- 1Department of Clinical Laboratory, The First Affiliated Hospital of Nanchang University, Nanchang, China
- 2Department of Tuberculosis, Jiangxi Chest Hospital, Nanchang, China
- 3Department of Clinical Laboratory, Xiangya Hospital, Central South University, Changsha, China
- 4Department of Clinical Laboratory, The Second Affiliated Hospital of Nanchang University, Nanchang, China
The roles and characteristics of low-density granulocytes (LDGs) have recently attracted attention; however, the mechanism of the formation of LDGs is yet unclear. In one of our previous studies, the frequency of LDGs was significantly elevated in the peripheral blood of tuberculosis patients, and in situ activation contributed to the generation of LDGs upon Mycobacterium tuberculosis infection. However, the underlying molecular mechanisms are yet to be elucidated. In the present study, the release of neutrophil extracellular traps (NETs) and the levels of ROS were regulated before the normal-density granulocytes (NDGs) to be infected with M. tuberculosis, and the conversion of NDGs to LDGs was monitored subsequently as well. The results showed that tuberculosis-related LDGs spontaneously released high levels of NETs. Promoting the release of NETs led to increase in the conversion of NDGs to LDGs in M. tuberculosis infection, while inhibiting the release of NETs suppressed this conversion after the infection. The M. tuberculosis infection significantly increased the ROS levels in neutrophils and the conversion of NDGs to LDGs. Scavenging ROS or blocking the ROS generation of M. tuberculosis-infected NDGs significantly suppressed the release of NETs and blocked the generation of LDGs. Moreover, inhibiting the formation of NETs without affecting the levels of ROS significantly decreased the conversion of NDGs to LDGs after M. tuberculosis infection. Overall, this study demonstrated that M. tuberculosis could induce the generation of LDGs by promoting the release of NET via ROS pathway.
Introduction
Tuberculosis is a chronic infectious disease caused by Mycobacterium tuberculosis infection, which is the ninth leading cause of deaths worldwide and the leading cause of deaths from a single infectious agent. Approximately, one-third of the world’s population is estimated to be infected by M. tuberculosis. About 10.4 million cases of tuberculosis occur and 1.7 million individuals succumb to death due to this disease annually (World Health Organization, 2017). M. tuberculosis does not produce any toxin, exotoxin, or invasive enzymes. The pathological damage by tuberculosis is primarily mediated by the pathological immune response to M. tuberculosis infection (Mourik et al., 2017; Scriba et al., 2017). Neutrophils are the first cells that infiltrate in the local tissue after M. tuberculosis infection and are the most abundant leukocytes in the airways of patients with pulmonary tuberculosis (Eum et al., 2010). Although some studies suggested that neutrophils might be involved in tuberculosis-related tissue damage and effectuate the activation of latent tuberculosis, however, the specific roles and characteristics of neutrophils in TB infection still need to be elucidated.
Recent studies described a specific subpopulation of neutrophils, termed as low-density granulocytes (LDGs) or low-density neutrophils (LDNs) in the peripheral blood of patients with autoimmune diseases, cancer, and infection (Hacbarth and Kajdacsy-Balla, 1986; Carmona-Rivera and Kaplan, 2013; Sagiv et al., 2015). LDGs are defined as such because these cells are found to sediment in the peripheral blood mononuclear cell (PBMC) fraction after density gradient centrifugation of the peripheral blood (Hacbarth and Kajdacsy-Balla, 1986). Notably, LDGs display different phenotype, maturation status, and function in different studies. Several studies demonstrated that LDGs are activated mature neutrophils that mediate enhanced proinflammatory and cytotoxic responses (Moses and Brandau, 2016). However, some other studies provided evidence to prove that LDGs are immature neutrophils that display immunosuppressive properties (Denny et al., 2010; Grayson et al., 2015).
In a previous study, we found an aberrantly high level of LDGs in the peripheral blood of patients with active tuberculosis, and the frequencies of LDGs were correlated with the severity of tuberculosis. This phenotype suggested the role of these cells in the occurrence of tuberculosis and tissue damage caused by the disease (Deng et al., 2016). Furthermore, we found that the in vitro infection with M. tuberculosis induces the generation of LDGs in both peripheral blood and purified normal-density granulocytes (NDGs) and that the in situ activation contributes to the generation of LDGs in M. tuberculosis infection (Deng et al., 2016). However, the underlying molecular mechanisms are still unknown.
Since neutrophils are primarily involved in the tissue damage in tuberculosis, and LDGs are a group of highly activated neutrophils, eliminating the mechanisms that generate the tuberculosis-related LDGs might be crucial for developing the techniques to block the generation of LDGs and promoting the treatment of active tuberculosis. In this study, we reported that the generation of M. tuberculosis infection-related LDGs is related to the intracellular ROS level and the release of neutrophil extracellular traps (NETs) in neutrophils.
Materials and Methods
Study Subjects
Patients with pulmonary tuberculosis were recruited from the Jiangxi Chest Hospital from April 2017 to July 2017. The diagnostic criteria for tuberculosis were in accordance with the “Guideline of Clinical Diagnosis and treatment: TB section of China” (Chinese Medical Association, 2005). Age- and sex-matched, TST-negative asymptomatic healthy volunteers were recruited from April 2017 to February 2018. All subjects with autoimmune diseases, cancer, current or recent infection were excluded from the study.
This study was approved by the Ethical Review Committees of the First Affiliated Hospital of Nanchang University and was carried out in compliance with the Helsinki Declaration. Written informed consent was obtained from all the subjects enrolled in this study.
Neutrophils Preparation
Peripheral venous blood was collected in vacuum containers containing EDTA-anticoagulant and processed within 4 h of phlebotomy. PBMCs were isolated by density gradient centrifugation with Ficoll-amidotrizoaat (LUMC, Leiden, Netherlands) as described previously (Deng et al., 2016). Briefly, 3 ml of venous blood was diluted 1:1 with sterile saline and centrifuged on Ficoll for 30 min at 300 ×g, 4°C. The PBMCs were collected by aspiration from the plasma-lymphocyte interface. The contaminating RBCs were lysed by erythrocyte lysis (OptiLyse C lysing solution, Beckman Coulter, Brea, CA, USA). The LDGs were purified from PBMC fraction by an immunomagnetic negative selection method using an EasySep Direct Human Neutrophil Isolation Kit (STEMCELL Technologies, Vancouver, BC, Canada) according to the manufacturer’s instructions. The purity of the LDG fraction was typically >95% as determined by staining with CD15-PE-Cy5 and CD14-ECD and analyzing with flow cytometry (FCM). The LDGs were identified as CD14low CD15+ granulocytes (Deng et al., 2016). The NDGs were isolated by dextran sedimentation from RBC pellets and erythrocyte lysis. The viability of the cells was monitored by trypan blue staining. Subsequently, the freshly prepared neutrophils were resuspended at a density of 5 × 105 cells/ml in RPMI 1640 medium (Life Technologies, Carlsbad, CA, USA) supplemented with 3% heat-inactivated human AB sera (Sigma-Aldrich, St. Louis, MO, USA).
Flow Cytometry Analysis
The PBMCs or neutrophils (106 cells each) were washed and incubated with FcR blocking reagent (Milteny Biotec, Auburn, CA, USA) for 5 min, followed by incubation at room temperature for 30 min with or without the mixture of fluorescent-labeled anti-human monoclonal antibodies: CD14-ECD and CD15-PE-Cy5 (Beckman Coulter, Brea, CA, USA). The dead cells were eliminated from the analysis as deduced by staining with Annexin V-FITC and propidium iodide. The cell doublets were eliminated based on the size of the event (FSC). The analysis was performed using a Cytomics FC 500 flow cytometer (Beckman Coulter, Brea, CA, USA), and isotype-matched controls were used to determine auto-fluorescence and nonspecific staining.
Bacterial Strains and Infection
M. tuberculosis H37Rv strain was cultured at 37°C up to early mid-log phase in Middlebrook 7H9 broth, supplemented with 10% OADC growth supplement (BD Bioscience, San Jose, CA, USA) and 0.5% glycerol. To obtain single cell suspension, the bacterial aggregates were diluted in RPMI 1640 medium, mixed with a pipette, and treated with an ultrasonic shaker for 15 min. The solution was left standing for 15 min before the supernatant was collected and density adjusted to 0.5 at OD600 (approximately 107 bacteria/ml). For in vitro infection, the purified neutrophils were infected with M. tuberculosis at the multiplicity of infection (MOI) of 5 (bacteria to neutrophils) as described previously (Deng et al., 2016). Furthermore, 20 nM phorbol 12-myristate 13-acetate (PMA, Sigma-Aldrich, St. Louis, MO, USA), 10 μM N,N,N,N,-tetrakis-2(pyridyl-methyl) ethylenediamine (TPEN, Sigma-Aldrich), 50 μM hydrogen peroxide (H2O2, Sigma-Aldrich), 25 μM diphenyleneiodonium (DPI, Sigma-Aldrich), 30 μM N-acetyl-L-cysteine (NAC, Sigma-Aldrich), or 200 μM Cl-amidine (AAT Bioquest, Sunnyvale, CA, USA) was added to the culture for 30 min before infection.
