- 1Microsensor Group, Max Planck Institute for Marine Microbiology, Bremen, Germany
- 2The Freddy & Nadine Herrmann Institute of Earth Sciences, The Hebrew University of Jerusalem, Jerusalem, Israel
- 3The Interuniversity Institute for Marine Sciences in Eilat, Eilat, Israel
- 4GEOMAR Helmholtz Center for Ocean Research Kiel, Kiel, Germany
N2-fixing cyanobacteria mediate H2 fluxes through the opposing processes of H2 evolution, which is a by-product of the N2 fixation reaction, and H2 uptake, which is driven by uptake hydrogenases. Here, we used microelectrodes to characterize H2 and O2 dynamics in single natural colonies of the globally important N2 fixer Trichodesmium collected from the Gulf of Eilat. We observed gradually changing H2 dynamics over the course of the day, including both net H2 evolution and net H2 uptake, as well as large differences in H2 fluxes between individual colonies. Net H2 uptake was observed in colonies amended with H2 in both light and dark. Net H2 evolution was recorded in the light only, reflecting light-dependent N2 fixation coupled to H2 evolution. Both net H2 evolution and H2 uptake rates were higher before 2 pm than later in the day. These pronounced H2 dynamics in the morning coincided with strong net O2 uptake and the previously reported diel peak in N2 fixation. Later in the afternoon, when photosynthesis rates determined by O2 measurements were highest, and N2 fixation rates decrease according to previous studies, the H2 dynamics were also less pronounced. Thus, the observed diel variations in H2 dynamics reflect diel changes in the rates of O2 consumption and N2 fixation. Remarkably, the presence of H2 strongly stimulated the uptake of mineral iron by natural colonies. The magnitude of this effect was dependent on the time of day, with the strongest response in incubations that started before 2 pm, i.e., the period that covered the time of highest uptake hydrogenase activity. Based on these findings, we propose that by providing an electron source for mineral iron reduction in N2-fixing cells, H2 may contribute to iron uptake in Trichodesmium colonies.
Introduction
Marine primary productivity is often limited by the availability of dissolved organic or inorganic nitrogen (Moore et al., 2013). Diazotrophic cyanobacteria can access an additional nitrogen source, dinitrogen (N2) gas, and reduce it to ammonia, thereby making it available for other phytoplankton. As part of the N2 fixation reaction catalyzed by nitrogenase, an equimolar amount of hydrogen (H2) is produced:
Since the reduction of protons (H+) consumes both reducing equivalents and ATP, H2 evolution contributes to the high energy costs related to N2 fixation. However, all N2-fixing cyanobacteria analyzed so far have either uptake hydrogenases or bidirectional hydrogenases that allow them to recycle some of this H2 (Tamagnini et al., 2002). As these hydrogenases can feed electrons from H2 into the respiratory electron transport chain, the uptake of H2 provides a mechanism for recycling reducing equivalents.
The cyanobacterium Trichodesmium is one of the major marine N2 fixers and has been estimated to contribute up to 50% to total nitrogen fixation in some areas (e.g., Karl et al., 1997; Dore et al., 2002). A range of different protective mechanisms has been proposed to shield the O2-sensitive nitrogenase from photosynthetically evolved O2 in Trichodesmium. Firstly, spatial separation of N2 fixation and photosynthesis was suggested for the colony-level (Paerl and Bebout, 1988) as well as the single-cell level (N2-fixing cells termed diazocytes; Bergman and Carpenter, 1991; Berman-Frank et al., 2001). Additionally, nitrogenase activity was shown to be strictly regulated over the diel cycle by daily synthesis of the nitrogenase pool in the morning and inactivation by post-translational modification in the afternoon, allowing for a relatively narrow peak of activity around midday (Zehr et al., 1993; Berman-Frank et al., 2001). A down-regulation of photosynthesis during this midday peak in N2 fixation was suggested as a mechanism to protect nitrogenase from O2 (Berman-Frank et al., 2001). Regarding the H2 metabolism of Trichodesmium, the genome of Trichodesmium erythraeum IMS101 encodes for the uptake hydrogenase hupSL1 and previous studies on its H2 metabolism showed that it can recapture ca. 70% of the evolved H2 (Wilson et al., 2010, 2012).
In natural systems, Trichodesmium often forms colonies that host a wide range of epibiotic bacteria with diverse functions (Hewson et al., 2009; Hmelo et al., 2012; Lee et al., 2017; Frischkorn et al., 2018). Apart from providing a substrate for bacteria, colonies can induce formation of distinct chemical microenvironments. Early studies suggested anoxic microenvironments in Trichodesmium colonies to facilitate N2 fixation in colonies (Paerl and Bebout, 1988), potentially including the Knallgas reaction (i.e., the reaction of H2 with O2 to form H2O) as a mechanism to reduce O2 concentrations (Saino and Hattori, 1982). However, more recent studies showed that during day-time in the light, when photosynthesis is active, O2 concentrations in colonies can increase to as much as 200% of air saturation, which would impose a considerable challenge to the O2-sensitive nitrogenase in Trichodesmium (Eichner et al., 2017, 2019). In view of this colony-specific potential impediment to N2 fixation, it becomes all the more relevant to identify the role of colony formation.
Recent studies have suggested colony formation to facilitate iron acquisition from dust, as Trichodesmium colonies were shown to efficiently capture dust particles, move them to the colony center and enhance iron dissolution from the dust (Rubin et al., 2011). The physiological and biochemical mechanisms of dust particle translocation to the colony center and iron dissolution from dust by Trichodesmium are still under investigation. In a parallel study (Eichner et al., submitted), we examined whether chemical gradients within colonies provide beneficial conditions for iron dissolution in the colony center. Measuring O2 and pH gradients within Trichodesmium colonies with microsensors, we found that the respiration-induced decreases in O2 and pH in colonies are not large enough to significantly enhance iron dissolution. Hence, the active centering of dust particles in the colony must have a different reason.
The release of soluble iron from minerals, where it is generally present in the form of Fe(III) oxide or oxyhydroxide, can be facilitated by ligand (L) binding (Eq. 2) and/or the reduction of Fe3+ to Fe2+ (Eq. 3):
Mineral iron dissolution by Trichodesmium has been suggested to involve ligand-promoted dissolution (Basu et al., in press) and/or reductive dissolution (Rubin et al., 2011). While Trichodesmium has been demonstrated to reduce dissolved iron (Roe and Barbeau, 2014; Lis et al., 2015), direct measurements of mineral iron reduction by this organism are still lacking. Reductive dissolution of mineral iron most likely occurs in the vicinity of the cells in the colony center, where the minerals are concentrated. One possibility is electron transfer to Fe(III) directly on the cell surface, using electrons derived from photosynthesis or respiration, by a yet unidentified mechanism. Alternatively, accumulation of a reductant in the colony microenvironment might enable reductive dissolution. Here, we propose that the uptake of H2, which is produced during N2 fixation, might play a previously unrecognized role in supplying reducing power for iron acquisition. Specifically, we hypothesized that H2 can act as a reductant for mineral-bound Fe(III), which is then liberated from the dust matrix for uptake and assimilation.
To address this hypothesis, we firstly used microelectrodes to characterize H2 fluxes and their dynamics in relation to O2 fluxes over the diel cycle in the microenvironment of single colonies collected in the Gulf of Eilat. In a second step, we used this data to analyze the kinetics of H2 uptake under different light conditions and stages of the diel cycle. Finally, to evaluate the potential of H2 as a reductant for mineral iron dissolution and acquisition, we investigated whether addition of H2 affects the uptake of mineral iron by Trichodesmium colonies.
Materials and Methods
Colony Sampling
Trichodesmium colonies were sampled from the Gulf of Eilat/Aqaba in the Red Sea over a period of 2 months from March to May 2018. Colonies were collected with a 200 μm net, either placed statically at ca. 1–2 m depth on a pole extended from the pier (3–4 m bottom depth) for approx. 2 h, or by vertical net tows from 20 m to the surface carried out from a boat at ca. 300 m bottom depth in the Gulf. Puff-shaped colonies were then hand-picked with Pasteur pipettes and washed with trace metal free filtered sea water (cleaned using ion exchange resin Chelex 100). Colonies were collected multiple times during the day and kept in filtered seawater under stable light conditions (ca. 130 μmol photons m−2 s−1) at 25°C for ca. 1–5 h until measurements were started. Assuming that the diel rhythm of metabolic activity by the colonies is maintained under these conditions, rate measurements over the diel cycle are reported relative to the time of measurement rather than sample collection time.
Microsensor Measurements
Microsensor measurements were performed on single colonies. The colonies were placed in filtered seawater in Petri dishes of approx. 50 ml volume, and held in position with a thin glass needle above a nylon mesh to ensure unperturbed diffusion in all directions. The seawater was slowly mixed during measurements by an air flow over the water surface produced with a pipette connected to an aquarium air pump. Measurements were performed at approx. 25°C and 350–450 μmol photons m−2 s−1, unless specified otherwise.
Clark-type O2 microelectrodes with ca. 10 μm tip diameter were made at Max Planck Institute for Marine Microbiology as described earlier (e.g., Kühl and Revsbech, 2000). O2 sensors were calibrated with seawater bubbled with N2 gas (0% reading) and with air-equilibrated seawater (21% reading; 212 μmol L−1 O2 at 23°C and salinity of 40) and corrected for changes in electrode performance over the course of the experiments by assuming that O2 concentrations in the bulk seawater surrounding the colonies (i.e., outside of the microenvironment affected by cellular O2 fluxes) was in equilibrium with the room air, which was facilitated by the large surface area of the Petri dish used for measurements. H2 microelectrodes (Unisense) had a tip size of 50 μm and minimum detection limit below 50 nmol L−1 and were calibrated with solutions of known H2 concentrations prepared by dilution of H2-saturated seawater (solubility of 652 μmol L−1 at 26°C and salinity of 40) assuming a linear response of the electrode. Calibrations were performed daily to account for changes in electrode sensitivity over the duration of the study.
For recording depth profiles, microelectrodes were moved toward and through the colonies at 50–100 μm step size with a micromanipulator (VT-80, Micos) driven by a motor (Faulhaber minimotor, SA) while observing the colony at ca. 5× magnification in a stereomicroscope (SMZ1500, Nikon with DMC G5, Panasonic camera). For simultaneous measurements of O2 and H2 profiles on the same colony, the two sensors were mounted in a 90° angle (Figure 1A) on two separate micromanipulators. Each colony was photographed for determination of colony dimensions and calculation of volume and surface area assuming spherical geometry.
