- 1Department of Pharmacy, Shandong Provincial Qianfoshan Hospital, Shandong University, Jinan, China
- 2School of Pharmaceutical Sciences, Shandong University, Jinan, China
- 3Department of Pharmacy, Shandong Provincial Qianfoshan Hospital, The First Hospital Affiliated with Shandong First University, Jinan, China
- 4Department of Pharmacy, Qilu Children’s Hospital of Shandong University, Jinan, China
Candida albicans is a common opportunistic fungal pathogen that may cause nosocomial fungal infections. The resistance of Candida albicans to traditional antifungal drugs has been increasing rapidly in recent years, and it brings a great challenge in clinical treatment. N-butylphthalide is originally extracted from the seed of Apium graveolens and is currently used for the treatment of ischemic stroke in the clinic. This study demonstrated that n-butylphthalide exhibited antifungal activity against Candida albicans with minimum inhibitory concentrations of 128 μg/ml; moreover, n-butylphthalide combined with fluconazole showed synergistic antifungal effects against resistant Candida albicans, resulting in a decrease in the minimum inhibitory concentrations of fluconazole from >512 to 0.25–1 μg/ml. Time-killing curves verified the antifungal activity in dynamic. Besides, n-butylphthalide exhibited anti-biofilm activity against Candida albicans, biofilms preformed <12 h with sessile minimum inhibitory concentrations of 128–256 μg/ml and synergism was observed when n-butylphthalide combined with fluconazole against resistant Candida albicans biofilms preformed <12 h, resulting in a decrease in the sessile minimum inhibitory concentrations of fluconazole from >1,024 to 0.5–8 μg/ml. Furthermore, in vitro antifungal effects of n-butylphthalide were confirmed in vivo. N-butylphthalide prolonged survival rate of larvae infected by Candida albicans, reduced the fungal burden in larvae and caused less damage to larval tissues. Notably, n-butylphthalide inhibited hyphal growth and induced intracellular reactive oxygen species accumulation and a loss in mitochondrial membrane potential, which was a potential antifungal mechanism. Besides, the synergistic effects between n-butylphthalide and fluconazole potentially relied on the mechanism that n-butylphthalide significantly promoted drug uptake, and suppressed drug efflux via down-regulating the drug transporter encoding genes CDR1 and CDR2. These findings demonstrated the antifungal effects and mechanisms of n-butylphthalide against Candida albicans for the first time, which might provide broad prospects for the identification of new potential antifungal targets.
Introduction
Due to the extensive application of broad-spectrum antibiotics, immunosuppressive agents, and medical implant devices, the incidence of fungal infections has increased rapidly in the last few decades (Suleyman and Alangaden, 2016). The leading Candida species, Candida albicans (C. albicans), is the most common fungal pathogen that may cause epidermal and potentially life-threatening invasive infections, especially in immunocompromised patients (Dimopoulos et al., 2007). Fluconazole (FLC), a kind of azoles, is the most frequently used antifungal drug for prevention and treatment of C. albicans infections due to the high efficacy and low toxicity. However, drug resistance to antifungals, especially to FLC among C. albicans species, increased sharply along with long-term use of it (Whaley et al., 2016). Furthermore, biofilms adhered on the abiotic and biotic surfaces act as the natural barrier to the dispersion of antifungal drugs and are inherently resistant to most antifungal drugs (Bonhomme and d’Enfert, 2013; Desai et al., 2014). Thus, there is an urgent need to develop therapeutic strategies to combat drug resistance of C. albicans.
Natural products, especially extracted from traditional Chinese herbal medicine, provide a huge treasure pool for drug discovery by serving as compounds with metabolic activity in their natural form or synthetic modification (Mishra and Tiwari, 2011). It is worth noting that phytocompounds exhibit prominent potential as antifungal agents or as synergistic agents with FLC, particularly against Candida spp. (Lu et al., 2017). For example, Shao et al. confirmed that sodium houttuyfonate revealed relatively strong antifungal potential against C. albicans (Huang et al., 2015; Shao et al., 2017; Da et al., 2019). N-butylphthalide (NBP) (Figure 1A) is originally extracted from the seed of Apium graveolens, and it is a new drug that has been independently researched and developed in China (Zhao et al., 2014). NBP has a wide range of pharmacological effects on cerebrovascular diseases: resisting cerebral ischemia, improving brain cell energy metabolism, and inhibiting thrombosis. Currently, NBP is widely used in the clinic for the treatment of ischemic stroke because of its low toxicity and good safety (Zhao et al., 2014; Abdoulaye and Guo, 2016). Furthermore, it has been confirmed that compound1 (Figure 1B) and compound2 (Figure 1C) are the structural analogues of NBP and are also extracted from Apium graveolens seeds, have antifungal activity against C. albicas (Momin et al., 2000; Momin and Nair, 2001). However, at present, there are no reports investigating the antifungal activity of NBP, alone and combined with FLC, against C. albicans.
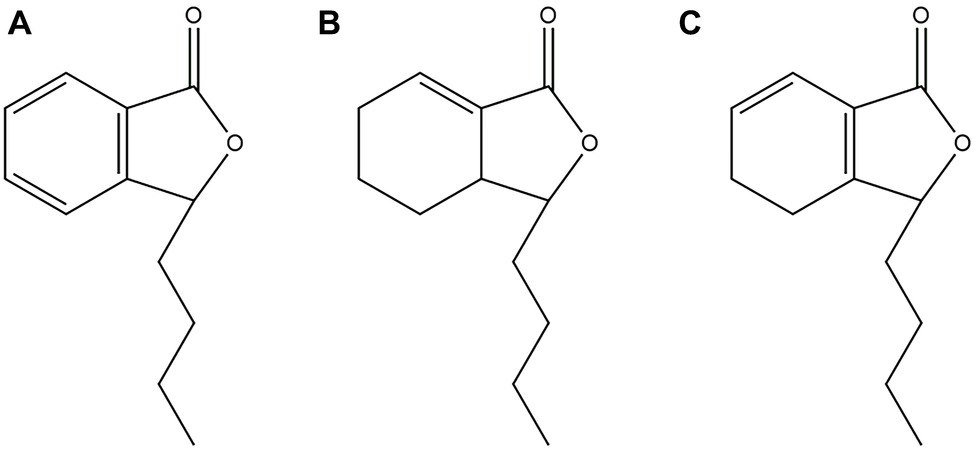
Figure 1. (A) Chemical structure of NBP. (B) Chemical structure of compound1. (C) Chemical structure of compound2.
In this study, the in vitro antifungal effects of NBP alone and in combinations with FLC against planktonic C. albicans and biofilms in different stages (4, 8, 12, and 24 h) were evaluated. The dynamical antifungal effects of NBP were demonstrated by time-killing curves. In addition, Galleria mellonella (G. mellonella)-C. albicans infection model was established and the survival rate, fungal burden, and histopathology were used to evaluate the effects of NBP in vivo. For the exploration of underlying mechanism, we investigated the effects of NBP on hyphal growth, the levels of intracellular reactive oxygen species (ROS) and mitochondrial membrane potential (Δψm). Furthermore, to explore the potential synergistic mechanism of NBP combined with FLC, we conducted rhodamine 6G assays to detect the effects of NBP on drug uptake and efflux of resistant strains. We also carried out real-time quantitative PCR assays (RT-PCR) to determine the gene expression levels of CDR1, CDR2, and MDR1, which encode efflux pump proteins.
Materials And Methods
Strains, Culture, and Agents
Six C. albicans strains were used in this study, including two FLC-susceptible strains (CA4 and 8) and four FLC-resistant strains (CA10, 16, 103, and 632). CA4, 8, 10, and 16 were collected from the clinical laboratory at Qianfoshan Hospital Affiliated to Shandong University (Jinan, China), and CA103 and 632 were kindly provided by Professor Changzhong Wang (School of integrated traditional and western medicine, Anhui University of traditional Chinese medicine, Hefei, China). Their susceptibilities were determined according to Clinical and Laboratory Standards Institute (CLSI) document M27-A3 (Institute CaLS, 2008a,b). C. albicans ATCC 10231, kindly provided by the Institute of Pharmacology, School of Pharmacy, Shandong University (Jinan, China), was used as the quality control strain. CA10 was used as a representative strain for time-killing test, in vivo experiment and mechanism exploration. Strains were refreshed from the frozen stocks at −80°C and inoculated at least twice onto sabouraud solid medium for 18 h at 35°C before all experiments. RPMI 1640 (pH 7.0) was used as the liquid medium for diluting drugs and strains.
All drugs (NBP, penicillin sodium and FLC) were purchased from Dalian Meilun Biotech Co. Ltd., China. Stock solution of NBP was dissolved in absolute ethyl alcohol with 0.5% tween80 at a final concentration of 12,800 μg/ml. Stock solutions of penicillin sodium and FLC were prepared in sterile distilled water to a final concentration of 2560 μg/ml. All stock solutions were stored at −20°C until use.
Determination of Minimum Inhibitory Concentrations of Planktonic Cells
The minimum inhibitory concentrations (MICs) of NBP alone and in combination with FLC against C. albicans isolates were determined with a broth microdilution method as described by the CLSI guidelines (Institute CaLS, 2008a,b). The tests were performed in 96-well flat-bottomed microtiter plates. The final concentration of fungal suspension in RPMI 1640 medium was 103CFU/ml, the final concentration of NBP ranged from 4 to 256 μg/ml and the final concentration of FLC ranged from 0.125 to 64 μg/ml. All of the wells were filled with RPMI 1640 to a final volume of 200 μl. A drug-free well served as a growth control, and wells containing RPMI 1640 medium only were set as negative controls. Plates were incubated at 35°C for 24 h. The growth inhibition was determined both by visual reading and by measuring the optical density at 492 nm using a microplate reader. MIC80 was defined as the lowest concentration of drug, alone and in combination that inhibited the growth of yeast by 80% compared with the control group (Lewis et al., 2002; Li et al., 2011; Khan and Ahmad, 2012). The in vitro interaction of the drug combination was interpreted in terms of the fractional inhibitory concentration index (FICI) (Odds, 2003). The FICI model was expressed as follows: FICI = FICFLC + FICNBP = (MIC80 of FLC in combination/MIC80 of FLC alone) + (MIC80 of NBP in combination/MIC80 of NBP alone). The interpretation of the FICI was defined as FICI of ≤0.5 for synergy, FICI >4.0 for antagonism and 0.5 < FICI≤4.0 for no interaction.
