure could affect the NPs toxicity against mycorrhizas/rhizobia, and therefore NP physicochemical properties can be modified to achieve a favorable outcome or avoid unwanted consequences in NP-mycorrhiza/rhizobia interactions.
Size of NPs was also found to influence NP-mycorrhiza interactions. Exposure to 2 nm-Ag NPs negatively affected root colonization in tomato, whereas a nil impact was observed for the larger Ag NPs of 15 nm at the same concentration of 12 mg kg–1 soil (Noori et al., 2017). Furthermore, TiO2 NP types differing in their size and crystalline structure were shown to move differently in soil, and the soil spiked with E171-TiO2 NPs (28 nm on average, consists of both anatase and rutile phases) had substantially elevated concentrations of Ti in the microcosm leachates compared with P25-TiO2 NPs (91 nm on average, consists of only anatase) (Klingenfuss, 2014), suggesting that NPs size and structure can potentially affect their bioavailability for soil microbes such as mycorrhizal fungi. Our knowledge is gravely limited on how the size of NPs might affect NP-rhizobia interactions, which warrants investigation. Chemical transformation of NPs can also possibly affect rhizobia, as partially or fully transformed NPs might possess different toxicity potential compared to the corresponding pristine materials (Liu et al., 2012; Reinsch et al., 2012).
Nanoparticles Concentration
Concentration of NPs in the soil is a critical factor driving the NP-mycorrhizas/ rhizobia interactions. Root AM fungal colonization was shown to be decreased (Li et al., 2015; Jing et al., 2016) or unaffected (Watts-Williams et al., 2014; Wang et al., 2016) following exposure to ZnO NPs. Lack of the toxic effects on AM fungal colonization in tomato (Watts-Williams et al., 2014) and maize (Wang et al., 2016) plants was probably due to low concentrations of ZnO NPs (25 and 400 mg kg–1 soil, respectively), whereas higher concentrations (500 to 3200 mg kg–1 soil) led to reduced colonization in maize (Li et al., 2015; Jing et al., 2016; Wang et al., 2016). While a low Ag NPs concentration (0.01 mg kg–1 soil) had no impact on AM fungal colonization in white clover (Trifolium repens), higher concentrations (0.1 and 1 mg kg–1 soil) significantly enhanced the extent of root colonization (Feng et al., 2013). A low concentration of FeO NPs (0.032 mg kg–1 soil) stimulated the AM fungal colonization in white clover while nil impact was observed at a 100-fold higher concentration (3.2 mg kg–1 soil).
Similar to the responses observed for mycorrhizal colonization, NPs concentration seems to play a role in rhizobia-NP interactions (Table 1), though there is limited experimental evidence. Increasing ZnO NPs concentration from 5 to 50 g kg–1 was accompanied by a shift from neutral to positive effects on nodulation in soybean plants inoculated with Bradyrhizobium japonicum (Priester et al., 2012). Nodulation and N2 fixation were not affected upon exposure to Kocide, a Cu-based fungicide containing a significant proportion of Cu NPs (Adeleye et al., 2014) at the recommended rate (1.7 mg kg–1) while a reduced nodulation was observed at higher application rates of 3.4 and 6.8 mg kg–1 (Baijukya and Semu, 1998). In negative NP-rhizobia interactions, the lack of a negative correlation between NPs concentration and nodule development (Table 1; Fan et al., 2014; Sarabia-Castillo and Fernández-Luqueño, 2016; Mohaddam et al., 2017) could possibly be because the employed concentration range was beyond the toxicity threshold of NPs under the respective experimental conditions (soil-less media) i.e., all the concentrations led to a negative impact on rhizobial symbioses.
Fungal/Bacterial Species
Different microbial taxa might exhibit various responses to NPs. Documented evidence indicates different mycorrhizal fungal taxa may exhibit various responses to NPs. The AM fungus Glomus caledonium was found to be more tolerant than G. versiforme against ZnO NPs toxicity based on extent of the negative impact of ZnO NPs on root colonization (Wang et al., 2016), which was attributed to higher tolerance of G. caledonium to heavy metals such as Zn, Cu, Pb, and Cd (Wang et al., 2007). Furthermore, although a DNA sequence analysis revealed five ectomycorrhizal (ECM)-forming genera on untreated roots of Bishop pine (Pinus muricata) including Laccaria, Thelephora, Rhizopogon, Tomentella, and Tuber, only Laccaria was found on roots of plants grown in soil spiked with 350 mg Ag NPs kg–1 (Sweet and Singleton, 2015).
There is currently not enough evidence regarding the sensitivity of different rhizobial species/strains against NPs, however, comparative studies between rhizobia and other bacteria suggest that differential responses might occur. For instance, the antibacterial action of ZnO NPs was demonstrated to be species-dependent as they exhibited bacteriostatic (preventing the growth of bacteria) effect on Pseudomonas putida (Gajjar et al., 2009), whereas the impact on the N2-fixing bacterium Sinorhizobium meliloti was of bactericidal (killing the bacteria) nature (Bandyopadhyay et al., 2012). Different strains of Escherichia coli were shown to be extremely sensitive or resistant to Ag NPs (Ashraf et al., 2014).
Substrate Properties
Substrate/soil physicochemical properties such as pH, ionic strength, clay and organic matter contents can influence the NPs mobility, dissolution, release of ions, agglomeration, aggregation, and potential effects on soil microorganisms (Klaine et al., 2008; Tourinho et al., 2012). However, scant attention has been paid to the possible role of soil properties in NP-mycorrhiza interactions. TiO2 NPs application (8 mg kg–1 soil) was shown to negatively affect AM fungal colonization in rice (Priyanka et al., 2017), which can be attributed to many factors such as binding of TiO2 NPs to roots (Priyanka et al., 2017), increases in root internal Ti concentration as Ti is highly toxic for root growth (Burke et al., 2014), or possibly the soil properties such as the soil cation exchange capacity (CEC) that can influence the Ti bioavailability for plants and microbes. The AM fungal colonization showed a recovering trend after 90 days of exposure to TiO2 compared to that of day 30, and 60 (Priyanka et al., 2017) that was ascribed to high mobility of TiO2 in soil (i.e., the sandy soil led to a loss of TiO2 via leaching) (Mura et al., 2013) and/or formation of new roots in the absence of a high concentration of TiO2 NPs.
Negative (Abd-Alla et al., 2016), neutral (Judy et al., 2015), or positive (Feng et al., 2013) effects on AM fungal colonization of plants exposed to a similar level (∼ 1 mg kg–1 soil) of Ag NPs, could possibly be related to the totally different soil types used for these studies in which NPs bioavailability and fate might substantially differ (Klaine et al., 2008). AM fungal communities in the rhizosphere of soybean plants were shown to be altered following exposure to TiO2 NPs (Burke et al., 2014). However, no change was reported for the AM fungal community composition inside the soybean roots (Burke et al., 2015). The latter was ascribed to low Ti concentration in the root (5−10 μ⋅Ti⋅g–1 root tissue) compared to soil (200−400 μg⋅Ti⋅g–1), presumably due to the high CEC of the soil used for the study. Mycorrhizal communities within the roots were, therefore protected against the TiO2 NPs toxicity, which was presumably influenced by the soil CEC. Within the soil (or growth medium), NPs can be chemically transformed and form coronae that could potentially affect NP kinetics and behavior (Lowry et al., 2012). Li et al. (2017) demonstrated that in paddy soils spiked with PVP-Ag NPs, the presence of soil organic matter enhanced Ag retention in the soil solids and decreased the dissolved Ag levels, whereas high redox potential led to reduced Ag sulfidation and increased the release of dissolved Ag. Accordingly, the natural chemical transformation of NPs in soil and possible impacts on mycorrhizas need to be considered.
Soil nutrient status may also play a role in NP-mycorrhiza interactions. While ZnO NPs were toxic to AM fungal colonization (by Funneliformis mosseae) in maize at 500 mg kg–1 soil, addition of the bulk counterpart (ZnSO4, 500 mg kg–1 soil) led to growth promoting and protective effects of the AM symbiosis against ZnO NPs toxicity (Li et al., 2015). These examples signify a highly context-dependent impact of NPs on mycorrhizas, and also suggest that favorable outcomes can be achieved by manipulation of the influencing factors, where applicable.