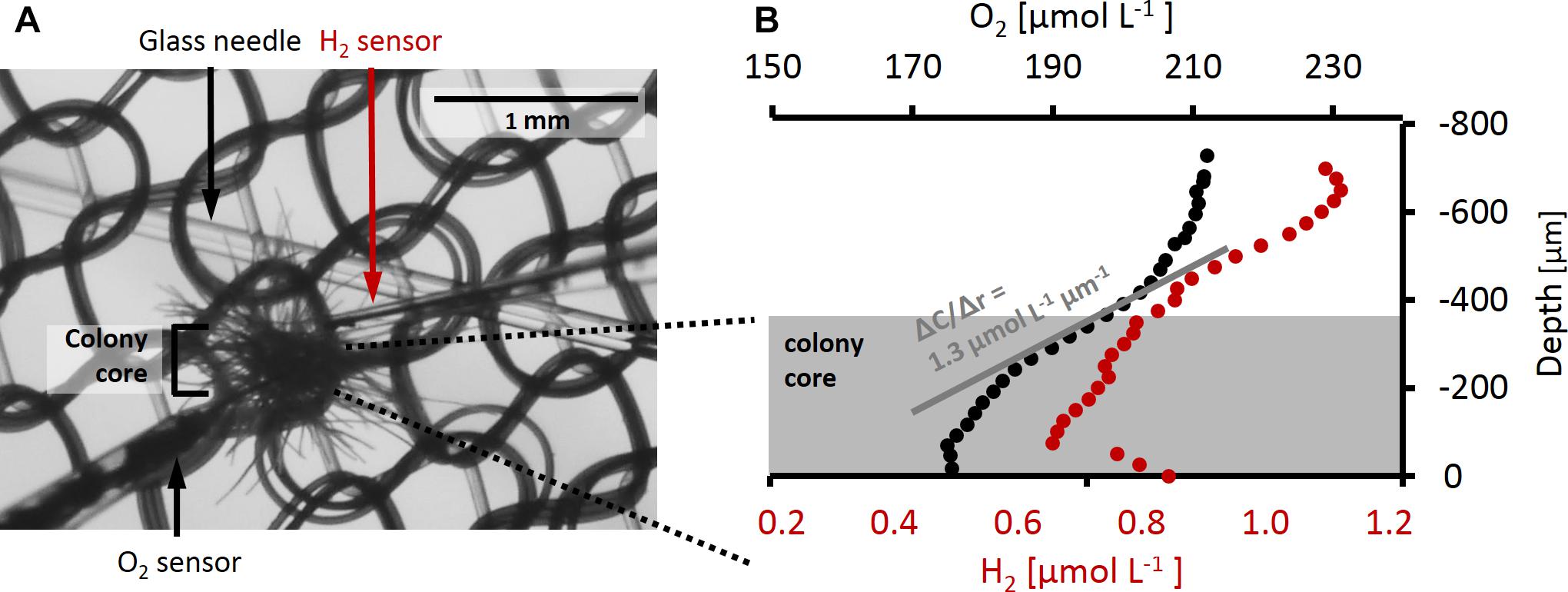
Figure 1. (A) Trichodesmium colony during a microsensor measurement. O2 sensor and H2 sensor as well as the glass needle and nylon mesh holding the colony in place are shown. The size of the colony core as used for flux calculations is indicated by the bracket. (B) Example of O2 and H2 depth profiles measured simultaneously on the same colony. Measurements were performed in the dark after H2 addition. Gray shading indicates the approximate area taken by the colony core, the center of the colony is approximately at depth –70 μm. The gray line shows an example of a linear fit to the O2 gradient at the colony surface. The slope of this line gives ΔC/Δr which is used to calculate the flux of O2 across the colony surface according to Eq. 4.
Net O2 and H2 fluxes in and out of the colonies were calculated from the steady state gradients at the colony surface (Figure 1B) according to Fick’s first law of diffusion:
where J represents the interfacial O2 or H2 flux, D the diffusion coefficient (2.2 × 10−9 m2 s−1 for O2 and 4.3 × 10−9 m2 s−1 for H2 at 25°C and salinity 40; Broecker and Peng, 1974), and ΔC the concentration difference measured over the respective distance, Δr, at the colony surface. Interfacial flux was converted to the volume-normalized rate using estimates of surface area and volume for each colony. The surface of the colony was defined visually based on measured profiles as the depth where gradients in O2 concentrations were steepest. Note that this was typically the boundary of the relatively dense colony core (as indicated in Figure 1), i.e., excluding single filaments protruding outward from the core area.
For detecting not only net H2 evolution but also potential net H2 uptake rates, H2 profiles were recorded under artificially elevated H2 concentrations. To this end, H2 was added by pipetting H2-saturated seawater into the measurement container, reaching final concentrations from below detection limit up to 13 μmol L−1 (6 ± 12 μmol L−1).
Iron Uptake Measurements
Iron uptake from radioactively labeled 55ferrihydrite colloids by natural colonies was determined as described by Basu and Shaked (2018). Briefly, ca. 50 colonies were placed in 5 ml acid-cleaned glass vials and 55ferrihydrite was added to a final concentration of 100 nmol L−1. Vials were then amended with H2-saturated seawater to reach a final H2 concentration of 45 ± 34 μmol L−1 (18–102 μmol L−1), closed without headspace and incubated at ca. 300 μmol photons m−2 s−1 and 25°C for 3–12 h. Control samples without added H2 were prepared for each experiment and H2 concentrations in all vials were determined at the end of incubations with H2 microelectrodes. At the end of the incubations, samples were transferred into Ti-EDTA-citrate solution (20 min) to ensure removal of absorbed 55ferrihydrite and effective detachment of bacteria, and subsequently filtered on 8 μm polycarbonate filters to retain Trichodesmium biomass. 55Fe internalized by Trichodesmium was determined by β-counting of the filters in a Tri-carb 1600 CA liquid scintillation counter (Packard).
Results
H2 Dynamics Over the Diel Cycle – Relation to O2 Fluxes
Microsensor measurements revealed a large variability in H2 and O2 fluxes between single colonies of Trichodesmium analyzed under similar conditions at the same time of the day (Figure 2). Both net evolution and net uptake of H2 were observed in the presence of added H2 (Figures 2A,B). The range of net H2 and O2 fluxes was clearly dependent on light and showed a distinct pattern over the diel cycle. Net H2 uptake was observed in both light and dark, whereas net H2 evolution was observed only in the light. Both net H2 evolution and net H2 uptake were highest during morning and midday. In the afternoon, there was no net H2 evolution and H2 uptake rates were relatively low. The peak in net H2 evolution and uptake in the late morning/midday coincided with a peak in O2 uptake (respiration) rates (Figures 2C,D) as well as the previously reported daily maximum in N2 fixation rates in Trichodesmium, ca. 5 h after beginning of the light period (e.g., Berman-Frank et al., 2001; Levitan et al., 2007; Milligan et al., 2007; Kranz et al., 2010; Eichner et al., 2014). Following a transient decrease in O2 fluxes (Figures 2C,D) after midday, respiration and photosynthesis increased again. Photosynthesis rates reached their daily maximum in the late afternoon, when there was no net H2 evolution, moderate H2 uptake and N2 fixation rates were reported to be low (Figure 2C; Berman-Frank et al., 2001; Levitan et al., 2007; Milligan et al., 2007; Kranz et al., 2010; Eichner et al., 2014).
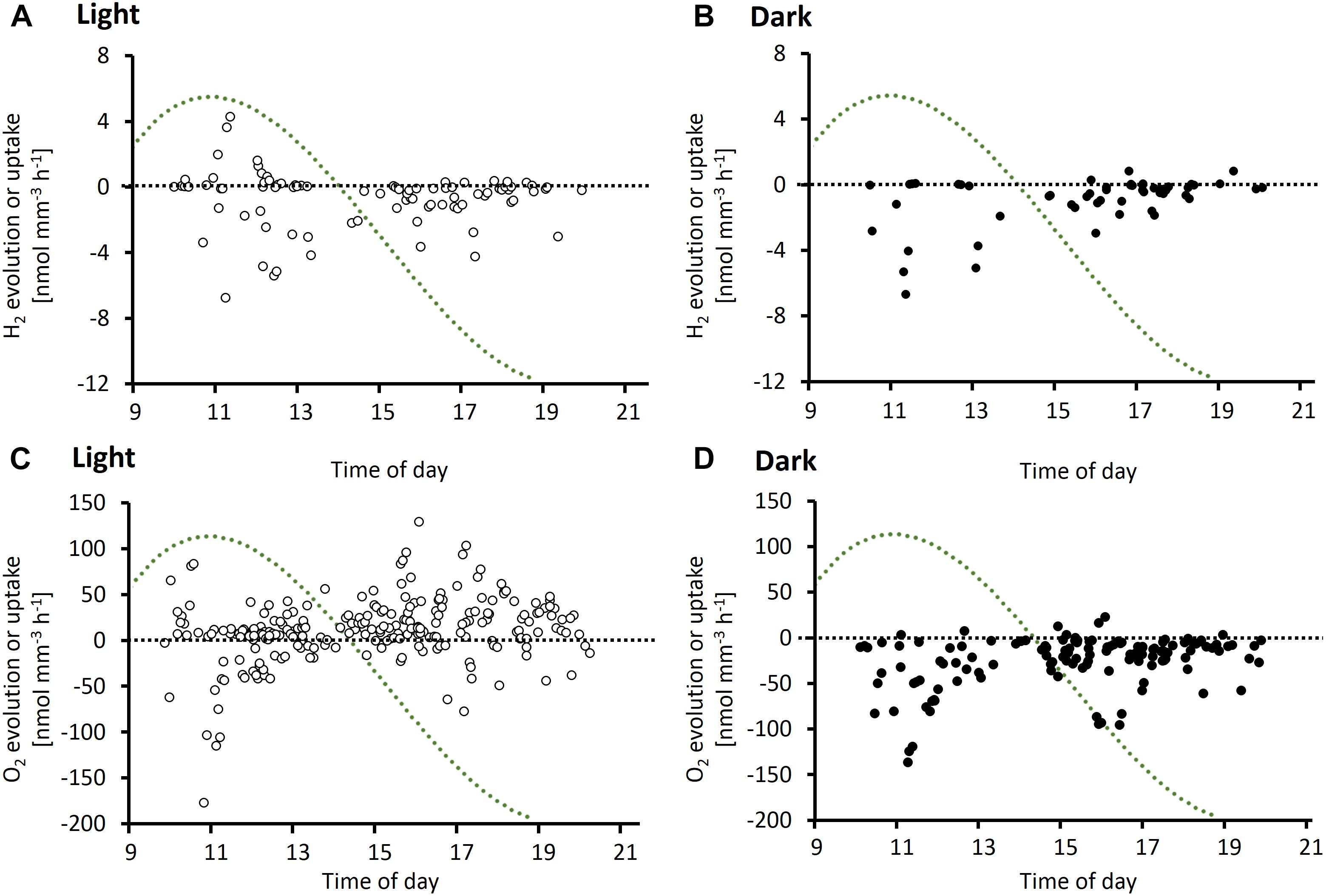
Figure 2. Diel cycle of potential H2 evolution or uptake rates (A,B) and O2 evolution or uptake rates (C,D) based on depth profiles measured on Trichodesmium colonies in the light (A,C) and dark (B,D). Data points represent single measurements of H2 or O2 profiles. Measurements were performed on a total of 130 and 23 colonies for O2 and H2, respectively. X-axis denotes time of measurement. Note that all data shown are net rates, with positive numbers reflecting net evolution of H2 or O2 by a specific colony, and negative numbers reflecting net uptake of H2 or O2, respectively. The green dotted line represents a typical diel cycle in N2 fixation (arbitrary units), which was obtained by a third order polynomial fit to an average of N2 fixation rates measured by Berman-Frank et al. (2001), Milligan et al. (2007), Kranz et al. (2010), and Eichner et al. (2014). N2 fixation rates were normalized to the diel maximum in the respective study. For laboratory studies, start and end of the 12 h light period were aligned to the times of sunrise (6 am) and sunset (7 pm), respectively, in Eilat at the time of the experiments.