Determination of Sessile Minimum Inhibitory Concentrations of C. albicans Biofilms
Sessile MICs (SMICs) of NBP, alone and combined with FLC against C. albicans (CA4, 10 and 16), were evaluated as described by Ramage and Lopez-Ribot (2005) with moderate modifications. In brief, biofilms were formed by adding 200 μl cell suspension (103CFU/ml) into 96-well flat-bottomed microtiter plates over four time intervals (4, 8, 12, and 24 h) at 35°C. At each time point, each well was washed with 200 μl PBS three times to remove the planktonic and nonadherent cells. Subsequently, drugs of different concentrations were added and the plates were incubated for another 24 h at 35°C. The final concentration of FLC and NBP in wells ranged from 0.125 to 64 μg/ml and from 4 to 256 μg/ml, respectively. A metabolic assay based on the reduction of 2,3–bis (2–methoxy–4–nitro–5–sulfophenyl)–2H–tetrazolium–5–carboxanilide (XTT) was carried out to determine the sMICs. SMIC80 referred to the lowest concentrations, where there was an 80% reduction in the XTT-colorimetric readings compared with the drug-free control (Prazynska and Gospodarek, 2014; Zhong et al., 2017). Colorimetric absorbance was measured at 492 nm in a microtiter plate reader. The FICI model was used to illustrate the interaction between NBP and FLC against C. albicans biofilms as described above.
Time-Killing Curve Assay
Groups containing NBP (64, 128, and 256 μg/ml, respectively), FLC (1 μg/ml), NBP/FLC (128 and 1 μg/ml, respectively) and 105CFU/ml of C. albicans suspension were then incubated at 35°C with constant shaking (200 rpm). The group with no drug was served as a control growth group. At prearranged time points (0, 6, 12, 24, and 48 h) after incubation, the amount of living cells was then measured by colony counting methods (Li et al., 2008, 2015; Shrestha et al., 2015). For judgment of the interaction between NBP and FLC, synergism was defined as a ≥ 2lg10 decrease in CFU/ml and indifference as a <2lg10decrease in CFU/ml compared to the most active drug, and antagonism as a ≥ 2lg10 increase in CFU/ml compared to the least active drug (Li et al., 2014b).
Determination of in vivo Antifungal Effects by G. mellonella Infection Model
Three in vivo experiments, survival assay, fungal burden determination and histological study, were carried out. The initial steps of each experiment were identical. G. mellonella larvae during the last instar of the larval development were selected to be absent of dark spots and similar in size (approximately 0.25 ± 0.02 g). Each group contained 20 randomly chosen larvae and they were placed in perish dishes at 35°C overnight before experiments. About 10 μl of C. albicans suspension (108CFU/ml) was inoculated directly to the last left pro-leg. Before injection, the area was swabbed by ethanol for disinfection. After 2 h injection, where four groups of the larvae were injected via the last right pro-leg with 10 μl of sterile PBS, NBP (40 μg/ml), FLC (160 μg/ml), and NBP + FLC (40 +160 μg/ml), all groups of larvae were incubated at 35°C in the dark (Frenkel et al., 2016; Lukowska-Chojnacka et al., 2016; Li et al., 2017).
For survival assay, four groups of larvae were pretreated as described above. Survival was recorded every day for 4 days. Larva was considered dead if they gave no response to slight touch with forceps.
For fungal burden determination, another four groups of larvae were pretreated as described above. Three larvae were randomly taken from per group daily over 4 days and then homogenized in 3 ml sterile PBS/penicillin sodium using a homogenizer. Subsequently, fungal burden of each group was determined by colony counting methods (Krezdorn et al., 2014).
For histological study, four groups of larvae were pretreated as described above and a group of larvae was treated as the blank group without injectant. After 2 days of incubation, two larvae were taken randomly from each group and then were immersed in 4% paraformaldehyde fixative overnight. Subsequently, larvae were fixed in tissue OCT-freeze medium and cut into 14 μm tissue sections using a freezing microtome (Gu et al., 2016). The tissue sections then were stained with Periodic acid Schiff (PAS) and were observed under a microscope.
Hyphal Growth Assay
Hyphal growth assay was performed in hypha-inducing media, RPMI1640 and spider medium in the well-plate (Li et al., 2014a; Haque et al., 2016). C. albicans suspension (2 × 105 CFU/ml) was treated with different concentrations of NBP (32, 64, and 128 μg/ml) at 35°C for 4 h. The group treated without NBP was served as a control group. The cell suspension was then aspirated and each well was washed with 200 μl PBS to remove the nonadherent cells. The samples were examined under bright field using 20X objective lens by TH4-200 fluorescence microscope (Olympus, Japan) and photographed.
Measurement of Reactive Oxygen Species Levels Assay
The levels of ROS produced by C. albicans treated with different concentrations of NBP were measured using the DCFH-DA (MedChem Express, USA). C. albicans suspension (5 × 105 CFU/ml) was treated with NBP (32, 64, and 128 μg/ml) for 4 h and the group treated without NBP was set as a control group. The cells were then washed with PBS, incubated with 40 μM DCFH-DA in the dark for 30 min and detected by a BD FACS Aria II flow cytometer (Becton Dickinson, USA) with an excitation wavelength at 488 nm and emission wavelength at 530 nm.
Analysis of Δψm
Rhodamine123 (Rh123, Sigma, USA) was used to examine the effect of NBP on the C. albicans Δψm in this study (Zheng et al., 2018). The yeast cells were pretreated with different concentrations of NBP as described in “Measurement of ROS levels assay.” The cells were stained then with 15 μM Rh123 for 30 min in the dark and detected by the flow cytometer with an excitation wavelength at 488 nm and emission wavelength at 530 nm.
Rh6G Uptake and Efflux Assay
The drug uptake and efflux of C. albicans were measured by Rh6G assay due to both Rh6G and FLC are substrates of drug transporters (Pina-Vaz et al., 2005). C. albicans suspension (107CFU/ml) was first de-energized for 1 h in PBS (without glucose), collected, and resuspended again to obtain the concentration as above.
For Rh6G uptake assay, final concentrations of 10 μM Rh6G and 32 μg/ml NBP were added to the de-energized cells simultaneously, and cells without NBP were served as the control group. The mean fluorescence intensity (MFI) of intracellular Rh6G was measured every 10 min for a total of 60 min by the flow cytometer with excitation wavelength at 488 nm and emission wavelength at 530 nm.
For Rh6G efflux assay, Rh6G was added to the de-energized cells suspension at a final concentration of 10 mM. The samples were incubated in a shaking incubator at 35°C for 1 h and later transferred to an ice-water bath for 30 min to stop the uptake of Rh6G. Then cells were collected, washed and resuspended in glucose/PBS (5%). At the same time, NBP at a final concentration of 32 μg/ml was added and Rh6G alone served as the control group. At special time intervals (0.40, 80, 120, 160, and 200 min), the MFI of intracellular Rh6G was measured using a flow cytometer with excitation wavelength at 488 nm and emission wavelength at 530 nm (Peralta et al., 2012).
Real-Time Quantitative Polymerase Chain Reaction
C. albicans suspension (5 × 105 CFU/ml) was treated with 32 μg/ml NBP diluted with sabouraud liquid medium for 16–18 h and the group treated without NBP was set as a control group. C. albicans cells were collected, washed, and total RNA was isolated by the E.Z.N.A Yeast RNA kit (e9080, OMEGA). Diluted RNA was then treated with PrimeScript RT reagent kit (RR047A, TaKaRa Biotechnology) to obtain cDNA through a reverse transcription reaction. The thermal cycling condition was 95°C for 30 s as an initial denaturation step, followed by 40 cycles of 95°C for 10 s and 60°C for 34 s and ended by the melting conditions of 95°C for 15 s, 60°C for 1 min and 95°C for 15 s. The expression of each gene was normalized to that of the ACT1 gene. Drug transporter encoding genes, CDR1, CDR2, and MDR1 were determined by the RT-PCR assay as mentioned above. Sequences of the primers are listed in Table 1. The result was calculated using the 2−(ΔΔCt) method (Pfaffl, 2001).
Statistics
All experiments were performed at least three times independently. Graphs and statistical analyses were performed with GraphPad Prism 7 (GraphPad, La Jolla, CA) and IBM SPSS Statistics 22 (SPSS, Chicago, IL). All the experimental data measuring by the flow cytometer were analyzed by BD FACSDiva v6.1.3 and FlowJo v7.10.1 software. Fungal burden, rhodamine 6G uptake and efflux and relative expression levels of genes was analyzed using an unpaired t-test. The levels of ROS and Δψm were analyzed using one-way analysis of variance (ANOVA). p < 0.05 was considered significant.
Results
Minimum Inhibitory Concentrations of N-Butylphthalide Alone and in Combination With Fluconazole Against C. albicans
The MICs of NBP and FLC, alone and in combination against the six tested C. albicans isolates, were listed in Table 2. NBP exhibited antifungal activity against C. albicans with MICs of 128 μg/ml, and also exhibited synergistic effects combined with FLC against resistant C. albicans with FICIs of 0.25, resulting in a decrease in the MICs of NBP from 128 to 32 μg/ml and the MICs of FLC from >512 to 0.25–1 μg/ml. Besides, although no synergism was observed with FICIs of >0.5 when NBP combined with FLC against susceptible C. albicans, the MICs of NBP could decrease from 128 to 8–64 μg/ml and the MICs of FLC could decrease from 0.5–1 to 0.25–0.5 μg/ml.