Although the extent of root colonization is a universally recognized index for mycorrhizal development, it does not necessarily reflect the actual mycorrhizal functioning and mycorrhiza-mediated benefits for host plants such as nutrient gain and tolerance against environmental stresses (Jakobsen, 1995; Smith et al., 2004, 2009; Kariman et al., 2014a,b, 2018). To better understand the impact of NPs on mycorrhizas, along with the quantitative measurement of mycorrhizal development (i.e., root colonization), the mycorrhizal benefits for NP-treated plants need to be experimentally investigated.
Likewise, the properties of substrate/growth medium can have a dramatic effect on NP-rhizobia interactions. As summarized in Table 1, many studies on NP-rhizobia interactions have been conducted in soil-less media. Although insight into nodulation responses to NPs can be gained from soil-less studies, such piecemeal evidence is inadequate to infer how NPs might affect development and functioning of legume-rhizobia symbioses in complex soil environments in which NPs bioavailability and impact on microbial communities could vary dramatically (Tong et al., 2007; Ge et al., 2011; Abd-Alla et al., 2016). For instance, soil application of ZnO NPs at 50 mg kg–1 resulted in a positive impact on nodule development in soybean plants associated with Bradyrhizobium japonicum (Priester et al., 2012), whereas comparably lower concentrations of ZnO NPs had severe adverse effects on nodule development in plants grown in soil-less media such as vermiculite (Sarabia-Castillo and Fernández-Luqueño, 2016), hydroponic cultures (Huang et al., 2014), and Jensen N free agar medium (Mohaddam et al., 2017). Most studies indicating negative effects of NPs on rhizobial development have been carried out in soil-less media in which the supplemental NPs are highly available (with enhanced NPs dissolution kinetics compared to soil) for both host plants and rhizobia (Fan et al., 2014; Huang et al., 2014; Sarabia-Castillo and Fernández-Luqueño, 2016; Mohaddam et al., 2017). Adverse effects on soil microbial community composition (more evidently on Rhizobials) were detected in a sandy loam soil spiked with CuO NPs, whereas limited effects were observed for a sandy clay loam soil, which contained a higher clay proportion (Abd-Alla et al., 2016). The decreased toxicity of NPs against soil microorganisms has been attributed to certain soil properties such as high clay content (Schlich and Hund-Rinke, 2015; Abd-Alla et al., 2016), alkaline pH (Shen et al., 2015), and low organic matter content, presumably due to the instability of the NP aggregates (Simonin et al., 2015).
Mechanisms Underlying the Effects of Nanoparticles on Mycorrhizal/Rhizobial Development
Our mechanistic understanding of NP-mycorrhiza/rhizobial interactions is limited (Figure 2). Some studies have attributed the negative impact of NPs on mycorrhizal colonization to antifungal activities of NPs including the release of metal ions such as Zn+2 (Wang et al., 2016) and Ag (Yin et al., 2011; Sweet and Singleton, 2015), binding of NPs to roots (Seeger et al., 2009; Priyanka et al., 2017), and increases in root internal NPs concentration and root growth inhibition (Burke et al., 2014; Priyanka et al., 2017). The adverse effects of NPs on mycorrhizal colonization could be directly due to the antifungal properties of NPs that include: adherence of NPs to the cell surface and physical damage to cell wall and membrane, increasing the membrane permeability, blocking the water channels, and cell death due to penetration and deposition of NPs into cells (Wang et al., 2014); cutting fungal structures and cell walls due to the sharp edges of NPs (Xie et al., 2016); inhibiting spore germination by forming NP-spore aggregates through van der Waals forces (Wang et al., 2014); accumulation of reactive oxygen species (ROS) via impairing the ROS-scavenging defense systems such as the cycle and regulation of glutathione (Akhavan and Ghaderi, 2012; Liu et al., 2016); release of ions from metal-based NPs (Kanhed et al., 2014; Hao et al., 2017; Zarzuela et al., 2017); and photocatalytic activities of certain NPs such as TiO2 (De Filpo et al., 2013).
Mycorrhizal colonization may decline following exposure of host plants to environmental stresses such as drought (Huang et al., 2017) and salinity (Wang et al., 2018) caused by a reduction in plant growth and photosynthesis, which is accompanied by reduced C allocation to root fungal symbionts. Other than the direct antifungal activities of NPs mentioned above, the decline in root colonization of NP-treated plants could be related to the possible negative effects of NPs on plant growth and fitness, where NPs exhibit phytotoxicity (Abd-Alla et al., 2016; Noori et al., 2017). Taken together, the negative effects of NPs on mycorrhizal development could be due to their direct (antifungal) and indirect (phytotoxic) effects.
Mechanisms behind the positive NP-mycorrhiza interactions are barely explored, and warrant investigation. Ag NPs were found to stimulate AM fungal colonization (Feng et al., 2013). An increase in AM fungal colonization has also been observed in plants under heavy metals stress (Vogel-Mikus et al., 2006). The stimulatory effect of Ag NPs (and heavy metals) on mycorrhizal colonization could be ascribed to additional C allocation to mycorrhizal fungi in order to benefit from their protective effects (Kiers et al., 2011).
Decline in rhizobial nodule development of plants exposed to NPs might be a direct consequence of the antibacterial activities of NPs. The antibacterial modes of action of NPs could generally be an outcome of oxidative stress induction (Gurunathan et al., 2012), metal ion release (Nagy et al., 2011; Huang et al., 2014), or non-oxidative mechanisms such as cell membrane damage (Fan et al., 2014; Leung et al., 2014), all of which can occur simultaneously. Morphological changes and cell surface damage in Rhizobium leguminosarum bv. viciae 3841 exposed to ZnO NPs was ascribed to adhesion of NPs onto cell walls, release of Zn2+ ions, and possible generation of ROS (Huang et al., 2014). Scanning electron microscopy (SEM) of cell surface structure of Rhizobium leguminosarum bv. viciae 3841 (Rlv 3841) showed that exposure to TiO2 NPs caused cracks and damage in cell membrane (Figures 1R,S; Fan et al., 2014). Transmission electron micrographs (TEM) of the infected zone of Ag NP-treated nodules indicated the digestion of peribacteroid membrane and presence of deformed/disintegrated bacteroids in nodules (Figure 1U; Abd-Alla et al., 2016). The decline in pea-Rhizobium leguminosarum symbiosis following exposure to TiO2 NPs was accompanied by morphological changes in the outer membrane of the rhizobia, changes in the composition of the cell wall polysaccharides of nodules, and a stress possibly caused by generation of hydroxyl radicals that could attach onto the nodules cell wall (Fan et al., 2014). These examples clearly emphasize on the antibacterial activities of NPs as a key mechanism behind the declined rhizobial symbiosis of the NP-treated plants. Nevertheless, as discussed about the mycorrhiza-NP interactions, the impact of NPs on host plants might also influence development of the rhizobial symbiosis. Environmental stresses such as drought can substantially decrease nodulation and N2 fixation as a consequence of C shortage, oxygen limitation, or feedback regulation by nitrogen accumulation (Serraj et al., 1999). Inhibition or retardation of the rhizobial symbiosis in pea plants exposed to ZnO NPs was shown to be linked with reductions in root and shoot growth (Huang et al., 2014; Sarabia-Castillo and Fernández-Luqueño, 2016) i.e., reduced growth and C allocation to microbial symbionts.
Limited evidence is available about the mechanisms underlying the stimulatory effects of NPs on nodule formation and development, but there have been some speculations. For instance, the enhanced nodule development in soybean plants exposed to positively charged Fe3O4 NPs was attributed to supply of Fe (Burke et al., 2015), which is essential for N2 fixing bacteria (Brear et al., 2013). In addition, nodule factor (nodf) and genistein (a major root-secreted isoflavone that induces the expression of Bradyrhizobium japonicum nod YABC operon) production were shown to be up-regulated by Fe3O4 NPs, which were suggested to be linked with the improved nodulation observed in the symbiosis between soybean plants and Bradyrhizobium japonicum (Ghalamboran, 2011).
Benefits of Mycorrhizal and Rhizobial Symbioses for Plants Exposed to Toxic Levels of Nanoparticles
Arbuscular mycorrhizal fungal colonization generally enhances the activities of antioxidant enzymes such as superoxide dismutase (SOD) under heavy metal stress, thus reducing the oxidative damage to biomolecules via scavenging the generated ROS (Azcon et al., 2009). Reduced bioavailability of heavy metals (accompanied by reduced phytotoxicity) in plants colonized by AM fungi have been attributed to the fungal capacity in increasing soil pH and producing glomalin-related soil proteins that can bind to metals (Wang et al., 2012).