Determination of Normal-Density Granulocyte-to-Low-Density Granulocyte Conversion
Purified NDGs were pretreated with or without PMA, TPEN, NAC, H2O2, DPI, or Cl-amidine for 30 min, and then infected with or without M. tuberculosis for 2 h at MOI 5. After washing three times with phosphate-buffered saline (PBS), the treated cells were resuspended in 3 ml of sterile saline and added to 3 ml of freshly collected autologous whole blood. Immediately after the treated cells were mixed into the autologous blood, the PBMCs in this mixture and in another equal division of untreated autologous peripheral blood were isolated by Ficoll density gradient centrifugation. The LDGs in these PBMCs were identified as CD14low CD15+ cells and enumerated by FCM. The number of LDGs in the treated neutrophil samples was calculated by subtracting the number of LDGs in the untreated autologous blood with LDGs in the mixture.
Determination of Neutrophil Extracellular Traps
After in vitro culture with or without stimulation or infection, the neutrophils were fixed with 4% formaldehyde, permeabilized by 0.1% Triton X-100, and then blocked with 5% bovine serum albumin (BSA) for 1 h. The formation of NETs was detected as described previously (Zhu et al., 2018). Briefly, the cells were incubated with the anti-myeloperoxidase antibody (MPO; ab45977; Abcam, Cambridge, UK), followed by secondary antibody coupled with Alexa Fluor 488 (Thermo Fisher Scientific, Waltham, MA, USA). After removal of the unbound antibodies, DNA was stained using 4′,6-diamidino-2-phenylindole (DAPI). At least two independent observers manually quantified the neutrophils and NETs. The NETs were identified as structures positive for both MPO and DAPI. The images were acquired with an Olympus IX-71 microscope (Olympus, Tokyo, Japan) at magnification of 200×. The percentage of NET-releasing cells was calculated as an average of 10 random fields normalized to the total number of neutrophils.
In order to further confirm the result of immunofluorescence staining, the QuantiFluor dsDNA System (Promega, Madison, WI, USA) was used to quantify the levels of cell-free DNA (cfDNA) in the culture supernatant of neutrophils according to the manufacturer’s instructions. The fluorescence (504 nm Ex/531 nm Em) was measured using a plate reader.
Measurement of Intracellular ROS
The levels of intracellular ROS were measured using the oxidation-sensitive fluorescent dye H2DCF-DA (20,70-dichlorodihydrofluorescein diacetate, Invitrogen, Carlsbad, CA, USA). Briefly, the cells were loaded with 10 μM H2DCF-DA fluorescence probe, and incubated at room temperature for 30 min in the dark, followed by that in ice bath for 5 min to quench the reaction. Then, the cells were washed one time with PBS, and ROS levels were analyzed immediately by FCM.
Statistical Analysis
Values were presented as mean ± standard deviation (SD) or mean ± standard error of the mean (SEM), and analyzed using GraphPad Prism 5.01 software. p’s were derived from the one-way ANOVA test with post hoc analysis and Kruskal-Wallis test. Differences were considered statistically significant at p < 0.05.
Result
Low-Density Granulocytes in TB Patients Release High Level of Neutrophil Extracellular Traps
In a previous study, we found that LDGs in TB patients might originate from in situ activation, with increased expression of membrane CD15 and CD66b and decreased expression of membrane CD62L (Deng et al., 2016). Some studies showed that LDGs in patients with autoimmune diseases, such as SLE and RA, spontaneously released high levels of NETs (Zhang et al., 2017; Kanamaru et al., 2018). These studies hinted to us that LDGs in TB patients might also result in high level of NETs. To confirm this hypothesis, LDGs and autologous NDGs in patients with tuberculosis were isolated and cultured in RPMI 1640 medium containing 3% human serum AB. The baseline level of NET was detected using immunofluorescence staining with anti-MPO antibody and DAPI staining. The extracellular NET DNA was qualified by the QuantiFluor dsDNA System. In addition, the levels of NET formation were detected after stimulation with 20 nM PMA. The results showed that LDGs obtained from TB patients spontaneously released a higher level of NETs than autologous NDGs. However, NDGs released a significantly high level of NETs than LDGs after stimulation of PMA (Figure 1).
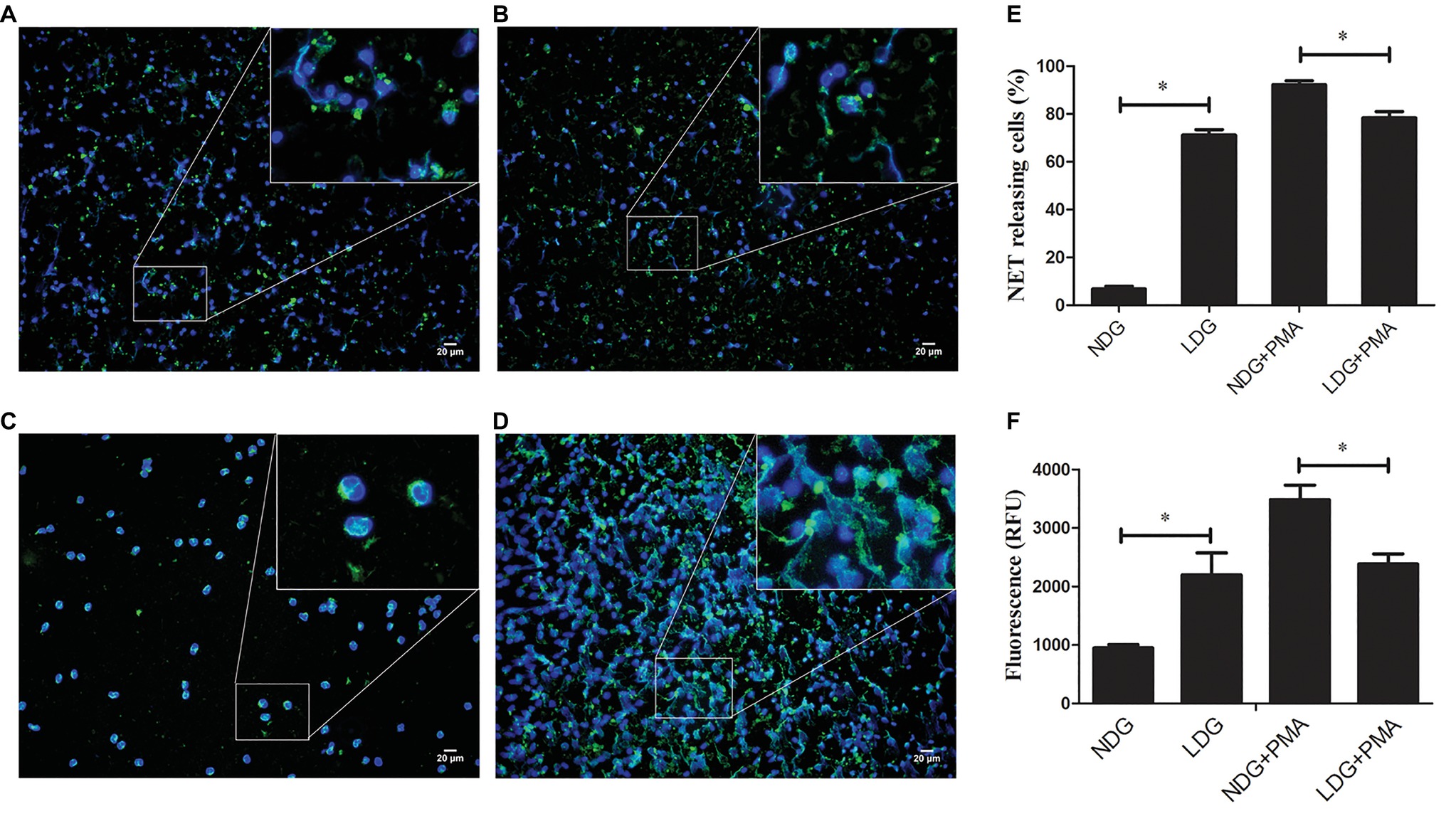
Figure 1. LDGs from tuberculosis patients spontaneously released a high level of NETs. LDGs (A,B) and NDGs (C,D) were isolated from TB patients and cultured in RPMI 1640 medium containing 3% human serum AB. The cells were either untreated (A,C) or stimulated with phorbol 12-myristate 13-acetate (PMA, 20 nM) for 2 h (B,D). MPO was detected using specific antibody (green). Nuclei and extracellular DNA were stained blue with DAPI. The dual-labeled immunofluorescence staining is shown as a merged figure at ×200 as a representative image from 1/5 patients. (E) The percentage of NET-releasing cells was calculated manually by at least two independent observers in 10 fields and normalized to the total number of neutrophils, and the results were expressed as mean ± standard error of the mean (SEM) of at least three independent experiments. p’s were derived from Kruskal-Wallis test. (F) The levels of cfDNA in the culture supernatant were determined by QuantiFluor dsDNA System, and data were expressed as mean ± standard deviation (SD) of at least three independent experiments. Statistical significance was determined by one-way analysis of variance (ANOVA) followed by post hoc test (*p < 0.05).