Sessile Minimum Inhibitory Concentrations of N-Butylphthalide Alone and Combined With Fluconazole Against C. albicans Biofilms
The sMICs of NBP and FLC, alone and in combination against the biofilms formed by C. albicans isolates, CA4, 10, and 16, were listed in Table 3. NBP exhibited anti-biofilm activity against C. albicans biofilms pre-formed <12 h with sMICs of 128-256 μg/ml, and also exhibited synergistic effects combined with FLC against resistant C. albicans biofilms pre-formed <12 h with FICIs <0.5, resulting in a decrease in the sMICs of NBP from 128–256 to 32–64 μg/ml and the sMICs of FLC from >1,024 to 0.5–8 μg/ml. Furthermore, although no synergism was observed when NBP combined with FLC against susceptible C. albicans biofilms pre-formed <12 h with FICIs of >0.5, the sMICs of NBP could decrease from 128 to 64 μg/ml and the sMICs of FLC could decrease from >1,024 to 0.5–4 μg/ml. In addition, NBP alone or combined with FLC hardly inhibited mature biofilms pre-formed over more than 24 h, demonstrating the limitation of NBP; being that it only has antifungal activity against immature biofilms.
Time-Killing Curves
The results showed that, in the presence of 256 μg/ml NBP, a significant enhancement in the degree of antifungal activity was observed after 6 h, and there was a 1.91/2.05 log10 CFU ml−1 decrease at 24/48 h time point compared with the control group (Figure 2A). For the drug combination experiment, a fungal growth delay could be seen in the FLC alone group, however, it was more evident in the combination group and there was a 2.01/2.07 log10 CFU ml−1 decrease at 24/48 h time point compared with the FLC alone group (Figure 2B), indicating a synergistic antifungal effect in dynamic.
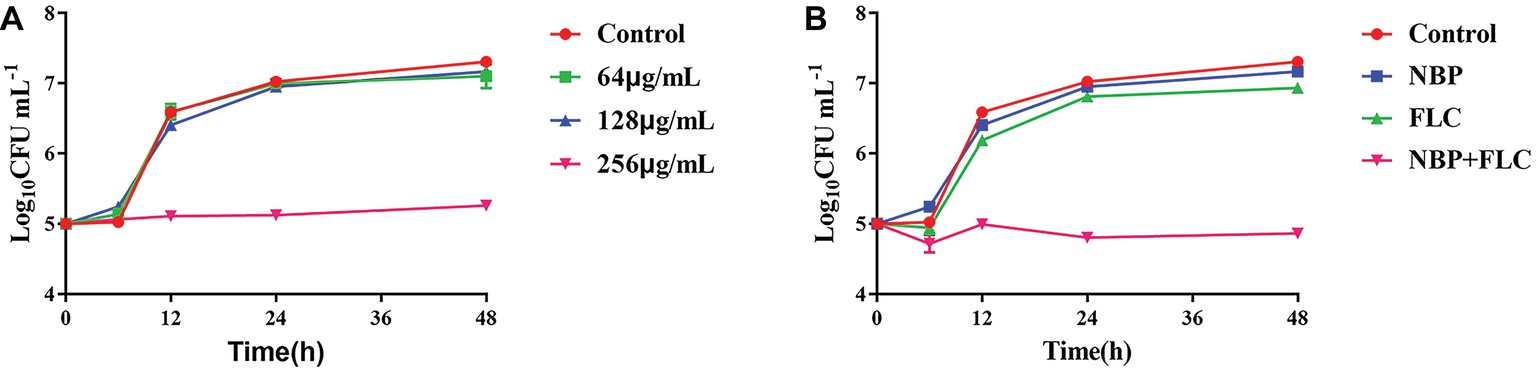
Figure 2. Time-killing curves of NBP alone (A) and in combination with FLC (B) against C. albicans. The initial yeast concentrations were adjusted to 105CFU/mL. (A) The concentrations of NBP were 64, 128, and 256 μg/ml, respectively. (B) The concentrations of NBP were 128 μg/ml when combined with FLC (1 μg/ml). Values represent the means ± standard deviation of three replicates.
Antifungal Effects of N-Butylphthalide Against C. albicans in vivo
The survival rate is the most important index to evaluate the effect of drugs in vivo with G. mellonella infection model. After 4 days of incubation, the control group showed a survival rate of 20%, while NBP group showed a survival rate of 35%, which was higher than the control group. The combinations of NBP and FLC significantly enhanced the survival rate to 70% compared with the NBP group (p < 0.05) (Figure 3A).
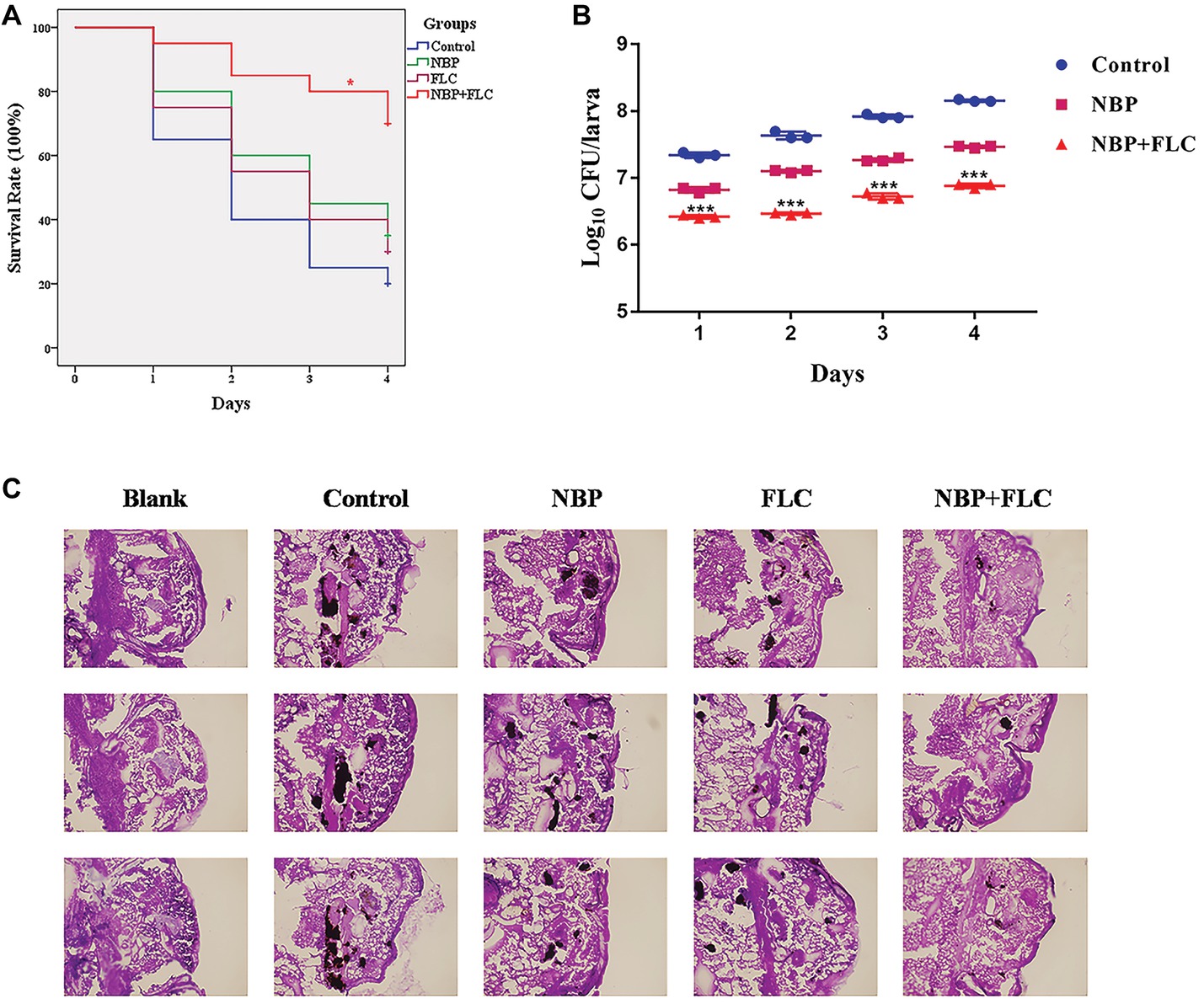
Figure 3. Effect of NBP in combination with FLC during G. mellonella infection with C. albicans. Larvae were injected with the inoculum of a cell concentration of 106CFU/larva and then treated with PBS (control), FLC (160 μg/ml), NBP (40 μg/ml), or FLC (160 μg/ml) combined with NBP (40 μg/ml). (A) Survival curve of G. mellonella infected with C. albicans. Values represent the means ± standard deviation of three replicates. *p < 0.05 compared with the NBP-treated group (Kaplan-Meier test). (B) Fungal burdern of G. mellonella infected with C. albicans. Data for the treatment of FLC monotherapy group were not showed because the data were similar with that of the NBP group. Values represent the means ± standard deviation of three replicates. ***p < 0.001 compared with the NBP-treated group (unpaired t-test). (C) Histopathology of G. mellonella infected with C. albicans. Larvae of the blank group were treated with neither C. albicans nor drugs. Melanized nodules containing yeast clusters and filaments could be observed except in blank group. The photographs were collected from three independent experiments.