In a separate experiment, O2 and H2 concentrations were followed over time at the same position close to the center of a colony during consecutive light and dark phases (Figure 3A). An increase in H2 concentrations by up to 200 nmol L−1 was observed when the lights were switched on in four out of eight colonies tested (Figure 3B). One colony tested in the morning showed the opposite response to light, i.e., higher H2 concentrations in the dark than in the light (colony b, Figure 3B). The response to light was strongest around midday, as observed for absolute fluxes of H2 (Figure 2), and in concert with the previously reported peak in N2 fixation. Two colonies tested in the afternoon, i.e., outside of the N2 fixation period, showed no light response in H2 fluxes, although the same colonies were clearly active as indicated by their strong response in O2 concentrations to light (colonies g and h, Figures 3B,C). O2 responses to light showed an opposite diel pattern compared to H2 responses, with stronger responses in the afternoon than at morning/midday (Figure 3C).
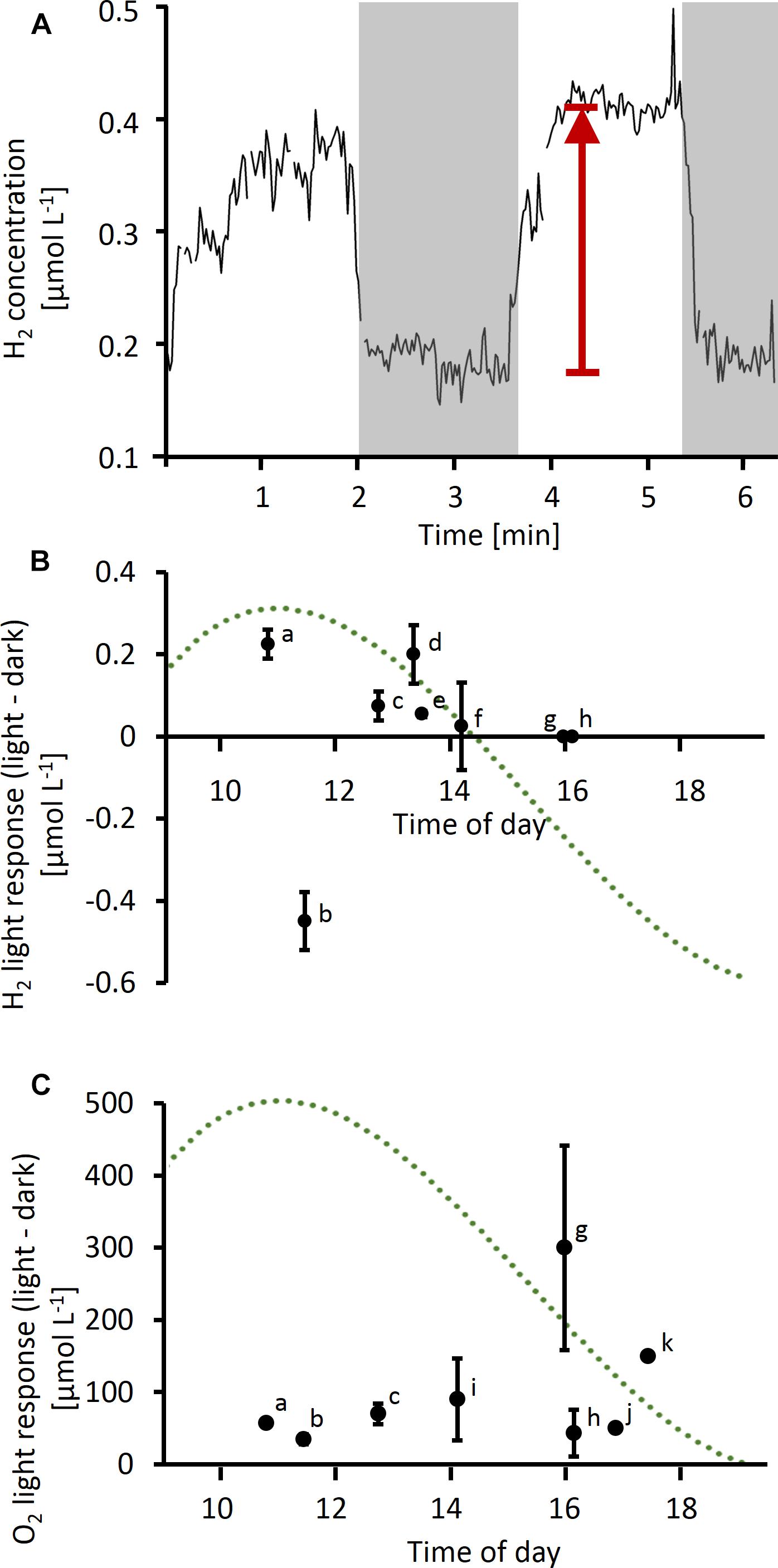
Figure 3. (A) Example of time series measurement of H2 performed on a single Trichodesmium colony. Gray shading indicates dark phases, arrow indicates light response (as plotted in B). (B,C) Light response in H2 (B) and O2 (C) concentrations (i.e., difference in concentration in light versus dark) measured at different times of the day. Data points labeled with the same letter in (B) and (C) were measured simultaneously using two microelectrodes (H2 and O2) on the same colony. Error bars indicate standard deviation of multiple measurements on the same colony (n ≥ 2, except for colonies j and k). The dotted line represents a typical diel cycle in N2 fixation (arbitrary units), which was obtained by a third order polynomial fit to N2 fixation rates measured in previous studies as specified in the legend of Figure 2.
Simultaneous measurements of H2 and O2 gradients performed by using two microelectrodes on the same colony showed a positive correlation (R2 = 0.67) between net O2 and H2 uptake when measured in the afternoon in the dark, with a ratio of ca. 50 mol O2 per mol H2 taken up (Figure 4A). In light, when photosynthesis was active, O2 fluxes were not strongly correlated with H2 uptake (R2 = 0.2; Figure 4B).
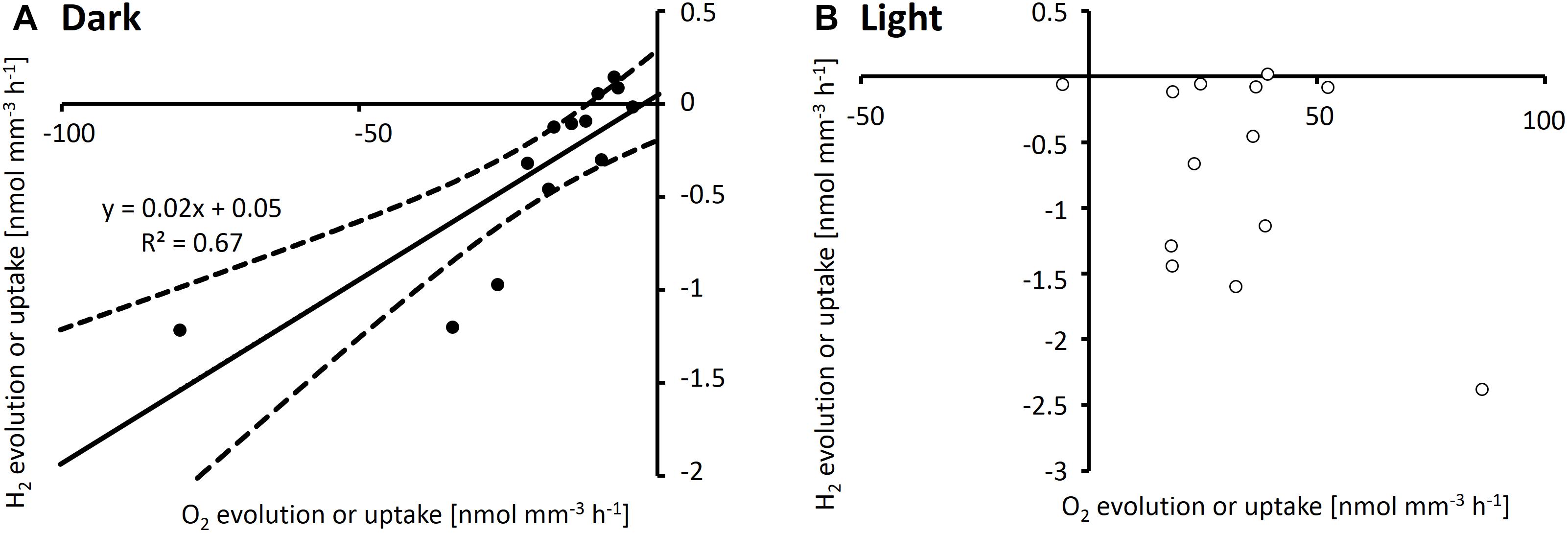
Figure 4. H2 and O2 evolution rates measured simultaneously by using two electrodes on the same Trichodesmium colonies in dark (A) and light (B). All measurements were conducted after 2 pm. Note that all data shown are net rates, with positive numbers reflecting net evolution of H2 or O2 by a specific colony, and negative numbers reflecting net uptake of H2 or O2, respectively. (A) shows a linear regression (solid line) and 95% confidence interval of the fit (dashed lines).