These results indicated that LDGs in TB patients are primed cells, which spontaneously form a high level of NETs. The morphological observation revealed that the nucleus of LDGs was relatively loose than that in NDGs (Figure 2), which demonstrated chromatin decondensation and release of NETs. Besides, LDGs that originated from peripheral blood of healthy controls by M. tuberculosis infection were shown to release a higher level of NETs than those by autologous NDGs (Figure 3).
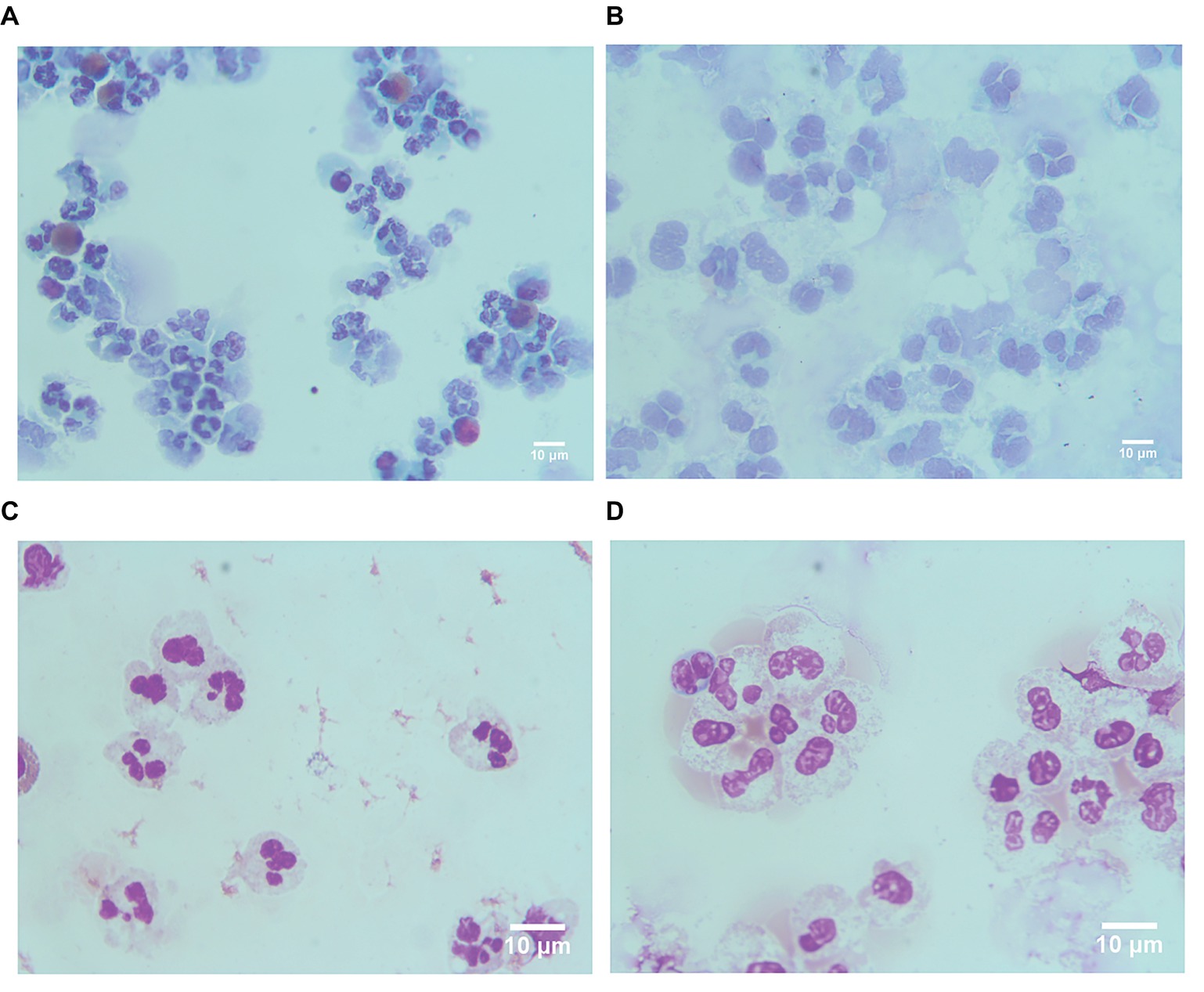
Figure 2. LDGs originated from TB patients show loose nucleus. LDGs (A,B) and NDGs (C,D) were separated from the TB patient, stained by Papanicolaou stain (A,C) and Wright’s stain (B,D), and observed under a microscope (×1,000 magnification).
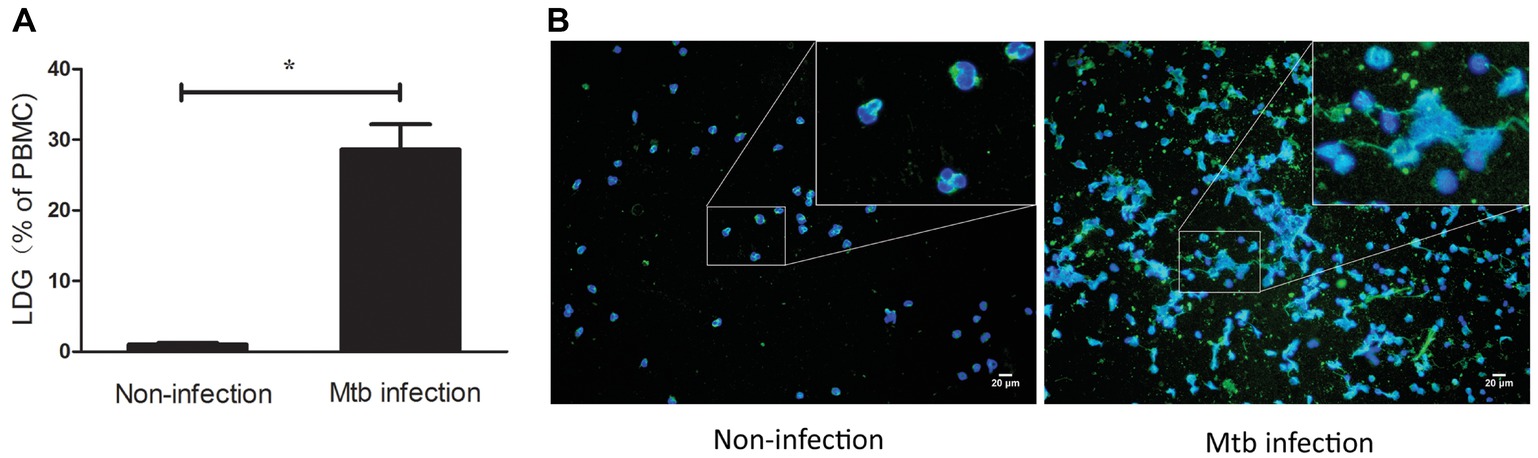
Figure 3. LDGs originated from in vitro M. tuberculosis infection released a high level of NETs. Freshly collected whole blood from healthy controls was infected with M. tuberculosis for 2 h and centrifuged by Ficoll density gradient centrifugation. LDGs in the PBMCs were identified as CD14low CD15+ cells and enumerated by FCM. Data are represented as mean ± SD of at least three independent experiments and the statistical significance was determined by unpaired t-test (*p < 0.05) (A). NDGs from the RBC fraction and LDGs from the PBMC fraction were isolated individually by the immunomagnetic negative selective method, stained with anti-MPO (green) antibody and DAPI (blue), and observed under a fluorescence microscope (B) (×200).
Release of Neutrophil Extracellular Traps Is a Mechanism of Low-Density Granulocyte Generation
The phenomenon that LDGs purified from both TB patients and those induced from NDGs by M. tuberculosis infection could release high levels of NETs prompting the necessity to investigate whether release of NET plays a role in the generation of LDGs. LDGs were purified from TB patients and induced from NDGs by M. tuberculosis infection, which released high levels of NETs, thereby necessitating to understand the role of NET release in the generation of LDGs. To explore the putative role of NETs’ release in the generation of LDGs, purified NDGs from healthy controls were pretreated with or without TPEN, a membrane-permeable Zn2+-selective chelator, which is essential for NET formation in neutrophils (Hasan et al., 2013) for 30 min, followed by stimulation with NET inducer PMA (Brinkmann et al., 2004) for 2 h. The release of NETs and the conversion of NDGs to LDGs were determined as described in Section “Materials and Methods.” The results showed that LDGs in PBMCs could be distinguished clearly based on the expression of the neutrophil marker CD15 and the monocyte marker CD14, and the conversion of NDGs to LDGs could be calculated with good repeatability (Figure 4B). Additionally, PMA significantly promoted the release of NETs accompanied by the increase in LDG generation. However, when the release of NET was inhibited by TPEN, the generation of LDG was also significantly inhibited upon stimulation with PMA (Figure 4).