The fungal burden analysis suggested that the larval fungal burden increased gradually over 4 days after injection in all groups. Treatment with NBP slightly decreased fungal burden than the control group and data of FLC monotherapy group were not shown in figure because the data were similar with those of the NBP monotherapy group. The combination of NBP and FLC significantly reduced fungal burden compared with the NBP group (p’s of all 4 days was <0.001), especially in the last 3 days (Figure 3B).
Histopathology studies (Figure 3C) revealed that in comparison with the blank group, the C. albicans cells mainly existed in the form of filamentous clusters in larval tissues after infection. In the drug monotherapy groups, although the amounts of melanized nodules were similar with the control group, the size of these melanized nodules of these two groups was smaller than those of the control group. However, only few small melanized nodules were discovered in the drug combination group.
Effects of N-Butylphthalide on C. albicans Hyphal Growth
C. albicans hyphae induced by RPMI 1640 medium and spider medium were shown in Figures 4, 5, respectively. As shown, C. albicans could form long and interlaced hyphae in both RPMI 1640 and spider medium, and NBP inhibited C. albicans hyphal growth in both media tested in a dose dependent manner. 32 μg/ml NBP could lead to form slightly shorter hyphae than the control group. 64 μg/ml NBP could induce to form loose and patchy hyphae, while when the concentration increased to 128 μg/ml, cells were mainly maintained as yeasts and few filament could be observed in the field of vision.
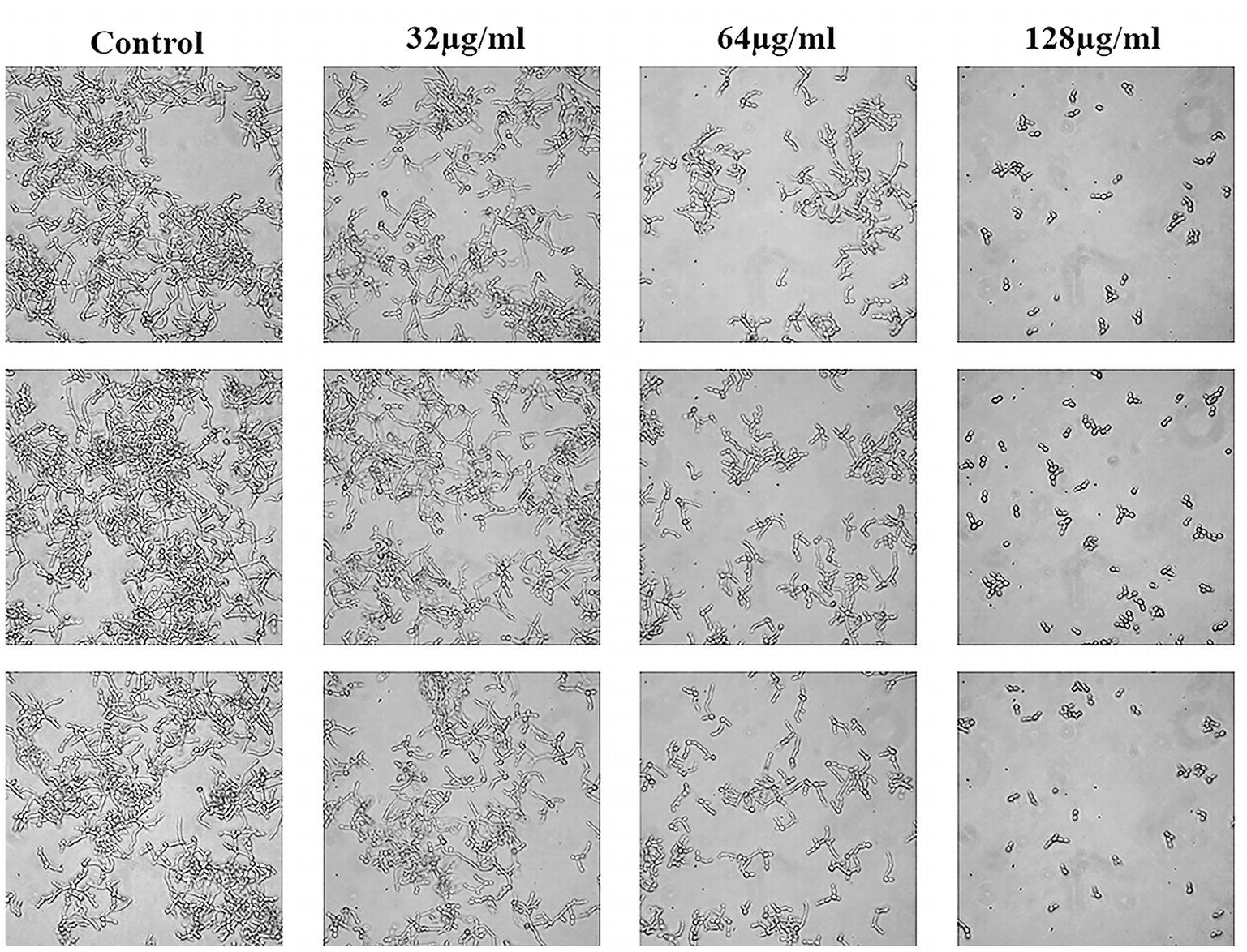
Figure 4. Effect of NBP on C. albicans hyphal growth induced by RPMI 1640 medium. NBP was diluted in hyphae-inducing media, RPMI 1640 medium, at a final concentration of 0, 32, 64, and 128 μg/ml, respectively. The cellular morphology was photographed after incubation at 37°C for 4 h. The photographs were collected from three independent experiments.
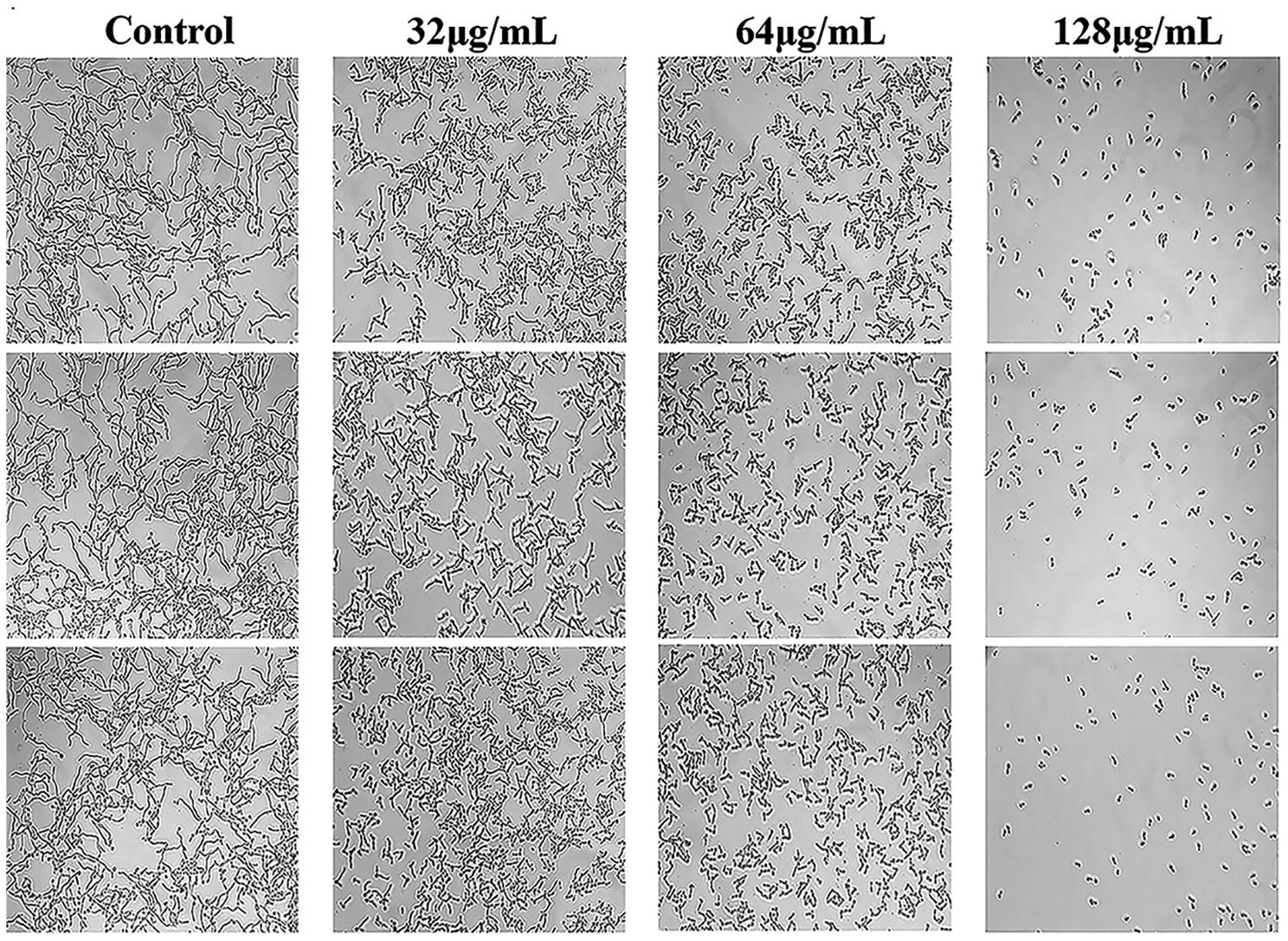
Figure 5. Effect of NBP on C. albicans hyphal growth induced by spider medium. NBP was diluted in hyphae-inducing media, spider medium, at a final concentration of 0, 32, 64, and 128 μg/ml, respectively. The cellular morphology was photographed after incubation at 37°C for 4 h. The photographs were collected from three independent experiments.
Effects of N-Butylphthalide on Reactive Oxygen Species Production
The generation of excessive ROS is considered as a potential fungicidal mechanism and we measured the levels of intracellular ROS after treated with NBP. The results showed that NBP significantly induced intracellular ROS accumulation of C. albicans in a dose-dependent manner (p < 0.001) (Figure 6). The levels of ROS resulted in approximately 165, 245, and 283% increases in MFI after treated with 32, 64, and 128 μg/ml NBP, respectively, compared with that of control group.