H2 Dynamics Over the Diel Cycle – Reaction Kinetics
Aiming for a more systematic understanding of the kinetics of H2 uptake and evolution in Trichodesmium, we analyzed the dependence of H2 fluxes across the colony surface on the concentration of added H2 under different conditions (Figure 5). This approach of measuring H2 evolution under different H2 concentrations as well as light conditions over the diel cycle allowed us to explore the regulation of uptake hydrogenase activity on different levels, separating the roles of substrate availability, instantaneous light intensity and the underlying diel rhythm. To evaluate the relative importance of each of these parameters for H2 uptake, we firstly focused on H2 fluxes under conditions when we expected no H2 evolution, i.e., measurements carried out in the dark and in the afternoon, when nitrogenase is presumably inactive (Figures 5A–C). Under these conditions, the rate of H2 uptake can be simply described as
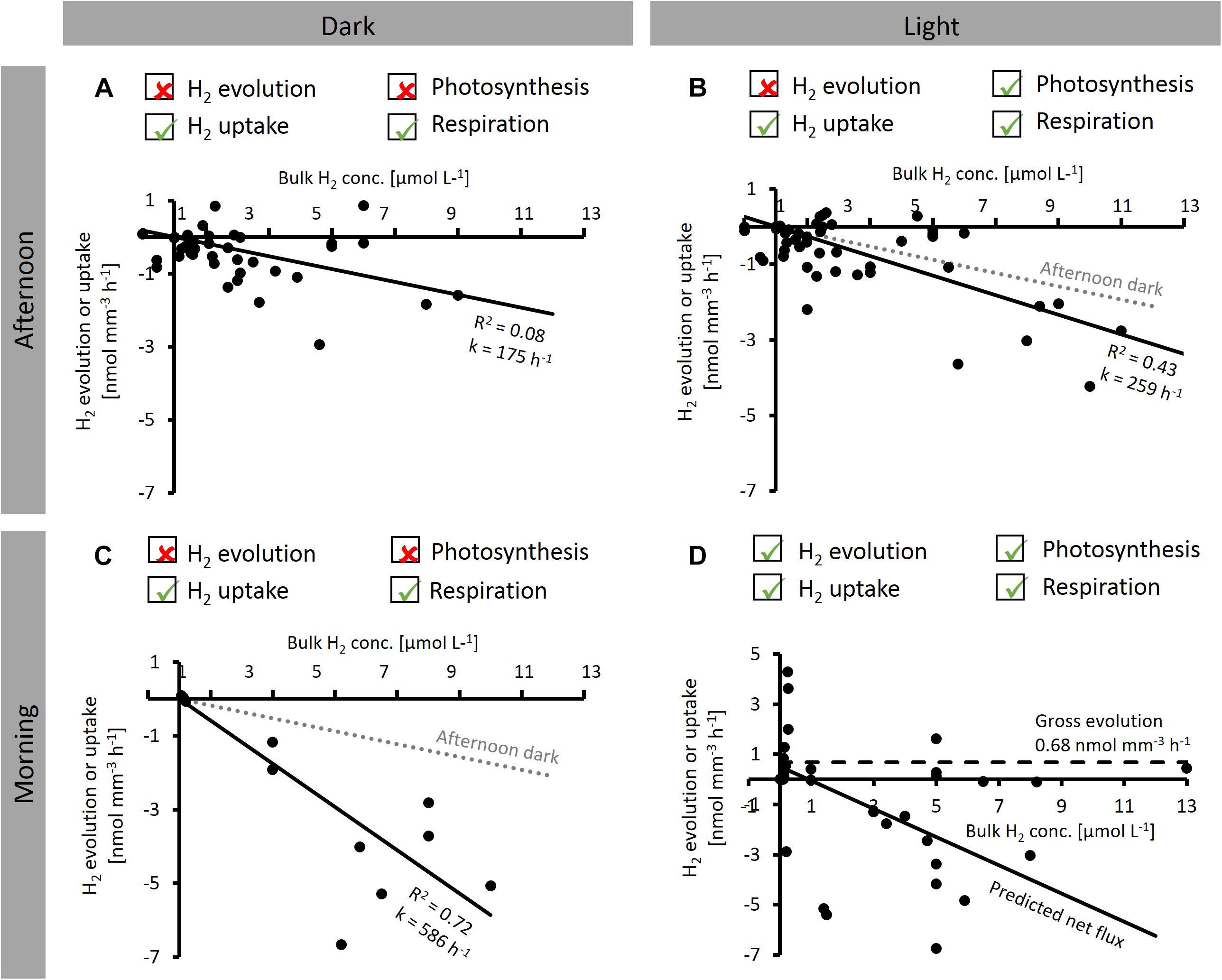
Figure 5. Dependence of net H2 evolution and uptake by Trichodesmium colonies on bulk H2 concentration measured at different times of the day [before 2 pm (morning) and after 2 pm (afternoon)] and under different light conditions. Ticked boxes above the plots indicate the dominant H2 and O2 producing and consuming processes under each condition, as indicated by microsensor results from this study. (A–C) show linear regression lines (y-axis intercept forced to zero). (D) Solid line shows predicted net H2 flux, dashed line shows gross H2 evolution, predicted as indicated in the text. Note that all data shown are net rates with positive numbers reflecting net evolution of H2 by a specific colony, and negative numbers reflecting net uptake of H2.
where k is a pseudo first order kinetic rate constant with units of h−1, which appears as the slope of the regression lines in Figures 5A–C.
In the afternoon, H2 uptake was not significantly different between light and dark (F-test, p > 0.05; Figures 5A,B), with k values of 175 and 259 h−1 for dark and light, respectively. On the other hand, comparison of the dark k value determined in the afternoon to that determined in the morning shows that H2 uptake was strongly affected by the time of the day, with a threefold stimulation in the morning (k = 586 h−1; F-test, p < 0.05; Figure 5C).
To estimate the implications of our findings for H2 fluxes under natural conditions, we furthermore used our experimental data to predict the average H2 flux under typical bulk H2 concentrations in natural systems. Under N2-fixing conditions, when H2 evolution (by nitrogenase) and H2 uptake (by hydrogenase) co-occur (Figure 5D), the net H2 flux can either be positive (net evolution) or negative (net uptake), depending on the bulk H2 concentration. The rate of net H2 evolution under these conditions can be described as the difference between gross H2 evolution and H2 uptake, which is, in turn, given by the product of k and [H2] (cf. Eq. 5):
Given that H2 uptake across the colony surface cannot persist when there is no H2 present in the bulk seawater, the rate of gross H2 evolution was estimated from net H2 evolution rates measured at the lowest bulk H2 concentrations in our experiments (average net H2 evolution below 0.5 μmol L−1 bulk H2). Please note that this concept of “gross H2 evolution” refers to fluxes across the colony surface, whereas significantly larger fluxes may occur within the colony. Assuming that nitrogenase activity is not dependent on external H2 concentrations, the gross H2 evolution rate would be constant across all H2 concentrations at 0.68 nmol mm−3 h−1 (dashed line in Figure 5D). Since light had only a minor effect on k (Figure 5B), we assumed k determined in the morning in dark to be valid also under light conditions. The resulting predicted net H2 flux is plotted in Figure 5D (solid line). Based on the x-axis intercept of this line, our data suggest net H2 evolution to occur below a bulk H2 concentration of ca. 1 μmol L−1, whereas at higher H2 concentrations, H2 uptake is dominant.
Response of Mineral Iron Uptake to H2 Addition
Mineral iron uptake by Trichodesmium colonies in incubations without added H2 was similar across five experiments conducted at different times of the day (Figure 6), with average uptake rates of 14 ± 3 fmol Fe colony−1 day−1. In the presence of H2, mineral iron uptake was strongly elevated in three out of five experiments, including both puff-shaped colonies (experiments #2 and #3; Figure 6) and tuft-shaped colonies (experiment #1; Figure 6). Over a whole day-night cycle, iron uptake was increased by more than a factor of 2 in the presence of H2 (experiment #1, Figure 6). In shorter incubations, the response in uptake to H2 was dependent on the time of the day. The strongest response was observed in incubations that were started at midday (experiments #2 and 3, Figure 6), and thus included the previously reported maximum in N2 fixation rates. In incubations lasting from the end of the N2 fixation period till the evening (experiments #4 and 5, Figure 6), there was no response to H2.