Although few studies have specifically monitored the benefits of mycorrhizas for host plants under NP toxicity, several lines of evidence indicate their positive role. ZnO NPs were shown to be toxic to AM development in maize plants, however, the symbiosis could still alleviate the phytotoxic effects of ZnO NPs by decreasing Zn bioavailability (via hyphal sequestration) and accumulation in plant tissues, Zn translocation to shoots, and ROS generation, as well as improving mineral nutrition (Mg, in particular) and antioxidant capacity of host plants (Wang et al., 2016). Under elevated levels of Ag NPs (concentrations over 0.1 mg kg–1), the AM fungus G. caledonium ameliorated the NPs stress in white clover plants as compared with the uninoculated control (Feng et al., 2013). AM fungal colonization was almost completely inhibited in the presence of Ag NPs and Ag+ ions (at 100 mg kg–1 soil), while it was not affected by the Ag2S NPs treatment (Judy et al., 2015). The latter is a typical example of differential interaction of pristine vs. transformed NPs with mycorrhizas. The Ag2S NP-treated plants had higher root colonization but lower shoot Ag concentrations, proposing a mycorrhiza-mediated tolerance against Ag2S NPs via reduced Ag uptake relative to uninoculated plants. Likewise, mycorrhizal tomato plants exposed to 36 mg kg–1 of Ag NPs (2 nm) accumulated 14% less Ag in their shoot tissues compared to non-mycorrhizal plants (Noori et al., 2017), and the expression of potassium channel (KC), plasma membrane intrinsic protein (PIP), and a tonoplast membrane intrinsic protein (TIP) genes in mycorrhizal plants was lower than that of the non-mycorrhizal control. These findings demonstrated that mycorrhizal colonization can decrease Ag accumulation in Ag NP-exposed plants and moderate changes in expression level of membrane transport proteins that are possibly involved in Ag uptake. Judy et al. (2016) demonstrated that AM fungi did not play a significant role in the transfer of Au NPs to tomato plants. Au NPs accumulated at the rhizoplane of plants that developed a robust (about 35%) AM fungal colonization, suggesting a low toxicity of Au NPs for AM fungi and their possible protective effects on plants. Likewise, mycorrhizal tomato plants (76R) supplied with ZnO NPs were shown to accumulate lower Zn in their shoot compared to the non-mycorrhizal tomato mutant (rmc) (Watts-Williams et al., 2014). These examples suggest that mycorrhizas can possibly confer tolerance to host plants against NP toxicity via reduced NP uptake. Nevertheless, Whiteside et al. (2009) showed that AM fungi were actively involved in uptake and transfer of quantum dots (QDs) to roots of the annual bluegrass (Poa annua), which could be a specific response to QDs due to their very small size and unique properties (Al-Salim et al., 2011).
Other than conferring protective effects to host plants against NP toxicity, AM fungi were shown to alleviate the toxic effects of NPs on other soil biota. No significant changes in the relative abundance of Planctomycea, Sphingobacteria, Chloracidobacteria, Acidobacteria and Actinobacteria were found under a toxic concentration of Fe3O4 NPs in plants colonized by AM fungi, whereas these bacterial taxa were altered in non-mycorrhizal plants (Cao et al., 2016). Moreover, the relative abundance of Nitrospira (nitrite-oxidizing bacteria) and Anaerolineae (organic matter-decomposing bacteria) increased significantly by AM symbiosis compared to non-mycorrhizal plants under Fe3O4 NPs treatment, which can potentially contribute to N and C cycling in soil, respectively. Overall, the above-mentioned evidence suggests that mycorrhizal symbiosis has the capacity to protect host plants, beneficial microbes, and maintain soil function under NP toxicity. Mycorrhizas may also enhance the efficiency of nanofertilizers. Growth stimulation by the nanofertilizer Ca3(PO4)2 NPs in maize was shown to be improved by the AM fungus G. mosseae and/or the sebacinalean endophyte Serendipita indica (Rane et al., 2015).
There is no direct experimental evidence on whether rhizobial symbioses could aid plants to survive and thrive under toxic levels of NPs because most studies have considered untreated controls (i.e., with rhizobia, with or without NPs), but not uninoculated controls (i.e., without rhizobia, with or without NPs). However, rhizobia possess the biochemical and ecological capacity to decrease the risks associated with metals, metalloids, and organic pollutants in contaminated soils (Teng et al., 2015). For instance, the acidic-Al tolerant Burkholderia fungorum VTr35 strain was shown to induce tolerance to soybean plants against acid-Al stress conditions (Ramirez et al., 2018). Furthermore, rhizobia were shown to confer tolerance to host plants against heavy metals and oxidative stress through production of hydrogen (H2), which is a by-product of the symbiotic N2 fixation process and possess novel bioactive properties (Cui et al., 2013; Jin et al., 2013).
Do Nanoparticles Levels that are Toxic to Mycorrhizas and Rhizobia Occurring in Soil?
Manifold examples of negative effects of NPs on mycorrhizal and rhizobial symbioses were presented (Tables 1, 2) and discussed. Table 3 compares the mycorrhiza-toxic concentrations of NPs with plant-promoting concentrations of nanoagrochemicals (i.e., the potential soil application rate), along with NP concentrations reported from NP-polluted soils. It can be concluded that most of the adverse effects on mycorrhizas have been observed at NP concentrations exceeding those that can be realistically expected in agroecosystems and the natural environments. With respect to rhizobia, most studies dealing with negative rhizobia-NP interactions (Table 1) have been carried out in soil-less media in which NPs bioavailability and effects might substantially differ from soil systems. Hence, studies using more realistic NP concentrations and soils with different physicochemical properties (instead of vermiculite, agar-based media, or nutrient solutions) would be of paramount significance to further elucidate the inhibitory or stimulatory effects of NPs on mycorrhizas and rhizobia.
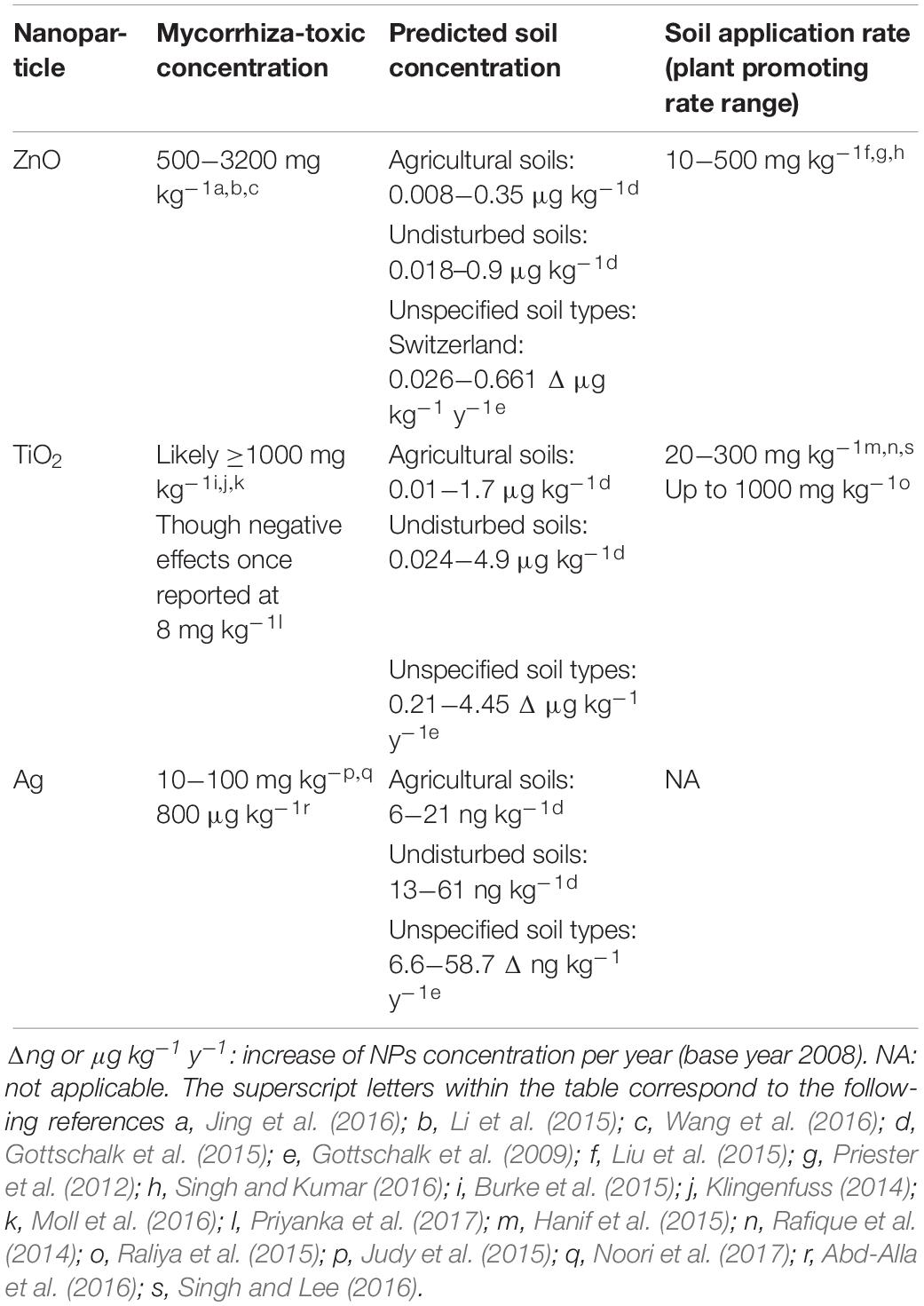
Table 3. Mycorrhiza-toxic concentrations of nanoparticles vs. the predicted soil nanoparticles concentrations and/or plant-promoting soil application rates of nanoagrochemicals.