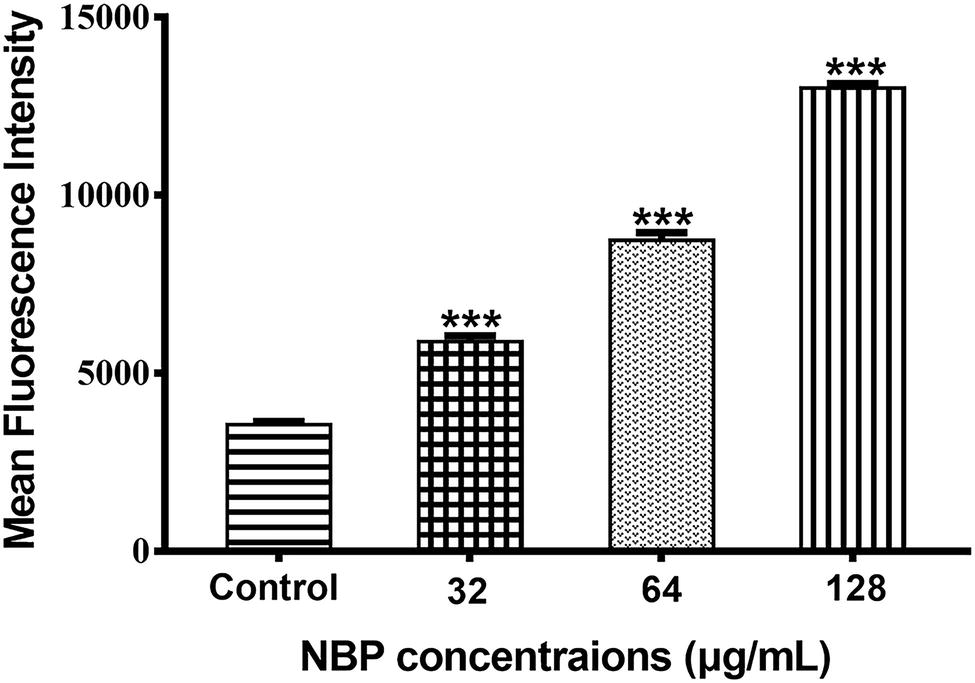
Figure 6. Effect of NBP on the levels of ROS in C. albicans. Yeast cells pretreated with diverse concentrations of NBP (0, 32, 64, and 128 μg/ml) for 4 h were incubated with 40 μM DCFH-DA and then analyzed by a flow cytometer. Values represent the means ± standard deviation of three replicates.***p < 0.001 compared with the control group (one-way ANOVA).
Effects of N-Butylphthalide on Δψm
To determine whether the NBP induced ROS accumulation was involved in the metabolic state of mitochondria, Δψm treated with NBP was measured. As shown in Figures 7A–D, NBP significantly reduced the number of C. albicans cells with an intact Δψm and increased the number of cells with low Δψm in a dose dependent manner. The percentage of C. albicans with reduced Δψm increased from 2.49% in the control group to 8.93, 23.5, and 25.5% at 32, 64, and 128 μg/ml NBP, respectively. Besides, NBP obviously reduced intracellular Rh123 MFI of C. albicans in a dose dependent manner (Figure 7E), also suggesting a decrease in Δψm. Thus, NBP induced a loss in Δψm of C. albicans and consequently caused mitochondrial dysfunction.
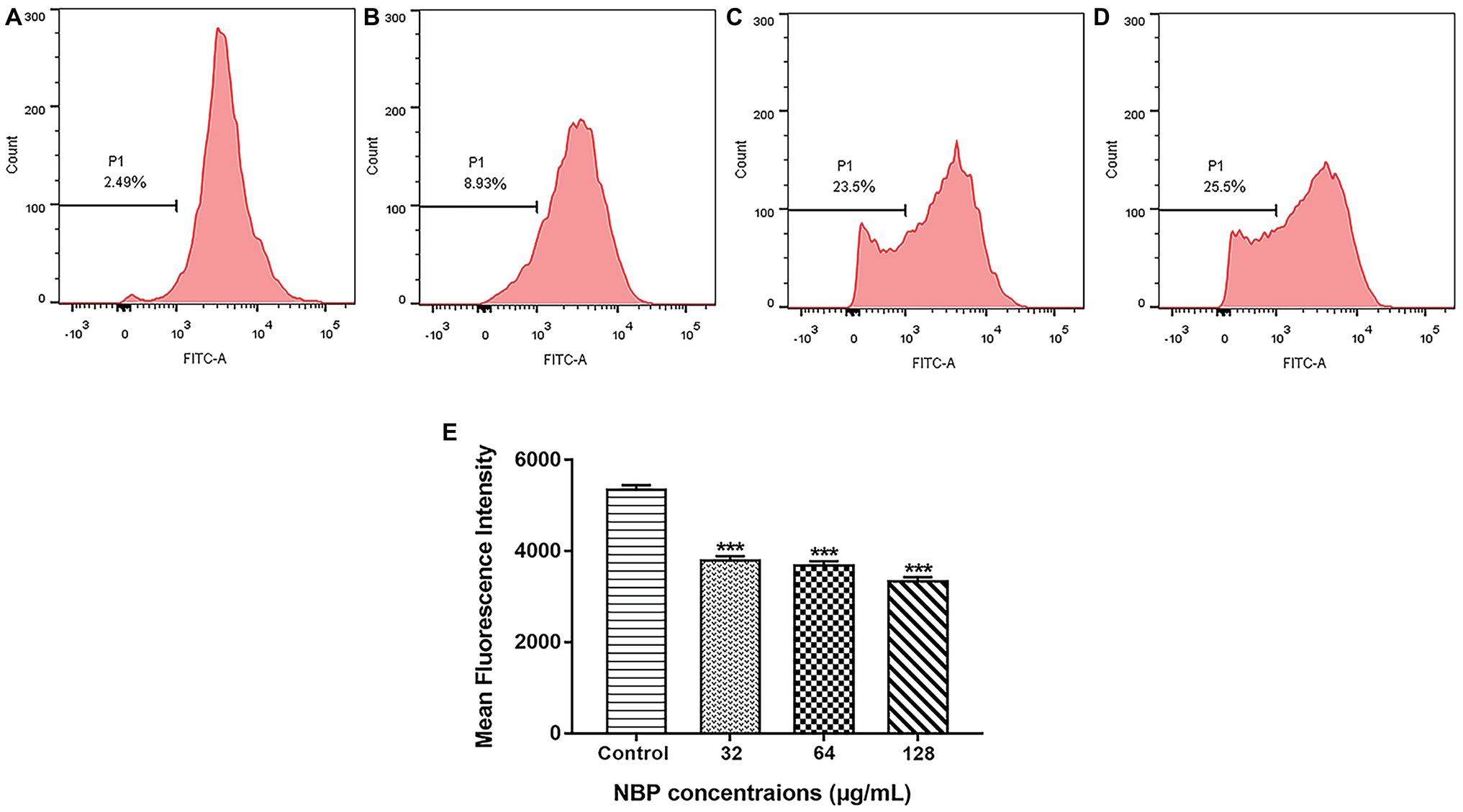
Figure 7. Effect of NBP on the Δψm of C. albicans. Yeast cells pretreated with diverse concentrations of NBP (0, 32, 64, and 128 μg/ml) for 4 h were incubated with 15 μM Rh123 and then analyzed by a flow cytometer. (A–D) Effect of different doses of NBP on Δψm loss in C. albicans. (A) Negative control, (B) 64, (C) 128, (D) 256 μg/ml. Representative images from three independent experiments are shown. The percentages of cells in the left section of the histogram refer to the number of cells with depolarized mitochondria. (E) Effect of NBP on the mean fluorescence intensity of Rh123-stained C. albicans. ***p < 0.001 compared with the control group (one-way ANOVA).
Effects of N-Butylphthalide on Drug Uptake and Efflux of C. albicans and Drug Transporters Genes
In the drug uptake experiment, NBP significantly increased drug absorption after 20 min and cells in the NBP-treated group absorbed higher concentrations of Rh6G than the control group (p < 0.001) (Figure 8A). In the drug efflux experiment, after treated with glucose solution, the MFI both in the control group and NBP-treated group showed a downward trend. However, NBP evidently suppressed the decrease especially after 120 min (p < 0.001) and cells treated with NBP pumped out much lower concentration of Rh6G compared with the control group in 200 min (Figure 8B). Subsequently, gene expression experiments confirmed that NBP significantly down-regulated the expression levels of genes, CDR1 and CDR2, that encode C. albicans drug resistant protein (Cdrp), however, for the expression level of MDR1, there was no difference between the NBP-treated group and the control group (Figure 9). In a word, the results of Rh6G assay and RT-PCR method suggest that the synergistic antifungal effect of NBP and FLC was related to promote drug uptake and reverse drug efflux via down-regulating drug transporters genes, CDR1 and CDR2.