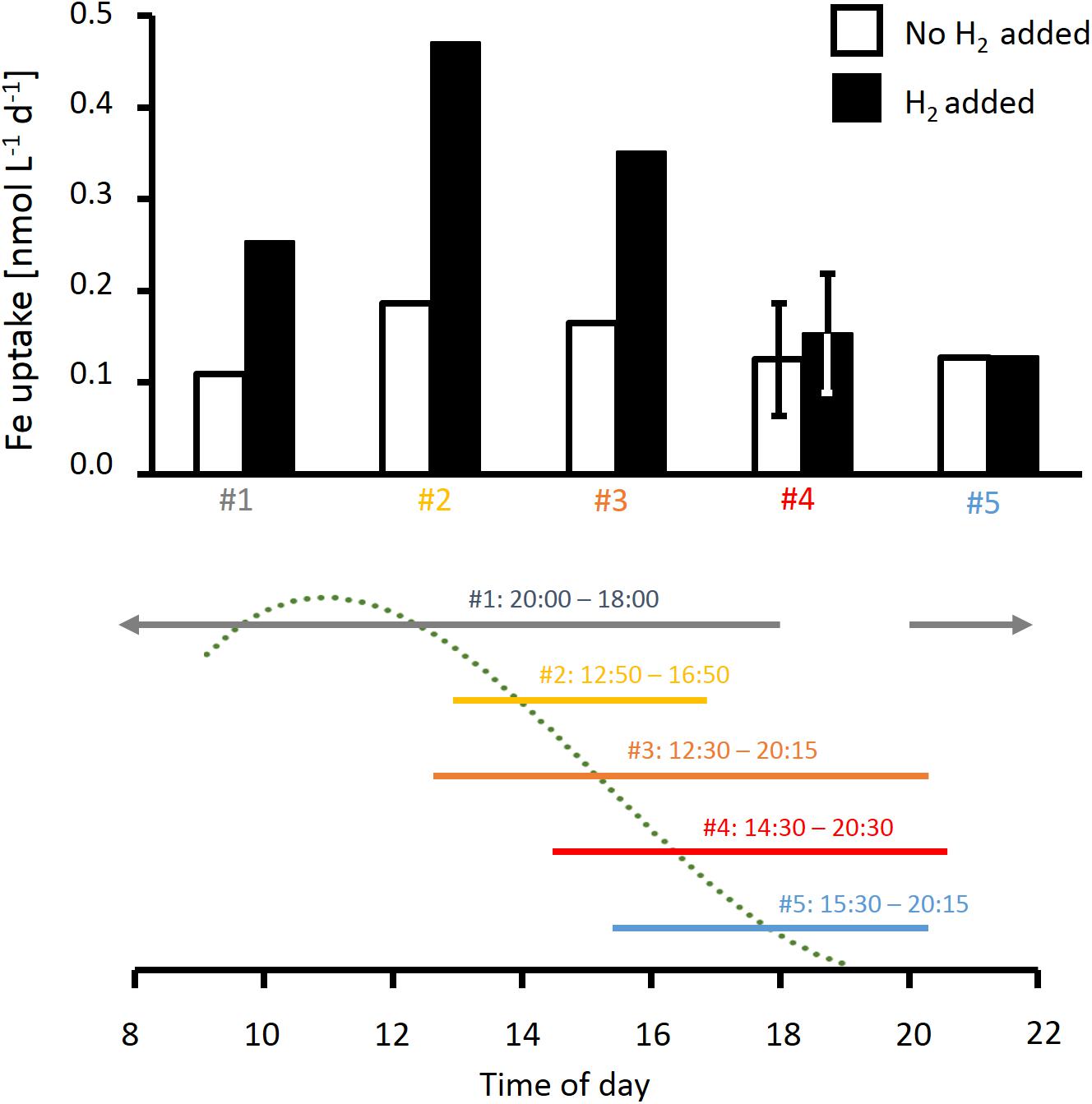
Figure 6. Effect of H2 on rates of iron uptake from ferrihydrite by natural Trichodesmium colonies measured at different times of the day. The time line below the plot indicates timing of the respective incubations as well as a typical diel cycle in N2 fixation (dotted line), which was obtained by a third order polynomial fit to N2 fixation rates measured in previous studies as specified in the legend of Figure 2. Error bars for incubation #4 show standard deviation of two replicate samples. Note incubation #1 continued through the night.
Discussion
H2 Uptake and Evolution by Individual Colonies
Our microsensor measurements of H2 and O2 gradients in single, field-collected Trichodesmium colonies demonstrate significant variability in H2 metabolism between individual colonies, which resulted in net H2 fluxes directed either in or out of the colony. Single Trichodesmium colonies thus provide distinct and highly diverse microenvironments with regard to H2 concentrations. Our experimental approach does not distinguish between the contribution of Trichodesmium and its associated bacteria to the H2 fluxes. However, the fact that Trichodesmium colonies in our study were always oxic (>100 μmol L−1 O2) precludes activity of many of the known H2-consuming taxa, such as anoxygenic phototrophs and sulfate reducing bacteria, and H2-producers such as fermenting cyanobacteria. It should be noted that gross H2 fluxes may be significantly larger than the net H2 fluxes determined in this study, particularly in the confined space of a colony microenvironment with close spatial coupling of sources and sinks.
Previous studies have reported a wide range of H2 evolution rates by Trichodesmium. Field studies in different locations across the North Atlantic and Caribbean showed H2 evolution rates ranging between 0.002 and 0.05 nmol colony−1 h−1 (Scranton, 1983, 1984; Scranton et al., 1987). The maximum net H2 evolution rate per colony in our study (0.08 nmol colony−1 h−1) strongly exceeds these previous field-based estimates. For laboratory cultures grown in single filaments under ca. 50 μmol photons m−2 s−1, net H2 evolution rates in the order of 1 nmol (μg chl a)−1h−1 and up to 3 nmol (μg chl a)−1h−1 were reported (Wilson et al., 2010, 2012). Using a chlorophyll a content per colony determined previously in the North Pacific Subtropical Gyre (14 ng colony−1; Eichner et al., 2017) to normalize H2 fluxes, our maximum estimate also exceeds net H2 evolution determined in the laboratory by approx. a factor of two (Wilson et al., 2010). Similarly, maximum net H2 uptake in our study [9 nmol H2 (μg chl a)−1 h−1] exceeds previous estimates of H2 uptake for Trichodesmium (estimated as the effect of the hydrogenase inhibitor carbon monoxide on net H2 evolution; Saino and Hattori, 1982) by approx. a factor of two. Aside from potential effects of experimental conditions among these studies, these comparisons demonstrate that H2 fluxes in and out of the microenvironment of single colonies can be significantly larger (at their diel maximum) than average H2 fluxes determined in incubations of several colonies or homogenous cultures.
In contrast to the previous reports of net H2 evolution, the average across all our measurements in light was a net H2 uptake rate of 0.01 ± 0.03 nmol colony−1 h−1. While experimental conditions in previous laboratory as well as field studies (such as different light intensities, colony formation and different species) most probably contribute to the differences between ours and previous results, possibly the most important difference was that our measurements were performed under micromolar H2 concentrations, while in natural waters H2 concentrations are typically in the low nanomolar range (e.g., Scranton, 1983; Wilson et al., 2013). Assuming a linear relation between net H2 evolution/uptake and bulk H2 concentration (Figure 5D), we extrapolated our data to lower H2 concentrations, and predicted that under bulk H2 concentrations below ca. 1 μmol L−1, H2 evolution would exceed H2 uptake. Under natural H2 conditions, the net H2 flux would thus – on average – be directed out of the colony, implying net H2 evolution in line with previous observations. However, our measurements also demonstrate that substantial variability in H2 fluxes (as observed within and between previous studies) can even be manifested on the level of single colonies sampled over a short period of time at the same location.
Dependence of H2 Fluxes on Light and the Diel Cycle
Measuring the steady-state H2 gradients on the colony surface against a background of added H2 enabled us to estimate the capacity of Trichodesmium colonies for H2 uptake under various conditions. Since the H2 uptake rates were measured under artificially elevated background concentrations of H2, the rates we report should be considered as potential rates. The capacity for H2 uptake was not uniform over the diel cycle and dependent on light (Figures 2, 3), indicating that uptake hydrogenase is not only regulated by the availability of its substrate H2, in line with previous findings (Tamagnini et al., 2002). The fact that both net evolution and net uptake of H2 were observed reflects the delicate balance between the two highly active, opposing processes of H2 evolution by nitrogenase and H2 uptake by uptake hydrogenase. However, considering only conditions where nitrogenase is presumably inactive (afternoon and/or dark) allowed us to separate these process and indicated that light itself had only a minor effect on uptake hydrogenase (Figures 5A–C). Consequently, the light effects on net H2 fluxes in our study (Figures 2, 3) were most likely driven by the light response of nitrogenase activity, whereas uptake hydrogenase was not directly affected by light. The strong stimulation of dark H2 uptake in the morning compared to the afternoon (Figure 5C), in turn, indicates that H2 uptake is regulated over the diel cycle, potentially via an endogenous rhythm. The links between H2 metabolism, respiratory and photosynthetic electron transport in cyanobacteria make for a complex regulatory system. O2 fluxes measured at the same time and/or on the same colonies thus provided an important reference for gaining insights into the physiological mechanisms driving the H2 dynamics we observed in Trichodesmium colonies. In the following paragraph, we discuss these potential physiological mechanisms.
Underlying Physiological Mechanisms
Processes Involved in the Dark
In the dark, O2 uptake by respiration and H2 uptake by hydrogenase were correlated (Figure 4A), in line with previously suggested links between these processes (e.g., Appel and Schulz, 1998). Uptake hydrogenases have been reported to be located on either the thylakoid membrane or the cytoplasmic membrane and to feed electrons into the plastoquinone (PQ) pool of the respiratory electron transport chain (ETC), potentially via a cytochrome-like anchoring unit that binds the H2-oxidizing subunit to the membrane and facilitates electron transport to the respiratory ETC (Appel and Schulz, 1998; Khanna et al., 2016). Electrons originating from H2 could then be transported from PQ to cytochrome b6f, and further to a terminal oxidase where they are used to reduce O2. The ratio of O2 to H2 uptake observed in the dark in the afternoon (Figure 4A) suggests that, under these conditions, 1 out of 50 electrons reducing O2 comes from H2, with the remainder originating from carbohydrates.
Processes Involved in the Light
In the light, O2 and H2 fluxes are determined by photosynthesis and N2 fixation in addition to respiration and H2 uptake. Net H2 evolution was observed in light but not in dark (Figure 2), which we attribute to the light-dependence of nitrogenase activity observed in previous studies on Trichodesmium (e.g., Rabouille et al., 2006; Staal et al., 2007). Activation of nitrogenase by light was also reflected in the instantaneous increase in H2 concentrations when the light was switched on during the time of highest N2 fixation (Figure 3). These findings are in agreement with previous studies showing instantaneous decreases in net H2 evolution in response to addition of NH4+ (which inhibits nitrogenase), DCMU (an inhibitor of PSII), and darkness in Trichodesmium (Wilson et al., 2010, 2012), as well as a direct dependence of H2 evolution on PSI activity in Cyanothece (Min and Sherman, 2010; Skizim et al., 2012). Notably, the interaction between O2 evolution, nitrogenase activity and H2 uptake can be modulated by the reduction state of the photosynthetic electron transport chain (Wilson et al., 2012), suggesting that the quantitative relationship between these processes is strongly dependent on the instantaneous light intensity. Due to the O2-sensitivity of uptake hydrogenase (e.g., Arp and Burris, 1981; Houchins and Burris, 1981; Axelsson and Lindblad, 2002), the high levels of photosynthetic O2 evolution in the afternoon (Figure 2C) might impede not only H2 evolution by nitrogenase but also H2 uptake, leading to lower levels of H2 uptake in the afternoon than in the morning (Figure 2B), and causing a loss of the correlation between H2 and O2 fluxes in the light (Figure 4B).