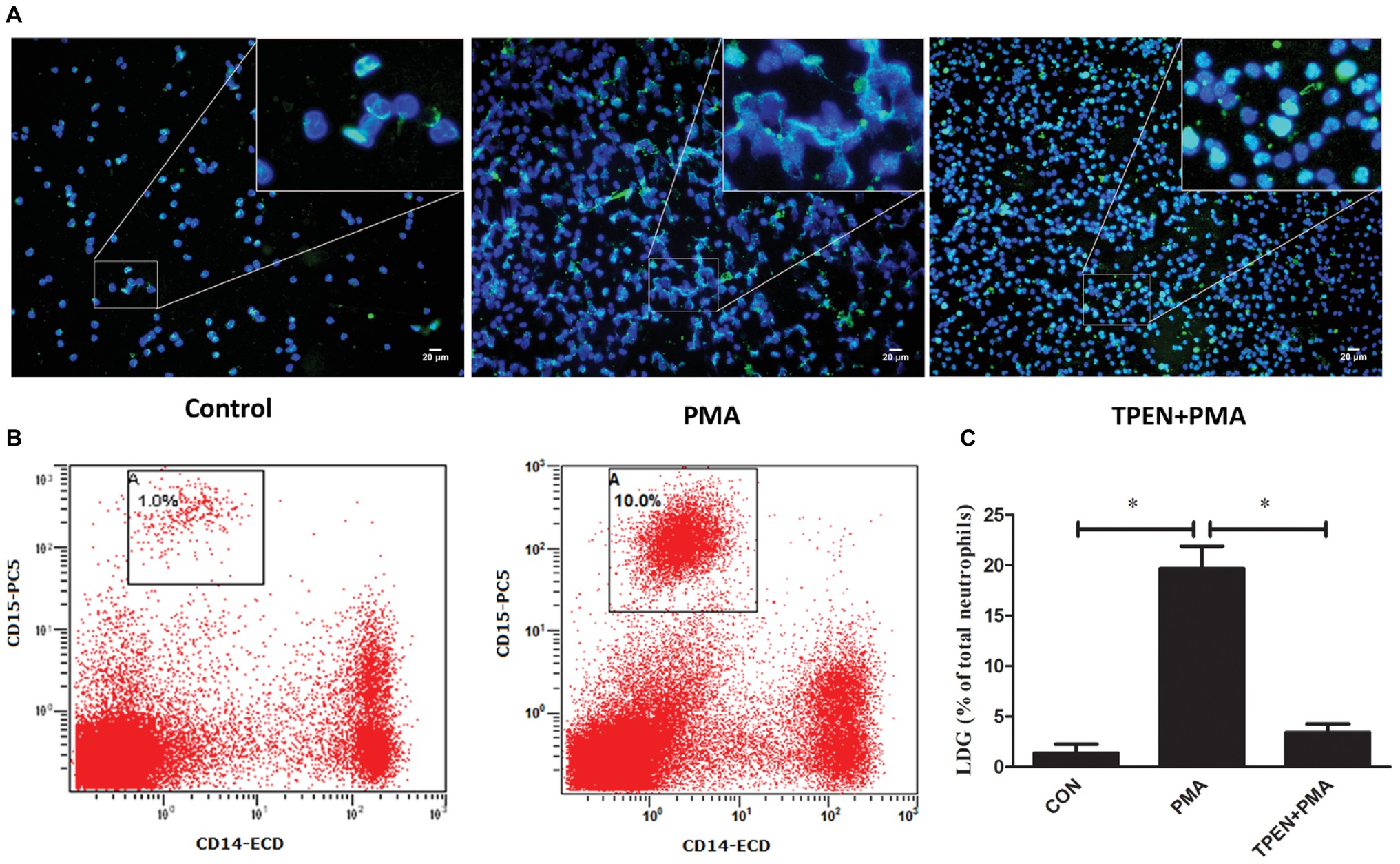
Figure 4. Prompting the release of NETs induces the conversion of NDGs to LDGs. NDGs were separated from healthy controls and pretreated with or without TPEN (10 μM) for 30 min, followed by stimulation with PMA (20 nM) for 2 h. (A) The release of NETs was detected by immunofluorescence staining. Nuclei were stained with DAPI (blue), and MPO (green) was detected using specific antibody. The dual-labeled immunofluorescence staining is shown as a merged figure. The magnification was ×200. (B) Neutrophils were washed three times, resuspended in 3 ml of sterile saline, and added into 3 ml of freshly collected autologous whole blood. PBMCs in this mixture and another equal division of untreated autologous peripheral blood were immediately isolated by Ficoll density gradient centrifugation. LDGs in these PBMCs were identified as CD14low CD15+ cells and enumerated by FCM. (C) The number of LDGs in stimulated neutrophils was calculated by subtracting the number of LDGs in the untreated blood with LDGs in the mixture. The results are expressed as mean ± SD of at least three independent experiments, and the statistical significance was determined by one-way ANOVA followed by post hoc test (*p < 0.05).
To further confirm the association between NET release and LDG generation upon M. tuberculosis infection, NDGs from healthy controls were untreated or pretreated with TPEN, the inhibitor of NET formation, or PMA, the inducer of NET formation, for 30 min, followed by infection with M. tuberculosis at MOI 5. The results showed that PMA further increased the release of NETs in neutrophils and promoted the generation of LDGs post-M. tuberculosis infection. Conversely, TPEN significantly inhibited the release of NETs, as well as, the generation of LDGs induced by M. tuberculosis infection (Figure 5).
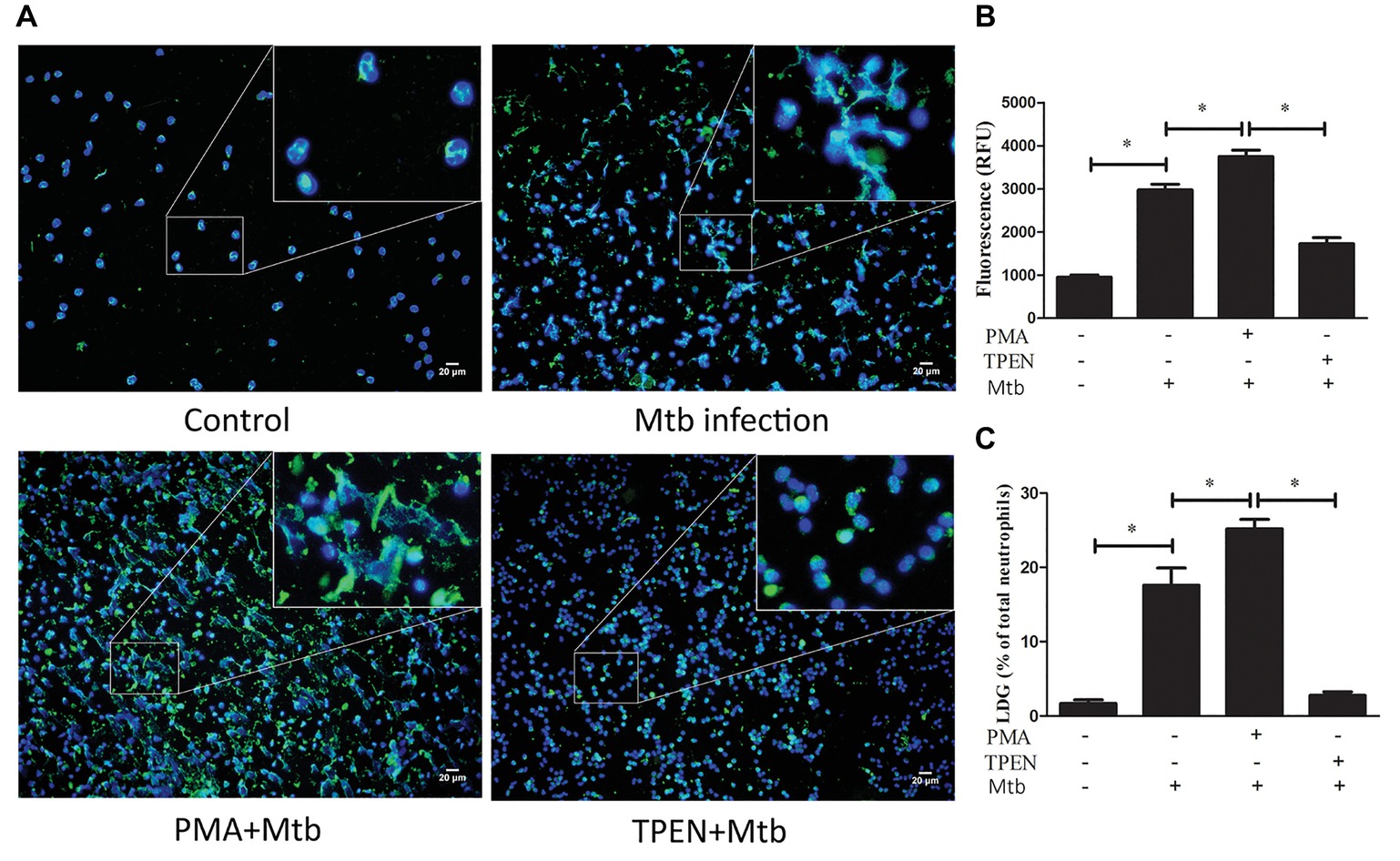
Figure 5. The levels of NET release regulate the conversion of NDGs to LDGs upon M. tuberculosis infection. NDGs were isolated from healthy controls, and either untreated or pretreated with TPEN (10 μM) or PMA (20 nM) for 30 min, followed by infection with M. tuberculosis (Mtb) for 2 h at the MOI of 5. (A) The release of NETs was detected by immunofluorescence staining. Nuclei were stained with DAPI (blue), and MPO (green) was detected with specific antibody. The dual-labeled immunofluorescence staining is shown as a merged figure at ×200. (B) The levels of cfDNA in the culture supernatant were determined by QuantiFluor dsDNA System, and results are expressed as mean ± SD of at least three independent experiments. (C) Neutrophils were washed three times and resuspended in 3 ml of sterile saline, and then added into 3 ml of freshly collected autologous whole blood. PBMCs in this mixture and a proportion of untreated autologous peripheral blood were immediately isolated by Ficoll density gradient centrifugation. LDGs in these PBMCs were identified as CD14low CD15+ cells and counted by FCM. The number of LDGs in these mixtures was calculated by subtracting the number of LDGs in the untreated blood with LDGs in the mixture. The results are expressed as mean ± SD of at least three independent experiments, and the statistical significance was determined by one-way ANOVA followed by post hoc test (*p < 0.05).