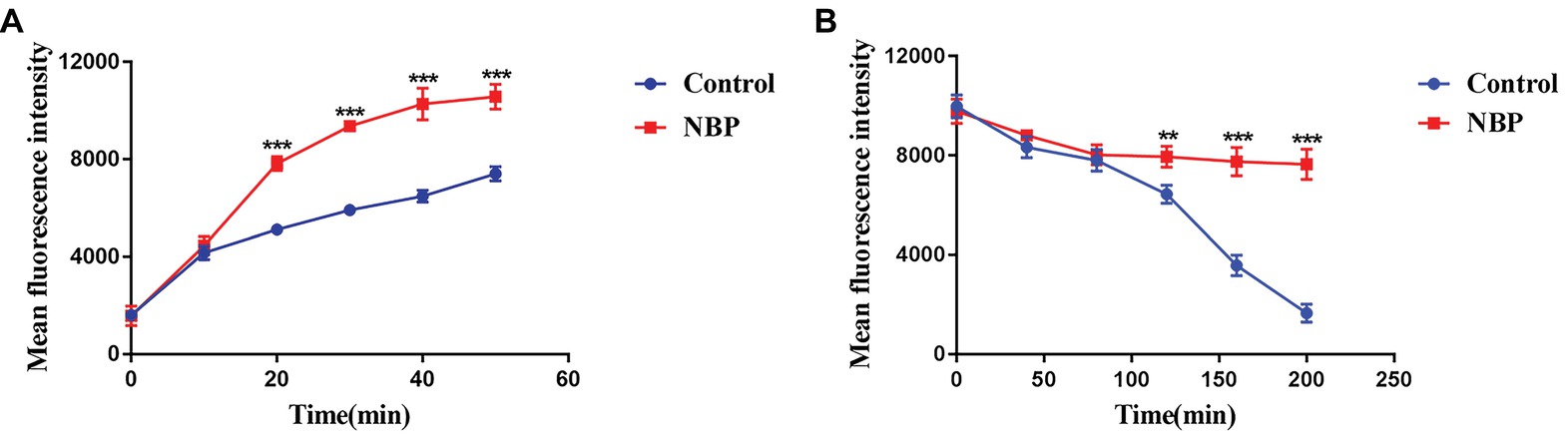
Figure 8. Effect of NBP on drug uptake (A) and efflux (B) of C. albicans tested by Rh6G assay. The concentration of NBP is 32 μg/ml and cells treated without NBP were served as the control group. Mean fluorescence intensity represented intracellular Rh6G and were measured by a flow cytometer. Values represent the means ± standard deviation of three replicates. **p < 0.01 and ***p < 0.001 compared with the control group (unpaired t-test).
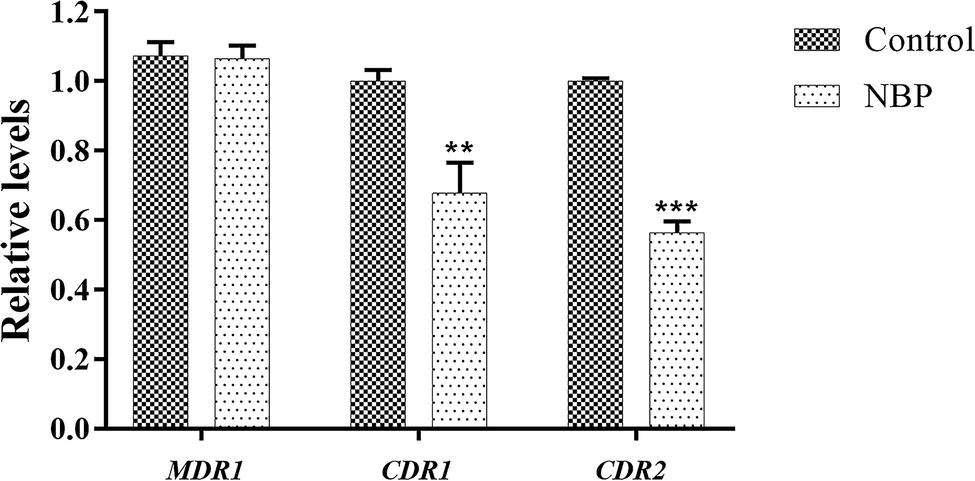
Figure 9. Relative expressions of drug transporter encoding genes MDR1, CDR1, and CDR2. The concentration of NBP is 32 μg/l and cells treated without NBP were served as the control group. Values represent the means ± standard deviation of three replicates. **p < 0.01 and ***p < 0.001 compared with the respective control group (unpaired t-test).
Discussion
Treatment of fungal infections, especially candidiasis, is still a challenging problem due to the rising isolation rates of resistant strains (Pfaller and Diekema, 2007). In our previous studies, we focused on studying the mechanism of drug resistance in C. albicans and exploring more non-antifungal drugs with antifungal activity to overcome drug resistance of C. albicans, especially sensitizers of traditional antifungal drugs, such as antibiotics, glucocorticoid and calcium channel blockers (Liu et al., 2016; Sun et al., 2017; Lu et al., 2018). In this study, we demonstrated NBP, a component of Apium graveolens seeds and currently used for the treatment of ischemic stroke in clinic, exerted antifungal activity against C. albicans with MICs of 128 μg/ml and NBP combined with FLC showed synergistic effects against resistant C. albicans, leading to a decrease in the MICs of FLC from >512 to 0.25–1 μg/ml. Besides, time-killing curves provided figures that described dynamic antifungal effects of NBP alone and synergistically combined with FLC on C. albicans, which was in accordance with those from broth microdilution assays.
Biofilms, consisting of complicated communities of microorganisms embedded in cells-derived matrix, build a heterogeneous and natural drug-tolerant environment (Bonhomme and d’Enfert, 2013). C. albicans biofilms, especially formed on medical implants, are common during C. albicans infections and pose a great challenge to clinical treatment due to the intrinsic resistance to most antifungal drugs (Mathe and Van Dijck, 2013). We demonstrated that NBP exerted anti-biofilm activity against C. albicans biofilms pre-formed <12 h with sMICs of 128–256 μg/ml and NBP combined with FLC showed synergistic effects against resistant C. albicans biofilms pre-formed <12 h, leading to a decrease in the sMICs of FLC from >1,024 to 0.5–8 μg/ml. These results indicated that NBP has broad prospects in prevention and treatment of immature C. albicans biofilms related infections.
The G. mellonella model, whose immune response is similar with that of mammals, is used as an infection model host and Galleria mellonella-Candida albicans infection model is widely applicable to rapidly evaluate the efficacy of drugs against C. albicans infections in vivo (Li et al., 2013; Aneja et al., 2016). Compared with mammalian model, G. mellonella model has many advantages, such as significant ethical, economical, accessible and easy manipulative (Mylonakis et al., 2007; Vilcinskas, 2011). Besides, the G. mellonella larvae infection model belongs to invertebrate and does not require ethical approval. In the present study, we found that NBP monotherapy enhanced survival rate of C. albicans infected larvae, cleared more C. albicans cells in larvae and caused less damage to larval tissues compared with the control group, while the combined treatment of NBP and FLC exhibited a better therapeutic effects. Acute toxicity test indicated that median lethal dose of NBP to mice was much higher than the effective dose; moreover, chronic toxicity test excluded chronic adverse events during long-term application of NBP (Tian et al., 2016). The doses (0.4 μg/larva for NBP and 1.6 μg/larva for FLC) used to treat larvae was converted from those used to treated human beings. Thus, in consideration of therapeutic effects on G. mellonella infection model and good safety of NBP, it is promising for application of NBP combined with antifungal drugs against C. albicans infections, while more researches are needed to be carried out in future.
For the potential mechanisms exploration, we first measured the effects of NBP on hyphal growth. As a dimorphic fungus, C. albicans proliferated in either a yeast form or a hypha form (Wilson et al., 2016). Hyphae are crucial components of C. albicans biofilms and required for virulence and pathogenicity, contributing to adhesion and invasion of host cells (Whiteway and Bachewich, 2007). The results showed that the morphological characteristics of C. albicans hyphae, induced by both RPMI 1640 and spider medium, were similar and NBP inhibited C. albicans hyphal growth in a dose-dependent manner. Hyphae induced by 64 μg/ml NBP were shorter and looser than that of control group, while 128 μg/ml NBP obviously inhibited the yeast-to-hypha morphological transition and C. albicans were mainly maintained in yeast form. Thus, NBP exhibited antifungal activity via inhibiting hyphal growth, attenuating virulence factors and reducing invasiveness and pathogenicity.
Generation of ROS regulates the process of apoptosis in eukaryotes, leading to enzyme inactivation, cells dysfunction and subsequent cell death (Perrone et al., 2008; Chen et al., 2013). Mitochondria is a major subcellular source of intracellular ROS. ΔΨm plays a coupling role in mitochondrial oxidative phosphorylation and its stability is beneficial to maintain regular physiological functions of cells (Dai et al., 2015; Zhu et al., 2015). In this study, NBP significantly induced C. albicans intracellular ROS accumulation and a loss in C. albicans Δψm in a dose-dependent manner. Recent studies showed the induction of excessive ROS in C. albicans was a critical factor in cell death induced by many antifungal drugs (Chen et al., 2013; Chang et al., 2017). Depolarization of the mitochondrial membrane indicates the change of Δψm and the subsequent flow of the outer membrane is also a critical stage in the intrinsic apoptosis pathway (Chen et al., 2015; Wang et al., 2015). Thus, the results suggested NBP induced C. albicans cells death was probably triggered by stimulated intracellular ROS accumulation and dysfunction of mitochondrion, finally leading to cell death through mediation of apoptosis.
It is extensively accepted that resistance of C. albicans to most antifungal drugs is partly mediated by the efflux mechanism. The non-specificity of drug efflux pump transporters possibly explains the phenomenon of cross-resistance between diverse antifungal drugs (Pina-Vaz et al., 2005). Generous studies demonstrated that inhibiting the activity of drug efflux pumps might be a non-negligible mechanism to illustrate the synergism of interactions between non-antifungal drugs and conventional antifungal drugs (Pina-Vaz et al., 2005; Li et al., 2017). In this study, the results suggested that NBP significantly promoted drug uptake of C. albicans. Indeed, C. albicans could agglomerate and adhere to form biofilms that are important biological barrier for drug diffusion and inherent resistant to most antifungal therapy. With the formation of biofilms, cell viscosity increases, and drug diffusion slows down. NBP could inhibit the C. albicans biofilms, which may be one of the reasons why NBP promotes drug uptake by destroying the physical barrier of biofilms. Subsequently, it was demonstrated that NBP suppressed drug efflux, led to high levels of FLC concentrations in fungal cells and then possibly increased the susceptibility of C. albicans to FLC. RT-PCR method further confirmed that NBP down-regulated the expressions of drug transporters genes CDR1 and CDR2. Thus, the synergistic effect of NBP and FLC relied on promoting drug uptake and reversing the mechanism of drug efflux targeting drug transporters genes CDR1 and CDR2.