Regulation Over the Diel Cycle
The timing of highest variability in net H2 fluxes coincided with the time of highest net O2 uptake as well as the midday peak in N2 fixation reported previously (e.g., Berman-Frank et al., 2001). The down-regulation in net O2 evolution during this part of the day is believed to act as a mechanism to protect nitrogenase from O2 (e.g., Berman-Frank et al., 2001) and might additionally benefit uptake hydrogenase, which is also O2-sensitive (e.g., Houchins and Burris, 1981; Axelsson and Lindblad, 2002; Tamagnini et al., 2007). H2 evolution and N2 fixation rates have been previously shown to be correlated over a range of conditions (Tamagnini et al., 2002 and references therein), yet most previous studies on H2 evolution by Trichodesmium did not resolve H2 fluxes over the diel cycle. A laboratory study on Trichodesmium cultures grown in single filaments under a 12:12 h light-dark cycle at significantly lower intensities (44 μmol photons m−2 s−1) did not show the same diel pattern in net H2 evolution (Wilson et al., 2010), potentially due to feedbacks of colony formation and/or the range of light intensities in our field study.
During the morning, when N2 fixation and thus H2 evolution were presumably highest, elevated H2 concentrations might elicit an increase in the activity of uptake hydrogenase. The fact that we observed high net H2 evolution as well as uptake at this time of the day may reflect a mismatch in timing between the activation of nitrogenase and uptake hydrogenase – a slight offset in timing would result in strong net fluxes, while a balanced system with a constant fraction of H2 recycling would result in minimal net fluxes. Interestingly, H2 uptake was highest at morning/midday even in dark measurements (Figure 2B) where nitrogenase was presumably inactive, suggesting that uptake hydrogenase activity is regulated not only by H2 availability (i.e., by nitrogenase activity) or by light, but potentially also via an endogenous rhythm, as shown for nitrogenase in Trichodesmium (Chen et al., 1998). Both, uptake hydrogenases and nitrogen metabolism, are regulated by the transcriptional regulator NtcA (e.g., Tamagnini et al., 2002; Khanna et al., 2016), which was suggested to be under the control of a circadian rhythm in Cyanothece (Bradley and Reddy, 1997). In the afternoon, H2 fluxes were less pronounced (Figures 2A,B) and did not respond to light (Figure 3B) as strongly as in the morning, even though colonies were clearly active and generally responsive to light as indicated by the high O2 evolution in the light (Figures 2C, 3C). H2 evolution by nitrogenase can presumably not respond to light at this time of the day since nitrogenase becomes inactivated by post-translational modification in the afternoon (Zehr et al., 1993). The fact that also H2 uptake did not respond strongly to light (Figure 3) and uptake rates were low despite addition of H2 (Figure 2) at this time of the day suggests that uptake hydrogenase might be down-regulated in the afternoon in a similar way as nitrogenase.
The Potential Role of H2 in Iron Acquisition
Using a recently optimized radiotracer method for measuring iron uptake from mineral iron by Trichodesmium colonies (Basu and Shaked, 2018), we were able to investigate the effects of H2 on iron uptake under conditions that closely resembled the acquisition of iron from dust under natural conditions. Interestingly, these experiments revealed that the uptake of mineral iron was strongly stimulated by the presence of H2 (Figure 6). This effect was observed across different colony morphologies, which are generally assumed to represent different species (e.g., Hynes et al., 2012). Also the timing of H2 uptake and iron uptake was synchronized: Both H2 uptake and evolution as well as the response of iron uptake to H2 were strongest around midday, whereas in the afternoon, both H2 uptake and iron uptake responded less strongly to H2. Taken together, these findings suggest a potential mechanistic link between iron uptake and H2 metabolism. While the exact mechanisms of mineral iron acquisition by Trichodesmium are yet to be elucidated, there are several indications for a reductive step involved in the process, such as the slow-down of iron uptake from ferrihydrite in the presence of the Fe(II) ligand ferrozine (Supplementary Figure 1). The electron source for this reduction has not been identified. Based on the large H2 fluxes in colonies in combination with the stimulation of iron uptake by H2 and indications for co-regulation of uptake hydrogenase and iron uptake over the diel cycle, we propose that H2 may function as an electron source for mineral iron reduction. While the quantitative importance of this potential mechanism needs to be investigated in future studies under H2 concentrations that more closely mimic natural conditions, we focus here on identifying a potential pathway of electron flow that might provide such a link between H2 and mineral iron reduction on the cellular level (Figure 7).
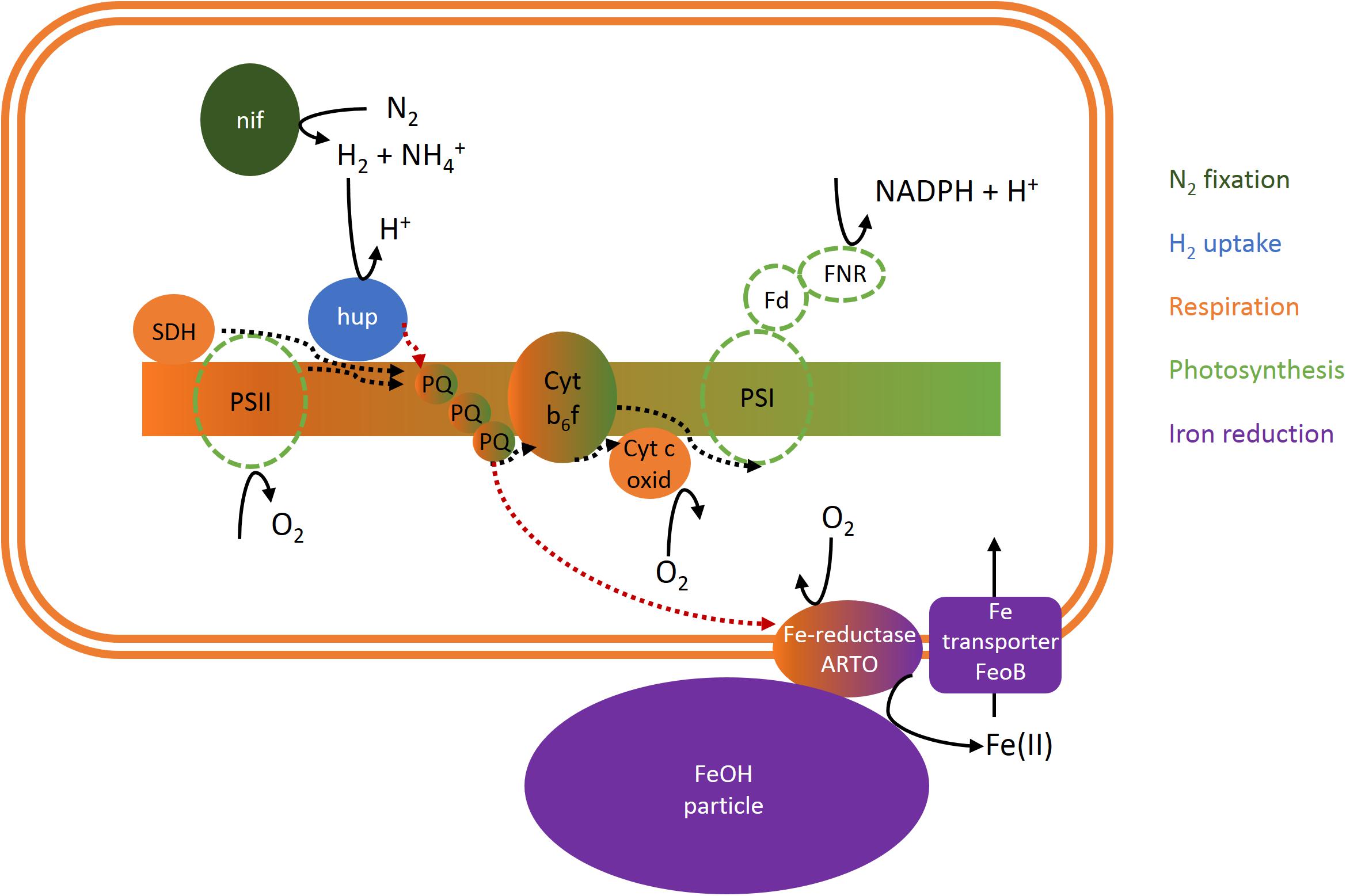
Figure 7. Scheme of a potential cellular link between N2 fixation, H2 and O2 fluxes and iron uptake in Trichodesmium. Dotted lines indicate electron flow. Please note that uptake hydrogenase may be restricted to non-photosynthetic diazocytes and/or located on the cytoplasmic membrane instead of the thylakoid membrane and thus coupled to respiratory electron transport but not photosynthesis (Tamagnini et al., 2002; Khanna et al., 2016). ARTO, alternative respiratory terminal oxidase; Cyt b6f, cytochrome b6f complex; Cyt c oxid, cytochrome c oxidase; Fd, ferredoxin; FeoB, ferrous iron transporter B; FeOH, iron oxide; FNR, ferredoxin-NADPH-oxidoreductase; hup, uptake hydrogenase; nif, nitrogenase; PQ, plastoquinone; PSI, photosystem I; PSII, photosystem II; SDH, succinate dehydrogenase.
The link between uptake hydrogenase and the respiratory electron transport chain has been reported previously (e.g., Appel and Schulz, 1998; Wilson et al., 2010), and was reflected in the correlation between H2 and O2 uptake in our study (Figure 4). In Synechocystis, mineral iron reduction was recently suggested to be facilitated by the alternative respiratory terminal oxidase ARTO (Kranzler et al., 2014). ARTO is also expressed and regulated in response to iron availability in Trichodesmium (Polyviou et al., 2018). As it accepts electrons from PQ (Lea-Smith et al., 2016), it could potentially serve as a link between the respiratory electron transport chain and iron reduction. Since on average, cellular iron uptake rates are several orders of magnitude lower than respiration rates (e.g., iron uptake of ca. 1–9 × 10−18 mol Fe cell−1 d−1, Basu and Shaked, 2018, as compared to respiration of ca. 3 × 10−12 mol O2 cell−1 d−1, Eichner et al., 2017), only a small fraction of electrons in the respiratory pathway would need to be channeled to iron reduction to meet cellular iron demands, while the remaining electrons would reduce O2. Nevertheless, a coupling of H2 uptake to iron acquisition via the respiratory ETC (i.e., electron flow from uptake hydrogenase via the PQ pool to ARTO) could explain the stimulation of iron uptake by H2 observed in this study (Figure 6) during the time of the day when high rates of H2 uptake were observed (Figures 2, 3). The reduced activity of uptake hydrogenase in the afternoon/evening (Figures 2, 3), potentially caused by a down-regulation/post-translational modification of the protein, in turn, could explain the lack of a response in iron uptake to H2 at this time of the day (Figure 6).