However, environmentally relevant concentrations of NPs were also shown to strongly affect soil microbial communities, critical ecosystem services such as nutrient turnover, and greenhouse gas emissions (McKee and Filser, 2016). In general, application of any substance that is persistent and immobile in soil should be considered extremely cautiously. Nanoparticles are elements and thus do not breakdown in the environment. For instance, if NP concentrations are one order of magnitude apart, toxic levels may be reached in soil after 10 years of consecutive applications (once a year). Many NPs appear to be persistent and rather immobile in soil depending on the NPs and soil properties, and also incorporate into soil biota and plant tissues (Lin et al., 2010; McKee and Filser, 2016). Accordingly, the effects of repetitive applications of NPs on these key root-microbe symbioses need to be considered for nanoagrochemicals and NP-containing amendments (e.g., Ag NPs added through sludge application) via conducting spatial and temporal trials.
Historical use of Cu-based fungicides over decades has resulted in Cu accumulation in soils up to one order of magnitude higher concentration relative to the natural soils, which can cause adverse environmental impacts on soil fertility, organisms, and contaminate ground/surface water resources (Dumestre et al., 1999; Wightwick et al., 2010). Future research needs to address a fundamental question: “Could NPs accumulation in soil upon repetitive applications be an environmental concern similar to what that has been reported for Cu following long-term application of Cu-based fungicides?
Conclusion
Our in-depth evaluation of the literature shows that NPs may have negative, neutral or even positive effects on development of mycorrhizal and rhizobial symbioses. Most studies indicating adverse effects of NPs on mycorrhizas and rhizobia seem to have been performed using either unrealistically high NP concentrations that might not normally occur in soil, and/or irrelevant growth medium (mostly in rhizobial studies). A few reports also exist about the stimulatory impact of NPs on mycorrhizal/rhizobial development, suggesting that NPs might also be purposely used to promote these ecologically paramount associations.
The net effects of NPs on mycorrhizal colonization depend on various factors including NPs type, speciation, size, concentration, fungal species/strain, and the physicochemical properties of the soil or substrate. However, as expected for many potentially toxic compounds, NPs concentration was found to be the most crucial factor determining NP toxicity against mycorrhizas, as even less toxic types (e.g., ZnO NPs) could become toxic at high concentrations, or highly toxic types (e.g., Ag NPs) could be beneficial at low concentrations. Likewise, rhizobial responses to NPs are highly context-driven and depend on the concentration and properties of NPs, rhizobia, and the growth substrate. However, use of inappropriate growth media (e.g., hydroponic cultures) coupled with lack of nano-specific quality assurance and appropriate controls prevent us from drawing firm conclusions. Lack of nano-specific quality assurance is indeed a crucial issue. Nanoparticles need to be characterized as close as possible to the conditions of exposure. Many studies lacked adequate characterization, which prevents the elucidation of the mechanisms involved and comparison of the results of different studies. Thorough understanding of the key drivers of NP-mycorrhizas/rhizobia interactions would help us manage the possible consequences toward protection and/or promotion of these beneficial associations.
It is crucial to exploit the promising potential of nanotechnology to improve global food security and diminish the environmental footprint of modern agriculture. To maintain ecosystem functioning and resilience, research needs to be intensified on interactions between NPs and these key root-microbe symbioses. We have identified the future research priorities and experimental considerations as follows:
• Experiments should be conducted under more realistic experimental conditions. For instance, future studies should consider using agronomically/environmentally relevant NP concentrations in soils representing a range of properties (rather than soil-less systems).
• Adequate controls should be considered, including treatments with or without mycorrhizal fungi/rhizobia and NPs, and comparisons with non-nano analogs and currently used agrochemicals.
• A broader range of endpoints representing both structural development and functionality of the symbioses in NP-treated plants should be considered in the future studies.
• To investigate the functionality of mycorrhizal/rhizobial associations exposed to NPs, factors linked to viability and functionality of the symbioses need to be explored including the germination of mycorrhizal fungal spores, hyphal growth and function, multiplication of bacteroids in rhizobial nodules, and nutritional symbiotic benefits.
• Transformation and accumulation of NPs in soil over time and their long-term effects on mycorrhizal fungi and rhizobia need to be monitored, particularly for NPs that are intentionally and potentially repeatedly applied.
• Testing aged/transformed NPs relative to pristine NPs, and hence, considering the appropriate environmental media (soil) and time scale that allow these transformation processes to take place.
• Greater attention should be directed toward NP-based agrochemicals such as nanofertilizers, nanopesticides, plant growth stimulators (such as TiO2 NPs, SiO2 NPs, and carbon nanotubes) and organic nanocarriers (e.g., chitosan, graphene oxide, polymers etc.), because their concentrations in soil are more likely to cross the mycorrhizas/rhizobia-toxic threshold rather than those NPs originating from environmental pollutants such as Ag NPs.
• Most studies have focused on AM symbiosis and a crucial knowledge gap exists about interactions between NPs and other mycorrhizal associations. Bearing in mind the unavoidable release of NPs in natural ecosystems, it is essential to explore the interactions between NPs and the other key mycorrhizal associations such as ECM and orchid mycorrhizas.
• Whether or not mycorrhizal fungal and rhizobial strains can become resistant against NPs following repeated applications over time is unknown and deserves further investigations.
Author Contributions
HT conceptualized the manuscript. HT and KK wrote the manuscript. MK provided valuable input and insight on different components of the manuscript.
Funding
This work was supported by the National Key R&D Plan (SQ2017ZY060068).
Conflict of Interest Statement
The authors declare that the research was conducted in the absence of any commercial or financial relationships that could be construed as a potential conflict of interest.
Acknowledgments
We thank Mr. Zhiyuan Gao for checking the references.