In summary, NBP alone exhibited antifungal activity against both planktonic C. albicans and biofilms. Strong synergism was observed when NBP combined with FLC against resistant C. albicans and biofilms pre-formed by resistant strains. Time-killing curves confirmed antifungal effects of NBP in dynamic. The antifungal activity of NBP was further confirmed in vivo with G. mellonella infection model. Mechanism researches showed that NBP could inhibit the hyphal growth, induced intracellular ROS accumulation and caused mitochondrial dysfunction. Besides, the mechanism of synergism between NBP and FLC relied on promotion of drug uptake, suppression drug efflux by down-regulating drug transporters genes CDR1 and CDR2. To the best of our knowledge, this study is the first to elucidate the antifungal activity of NBP both in vitro and in vivo and explore the potential mechanisms. These findings might provide insights into the possible therapeutic application of NBP as antifungal agents or sensitizers of traditional antifungal drugs in the future.
Author Contributions
YG, WL, and SS designed the experiments. YG and YL performed the experiments. YG, XH, and LH interpreted the data. YG and SS wrote the manuscript. All authors approved the manuscript for publication.
Funding
This study was supported by the Administration of Traditional Chinese Medicine of Shandong Province, China (2017-166); Health and Family Planning Commission of Jinan Municipality, China (2017-2-20); and Department of Science and Technology of Shandong Province, China (2017G006038).
Conflict of Interest Statement
The authors declare that the research was conducted in the absence of any commercial or financial relationships that could be construed as a potential conflict of interest.
Acknowledgments
We are grateful to the Translational Medicine Research Centre in Qianfoshan Hospital Affiliated to Shandong University, China, for laboratory assistance, and all members of the anti-fungal resistance study group for support and valuable opinions.
References
Abdoulaye, I. A., and Guo, Y. J. (2016). A review of recent advances in neuroprotective potential of 3-n-butylphthalide and its derivatives. Biomed. Res. Int. 2016:5012341. doi: 10.1155/2016/5012341
Aneja, B., Irfan, M., Kapil, C., Jairajpuri, M. A., Maguire, R., Kavanagh, K., et al. (2016). Effect of novel triazole-amino acid hybrids on growth and virulence of Candida species: in vitro and in vivo studies. Org. Biomol. Chem. 14, 10599–10619. doi: 10.1039/c6ob01718e
Bonhomme, J., and D’enfert, C. (2013). Candida albicans biofilms: building a heterogeneous, drug-tolerant environment. Curr. Opin. Microbiol. 16, 398–403. doi: 10.1016/j.mib.2013.03.007
Chang, W., Li, Y., Zhang, M., Zheng, S., Li, Y., and Lou, H. (2017). Solasodine-3-O-beta-d-glucopyranoside kills Candida albicans by disrupting the intracellular vacuole. Food Chem. Toxicol. 106, 139–146. doi: 10.1016/j.fct.2017.05.045
Chen, N. J., Hao, F. Y., Liu, H., Zhao, H., and Li, J. M. (2015). Capillarisin exhibits anticancer effects by inducing apoptosis, cell cycle arrest and mitochondrial membrane potential loss in osteosarcoma cancer cells (HOS). Drug Res. 65, 422–427. doi: 10.1055/s-0034-1387728
Chen, Y., Zeng, H., Tian, J., Ban, X., Ma, B., and Wang, Y. (2013). Antifungal mechanism of essential oil from Anethum graveolens seeds against Candida albicans. J. Med. Microbiol. 62, 1175–1183. doi: 10.1099/jmm.0.055467-0
Da, W., Shao, J., Li, Q., Shi, G., Wang, T., Wu, D., et al. (2019). Physical interaction of sodium houttuyfonate with beta-1,3-glucan evokes Candida albicans cell wall remodeling. Front. Microbiol. 10:34. doi: 10.3389/fmicb.2019.00034
Dai, J. Q., Huang, Y. G., and He, A. N. (2015). Dihydromethysticin kavalactone induces apoptosis in osteosarcoma cells through modulation of PI3K/Akt pathway, disruption of mitochondrial membrane potential and inducing cell cycle arrest. Int. J. Clin. Exp. Pathol. 8, 4356–4366.
Desai, J. V., Mitchell, A. P., and Andes, D. R. (2014). Fungal biofilms, drug resistance, and recurrent infection. Cold Spring Harb. Perspect. Med. 4, 1–18. doi: 10.1101/cshperspect.a019729
Dimopoulos, G., Karabinis, A., Samonis, G., and Falagas, M. E. (2007). Candidemia in immunocompromised and immunocompetent critically ill patients: a prospective comparative study. Eur. J. Clin. Microbiol. Infect. Dis. 26, 377–384. doi: 10.1007/s10096-007-0316-2
Frenkel, M., Mandelblat, M., Alastruey-Izquierdo, A., Mendlovic, S., Semis, R., and Segal, E. (2016). Pathogenicity of Candida albicans isolates from bloodstream and mucosal candidiasis assessed in mice and Galleria mellonella. J. Mycol. Med. 26, 1–8. doi: 10.1016/j.mycmed.2015.12.006
Gu, W., Guo, D., Zhang, L., Xu, D., and Sun, S. (2016). The synergistic effect of azoles and fluoxetine against resistant Candida albicans strains is attributed to attenuating fungal virulence. Antimicrob. Agents Chemother. 60, 6179–6188. doi: 10.1128/AAC.03046-15
Haque, F., Alfatah, M., Ganesan, K., and Bhattacharyya, M. S. (2016). Inhibitory effect of sophorolipid on Candida albicans biofilm formation and hyphal growth. Sci. Rep. 6:23575. doi: 10.1038/srep23575
Huang, W., Duan, Q., Li, F., Shao, J., Cheng, H., and Wu, D. (2015). Sodium houttuyfonate and EDTA-Na(2) in combination effectively inhibits Pseudomonas aeruginosa, Staphylococcus aureus and Candida albicans in vitro and in vivo. Bioorg. Med. Chem. Lett. 25, 142–147. doi: 10.1016/j.bmcl.2014.10.072
Institute, CaLS (2008a). “Reference method for broth dilution antifungal susceptibility testing of yeasts; fourth informational supplement” in CLSI Document M27-S4. eds. J. H. Rex, B. D. Alexander, D. Andes, B. Arthington-Skaggs, S. D. Brown, and V. Chaturvedi, et al.(Wayne, PA: Clinical and Laboratory Standards Institute).
Institute, CaLS (2008b). “Reference method for broth dilution antifungal susceptibility testing of yeasts; approved standard. 3rd Edn” in CLSI Document M27-A3. eds. J. H. Rex, B. D. Alexander, D. Andes, B. Arthington-Skaggs, S. D. Brown, and V. Chaturvedi, et al.(Wayne, PA: Clinical and Laboratory Standards Institute).