Previous studies on heterocystous, filamentous cyanobacteria suggested that uptake hydrogenase is present only in heterocysts (e.g., Tamagnini et al., 2007). Such a restriction of uptake hydrogenase to N2-fixing cells would provide not only a tight spatial coupling between H2 evolution and uptake, but also the benefit of reduced O2 concentrations that would protect both nitrogenase and uptake hydrogenase from oxidative damage. In Trichodesmium, it has been suggested that only 10–20% of cells in each filament, the diazocytes, express nitrogenase and actively fix N2 (e.g., Bergman and Carpenter, 1991, Berman-Frank et al., 2001). Notably, those cells would have a significantly higher iron requirement associated to synthesis of the iron-rich nitrogenase than vegetative cells (19–53% of cellular iron was estimated to be bound in nitrogenase on average across all cell types; Kustka et al., 2003). The pathway of iron reduction coupled to H2 uptake we propose here could provide a mechanism of surplus iron reduction to fuel the enhanced iron demand in these cells. The restriction of hydrogenase to diazocytes would also imply that H2 uptake and photosynthesis are separated on a single-cell level, and thus do not share the same electron transport chain, potentially explaining why light did not have strong direct effects on H2 uptake (at the time when nitrogenase was presumably inactive; Figure 5). Furthermore, assuming that the H2 fluxes we detected as an average of all cells in a single colony were actually catalyzed by only 10–20% of the cells, it should be noted that the ratio of H2:O2 uptake in those cells would be 5–10× larger than the average ratio we measured (Figure 4). Particularly since photosynthetic water splitting is presumably not available as an electron source in diazocytes, H2 as an alternative electron source might be relatively more important in these cells. In summary, although the contribution of H2 to total electron flow in the ETC (as estimated from the ratio of H2:O2 uptake; Figure 4) was relatively low when averaged over all cells and determined outside of the reported peak in N2 fixation, we suggest that in specialized N2-fixing cells at the time of active N2 fixation, a significant part of the electron flux in the respiratory electron transport chain might be channeled from H2 to iron. Future studies should thus aim to confirm the physiological mechanism and quantitative importance of this pathway in Trichodesmium. Complementary molecular biological studies on the metabolic potential of associated bacteria in Trichodesmium colonies will bring further insights into the potential contribution of these bacteria to both hydrogen uptake and iron reduction within colonies.
Conclusion
By high-resolution measurements of H2 and O2 gradients on single colonies, we were able to demonstrate highly variable H2 microenvironments in colonies of the important N2 fixer Trichodesmium. We found that the net H2 flux could be directed either in or out of the colony. H2 uptake was regulated not only by H2 availability and by light, but also over the diel cycle, potentially by an endogenous clock. Both H2 uptake and evolution were generally most active in the morning at the time of highest N2 fixation, reflecting the tight link between nitrogenase and uptake hydrogenase activity. The large variability in H2 fluxes between individual colonies analyzed under similar conditions furthermore highlights that single colonies may provide diverse micro-niches differing strongly in H2 metabolism, including systems characterized by either net H2 evolution or net H2 uptake. Given that the experimental approaches used in previous studies (measuring bulk H2 evolution rates under natural H2 concentrations) do not allow for detecting such potential hot-spots of net H2 uptake, our findings highlight the need for more broadly applying (1) methods that allow for quantifying H2 uptake rather than only net evolution (such as 3H2 assays; Wilson et al., 2013), and (2) high-resolution measurements that can reveal small-scale environmental variability (such as microsensors).
Combining H2 measurements with radiotracer experiments, we furthermore observed a strong stimulation of mineral iron uptake in the presence of H2, indicating a previously unrecognized link between H2 and acquisition of iron. We propose a potential pathway of electron flow linking N2 fixation, H2 uptake, respiration and iron reduction on the cellular level, which should be the subject of further physiological investigations. This link between H2 uptake and mineral iron acquisition adds a new component to the complex network of nutrient acquisition mechanisms employed by Trichodesmium in the colony microenvironment.
Data Availability
The raw data supporting the conclusions of this manuscript will be made available by the authors, without undue reservation, to any qualified researcher.
Author Contributions
ME, YS, and DB designed the study. ME performed the microsensor measurements with help by DB and analyzed the data. SB performed the iron uptake experiments and analyzed the data. ME drafted the manuscript. All authors contributed to the data interpretation and writing the manuscript, and read and approved the final version of the manuscript for submission.
Funding
This work was supported in part by Israel Science Foundation (www.isf.org.il) Grant 458/15 and the German–Israeli Foundation for Scientific Research and Development (www.GIF.org.il) Grant 1349 awarded to YS and MG (and enabling visits by ME to IUI). Additional funding was provided by the Minerva Foundation (awarded to ME) and the Max Planck Society.
Conflict of Interest Statement
The authors declare that the research was conducted in the absence of any commercial or financial relationships that could be construed as a potential conflict of interest.
Acknowledgments
We are grateful to Murielle Dray for technical assistance at IUI, to the technical assistants in the Microsensor Group at MPI for producing the microsensors, as well as to the staff of the Electronics Workshop at MPI for their technical support with the associated equipment. We also thank Ondrej Prasil and Nir Keren for providing valuable feedback on earlier versions of this manuscript.
Supplementary Material
The Supplementary Material for this article can be found online at: https://www.frontiersin.org/articles/10.3389/fmicb.2019.01565/full#supplementary-material
Footnotes
References
Appel, J., and Schulz, R. (1998). Hydrogen metabolism in organisms with oxygenic photosynthesis: hydrogenases as important regulatory devices for a proper redox poising? J. Photochem. Photobiol. B Biol. 47, 1–11. doi: 10.1016/s1011-1344(98)00179-1
Arp, D. J., and Burris, R. H. (1981). Kinetic mechanism of the hydrogen-oxidizing hydrogenase from soybean nodule bacteroids. Biochemistry 20, 2234–2240. doi: 10.1021/bi00511a025
Axelsson, R., and Lindblad, P. (2002). Transcriptional regulation of Nostoc hydrogenases: effects of oxygen, hydrogen, and nickel. Appl. Environ. Microbiol. 68, 444–447. doi: 10.1128/aem.68.1.444-447.2002
Basu, S., and Shaked, Y. (2018). Mineral iron utilization by natural and cultured Trichodesmium and associated bacteria. Limnol. Oceanogr. 63, 2307–2320. doi: 10.1002/lno.10939
Basu, S., Gledhill, M., de Beer, D., Matondkar, S. G. P., and Shaked, Y. (in press) Colonies of marine cyanobacteria Trichodesmium interact with associated bacteria to acquire iron from dust. Biol. Commun.
Bergman, B., and Carpenter, E. J. (1991). Nitrogenase confined to randomly distributed trichomes in the marine cyanobacterium Trichodesmium thiebautii. J. Phycol. 27, 158–165. doi: 10.1111/j.0022-3646.1991.00158.x
Berman-Frank, I., Lundgren, P., Chen, Y. B., Küpper, H., Kolber, Z., Bergman, B., et al. (2001). Segregation of nitrogen fixation and oxygenic photosynthesis in the marine cyanobacterium Trichodesmium. Science 294, 1534–1537. doi: 10.1126/science.1064082
Bradley, R. L., and Reddy, K. J. (1997). Cloning, sequencing, and regulation of the global nitrogen regulator gene ntcA in the unicellular diazotrophic cyanobacterium Cyanothece sp. strain BH68K. J. Bacteriol. 179, 4407–4410. doi: 10.1128/jb.179.13.4407-4410.1997
Broecker, W. S., and Peng, T. H. (1974). Gas exchange rates between air and sea. Tellus 26, 21–35. doi: 10.1111/j.2153-3490.1974.tb01948.x
Chen, Y. B., Dominic, B., Mellon, M. T., and Zehr, J. P. (1998). Circadian rhythm of nitrogenase gene expression in the diazotrophic filamentous nonheterocystous cyanobacterium Trichodesmium sp. strain IMS 101. J. Bacteriol. 180, 3598–3605.
Dore, J. E., Brum, J. R., Tupas, L. M., and Karl, D. M. (2002). Seasonal and interannual variability in sources of nitrogen supporting export in the oligotrophic subtropical North Pacific Ocean. Limnol. Oceanogr. 47, 1595–1607. doi: 10.4319/lo.2002.47.6.1595
Eichner, M., Klawonn, I., Wilson, S. T., Littmann, S., Whitehouse, M., Church, M., et al. (2017). Chemical microenvironments and single-cell carbon and nitrogen uptake in field-collected colonies of Trichodesmium under different pCO2. ISME J. 11, 1305–1317. doi: 10.1038/ismej.2017.15
Eichner, M., Kranz, S. A., and Rost, B. (2014). Combined effects of different CO2 levels and N sources on the diazotrophic cyanobacterium Trichodesmium. Physiol. Plant 152, 316–330. doi: 10.1111/ppl.12172
Eichner, M., Thoms, S., Rost, B., Mohr, W., Ahmerkamp, S., Ploug, H., et al. (2019). N2 fixation in free-floating filaments of Trichodesmium is higher than in transiently suboxic colony microenvironments. New Phytol. 222, 842–863. doi: 10.1111/nph.15621
Frischkorn, K. R., Haley, S. T., and Dyhrman, S. T. (2018). Coordinated gene expression between Trichodesmium and its microbiome over day–night cycles in the North Pacific Subtropical Gyre. ISME J. 12, 997–1007. doi: 10.1038/s41396-017-0041-5
Hewson, I., Poretsky, R. S., Dyhrman, S. T., Zielinski, B., White, A. E., Tripp, H. J., et al. (2009). Microbial community gene expression within colonies of the diazotroph, Trichodesmium, from the Southwest Pacific Ocean. ISME J. 3, 1286–1300. doi: 10.1038/ismej.2009.75
Hmelo, L. R., Van Mooy, B. A. S., and Mincer, T. J. (2012). Characterization of bacterial epibionts on the cyanobacterium Trichodesmium. Aquat. Microb. Ecol. 67, 1–14. doi: 10.3354/ame01571
Houchins, J. P., and Burris, R. H. (1981). Occurrence and localization of two distinct hydrogenases in the heterocystous cyanobacterium Anabaena sp. strain 7120. J. Bacteriol. 146, 209–214.