ROS Are Involved in the Generation of Low-Density Granulocytes
The above results demonstrated that NETs’ release is one of the mechanisms of M. tuberculosis infection-related LDG generation. Previous studies have proved that the formation of NETs is dependent on the production of ROS (Fuchs et al., 2007; Papayannopoulos et al., 2010). Our previous study also found that LDGs from TB patients produced increased levels of ROS than autologous NDGs (Deng et al., 2016). These studies suggested that ROS might be involved in the generation of TB-related LDGs. To confirm this hypothesis, purified NDGs from healthy controls were infected with or without M. tuberculosis, and the intracellular ROS levels were detected. The results showed that the in vitro infection with M. tuberculosis significantly increased the levels of intracellular ROS and promoted the conversion of NDGs to LDGs (Figures 6A,B). In combination with our previous findings (Deng et al., 2016), the present results showed that both in vitro and in vivo infection of M. tuberculosis significantly increased the intracellular levels of ROS in neutrophils and the frequency of LDGs.
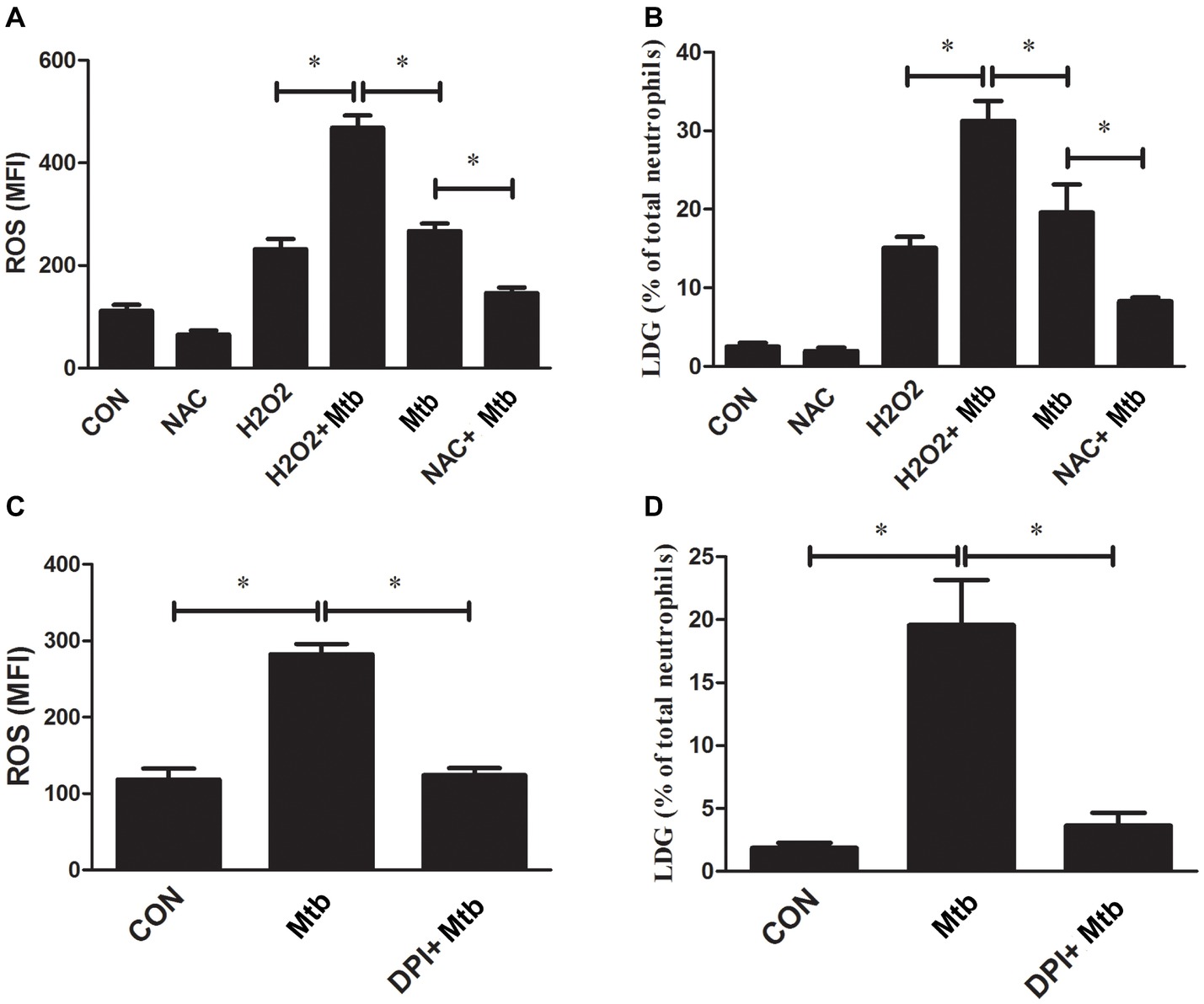
Figure 6. The level of ROS regulates the generation of LDG. NDGs were isolated from healthy controls and either untreated or pretreated with NAC (30 μM) or H2O2 (50 μM) for 30 min, and then, infected with or without M. tuberculosis for 2 h. (A) Cells were loaded with H2DCF-DA fluorescence probe (10 μM), and the intracellular ROS levels were determined by FCM. (B) The generation of LDGs was detected as described above. (C,D) NDGs were separated from healthy controls and pretreated with or without DPI (25 μM) for 30 min, followed by infection with M. tuberculosis for 2 h. The intracellular ROS levels in neutrophils and generation of LDNs were detected as described above. The results are expressed as mean ± SD of at least three independent experiments and the statistical significance was determined by one-way ANOVA and post hoc test (*p < 0.05).
Next, purified NDGs from healthy controls were pretreated with the ROS scavenger NAC or oxidizer H2O2 for 30 min, followed by infection with M. tuberculosis for 2 h and detection of intracellular ROS levels and generation of LDGs. The results confirmed that the formation of NETs was associated with the levels of ROS (data not shown), and showed that H2O2 significantly increased the levels of intracellular ROS in neutrophils accompanied by the increased level of LDG generation even without an infection. Also, H2O2 further increased the level of LDG generation under M. tuberculosis infection. However, NAC pretreatment significantly decreased the ROS levels in M. tuberculosis-infected NDGs and suppressed the conversion of NDGs to LDGs upon M. tuberculosis infection (Figures 6A,B).
To further confirm the association between ROS and M. tuberculosis infection-related LDGs, purified NDGs from healthy controls were untreated or pretreated for 30 min with DPI, an inhibitor of neutrophil NADPH oxidase, and then infected with M. tuberculosis. The generation of LDGs was detected at 2 h post-infection. Data showed that DPI suppressed the generation of intracellular ROS in neutrophils and inhibited the generation of M. tuberculosis-induced LDGs (Figures 6C,D).