Khan, M. S., and Ahmad, I. (2012). Antibiofilm activity of certain phytocompounds and their synergy with fluconazole against Candida albicans biofilms. J. Antimicrob. Chemother. 67, 618–621. doi: 10.1093/jac/dkr512
Krezdorn, J., Adams, S., and Coote, P. J. (2014). A Galleria mellonella infection model reveals double and triple antibiotic combination therapies with enhanced efficacy versus a multidrug-resistant strain of Pseudomonas aeruginosa. J. Med. Microbiol. 63, 945–955. doi: 10.1099/jmm.0.074245-0
Lewis, R. E., Diekema, D. J., Messer, S. A., Pfaller, M. A., and Klepser, M. E. (2002). Comparison of Etest, chequerboard dilution and time-kill studies for the detection of synergy or antagonism between antifungal agents tested against Candida species. J. Antimicrob. Chemother. 49, 345–351. doi: 10.1093/jac/49.2.345
Li, Y., Chang, W., Zhang, M., Li, X., Jiao, Y., and Lou, H. (2015). Synergistic and drug-resistant reversing effects of diorcinol D combined with fluconazole against Candida albicans. FEMS Yeast Res. 15, 1–10. doi: 10.1093/femsyr/fov001
Li, D. D., Deng, L., Hu, G. H., Zhao, L. X., Hu, D. D., Jiang, Y. Y., et al. (2013). Using Galleria mellonella-Candida albicans infection model to evaluate antifungal agents. Biol. Pharm. Bull. 36, 1482–1487. doi: 10.1248/bpb.b13-00270
Li, H. Z., Guo, J., Gao, J., Han, L. P., Jiang, C. M., Li, H. X., et al. (2011). Role of dopamine D2 receptors in ischemia/reperfusion induced apoptosis of cultured neonatal rat cardiomyocytes. J. Biomed. Sci. 18:18. doi: 10.1186/1423-0127-18-18
Li, Y., Sun, S., Guo, Q., Ma, L., Shi, C., Su, L., et al. (2008). In vitro interaction between azoles and cyclosporin a against clinical isolates of Candida albicans determined by the chequerboard method and time-kill curves. J. Antimicrob. Chemother. 61, 577–585. doi: 10.1093/jac/dkm493
Li, H., Zhang, C., Chen, Z., Shi, W., and Sun, S. (2014b). A promising approach of overcoming the intrinsic resistance of Candida krusei to fluconazole (FLC)–combining tacrolimus with FLC. FEMS Yeast Res. 14, 808–811. doi: 10.1111/1567-1364.12163
Li, X., Zhao, Y., Huang, X., Yu, C., Yang, Y., and Sun, S. (2017). Ambroxol hydrochloride combined with fluconazole reverses the resistance of Candida albicans to fluconazole. Front. Cell. Infect. Microbiol. 7:124. doi: 10.3389/fcimb.2017.00124
Li, D. D., Zhao, L. X., Mylonakis, E., Hu, G. H., Zou, Y., Huang, T. K., et al. (2014a). In vitro and in vivo activities of pterostilbene against Candida albicans biofilms. Antimicrob. Agents Chemother. 58, 2344–2355. doi: 10.1128/AAC.01583-13
Liu, S., Yue, L., Gu, W., Li, X., Zhang, L., and Sun, S. (2016). Synergistic effect of fluconazole and calcium channel blockers against resistant Candida albicans. PLoS One 11:e0150859. doi: 10.1371/journal.pone.0168743
Lu, M., Li, T., Wan, J., Li, X., Yuan, L., and Sun, S. (2017). Antifungal effects of phytocompounds on Candida species alone and in combination with fluconazole. Int. J. Antimicrob. Agents 49, 125–136. doi: 10.1016/j.ijantimicag.2016.10.021
Lu, M., Yu, C., Cui, X., Shi, J., Yuan, L., and Sun, S. (2018). Gentamicin synergises with azoles against drug-resistant Candida albicans. Int. J. Antimicrob. Agents 51, 107–114. doi: 10.1016/j.ijantimicag.2017.09.012
Lukowska-Chojnacka, E., Mierzejewska, J., Milner-Krawczyk, M., Bondaryk, M., and Staniszewska, M. (2016). Synthesis of novel tetrazole derivatives and evaluation of their antifungal activity. Bioorg. Med. Chem. 24, 6058–6065. doi: 10.1016/j.bmc.2016.09.066
Mathe, L., and Van Dijck, P. (2013). Recent insights into Candida albicans biofilm resistance mechanisms. Curr. Genet. 59, 251–264. doi: 10.1007/s00294-013-0400-3
Mishra, B. B., and Tiwari, V. K. (2011). Natural products: an evolving role in future drug discovery. Eur. J. Med. Chem. 46, 4769–4807. doi: 10.1016/j.ejmech.2011.07.057
Momin, R. A., and Nair, M. G. (2001). Mosquitocidal, nematicidal, and antifungal compounds from Apium graveolens L. seeds. J. Agric. Food Chem. 49, 142–145. doi: 10.1021/jf001052a
Momin, R. A., Ramsewak, R. S., and Nair, M. G. (2000). Bioactive compounds and 1,3-Di[(cis)-9-octadecenoyl]-2-[(cis,cis)-9, 12-octadecadienoyl]glycerol from Apium graveolens L. seeds. J. Agric. Food Chem. 48, 3785–3788. doi: 10.1021/jf991383r
Mylonakis, E., Casadevall, A., and Ausubel, F. M. (2007). Exploiting amoeboid and non-vertebrate animal model systems to study the virulence of human pathogenic fungi. PLoS Pathog. 3:e101. doi: 10.1371/journal.ppat.0030101
Odds, F. C. (2003). Synergy, antagonism, and what the chequerboard puts between them. J. Antimicrob. Chemother. 52:1. doi: 10.1093/jac/dkg301
Peralta, M. A., Calise, M., Fornari, M. C., Ortega, M. G., Diez, R. A., Cabrera, J. L., et al. (2012). A prenylated flavanone from Dalea elegans inhibits rhodamine 6 G efflux and reverses fluconazole-resistance in Candida albicans. Planta Med. 78, 981–987. doi: 10.1055/s-0031-1298627
Perrone, G. G., Tan, S. X., and Dawes, I. W. (2008). Reactive oxygen species and yeast apoptosis. Biochim. Biophys. Acta 1783, 1354–1368. doi: 10.1016/j.bbamcr.2008.01.023
Pfaffl, M. W. (2001). A new mathematical model for relative quantification in real-time RT-PCR. Nucleic Acids Res. 29:e45. doi: 10.1093/nar/29.9.e45
Pfaller, M. A., and Diekema, D. J. (2007). Epidemiology of invasive candidiasis: a persistent public health problem. Clin. Microbiol. Rev. 20, 133–163. doi: 10.1128/CMR.00029-06
Pina-Vaz, C., Rodrigues, A. G., Costa-De-Oliveira, S., Ricardo, E., and Mardh, P. A. (2005). Potent synergic effect between ibuprofen and azoles on Candida resulting from blockade of efflux pumps as determined by FUN-1 staining and flow cytometry. J. Antimicrob. Chemother. 56, 678–685. doi: 10.1093/jac/dki264
Prazynska, M., and Gospodarek, E. (2014). In vitro effect of amphotericin B on Candida albicans, Candida glabrata and Candida parapsilosis biofilm formation. Mycopathologia 177, 19–27. doi: 10.1007/s11046-014-9727-7
Ramage, G., and Lopez-Ribot, J. L. (2005). Techniques for antifungal susceptibility testing of Candida albicans biofilms. Methods Mol. Med. 118, 71–79. doi: 10.1385/1-59259-943-5:071
Shao, J., Cui, Y., Zhang, M., Wang, T., Wu, D., and Wang, C. (2017). Synergistic in vitro activity of sodium houttuyfonate with fluconazole against clinical Candida albicans strains under planktonic growing conditions. Pharm. Biol. 55, 355–359. doi: 10.1080/13880209.2016.1237977
Shrestha, S. K., Fosso, M. Y., and Garneau-Tsodikova, S. (2015). A combination approach to treating fungal infections. Sci. Rep. 5:17070. doi: 10.1038/srep17070
Suleyman, G., and Alangaden, G. J. (2016). Nosocomial fungal infections: epidemiology, infection control, and prevention. Infect. Dis. Clin. North. Am. 30, 1023–1052. doi: 10.1016/j.idc.2010.11.003
Sun, W., Wang, D., Yu, C., Huang, X., Li, X., and Sun, S. (2017). Strong synergism of dexamethasone in combination with fluconazole against resistant Candida albicans mediated by inhibiting drug efflux and reducing virulence. Int. J. Antimicrob. Agents 50, 399–405. doi: 10.1016/j.ijantimicag.2017.03.015
Tian, X., He, W., Liu, S., Yang, R., and Liu, S. (2016). Studies on the acute and chronic toxicity test of dl-3-n-butylphthalide in mice (in Chinese). J. HeBei Med. Univ. 37, 1250–1252. doi: 10.3969/j.issn.1007-3205.2016.11.003
Vilcinskas, A. (2011). Insects emerge as valuable model hosts to explore virulence. Virulence 2, 376–378. doi: 10.4161/viru.2.5.18289
Wang, L., He, H. S., Yu, H. L., Zeng, Y., Han, H., He, N., et al. (2015). Sclareol, a plant diterpene, exhibits potent antiproliferative effects via the induction of apoptosis and mitochondrial membrane potential loss in osteosarcoma cancer cells. Mol. Med. Rep. 11, 4273–4278. doi: 10.3892/mmr.2015.3325
Whaley, S. G., Berkow, E. L., Rybak, J. M., Nishimoto, A. T., Barker, K. S., and Rogers, P. D. (2016). Azole antifungal resistance in Candida albicans and emerging non-albicans Candida species. Front. Microbiol. 7:2173. doi: 10.3389/fmicb.2016.02173
Whiteway, M., and Bachewich, C. (2007). Morphogenesis in Candida albicans. Annu. Rev. Microbiol. 61, 529–553. doi: 10.1146/annurev.micro.61.080706.093341
Wilson, D., Naglik, J. R., and Hube, B. (2016). The missing link between Candida albicans hyphal morphogenesis and host cell damage. PLoS Pathog. 12:e1005867. doi: 10.1371/journal.ppat.1005867
Zhao, W., Luo, C., Wang, J., Gong, J., Li, B., Gong, Y., et al. (2014). 3-N-butylphthalide improves neuronal morphology after chronic cerebral ischemia. Neural Regen. Res. 9, 719–726. doi: 10.4103/1673-5374.131576
Zheng, S., Chang, W., Zhang, M., Shi, H., and Lou, H. (2018). Chiloscyphenol A derived from Chinese liverworts exerts fungicidal action by eliciting both mitochondrial dysfunction and plasma membrane destruction. Sci. Rep. 8:326. doi: 10.1038/s41598-017-18717-9
Zhong, H., Hu, D. D., Hu, G. H., Su, J., Bi, S., Zhang, Z. E., et al. (2017). Activity of Sanguinarine against Candida albicans biofilms. Antimicrob. Agents Chemother. 61, 1–24. doi: 10.1128/AAC.02259-16
Keywords: antifungal activity, Candida albicans, fluconazole, n-butylphthalide, potential mechanism
Citation: Gong Y, Liu W, Huang X, Hao L, Li Y and Sun S (2019) Antifungal Activity and Potential Mechanism of N-Butylphthalide Alone and in Combination With Fluconazole Against Candida albicans. Front. Microbiol. 10:1461. doi: 10.3389/fmicb.2019.01461
Edited by:
Miguel Cacho Teixeira, University of Lisbon, PortugalReviewed by:
Rajendra Prasad, Jawaharlal Nehru University, IndiaDaqiang Wu, Anhui University of Chinese Medicine, China
Copyright © 2019 Gong, Liu, Huang, Hao, Li and Sun. This is an open-access article distributed under the terms of the Creative Commons Attribution License (CC BY). The use, distribution or reproduction in other forums is permitted, provided the original author(s) and the copyright owner(s) are credited and that the original publication in this journal is cited, in accordance with accepted academic practice. No use, distribution or reproduction is permitted which does not comply with these terms.
*Correspondence: Shujuan Sun, c3Vuc2h1anVhbjg4OEAxNjMuY29t