References
Abd-Alla, M. H., Nafady, N. A., and Khalaf, D. M. (2016). Assessment of silver nanoparticles contamination on faba bean-Rhizobium leguminosarum bv. viciae-Glomus aggregatum symbiosis: implications for induction of autophagy process in root nodule. Agri. Ecosys. Environ. 218, 163–177. doi: 10.1016/j.agee.2015.11.022
Adeleye, A. S., Conway, J. R., Perez, T., Rutten, P., and Keller, A. A. (2014). Influence of extracellular polymeric substances on the long-term fate, dissolution, and speciation of copper-based nanoparticles. Environ. Sci. Technol. 48, 12561–12568. doi: 10.1021/es5033426
Akhavan, O., and Ghaderi, E. (2012). Escherichia coli bacteria reduce graphene oxide to bactericidal graphene in a self-limiting manner. Carbon 50, 1853–1860. doi: 10.1016/j.carbon.2011.12.035
Al-Salim, N., Barraclough, E., Burgess, E., Clothier, B., Deurer, M., Green, S., et al. (2011). Quantum dot transport in soil, plants, and insects. Sci. Total Environ. 409, 3237–3248. doi: 10.1016/j.scitotenv.2011.05.017
Asadishad, B., Chahal, S., Akbari, A., Cianciarelli, V., Azodi, M., Ghoshal, S., et al. (2018). Amendment of agricultural soil with metal nanoparticles: effects on soil enzyme activity and microbial community composition. Environ. Sci. Technol. 52, 1908–1918. doi: 10.1021/acs.est.7b05389
Ashraf, S., Chatha, M. A., Ejaz, W., Janjua, H. A., and Hussain, I. (2014). Lysozyme-coated silver nanoparticles for differentiating bacterial strains on the basis of antibacterial activity. Nano. Res. Lett. 9:565. doi: 10.1186/1556-276X-9-565
Auffan, M., Rose, J., Bottero, J. Y., Lowry, G. V., Jolivet, J. P., and Wiesner, M. R. (2009). Towards a definition of inorganic nanoparticles from an environmental, health and safety perspective. Nat. Nanotechnol. 4, 634–641. doi: 10.1038/nnano.2009.242
Azcon, R., Peralvarez, M. D., Biro, B., Roldan, A., and Ruiz-Lozano, J. M. (2009). Antioxidant activities and metal acquisition in mycorrhizal plants growing in a heavy-metal multicontaminated soil amended with treated lignocellulosic agrowaste. Appl. Soil Ecol. 41, 168–177. doi: 10.1016/j.apsoil.2008.10.008
Baijukya, F. P., and Semu, E. (1998). Effects of kocide 101 (R) on the bean (Phaseolus vulgaris L.)-Rhizobium symbiosis. Acta Agri. Scand. Sect. B Soil Plant Sci. 48, 175–183.
Bandyopadhyay, S., Peralta-Videa, J. R., Plascencia-Villa, G., Jose-Yacaman, M., and Gardea-Torresdey, J. L. (2012). Comparative toxicity assessment of CeO2 and ZnO nanoparticles towards Sinorhizobium meliloti, a symbiotic alfalfa associated bacterium: use of advanced microscopic and spectroscopic techniques. J. Hazard. Mater. 241, 379–386. doi: 10.1016/j.jhazmat.2012.09.056
Brear, E. M., Day, D. A., and Smith, P. M. C. (2013). Iron: an essential micronutrient for the legume-rhizobium symbiosis. Front. Plant Sci. 4:359. doi: 10.3389/fpls.2013.00359
Brundrett, M. C., and Tedersoo, L. (2018). Evolutionary history of mycorrhizal symbioses and global host plant diversity. New Phytol. 220, 1108–1115. doi: 10.1111/nph.14976
Bundschuh, M., Filser, J., Luderwald, S., Mckee, M. S., Metreveli, G., Schaumann, G. E., et al. (2018). Nanoparticles in the environment: where do we come from, where do we go to? Environ. Sci. Eur. 30:6. doi: 10.1186/s12302-018-0132-6
Burke, D. J., Pietrasiak, N., Situ, S. F., Abenojar, E. C., Porche, M., Kraj, P., et al. (2015). Iron oxide and titanium dioxide nanoparticle effects on plant performance and root associated microbes. Int. J. Mol. Sci. 16, 23630–23650. doi: 10.3390/ijms161023630
Burke, D. J., Zhu, S., Pablico-Lansigan, M. P., Hewins, C. R., and Samia, A. C. S. (2014). Titanium oxide nanoparticle effects on composition of soil microbial communities and plant performance. Biol. Fert. Soils 50, 1169–1173. doi: 10.1007/s00374-014-0938-3
Buzea, C., Pacheco, I. I., and Robbie, K. (2007). Nanomaterials and nanoparticles: sources and toxicity. Biointerphases 2:Mr17. doi: 10.1116/1.2815690
Cao, J. L., Feng, Y. Z., Lin, X. G., and Wang, J. H. (2016). Arbuscular mycorrhizal fungi alleviate the negative effects of iron oxide nanoparticles on bacterial community in rhizospheric soils. Front. Environ. Sci. 4:10. doi: 10.3389/fenvs.2016.00010
Chen, C., Tsyusko, O. V., McNear, D. H., Judy, J., Lewis, R. W., and Unrine, J. M. (2017). Effects of biosolids from a wastewater treatment plant receiving manufactured nanomaterials on Medicago truncatula and associated soil microbial communities at low nanomaterial concentrations. Sci. Total Environ. 609, 799–806. doi: 10.1016/j.scitotenv.2017.07.188
Crews, T. E., and Peoples, M. B. (2004). Legume versus fertilizer sources of nitrogen: ecological tradeoffs and human needs. Agri. Ecosyst. Environ. 102, 279–297. doi: 10.1016/j.agee.2003.09.018
Cui, W. T., Gao, C. Y., Fang, P., Lin, G. Q., and Shen, W. B. (2013). Alleviation of cadmium toxicity in Medicago sativa by hydrogen-rich water. J. Hazard. Mater. 260, 715–724. doi: 10.1016/j.jhazmat.2013.06.032
De Filpo, G., Palermo, A. M., Rachiele, F., and Nicoletta, F. P. (2013). Preventing fungal growth in wood by titanium dioxide nanoparticles. Int. Biodeterior. Biodegr. 85, 217–222. doi: 10.1016/j.ibiod.2013.07.007
Dimkpa, C. O., and Bindraban, P. S. (2018). Nanofertilizers: new products for the Industry? J. Agri. Food Chem. 66, 6462–6473. doi: 10.1021/acs.jafc.7b02150
Dumestre, A., Sauve, S., McBride, M., Baveye, P., and Berthelin, J. (1999). Copper speciation and microbial activity in long-term contaminated soils. Arch. Environ. Contam. Toxicol. 36, 124–131. doi: 10.1007/s002449900451
El-Argawy, E., Rahhal, M., El-Korany, K., Elshabrawy, E., and Eitahan, R. (2017). Efficacy of some nanoparticles to control dampping off and root rot of sugar beet in El-Behiera. Asian J. Plant Pathol. 11, 35–37.
Fan, R. M., Huang, Y. C., Grusak, M. A., Huang, C. P., and Sherrier, D. J. (2014). Effects of nano-TiO2 on the agronomically-relevant Rhizobium-legume symbiosis. Sci. Total Environ. 466, 503–512. doi: 10.1016/j.scitotenv.2013.07.032
Feng, Y. Z., Cui, X. C., He, S. Y., Dong, G., Chen, M., Wang, J. H., et al. (2013). The role of metal nanoparticles in influencing arbuscular mycorrhizal fungi effects on plant growth. Environ. Sci. Technol. 47, 9496–9504. doi: 10.1021/es402109n
Finlay, R. D. (2008). Ecological aspects of mycorrhizal symbiosis: with special emphasis on the functional diversity of interactions involving the extraradical mycelium. J. Exp. Bot. 59, 1115–1126. doi: 10.1093/jxb/ern059
Fraceto, L. F., Grillo, R., Medeiros, G. A. D., Scognamiglio, V., Rea, G., and Bartolucci, C. (2016). Nanotechnology in agriculture: which innovation potential does it have? Front. Environ. Sci. 4:20. doi: 10.3389/fenvs.2016.00020
Gajjar, P., Pettee, B., Britt, D. W., Huang, W. J., Johnson, W. P., and Anderson, A. J. (2009). Antimicrobial activities of commercial nanoparticles against an environmental soil microbe, Pseudomonas putida KT2440. J. Biol. Eng. 3:9. doi: 10.1186/1754-1611-3-9
Gatahi, D. M., Wanyika, H. N., Kavoo, A., Kihurani, A., and Ateka, E. M. (2016). Enhancement of bacterial wilt resistance and rhizosphere health in tomato using bionanocomposites. Int. J. Horticult. Sci. Technol. 3, 129–144.
Ge, Y. G., Schimel, J. P., and Holden, P. A. (2011). Evidence for negative effects of TiO2 and ZnO nanoparticles on soil bacterial communities. Environ. Sci. Technol. 45, 1659–1664. doi: 10.1021/es103040t
Ghalamboran, M. R. (2011). Symbiotic Nitrogen Fixation Enhancement due to Magnetite Nanoparticles. PhD, EngD and MSc thesis, Cranfield: Cranfield University.