ROS Induce the Generation of Low-Density Granulocytes by Regulating the Formation of Neutrophil Extracellular Traps
The results indicated that the increased ROS level promotes the formation of NETs and the generation of LDGs, while the inhibition of ROS production can decrease the release of NETs and the generation of LDGs after M. tuberculosis infection. These observations demonstrated that ROS could promote the generation of M. tuberculosis-related LDGs, probably by regulating the formation of NET. To verify this hypothesis, Cl-amidine, an inhibitor of PAD4 which was commonly used as the chromatin decondensation and NET formation but did not affect the levels of intracellular ROS, was used to pretreat the purified NDGs from healthy controls for 30 min before M. tuberculosis infection. The results showed that Cl-amidine significantly inhibited the release of NETs after the infection. Although M. tuberculosis infection induced similar levels of intracellular ROS in neutrophils of both groups, fewer neutrophils that received Cl-amidine pretreatment were converted to LDGs as compared to those that did not receive pretreatment (Figure 7).
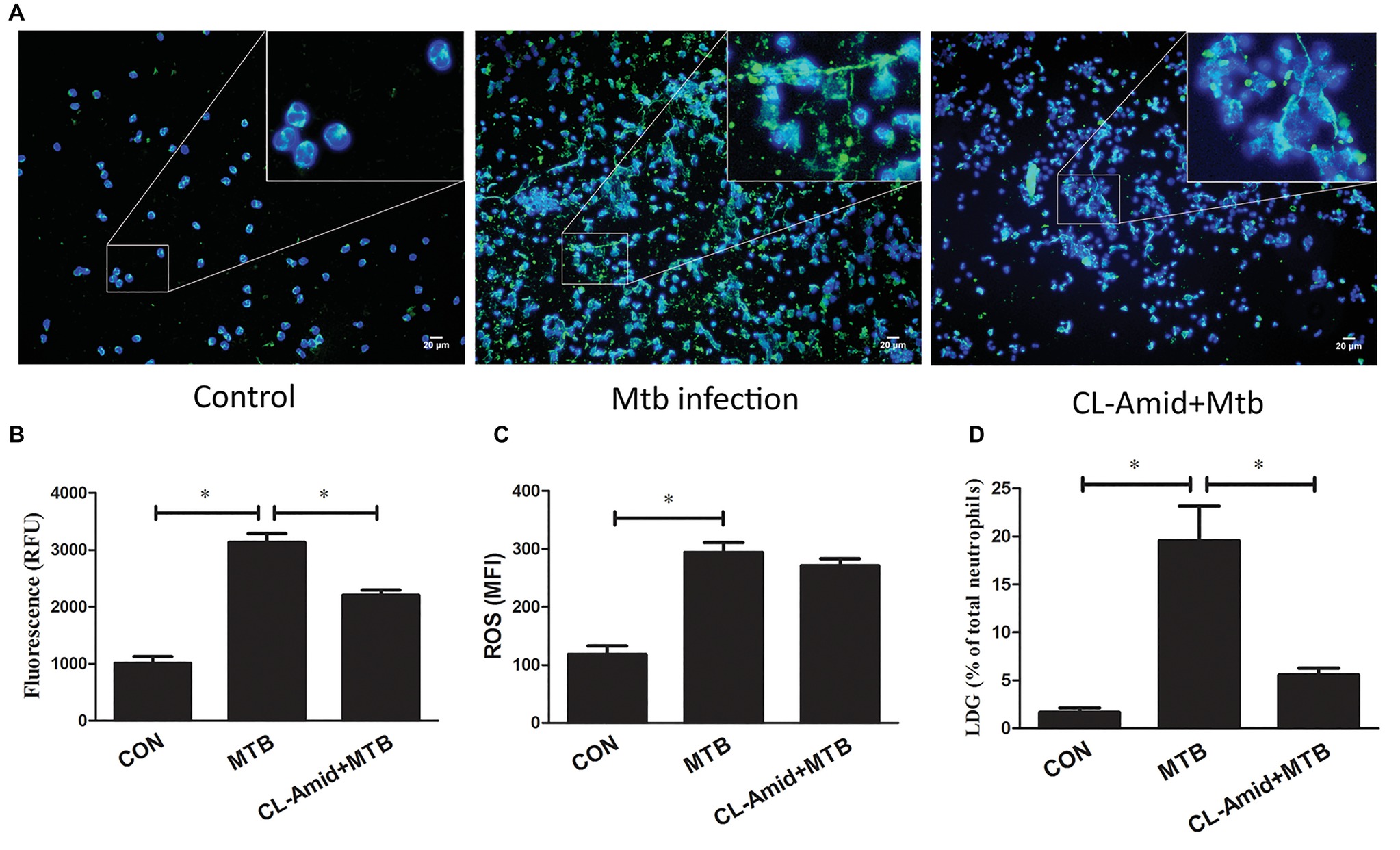
Figure 7. NDGs were separated from healthy controls, those either untreated or pretreated with Cl-amidine (200 μM) for 30 min, and infected with M. tuberculosis for 2 h at the MOI of 5. (A) The formation of NETs was detected by dual-labeled immunofluorescence. Nuclei were stained with DAPI (blue), and MPO (green) was detected using specific antibody. (B) The levels of cfDNA in the culture supernatant were determined by QuantiFluor dsDNA System. (C) The intracellular ROS levels were determined by FCM after the loading of H2DCF-DA fluorescence probe (10 μM). (D) The generation of LDGs was detected as described above. The results are expressed as mean ± SD of at least three independent experiments, and the statistical significance was determined by one-way ANOVA, followed by post hoc test. *p < 0.05. CON, group received no treatment; Mtb, M. tuberculosis infection.
Discussion
The roles and characteristics of LDG are currently under intensive focus in the fields of cancer, autoimmune diseases, and infectious diseases, wherein, the fluctuation in the LDG proportion presents a correlation with the severity of the disease and responsiveness to the treatment (Cloke et al., 2012; Carmona-Rivera and Kaplan, 2013; Sagiv et al., 2015; Deng et al., 2016). The variety in immunophenotype and specific functions in different diseases makes the LDGs be categorized into two main subgroups, immunosuppressive and pro-inflammatory. In some diseases such as solid tumors and hematological malignancies, LDGs were also named as granulocyte-like myeloid-derived suppressor cells (G-MDSCs) (Sagiv et al., 2015; Moses and Brandau, 2016). While in other conditions such as anti-neutrophil cytoplasmic antibody-associated vasculitis and chronic granulomatous disease, LDGs are mainly characterized as pro-inflammatory cells that mediate enhanced proinflammatory and cytotoxic responses as compared to those of autologous NDGs (Denny et al., 2010; Grayson et al., 2015). Our previous study revealed that LDGs in patients with tuberculosis are activated proinflammatory cells with mature neutrophils phenotype (Deng et al., 2016).
Recent studies suggested that LDGs might be involved in the occurrence and development of some diseases and may be a promising target for the treatment (Grayson et al., 2015; Rocha et al., 2015; Liu et al., 2017). Thus, the origin of LDGs in different conditions has also attracted increasing attention. A study based on the cytogenetic microarray analysis demonstrated that LDGs in SLE patients are a kind of immature neutrophil that result from disruption of granulocyte development (Singh et al., 2014). However, another study in SLE patient proposed that LDGs arise as a consequence of in situ activation of normal neutrophils (Garcia-Romo et al., 2011), which was confirmed subsequently in a murine model of cancer, wherein NDGs exposed to transforming growth factor beta 1 (TGF-β1) were switched into LDGs (Sagiv et al., 2015). Our recent study also proved that the in situ activation contributes to the generation of LDGs in M. tuberculosis infection (Deng et al., 2016).
Nonetheless, the molecular mechanism underlying the generation of LDGs is yet to be elucidated. We found that LDGs in patients with tuberculosis is a population of activated neutrophils that originated from in situ activation. The present study demonstrated that LDGs from TB patients spontaneously released a higher level of NETs than that by autologous NDGs, but released fewer NETs than the autologous NDGs after PMA stimulation. This phenomenon indicated that LDGs in TB patients are primed cells, which spontaneously form high level of NETs. These results are in agreement with the previous reports in other disease models (Denny et al., 2010; Fu et al., 2014; Zhang et al., 2017).