Hynes, A. M., Webb, E. A., Doney, S. C., and Waterbury, J. B. (2012). Comparison of cultured Trichodesmium (cyanophyceae) with species characterized from the field. J. Phycol. 48, 196–210. doi: 10.1111/j.1529-8817.2011.01096.x
Karl, D., Letelier, R., Tupas, L., Dore, J., Christian, J., and Hebel, D. (1997). The role of nitrogen fixation in biogeochemical cycling in the subtropical North Pacific Ocean. Nature 388, 533–538. doi: 10.1038/41474
Khanna, N., Raleiras, P., and Lindblad, P. (2016). “Fundamentals and recent advances in hydrogen production and nitrogen fixation in cyanobacteria,” in The Physiology of Microalgae. Developments in Applied Phycology, eds M. Borowitzka, J. Beardall, and J. Raven (Cham: Springer), 101–127. doi: 10.1007/978-3-319-24945-2_5
Kranz, S. A., Levitan, O., Richter, K.-U., Prasil, O., Berman-Frank, I., and Rost, B. (2010). Combined effects of CO2 and light on the N2-fixing cyanobacterium Trichodesmium IMS101: Physiological responses. Plant Physiol. 154, 334–345. doi: 10.1104/pp.110.159145
Kranzler, C., Lis, H., Finkel, O. M., Schmetterer, G., Shaked, Y., and Keren, N. (2014). Coordinated transporter activity shapes high-affinity iron acquisition in cyanobacteria. ISME J. 8, 409–417. doi: 10.1038/ismej.2013.161
Kühl, M., and Revsbech, N. P. (2000). “Biogeochemical microsensors for boundary layer studies,” in The Benthic Boundary Layer, eds B. Boudreau and B. B. Jørgensen (Oxford: Oxford University Press), 180–210.
Kustka, A., Sanudo-Wilhelmy, S. A., Carpenter, E. J., Capone, D., Burns, J., and Sunda, W. (2003). Iron requirements for dinitrogen- and ammonium-supported growth in cultures of Trichodesmium (IMS 101): comparison with nitrogen fixation rates and iron: carbon ratios of field populations. Limnol. Oceanogr. 48, 1869–1884. doi: 10.4319/lo.2003.48.5.1869
Lea-Smith, D. J., Bombelli, P., Vasudevan, R., and Howe, C. J. (2016). Photosynthetic, respiratory and extracellular electron transport pathways in cyanobacteria. Biochim. Biophys. Acta Bioenerg. 1857, 247–255. doi: 10.1016/j.bbabio.2015.10.007
Lee, M. D., Walworth, N. G., McParland, E. L., Fu, F. X., Mincer, T. J., Levine, N. M., et al. (2017). The Trichodesmium consortium: conserved heterotrophic co-occurrence and genomic signatures of potential interactions. ISME J. 11, 1813–1824. doi: 10.1038/ismej.2017.49
Levitan, O., Rosenberg, G., Setlik, I., Setlikova, E., Grigel, J., Klepetar, J., et al. (2007). Elevated CO2 enhances nitrogen fixation and growth in the marine cyanobacterium Trichodesmium. Glob. Chang. Biol. 13, 531–538. doi: 10.1111/j.1365-2486.2006.01314.x
Lis, H., Kranzler, C., Keren, N., and Shaked, Y. (2015). A comparative study of iron uptake rates and mechanisms amongst marine and fresh water cyanobacteria: prevalence of reductive iron uptake. Life 5, 841–860. doi: 10.3390/life5010841
Milligan, A. J., Berman-Frank, I., Gerchman, Y., Dismukes, G. C., and Falkowski, P. G. (2007). Light-dependent oxygen consumption in nitrogen-fixing cyanobacteria plays a key role in nitrogenase protection. J. Phycol. 43, 845–852. doi: 10.1111/j.1529-8817.2007.00395.x
Min, H., and Sherman, L. A. (2010). Hydrogen production by the unicellular, diazotrophic cyanobacterium Cyanothece sp. strain ATCC 51142 under conditions of continuous light. Appl. Environ. Microbiol. 76, 4293–4301. doi: 10.1128/aem.00146-10
Moore, C. M., Mills, M. M., Arrigo, K. R., Berman-Frank, I., Bopp, L., Boyd, P. W., et al. (2013). Processes and patterns of oceanic nutrient limitation. Nat. Geosci. 6, 701–710.
Paerl, H. W., and Bebout, B. M. (1988). Direct measurement of O2-depleted microzones in marine Oscillatoria: relation to N2 fixation. Science 241, 442–445. doi: 10.1126/science.241.4864.442
Polyviou, D., Baylay, A. J., Hitchcock, A., Robidart, J., Moore, C. M., and Bibby, T. S. (2018). Desert dust as a source of iron to the globally important diazotroph Trichodesmium. Front. Microbiol. 8:2683. doi: 10.3389/fmicb.2017.02683
Rabouille, S., Staal, M., Stal, L. J., and Soetaert, K. (2006). Modeling the dynamic regulation of nitrogen fixation in the cyanobacterium Trichodesmium sp. Appl. Environ. Microbiol. 72, 3217–3227. doi: 10.1128/aem.72.5.3217-3227.2006
Roe, K. L., and Barbeau, K. A. (2014). Uptake mechanisms for inorganic iron and ferric citrate in Trichodesmium erythraeum IMS101. Metallomics 11, 2042–2051. doi: 10.1039/c4mt00026a
Rubin, M., Berman-Frank, I., and Shaked, Y. (2011). Dust-and mineral-iron utilization by the marine dinitrogen-fixer Trichodesmium. Nat. Geosci. 4, 529–534. doi: 10.1038/ngeo1181
Saino, T., and Hattori, A. (1982). Aerobic nitrogen fixation by the marine non-heterocystous cyanobacterium Trichodesmium (Oscillatoria) spp.: its protective mechanism against oxygen. Mar. Biol. 70, 251–254. doi: 10.1007/bf00396843
Scranton, M. I. (1983). The role of the cyanobacterium Oscillatoria (Trichodesmium) thiebautii in the marine hydrogen cycle. Mar. Ecol. Prog. Ser. 11, 79–87. doi: 10.3354/meps011079
Scranton, M. I. (1984). Hydrogen cycling in the waters near Bermuda: the role of the nitrogen fixer, Oscillatoria thiebautii. Deep Sea Res. Part A Oceanogr. Res. Pap. 31, 133–143. doi: 10.1016/0198-0149(84)90020-7
Scranton, M. I., Novelli, P. C., Michaels, A., Horrigan, S. G., and Carpenter, E. J. (1987). Hydrogen production and nitrogen fixation by Oscillatoria thiebautii during in situ incubations. Limnol. Oceanogr. 32, 998–1006. doi: 10.4319/lo.1987.32.4.0998
Skizim, N. J., Ananyev, G. M., Krishnan, A., and Dismukes, G. C. (2012). Metabolic pathways for photobiological hydrogen production by nitrogenase- and hydrogenase-containing unicellular cyanobacteria Cyanothece. J. Biol. Chem. 287, 2777–2786. doi: 10.1074/jbc.M111.302125
Staal, M., Rabouille, S., and Stal, L. J. (2007). On the role of oxygen for nitrogen fixation in the marine cyanobacterium Trichodesmium sp. Environ. Microbiol. 9, 727–736. doi: 10.1111/j.1462-2920.2006.01195.x
Tamagnini, P., Axelsson, R., Lindberg, P., Oxelfelt, F., Wünschiers, R., and Lindblad, P. (2002). Hydrogenases and hydrogen metabolism of cyanobacteria. Microbiol. Mol. Biol. Rev. 66, 1–20. doi: 10.1128/mmbr.66.1.1-20.2002
Tamagnini, P., Leitão, E., Oliveira, P., Ferreira, D., Pinto, F., Harris, D. J., et al. (2007). Cyanobacterial hydrogenases: diversity, regulation and applications. FEMS Microbiol. Rev. 31, 692–720. doi: 10.1111/j.1574-6976.2007.00085.x
Wilson, S. T., del Valle, D. A., Robidart, J. C., Zehr, J. P., and Karl, D. M. (2013). Dissolved hydrogen and nitrogen fixation in the oligotrophic North Pacific subtropical gyre. Environ. Microbiol. Rep. 5, 697–704. doi: 10.1111/1758-2229.12069
Wilson, S. T., Foster, R. A., Zehr, J. P., and Karl, D. M. (2010). Hydrogen production by Trichodesmium erythraeum,. Aquat. Microb. Ecol. 59, 197–206. doi: 10.3354/ame01407
Wilson, S. T., Kolber, Z. S., Tozzi, S., Zehr, J. P., and Karl, D. M. (2012). Nitrogen fixation, hydrogen cycling, and electron transport kinetics in Trichodesmium erythraeum (cyanobacteria) strain IMS101. J. Phycol. 48, 595–606. doi: 10.1111/j.1529-8817.2012.01166.x
Keywords: Trichodesmium, colony, N2 fixation, H2 evolution, uptake hydrogenase, O2 fluxes, iron acquisition, dust
Citation: Eichner M, Basu S, Gledhill M, de Beer D and Shaked Y (2019) Hydrogen Dynamics in Trichodesmium Colonies and Their Potential Role in Mineral Iron Acquisition. Front. Microbiol. 10:1565. doi: 10.3389/fmicb.2019.01565
Received: 25 April 2019; Accepted: 24 June 2019;
Published: 10 July 2019.
Edited by:
Mar Benavides, Institut de Recherche pour le Développement (IRD), FranceReviewed by:
Samuel T. Wilson, University of Hawai‘i at Mānoa, United StatesMary I. Scranton, Stony Brook University, United States
Copyright © 2019 Eichner, Basu, Gledhill, de Beer and Shaked. This is an open-access article distributed under the terms of the Creative Commons Attribution License (CC BY). The use, distribution or reproduction in other forums is permitted, provided the original author(s) and the copyright owner(s) are credited and that the original publication in this journal is cited, in accordance with accepted academic practice. No use, distribution or reproduction is permitted which does not comply with these terms.
*Correspondence: Meri Eichner, ZWljaG5lckBhbGdhLmN6
†Present address: Meri Eichner, Laboratory of Photosynthesis, Centre Algatech, Institute of Microbiology of the Czech Academy of Sciences, Třeboň, Czechia