Gopinath, V., MubarakAli, D., Priyadarshini, S., Priyadharsshini, N. M., Thajuddin, N., and Velusamy, P. (2012). Biosynthesis of silver nanoparticles from Tribulus terrestris and its antimicrobial activity: a novel biological approach. Coll. Surfaces B Biointer. 96, 69–74. doi: 10.1016/j.colsurfb.2012.03.023
Gottschalk, F., Lassen, C., Kjoelholt, J., Christensen, F., and Nowack, B. (2015). Modeling flows and concentrations of nine engineered nanomaterials in the danish environment. Int. J. Environ. Res. Public Health 12, 5581–5602. doi: 10.3390/ijerph120505581
Gottschalk, F., Sonderer, T., Scholz, R. W., and Nowack, B. (2009). Modeled environmental concentrations of engineered nanomaterials (TiO2, ZnO, Ag, CNT, Fullerenes) for different regions. Environ. Sci. Technol. 43, 9216–9222. doi: 10.1021/es9015553
Gurunathan, S., Han, J. W., Dayem, A. A., Eppakayala, V., and Kim, J. H. (2012). Oxidative stress-mediated antibacterial activity of graphene oxide and reduced graphene oxide in Pseudomonas aeruginosa. Int. J. Nanomed. 7, 5901–5914. doi: 10.2147/IJN.S37397
Hanif, H. U., Arshad, M., Ali, M. A., Ahmed, N., and Qazi, I. A. (2015). Phyto-availability of phosphorus to lactuca sativa in response to soil applied TiO2 nanoparticles. Pakistan J. Agricult. Sci. 52, 177–182.
Hao, Y., Cao, X. Q., Ma, C. X., Zhang, Z. T., Zhao, N., Ali, A., et al. (2017). Potential applications and antifungal activities of engineered nanomaterials against gray mold disease agent botrytis cinerea on rose petals. Front. Plant Sci. 8:1332. doi: 10.3389/fpls.2017.01332
Hatami, M., Kariman, K., and Ghorbanpour, M. (2016). Engineered nanomaterial-mediated changes in the metabolism of terrestrial plants. Sci. Total Environ. 571, 275–291. doi: 10.1016/j.scitotenv.2016.07.184
He, L. L., Liu, Y., Mustapha, A., and Lin, M. S. (2011). Antifungal activity of zinc oxide nanoparticles against Botrytis cinerea and Penicillium expansum. Microbiol. Res. 166, 207–215. doi: 10.1016/j.micres.2010.03.003
Hildebrandt, U., Regvar, M., and Bothe, H. (2007). Arbuscular mycorrhiza and heavy metal tolerance. Phytochemistry 68, 139–146. doi: 10.1016/j.phytochem.2006.09.023
Huang, Y. C., Fan, R., Grusak, M. A., Sherrier, J. D., and Huang, C. P. (2014). Effects of nano-ZnO on the agronomically relevant Rhizobium-legume symbiosis. Sci. Total Environ. 497, 78–90. doi: 10.1016/j.scitotenv.2014.07.100
Huang, Y. M., Zou, Y. N., and Wu, Q. S. (2017). Alleviation of drought stress by mycorrhizas is related to increased root H2O2 efflux in trifoliate orange. Sci. Rep. 7:42335. doi: 10.1038/srep42335
Jin, Q. J., Zhu, K. K., Cui, W. T., Xie, Y. J., Han, B., and Shen, W. B. (2013). Hydrogen gas acts as a novel bioactive molecule in enhancing plant tolerance to paraquat-induced oxidative stress via the modulation of heme oxygenase-1 signalling system. Plant Cell Environ. 36, 956–969. doi: 10.1111/pce.12029
Jing, X. X., Su, Z. Z., Xing, H. E., Wang, F. Y., Shi, Z. Y., and Liu, X. Q. (2016). Biological effects of ZnO nanoparticles as influenced by arbuscular mycorrhizal inoculation and phosphorus fertilization. Environ. Sci. 37, 3208–3215. doi: 10.13277/j.hjkx.2016.08.049
Johansen, A., Pedersen, A. L., Jensen, K. A., Karlson, U., Hansen, B. M., Scott-Fordsmand, J. J., et al. (2008). Effects of C60 fullerene nanoparticles on soil bacteria and protozoans. Environ. Toxicol. Chem. 27, 1895–1903.
Judy, J. D., Kirby, J. K., Creamer, C., McLaughlin, M. J., Fiebiger, C., Wright, C., et al. (2015). Effects of silver sulfide nanomaterials on mycorrhizal colonization of tomato plants and soil microbial communities in biosolid-amended soil. Environ. Pollut. 206, 256–263. doi: 10.1016/j.envpol.2015.07.002
Judy, J. D., Kirby, J. K., McLaughlin, M. J., Cavagnaro, T., and Bertsch, P. M. (2016). Gold nanomaterial uptake from soil is not increased by arbuscular mycorrhizal colonization of Solanum lycopersicum (Tomato). Nanomaterials 6:68. doi: 10.3390/nano6040068
Kah, M., Kookana, R. S., Gogos, A., and Bucheli, T. D. (2018). A critical evaluation of nanopesticides and nanofertilizers against their conventional analogues. Nat. Nanotechnol. 13, 677–684. doi: 10.1038/s41565-018-0131-1
Kanhed, P., Birla, S., Gaikwad, S., Gade, A., Seabra, A. B., Rubilar, O., et al. (2014). In vitro antifungal efficacy of copper nanoparticles against selected crop pathogenic fungi. Mat. Lett. 115, 13–17. doi: 10.1016/j.matlet.2013.10.011
Kariman, K., Barker, S. J., Finnegan, P. M., and Tibbett, M. (2014a). Ecto-and arbuscular mycorrhizal symbiosis can induce tolerance to toxic pulses of phosphorus in jarrah (Eucalyptus marginata) seedlings. Mycorrhiza 24, 501–509. doi: 10.1007/s00572-014-0567-6
Kariman, K., Barker, S. J., Jost, R., Finnegan, P. M., and Tibbett, M. (2014b). A novel plant-fungus symbiosis benefits the host without forming mycorrhizal structures. New Phytol. 201, 1413–1422. doi: 10.1111/nph.12600
Kariman, K., Barker, S. J., and Tibbett, M. (2018). Structural plasticity in root-fungal symbioses: diverse interactions lead to improved plant fitness. PeerJ 6:e6030. doi: 10.7717/peerj.6030
Karunakaran, G., Suriyaprabha, R., Manivasakan, P., Yuvakkumar, R., Rajendran, V., Prabu, P., et al. (2013). Effect of nanosilica and silicon sources on plant growth promoting rhizobacteria, soil nutrients and maize seed germination. Iet Nanobiotechnol. 7, 70–77. doi: 10.1049/iet-nbt.2012.0048
Kiers, E. T., Duhamel, M., Beesetty, Y., Mensah, J. A., Franken, O., Verbruggen, E., et al. (2011). Reciprocal rewards stabilize cooperation in the mycorrhizal symbiosis. Science 333, 880–882. doi: 10.1126/science.1208473
Klaine, S. J., Alvarez, P. J. J., Batley, G. E., Fernandes, T. F., Handy, R. D., Lyon, D. Y., et al. (2008). Nanomaterials in the environment: behavior, fate, bioavailability, and effects. Environ. Toxicol. Chem. 27, 1825–1851.
Klingenfuss, F. (2014). Testing of TiO2 Nanoparticles on Wheat and Microorganisms in a Soil Microcosm. Gothenburg: University of Gothenburg.
Kumar, N., Shah, V., and Walker, V. K. (2011). Perturbation of an arctic soil microbial community by metal nanoparticles. J. Hazard. Mater. 190, 816–822. doi: 10.1016/j.jhazmat.2011.04.005
Leung, Y. H., Ng, A. M. C., Xu, X. Y., Shen, Z. Y., Gethings, L. A., Wong, M. T., et al. (2014). Mechanisms of antibacterial activity of MgO: non-ROS mediated toxicity of MgO nanoparticles towards escherichia coli. Small 10, 1171–1183. doi: 10.1002/smll.201302434
Li, M., Wang, P., Dang, F., and Zhou, D. M. (2017). The transformation and fate of silver nanoparticles in paddy soil: effects of soil organic matter and redox conditions. Environ. Sci. Nano 4, 919–928. doi: 10.1039/c6en00682e
Li, S., Liu, X. Q., Wang, F. Y., and Miao, Y. F. (2015). Effects of ZnO nanoparticles, ZnSO4 and arbuscular mycorrhizal fungus on the growth of maize. Environ. Sci. 36, 4615–4622.
Lin, D. H., Tian, X. L., Wu, F. C., and Xing, B. S. (2010). Fate and transport of engineered nanomaterials in the environment. J. Environ. Qual. 39, 1896–1908.