NETs are complex structures consisting of extracellular chromatin decorated with granular and cytoplasmic proteins, such as MPO and neutrophil elastase (Brinkmann et al., 2004). NETs have been described as a beneficial mechanism of host defense against pathogens that can immobilize and kill the pathogens. However, recent studies suggested that NET may be detrimental in various diseases such as cancer and atherosclerosis (Brinkmann et al., 2004; Nauseef, 2007; Kaplan and Radic, 2012). Consistently, NETs have also been shown to be released by M. tuberculosis-infected neutrophils. And, it was found that NETs could not kill M. tuberculosis, but was closely related to tuberculosis-related lung tissue damage (Ramos-Kichik et al., 2009; de Melo et al., 2019). The release of NETs is the result of several successive events. First, stimuli such as PMA, LPS, and bacteria activate the neutrophils. Then, the nuclear chromatin decondenses and the nuclear envelope disintegrates, followed by the release of nuclear content into the cytoplasm, and the nuclear chromatin, cytoplasmic, and granular components are mixed. Ultimately, the cell membrane ruptures and the mixture is released into the extracellular space by a process that is distinct from necrosis or apoptosis (Brinkmann et al., 2004; Yang et al., 2016).
PMA is the most common inducer for the formation of NETs (Brinkmann et al., 2004; Fuchs et al., 2007). In order to reveal whether the release of NETs plays a role in the generation of LDGs, purified NDGs were treated with PMA to trigger the release of NETs, and the conversion of NDGs to LDGs after M. tuberculosis infection was determined. The results showed that PMA significantly promoted the release of NETs accompanied by the increase in the generation of LDGs. In addition, the pretreatment with PMA further increases the level of LDGs post-M. tuberculosis infection, indicating that the NET release can induce the conversion of NDGs to LDGs. Furthermore, TPEN, a membrane-permeable Zn2+-selective chelator and inhibitor of NET formation (Hasan et al., 2013), blocks the conversion of NDGs to LDGs induced by M. tuberculosis infection. These results proposed that the release of NETs is one of the mechanisms involved in the generation of LDGs in M. tuberculosis infection. Considering that NETs’ release is associated with activation and tissue damage of tuberculosis (Schechter et al., 2017; de Melo et al., 2019), this study suggests that the generation of LDGs may also be associated with the activation of tuberculosis.
The process of NET formation is dependent on the generation of ROS by NADPH oxidase (Kohchi et al., 2009), and the stimulation with both PMA and M. tuberculosis triggers the activation of NADPH oxidase (Fuchs et al., 2007). Our previous study also found that LDGs from patients with tuberculosis produce significantly high levels of ROS than autologous NDGs (Deng et al., 2016). Therefore, we assessed whether ROS are involved in the generation of LDGs. The results showed that M. tuberculosis triggered the production of ROS and the generation of LDGs. Furthermore, H2O2 also promoted the generation of LDGs. The pretreatment with NAC, an antioxidant which scavenges the hydroxyl and superoxide radicals (Ahmed et al., 2011; Dean et al., 2011), significantly decreases the levels of intracellular ROS, inhibits the production of NETs, and decreases the generation of LDGs post-M. tuberculosis infection. Also, the NADPH oxidase inhibitor DPI blocks the release of NETs and prevents the conversion of NDGs to LDGs after M. tuberculosis infection. The current results revealed that the levels of intracellular ROS play a major role in regulating the production of NETs and generation of LDGs in M. tuberculosis infection.
Next, we evaluated whether ROS is required for the generation of LDGs in M. tuberculosis infection. Since NET formation is ROS-dependent, the release of NETs while decreasing or maintaining the levels of ROS cannot be triggered. Thus, we adopted an alternative strategy to investigate the correlation among ROS levels, NET formation, and LDG generation. Cl-amidine, a highly specific peptidylarginine deiminase 4 (PAD4) inhibitor (Knight et al., 2013) was used in this study to inhibit the formation of NETs without altering the levels of intracellular ROS. PAD4 catalyzes the citrullination of proteins, including histone, which is an obligatory step preceding chromatin decondensation and release, thereby exerting a crucial role in NET formation (Leshner et al., 2012; Rohrbach et al., 2012). Previous studies and current results showed that Cl-amidine significantly inhibits the formation of NETs in human neutrophils in vitro and mouse neutrophils in vivo (Leshner et al., 2012; Rohrbach et al., 2012), but does not alter the intracellular ROS levels in neutrophils (Knight et al., 2014). Although the ROS levels were robustly increased after M. tuberculosis infection, however, Cl-amidine could prevent the conversion of NDGs to LDGs after M. tuberculosis infection, which suggested that ROS-induced generation of LDGs is mediated by regulating the formation of NETs in neutrophils.
In summary, the present and our previous studies demonstrate that the infection of M. tuberculosis can induce the conversion of NDGs to LDGs, and the generation of M. tuberculosis infection-related LDGs is related to the intracellular ROS level and NET release in neutrophils. To the best of our knowledge, the molecular mechanisms underlying the generation of LDGs has not yet been reported. Thus, the present study, for the first time, reveals that ROS contributes to the generation of LDGs by regulating the formation of NETs in M. tuberculosis infection.
Ethics Statement
This study was approved by the Ethical Review Committees of the First Affiliated Hospital of Nanchang University and was carried out in compliance with the Helsinki Declaration. Written informed consent was obtained from all the subjects enrolled in this study.
Author Contributions
JL conceived the project and wrote the manuscript. JL, RS, and YP designed the experiments, collected the study subjects, performed the experiments, and analyzed the data. RS, YP, ZD, YD, JY, YG, ZH, QL, and HJ performed the experiments.
Funding
This work was supported by grants from the National Natural Science Foundation of China (No. 81560344 and No. 81760002), the Project for Academic and Technical Leaders of Major Disciplines of Jiangxi Province (No. 20172BCB22026), and the Key Projects of Jiangxi Natural Science Foundation (No. 20171ACB20032).
Conflict of Interest Statement
The authors declare that the research was conducted in the absence of any commercial or financial relationships that could be construed as a potential conflict of interest.
Acknowledgments
We acknowledge Prof. Guo-liang Xiong (Shanghai Jiao Tong University School of Medicine) for the help of collecting TB patients.
References
Ahmed, T., Pathak, R., Mustafa, M. D., Kar, R., Tripathi, A. K., Ahmed, R. S., et al. (2011). Ameliorating effect of N-acetylcysteine and curcumin on pesticide-induced oxidative DNA damage in human peripheral blood mononuclear cells. Environ. Monit. Assess. 179, 293–299. doi: 10.1007/s10661-010-1736-5
Brinkmann, V., Reichard, U., Goosmann, C., Fauler, B., Uhlemann, Y., Weiss, D. S., et al. (2004). Neutrophil extracellular traps kill bacteria. Science 303, 1532–1535. doi: 10.1126/science.1092385
Carmona-Rivera, C., and Kaplan, M. J. (2013). Low-density granulocytes: a distinct class of neutrophils in systemic autoimmunity. Semin. Immunopathol. 35, 455–463. doi: 10.1007/s00281-013-0375-7
Chinese Medical Association (2005). Guideline of clinical diagnosis and treatment: tuberculosis section. 1st Edn. (Beijing, China: People’s Medical Publishing House), 9–32.