Liu, J. Y., Wang, Z. Y., Liu, F. D., Kane, A. B., and Hurt, R. H. (2012). Chemical transformations of nanosilver in biological environments. ACS Nano. 6, 9887–9899. doi: 10.1021/nn303449n
Liu, X. Q., Wang, F. Y., Shi, Z. Y., Tong, R. J., and Shi, X. J. (2015). Bioavailability of Zn in ZnO nanoparticle-spiked soil and the implications to maize plants. J. Nanopart. Res. 17:175.
Liu, Z., Zhang, M., Han, X. Y., Xu, H. M., Zhang, B., Yu, Q. L., et al. (2016). TiO2 nanoparticles cause cell damage independent of apoptosis and autophagy by impairing the ROS-scavenging system in Pichia pastoris. Chem. Biol. Interact. 252, 9–18. doi: 10.1016/j.cbi.2016.03.029
Lowry, G. V., Gregory, K. B., Apte, S. C., and Lead, J. R. (2012). Transformations of nanomaterials in the environment. Environ. Sci. Technol. 46, 6893–6899. doi: 10.1021/es300839e
McKee, M. S., and Filser, J. (2016). Impacts of metal-based engineered nanomaterials on soil communities. Environ. Sci. Nano. 3, 506–533. doi: 10.1039/c6en00007j
Miransari, M. (2010). Contribution of arbuscular mycorrhizal symbiosis to plant growth under different types of soil stress. Plant Biol. 12, 563–569. doi: 10.1111/j.1438-8677.2009.00308.x
Mohaddam, M. N., Sabzevar, A. H., and Mortazaei, Z. (2017). Impact of ZnO and silver nanoparticles on legume-sinorhizobium symbiosis. Adv. Stud. Biol. 9, 83–90. doi: 10.12988/asb.2017.712
Moll, J., Gogos, A., Bucheli, T. D., Widmer, F., and van der Heijden, M. G. A. (2016). Effect of nanoparticles on red clover and its symbiotic microorganisms. J. Nanobiotechnol. 14:36. doi: 10.1186/s12951-016-0188-7
Mura, S., Seddaiu, G., Bacchini, F., Roggero, P. P., and Greppi, G. F. (2013). Advances of nanotechnology in agro-environmental studies. Italian J. Agron. 8, 127–140.
Nagy, A., Harrison, A., Sabbani, S., Munson, R. S., Dutta, P. K., and Waldman, W. J. (2011). Silver nanoparticles embedded in zeolite membranes: release of silver ions and mechanism of antibacterial action. Int. J. Nanomed. 6, 1833–1852. doi: 10.2147/IJN.S24019
Navale, G. R., Thripuranthaka, K., Late, D. J., and Shinde, S. S. (2015). Antimicrobial activity of ZnO nanoparticles against pathogenic bacteria and fungi. JSM Nanotechnol. Nanomed. 3:1033.
Noori, A., White, J. C., and Newman, L. A. (2017). Mycorrhizal fungi influence on silver uptake and membrane protein gene expression following silver nanoparticle exposure. J. Nanopart. Res. 19:66.
Nowack, B. (2009). The behavior and effects of nanoparticles in the environment. Environ. Pollut. 157, 1063–1064. doi: 10.1016/j.envpol.2008.12.019
Nowack, B., Ranville, J. F., Diamond, S., Gallego-Urrea, J. A., Metcalfe, C., Rose, J., et al. (2012). Potential scenarios for nanomaterial release and subsequent alteration in the environment. Environ. Toxicol. Chem. 31, 50–59. doi: 10.1002/etc.726
Oldroyd, G. E. D., and Downie, J. M. (2008). Coordinating nodule morphogenesis with rhizobial infection in legumes. Annu. Rev. Plant Biol. 59, 519–546. doi: 10.1146/annurev.arplant.59.032607.092839
Oldroyd, G. E. D., Murray, J. D., Poole, P. S., and Downie, J. A. (2011). The rules of engagement in the legume-rhizobial symbiosis. Ann. Rev. Genet. 45, 119–144. doi: 10.1146/annurev-genet-110410-132549
Pérez-de-Luque, A., and Hermosín, M. C. (2013). “Nanotechnology and its use in agriculture,” in A Revolution in Food, eds D. Bagchi, M. Bagchi, H. Moriyama, and F. Shahidi (Oxford: Blackwell Publishing Ltd.), 383–398. doi: 10.1002/9781118451915.ch20
Priester, J. H., Ge, Y., Mielke, R. E., Horst, A. M., Moritz, S. C., Espinosa, K., et al. (2012). Soybean susceptibility to manufactured nanomaterials with evidence for food quality and soil fertility interruption. Proc. Natl. Acad. Sci. U.S.A. 109, E2451–E2456. doi: 10.1073/pnas.1205431109
Priyanka, K. P., Harikumar, V. S., Balakrishna, K. M., and Varghese, T. (2017). Inhibitory effect of TiO2 NPs on symbiotic arbuscular mycorrhizal fungi in plant roots. Iet Nanobiotechnol. 11, 66–70. doi: 10.1049/iet-nbt.2016.0032
Raffi, M. M., and Husen, A. (2019). “Impact of fabricated nanoparticles on the rhizospheric microorganisms and soil environment,” in Nanomaterials and Plant Potential, eds A. Husen and M. Iqbal (Cham: Springer).
Rafique, R., Arshad, M., Khokhar, M. F., Qazi, I. A., and Virk, N. (2014). Growth response of wheat to titania nanoparticles application. NUST J. Eng. Sci. 7, 42–46.
Raliya, R., Nair, R., Chavalmane, S., Wang, W. N., and Biswas, P. (2015). Mechanistic evaluation of translocation and physiological impact of titanium dioxide and zinc oxide nanoparticles on the tomato (Solanum lycopersicum L.) plant. Metallomics 7, 1584–1594. doi: 10.1039/c5mt00168d
Ramirez, M. D. A., Silva, J. D., Ohkama-Ohtsu, N., and Yokoyama, T. (2018). In vitro rhizobia response and symbiosis process under aluminum stress. Can. J. Microbiol. 64, 511–526. doi: 10.1139/cjm-2018-0019
Rane, M., Bawskar, M., Rathod, D., Nagaonkar, D., and Rai, M. (2015). Influence of calcium phosphate nanoparticles, Piriformospora indica and Glomus mosseae on growth of Zea mays. Adv. Nat. Sci. Nanosci. Nanotechnol. 6:045014. doi: 10.1088/2043-6262/6/4/045014
Reinsch, B. C., Levard, C., Li, Z., Ma, R., Wise, A., Gregory, K. B., et al. (2012). Sulfidation of silver nanoparticles decreases escherichia coli growth inhibition. Environ. Sci. Technol. 46, 6992–7000. doi: 10.1021/es203732x
Saleem, M., Pervaiz, Z. H., and Traw, M. B. (2015). Theories, Mechanisms and Patterns of Microbiome Species Coexistence in an Era of Climate Change Microbiome Community Ecology. Cham: Springer briefs in ecology, 13–53.
Sarabia-Castillo, C. R., and Fernández-Luqueño, F. (2016). TiO2, ZnO, and Fe2O3 Nanoparticles Effect on Rhizobium Leguminosarum-Pisum Sativum L. symbiosis. Ciudad Obregón: 3rd Biotechnology Summit 2016, 144–149.