Cloke, T., Munder, M., Taylor, G., Müller, I., and Kropf, P. (2012). Characterization of a novel population of low-density granulocytes associated with disease severity in HIV-1 infection. PLoS One 7:e48939. doi: 10.1371/journal.pone.0048939
de Melo, M. G. M., Mesquita, E. D. D., Oliveira, M. M., da Silva-Monteiro, C., Silveira, A. K. A., Malaquias, T. S., et al. (2019). Imbalance of NET and alpha-1-antitrypsin in tuberculosis patients is related with hyper inflammation and severe lung tissue damage. Front. Immunol. 9:3147. doi: 10.3389/fimmu.2018.03147
Dean, O., Giorlando, F., and Berk, M. (2011). N-acetylcysteine in psychiatry: current therapeutic evidence and potential mechanisms of action. J. Psychiatry Neurosci. 36, 78–86. doi: 10.1503/jpn.100057
Deng, Y., Ye, J., Luo, Q., Huang, Z., Peng, Y., Xiong, G., et al. (2016). Low-density granulocytes are elevated in mycobacterial infection and associated with the severity of tuberculosis. PLoS One 11:e0153567. doi: 10.1371/journal.pone.0153567
Denny, M. F., Yalavarthi, S., Zhao, W., Thacker, S. G., Anderson, M., Sandy, A. R., et al. (2010). A distinct subset of proinflammatory neutrophils isolated from patients with systemic lupus erythematosus induces vascular damage and synthesizes type I IFNs. J. Immunol. 184, 3284–3297. doi: 10.4049/jimmunol.0902199
Eum, S. Y., Kong, J. H., Hong, M. S., Lee, Y. J., Kim, J. H., Hwang, S. H., et al. (2010). Neutrophils are the predominant infected phagocytic cells in the airways of patients with active pulmonary TB. Chest 137, 122–128. doi: 10.1378/chest.09-0903
Fu, J., Tobin, M. C., and Thomas, L. L. (2014). Neutrophil-like low-density granulocytes are elevated in patients with moderate to severe persistent asthma. Ann. Allergy Asthma Immunol. 113, 635–640.e2. doi: 10.1016/j.anai.2014.08.024
Fuchs, T. A., Abed, U., Goosmann, C., Hurwitz, R., Schulze, I., Wahn, V., et al. (2007). Novel cell death program leads to neutrophil extracellular traps. J. Cell Biol. 176, 231–241. doi: 10.1083/jcb.200606027
Garcia-Romo, G. S., Caielli, S., Vega, B., Connolly, J., Allantaz, F., Xu, Z., et al. (2011). Netting neutrophils are major inducers of type I IFN production in pediatric systemic lupus erythematosus. Sci. Transl. Med. 3:73ra20. doi: 10.1126/scitranslmed.3001201
Grayson, P. C., Carmona-Rivera, C., Xu, L., Lim, N., Gao, Z., Asare, A. L., et al. (2015). Neutrophil-related gene expression and low-density granulocytes associated with disease activity and response to treatment in antineutrophil cytoplasmic antibody-associated vasculitis. Arthritis Rheumatol. 67, 1922–1932. doi: 10.1002/art.39153
Hacbarth, E., and Kajdacsy-Balla, A. (1986). Low density neutrophils in patients with systemic lupus erythematosus, rheumatoid arthritis, and acute rheumatic fever. Arthritis Rheum. 29, 1334–1342. doi: 10.1002/art.1780291105
Hasan, R., Rink, L., and Haase, H. (2013). Zinc signals in neutrophil granulocytes are required for the formation of neutrophil extracellular traps. Innate Immun. 19, 253–264. doi: 10.1177/1753425912458815
Kanamaru, R., Ohzawa, H., Miyato, H., Matsumoto, S., Haruta, H., Kurashina, K., et al. (2018). Low density neutrophils (LDN) in postoperative abdominal cavity assist the peritoneal recurrence through the production of neutrophil extracellular traps (NETs). Sci. Rep. 8:632. doi: 10.1038/s41598-017-19091-2
Kaplan, M. J., and Radic, M. (2012). Neutrophil extracellular traps: double-edged swords of innate immunity. J. Immunol. 189, 2689–2695. doi: 10.4049/jimmunol.1201719
Knight, J. S., Luo, W., O’Dell, A. A., Yalavarthi, S., Zhao, W., Subramanian, V., et al. (2014). Peptidylarginine deiminase inhibition reduces vascular damage and modulates innate immune responses in murine models of atherosclerosis. Circ. Res. 114, 947–956. doi: 10.1161/CIRCRESAHA.114.303312
Knight, J. S., Zhao, W., Luo, W., Subramanian, V., O’Dell, A. A., Yalavarthi, S., et al. (2013). Peptidylarginine deiminase inhibition is immunomodulatory and vasculoprotective in murine lupus. J. Clin. Invest. 123, 2981–2993. doi: 10.1172/JCI67390
Kohchi, C., Inagawa, H., Nishizawa, T., and Soma, G. (2009). ROS and innate immunity. Anticancer Res. 29, 817–821. doi: 10.1111/j.1469-8749.2009.03380.x
Leshner, M., Wang, S., Lewis, C., Zheng, H., Chen, X. A., Santy, L., et al. (2012). PAD4 mediated histone hypercitrullination induces heterochromatin decondensation and chromatin unfolding to form neutrophil extracellular trap-like structures. Front. Immunol. 3:307. doi: 10.3389/fimmu.2012.00307
Liu, Y., Hu, Y., Gu, F., Liang, J., Zeng, Y., Hong, X., et al. (2017). Phenotypic and clinical characterization of low density neutrophils in patients with advanced lung adenocarcinoma. Oncotarget 8, 90969–90978. doi: 10.18632/oncotarget.18771
Moses, K., and Brandau, S. (2016). Human neutrophils: their role in cancer and relation to myeloid-derived suppressor cells. Semin. Immunol. 28, 187–196. doi: 10.1016/j.smim.2016.03.018
Mourik, B. C., Lubberts, E., Steenwinkel, J. E. M., Ottenhoff, T. H. M., and Leenen, P. J. M. (2017). Interactions between type 1 interferons and the Th17 response in tuberculosis: lessons learned from autoimmune diseases. Front. Immunol. 8:294. doi: 10.3389/fimmu.2017.00294
Nauseef, W. M. (2007). How human neutrophils kill and degrade microbes: an integrated view. Immunol. Rev. 219, 88–102. doi: 10.1111/j.1600-065X.2007.00550.x
Papayannopoulos, V., Metzler, K. D., Hakkim, A., and Zychlinsky, A. (2010). Neutrophil elastase and myeloperoxidase regulate the formation of neutrophil extracellular traps. J. Cell Biol. 191, 677–691. doi: 10.1083/jcb.201006052
Ramos-Kichik, V., Mondragón-Flores, R., Mondragón-Castelán, M., Gonzalez-Pozos, S., Muñiz-Hernandez, S., Rojas-Espinosa, O., et al. (2009). Neutrophil extracellular traps are induced by Mycobacterium tuberculosis. Tuberculosis 89, 29–37. doi: 10.1016/j.tube.2008.09.009
Rocha, B. C., Marques, P. E., Leoratti, F. M. S., Junqueira, C., Pereira, D. B., Antonelli, L. R. D. V., et al. (2015). Type I interferon transcriptional signature in neutrophils and low-density granulocytes are associated with tissue damage in malaria. Cell Rep. 13, 2829–2841. doi: 10.1016/j.celrep.2015.11.055
Rohrbach, A. S., Slade, D. J., Thompson, P. R., and Mowen, K. A. (2012). Activation of PAD4 in NET formation. Front. Immunol. 3:360. doi: 10.3389/fimmu.2012.00360
Sagiv, J. Y., Michaeli, J., Assi, S., Mishalian, I., Kisos, H., Levy, L., et al. (2015). Phenotypic diversity and plasticity in circulating neutrophil subpopulations in cancer. Cell Rep. 10, 562–573. doi: 10.1016/j.celrep.2014.12.039
Schechter, M. C., Buac, K., Adekambi, T., Cagle, S., Celli, J., Ray, S. M., et al. (2017). Neutrophil extracellular trap (NET) levels in human plasma are associated with active TB. PLoS One 12:e0182587. doi: 10.1371/journal.pone.0182587
Scriba, T. J., Coussens, A. K., and Fletcher, H. A. (2017). Human immunology of tuberculosis. Microbiol. Spectr. 5. doi: 10.1128/microbiolspec.TBTB2-0016-2016
Singh, N., Traisak, P., Martin, K. A., Kaplan, M. J., Cohen, P. L., and Denny, M. F. (2014). Genomic alterations in abnormal neutrophils isolated from adult patients with systemic lupus erythematosus. Arthritis Res. Ther. 16:R165. doi: 10.1186/ar4681
World Health Organization (2017). Global tuberculosis report 2017. (Geneva: World Health Organization).
Yang, H., Biermann, M. H., Brauner, J. M., Liu, Y., Zhao, Y., and Herrmann, M. (2016). New insights into neutrophil extracellular traps: mechanisms of formation and role in inflammation. Front. Immunol. 7:302. doi: 10.3389/fimmu.2016.00302
Zhang, S., Shen, H., Shu, X., Peng, Q., and Wang, G. (2017). Abnormally increased low-density granulocytes in peripheral blood mononuclear cells are associated with interstitial lung disease in dermatomyositis. Mod. Rheumatol. 27, 122–129. doi: 10.1080/14397595.2016.1179861
Keywords:Mycobacterium tuberculosis, low-density granulocytes, mechanism, neutrophil extracellular traps, reactive oxygen species
Citation: Su R, Peng Y-p, Deng Z, Deng Y-t, Ye J-q, Guo Y, Huang Z-k, Luo Q, Jiang H and Li J-m (2019) Mycobacterium tuberculosis Infection Induces Low-Density Granulocyte Generation by Promoting Neutrophil Extracellular Trap Formation via ROS Pathway. Front. Microbiol. 10:1468. doi: 10.3389/fmicb.2019.01468
Edited by:
Pere-Joan Cardona, Germans Trias i Pujol Health Science Research Institute (IGTP), SpainReviewed by:
Veronica Schmitz, Oswaldo Cruz Foundation (Fiocruz), BrazilClara I. Espitia, National Autonomous University of Mexico, Mexico
Copyright © 2019 Su, Peng, Deng, Deng, Ye, Guo, Huang, Luo, Jiang and Li. This is an open-access article distributed under the terms of the Creative Commons Attribution License (CC BY). The use, distribution or reproduction in other forums is permitted, provided the original author(s) and the copyright owner(s) are credited and that the original publication in this journal is cited, in accordance with accepted academic practice. No use, distribution or reproduction is permitted which does not comply with these terms.
*Correspondence: Jun-ming Li, ndyfy2140@ncu.edu.cn
†These authors have contributed equally to this work
‡Present address: Jun-ming Li, Department of Clinical Laboratory, The First Affiliated Hospital of Nanchang University, Nanchang, China