Schlich, K., and Hund-Rinke, K. (2015). Influence of soil properties on the effect of silver nanomaterials on microbial activity in five soils. Environ. Pollut. 196, 321–330. doi: 10.1016/j.envpol.2014.10.021
Seeger, E., Baun, A., Kastner, M., and Trapp, S. (2009). Insignificant acute toxicity of TiO2 nanoparticles to willow trees. J. Soils Sedim. 9, 46–53. doi: 10.1007/s11368-008-0034-0
Serraj, R., Sinclair, T. R., and Purcell, L. C. (1999). Symbiotic N2 fixation response to drought. J. Exp. Bot. 50, 143–155. doi: 10.1093/jexbot/50.331.143
Shen, Z. Y., Chen, Z., Hou, Z., Li, T. T., and Lu, X. X. (2015). Ecotoxicological effect of zinc oxide nanoparticles on soil microorganisms. Front. Environ. Sci. Eng. 9, 912–918. doi: 10.1007/s11783-015-0789-7s
Simonin, M., Guyonnet, J. P., Martins, J. M. F., Ginot, M., and Richaume, A. (2015). Influence of soil properties on the toxicity of TiO2 nanoparticles on carbon mineralization and bacterial abundance. J. Hazard. Mater. 283, 529–535. doi: 10.1016/j.jhazmat.2014.10.004
Singh, D., and Kumar, A. (2016). Impact of irrigation using water containing CuO and ZnO nanoparticles on spinach oleracea grown in soil media. Bull. Environ. Contam. Toxicol. 97, 548–553. doi: 10.1007/s00128-016-1872-x
Singh, J., and Lee, B. K. (2016). Influence of nano-TiO2 particles on the bioaccumulation of Cd in soybean plants (Glycine max): a possible mechanism for the removal of Cd from the contaminated soil. J. Environ. Manage. 170, 88–96. doi: 10.1016/j.jenvman.2016.01.015
Smith, F. A., Grace, E. J., and Smith, S. E. (2009). More than a carbon economy: nutrient trade and ecological sustainability in facultative arbuscular mycorrhizal symbioses. New Phytol. 182, 347–358. doi: 10.1111/j.1469-8137.2008.02753.x
Smith, S. E., Smith, F. A., and Jakobsen, I. (2004). Functional diversity in arbuscular mycorrhizal (AM) symbioses: the contribution of the mycorrhizal P uptake pathway is not correlated with mycorrhizal responses in growth or total P uptake. New Phytol. 162, 511–524. doi: 10.1111/j.1469-8137.2004.01039.x
Spatafora, J. W., Chang, Y., Benny, G. L., Lazarus, K., Smith, M. E., Berbee, M. L., et al. (2016). A phylum-level phylogenetic classification of zygomycete fungi based on genome-scale data. Mycologia 108, 1028–1046. doi: 10.3852/16-042
Sweet, M. J., and Singleton, I. (2015). Soil contamination with silver nanoparticles reduces Bishop pine growth and ectomycorrhizal diversity on pine roots. J. Nanopart. Res. 17:448.
Taran, N. Y., Gonchar, O. M., Lopatko, K. G., Batsmanova, L. M., Patyka, M. V., and Volkogon, M. V. (2014). The effect of colloidal solution of molybdenum nanoparticles on the microbial composition in rhizosphere of Cicer arietinum L. Nanoscale Res. Lett. 9:289. doi: 10.1186/1556-276X-9-289
Teng, Y., Wang, X. M., Li, L. N., Li, Z. G., and Luo, Y. M. (2015). Rhizobia and their bio-partners as novel drivers for functional remediation in contaminated soils. Front. Plant Sci. 6:32. doi: 10.3389/fpls.2015.00032
Tong, Z. H., Bischoff, M., Nies, L., Applegate, B., and Turco, R. F. (2007). Impact of fullerene (C60) on a soil microbial community. Environ. Sci. Technol. 41, 2985–2991. doi: 10.1021/es061953l
Tourinho, P. S., van Gestel, C. A. M., Lofts, S., Svendsen, C., Soares, A. M. V. M., and Loureiro, S. (2012). Metal-based nanoparticles in soil: fate, behavior, and effects on soil invertebrates. Environ. Toxicol. Chem. 31, 1679–1692. doi: 10.1002/etc.1880
van der Heijden, M. G. A., Bardgett, R. D., and van Straalen, N. M. (2008). The unseen majority: soil microbes as drivers of plant diversity and productivity in terrestrial ecosystems. Ecol. Lett. 11, 296–310. doi: 10.1111/j.1461-0248.2007.01139.x
Villamizar-Gallardo, R., Cruz, J. F. O., and Ortiz-Rodriguez, O. O. (2016). Fungicidal effect of silver nanoparticles on toxigenic fungi in cocoa. Pesquisa Agropecuaria Brasileira 51, 1929–1936. doi: 10.1590/s0100-204x2016001200003
Vogel-Mikus, K., Pongrac, P., Kump, P., Necemer, M., and Regvar, M. (2006). Colonisation of a Zn, Cd and Pb hyperaccumulator Thlaspi praecox Wulfen with indigenous arbuscular mycorrhizal fungal mixture induces changes in heavy metal and nutrient uptake. Environ. Pollut. 139, 362–371. doi: 10.1016/j.envpol.2005.05.005
Wang, F. Y., Lin, X. G., and Yin, R. (2007). Effect of arbuscular mycorrhizal fungal inoculation on heavy metal accumulation of maize grown in a naturally contaminated soil. Int. J. Phytoremediation 9, 345–353. doi: 10.1080/15226510701476214
Wang, F. Y., Liu, X. Q., Shi, Z. Y., Tong, R. J., Adams, C. A., and Shi, X. J. (2016). Arbuscular mycorrhizae alleviate negative effects of zinc oxide nanoparticle and zinc accumulation in maize plants-A soil microcosm experiment. Chemosphere 147, 88–97. doi: 10.1016/j.chemosphere.2015.12.076
Wang, F. Y., Wang, L., Shi, Z. Y., Li, Y. J., and Song, Z. M. (2012). Effects of AM inoculation and organic amendment, alone or in combination, on growth, P nutrition, and heavy-metal uptake of Tobacco in Pb-Cd-contaminated soil. J. Plant Growth Regul. 31, 549–559. doi: 10.1007/s00344-012-9265-9
Wang, X. P., Liu, X. Q., Chen, J. N., Han, H. Y., and Yuan, Z. D. (2014). Evaluation and mechanism of antifungal effects of carbon nanomaterials in controlling plant fungal pathogen. Carbon 68, 798–806. doi: 10.1016/j.carbon.2013.11.072
Wang, Y. H., Wang, M. Q., Li, Y., Wu, A. P., and Huang, J. Y. (2018). Effects of arbuscular mycorrhizal fungi on growth and nitrogen uptake of Chrysanthemum morifolium under salt stress. PLoS One 13:e0196408. doi: 10.1371/journal.pone.0196408
Watts-Williams, S. J., Turney, T. W., Patti, A. F., and Cavagnaro, T. R. (2014). Uptake of zinc and phosphorus by plants is affected by zinc fertiliser material and arbuscular mycorrhizas. Plant Soil 376, 165–175. doi: 10.1007/s11104-013-1967-7
Whiteside, M. D., Treseder, K. K., and Atsatt, P. R. (2009). The brighter side of soils: quantum dots track organic nitrogen through fungi and plants. Ecology 90, 100–108. doi: 10.1890/07-2115.1
Wightwick, A., Walters, R., Lilinson, G., Reichman, S., and Menzies, N. (2010). “Environmental risks of fungicides used in horticultural production systems,” in Fungicides, ed. O. C. Reijka (Croatia: InTech), 272–303.
Xie, J. R., Ming, Z., Li, H. L., Yang, H., Yu, B. W., Wu, R. H., et al. (2016). Toxicity of graphene oxide to white rot fungus Phanerochaete chrysosporium. Chemosphere 151, 324–331. doi: 10.1016/j.chemosphere.2016.02.097
Yin, L. Y., Cheng, Y. W., Espinasse, B., Colman, B. P., Auffan, M., Wiesner, M., et al. (2011). More than the ions: the effects of silver nanoparticles on Lolium multiflorum. Environ. Sci. Technol. 45, 2360–2367. doi: 10.1021/es103995x
Keywords: nanoparticles, soil, mycorrhiza, rhizobia, colonization, nodule, toxicity
Citation: Tian H, Kah M and Kariman K (2019) Are Nanoparticles a Threat to Mycorrhizal and Rhizobial Symbioses? A Critical Review. Front. Microbiol. 10:1660. doi: 10.3389/fmicb.2019.01660
Received: 27 February 2019; Accepted: 04 July 2019;
Published: 24 July 2019.
Edited by:
Saskia Bindschedler, Université de Neuchâtel, SwitzerlandReviewed by:
Alison Bennett, The Ohio State University, United StatesMuhammad Saleem, Alabama State University, United States
Copyright © 2019 Tian, Kah and Kariman. This is an open-access article distributed under the terms of the Creative Commons Attribution License (CC BY). The use, distribution or reproduction in other forums is permitted, provided the original author(s) and the copyright owner(s) are credited and that the original publication in this journal is cited, in accordance with accepted academic practice. No use, distribution or reproduction is permitted which does not comply with these terms.
*Correspondence: Hui Tian, dGlhbmhAbndzdWFmLmVkdS5jbg==