- 1State Key Laboratory of Ecological Pest Control for Fujian and Taiwan Crops, Fujian Agriculture and Forestry University, Fujian, China
- 2Fujian Provincial Key Laboratory of Insect Ecology, College of Plant Protection, Fujian Agriculture and Forestry University, Fujian, China
Red palm weevil (RPW), Rhynchophorus ferrugineus Olivier, is a destructive pest for palm trees worldwide. Recent studies have shown that RPW gut is colonized by microbes and alterations in gut microbiota can significantly modify its hemolymph nutrition content. However, the exact effects of gut microbiota on RPW phenotype and the underlying mechanisms remain elusive. Here germ-free (GF) RPW larvae were generated from dechorionated eggs which were reared on sterilized artificial food under axenic conditions. Compared with controls, the larval development of GF RPW individuals was markedly depressed and their body mass was reduced as well. Furthermore, the content of hemolymph protein, glucose and triglyceride were dropped significantly in GF RPW larvae. Interestingly, introducing gut microbiota into GF individuals could significantly increase the levels of the three nutrition indices. Additionally, it has also been demonstrated that RPW larvae monoassociated with Lactococcus lactis exhibited the same level of protein content with the CR (conventionally reared) insects while feeding Enterobacter cloacae to GF larvae increased their hemolymph triglyceride and glucose content markedly. Consequently, our findings suggest that gut microbiota profoundly affect the development of this pest by regulating its nutrition metabolism and different gut bacterial species show distinct impact on host physiology. Taken together, the establishment of GF and gnotobiotic RPW larvae will advance the elucidation of molecular mechanisms behind the interactions between RPW and its gut microbiota.
Introduction
It is ubiquitous that animal hosts, including insects, have established symbiotic interactions with microbes. Increasing evidence from invertebrate model organism Drosophila melanogaster have indicated that intestinal microbes can impact many host physiological traits such as development, immunity, maturation, longevity and nutrition (Shin et al., 2011; Storelli et al., 2011; Wong et al., 2014; Strigini and Leulier, 2016) and fitness-related behaviors including mating preference (Sharon et al., 2011), foraging decision (Wong et al., 2017) and social communications (Venu et al., 2014). Interestingly, the similar profound effects of commensal microbiota on growth, health and disease of honey bee, Apis mellifera, have been also determined recently (Zheng et al., 2017; Raymann and Moran, 2018). In recent years, the diversity and composition of gut microbiota associated with some notorious pests, containing the cotton bollworm Helicoverpa armigera (Xiang et al., 2006), Oriental fruit fly Bactrocera dorsalis Hendel (Shi et al., 2012), Diamondback moth Plutella xylostella L. (Xia et al., 2013), Red turpentine beetle Dendroctonus valens LeConte (Zhou F. et al., 2017; Cheng et al., 2018) and Spodoptera littoralis (Chen et al., 2016), have been intensively investigated.
Unfortunately, the physiological mechanisms by which these insect pests maintain the symbiotic interactions with their gut commensal bacteria are still poorly understood. It is increasingly recognized that one means by which intestinal microbiota communicate with their hosts is through metabolic byproducts that arise from bacterial catabolism of host diet (Kamareddine et al., 2018). Gut microbiota derived short chain fatty acids (SCFAs) have been confirmed to be crucial for host health by executing important metabolic functions (Canfora et al., 2015; Koh et al., 2016; Fellows et al., 2018). For instance, these metabolites are recognized by specific G protein-coupled receptors on enteroendocrine cells, and then these cells release small enteroendocrine peptides to modulate local and systemic lipid and carbohydrate metabolism to maintain host homeostasis (Bolognini et al., 2016; Miyamoto et al., 2016). Recently, a study on D. melanogaster has revealed that a SCFA, acetate, increases the secretion of the endocrine peptide Tachykinin to maintain the timely larval development and optimal lipid metabolism (Kamareddine et al., 2018). Insecta is the most diverse biological group which contains over a million known species of insects with distinct life history. They have been found in almost every possible nutritional niche on Earth. Therefore, the knowledge from D. melanogaster and mosquitoes cannot be simply generalized to the notorious agricultural pests. Given the pivotal effects of gut microbiota on hosts, it is of great significance to investigate the mechanisms underlying the interactions between pests and their gut microbiota which will uncover the novel potential target to boost the development of new promising management tactics on these notorious pests by disrupting these symbiotic associations.
Red palm weevil (RPW), Rhynchophorus ferrugineus Olivier, is the most devastating insect pest of palm trees worldwide (Giblin-Davis et al., 2013). This pest is native to South Eastern Asia, but it has spread to the Middle East, Africa and the Mediterranean due to the international exchange of infected plant materials. More recently, RPW has also been found in China, Japan, Australia, and the Caribbean (Zhang et al., 2003; Wang et al., 2015). Since its invasion in China, RPW has killed almost 20,000 coconut trees and its infestation area is over 10,000 km2, and thus has seriously threatened the ecological security of Chinese coastal system (Shi et al., 2014; Ge et al., 2016). RPW larvae are the major infestation agents which feed on the tender tissue inside the trunk which have abundant carbohydrates but poor assimilable nitrogen sources (Nagnan et al., 1992). Currently, it has been found that RPW gut harbors a complex bacterial community which can degrade polysaccharides and sucrose (Butera et al., 2012; Jia et al., 2013; Montagna et al., 2015). Furthermore, changes in the composition of gut microbiota can significantly influence the nutrition metabolism of this pest (Muhammad et al., 2017; Dawadi et al., 2018; Xiao et al., 2019). Because RPW gut microbiota is composed of a plethora of different bacterial species, distinguishing the impact of a specific bacterial species on host fitness is challenging. Interestingly, it is a powerful and effective way to study the intestinal mutualism by generating germ free (GF) and gnotobiotic experimental models in D. melanogaster and mice (Shin et al., 2011; Nicholson et al., 2012).
In the present study, we developed a protocol on how to generate GF RPW larvae firstly. And then the exact effects of gut microbiota on the development, body mass gain and survival rate of this pest were determined with this GF RPW model. Furthermore, the concentration of some important nutritional indices, containing hemolymph protein, glucose, triglyceride, and trehalose which can indicate the nutrition and metabolic status of animal host well (Ridley et al., 2012; Newell and Douglas, 2014), were measured to reveal the physiological consequences of gut microbiota on their host phenotype. It has been verified that Enterobacter cloacae can produce various glycolytic enzymes, containing cellulases, trehalases and other glucosidases, which participate in food digestion (Xia et al., 2017; Adhav et al., 2019). Moreover, Lactococcus lactis is a lactic acid bacterium which is involved in digesting and fermenting some plant polymers to improve insect nutrition acquisition (Passerini et al., 2013; Zhou H. et al., 2017). Both E. cloacae RPWL3 (access no. MF185375) and L. lactis RPWL8 (access no. MF185378) are present in RPW gut with higher abundance (Muhammad et al., 2017), so their influence on host nutrition status were determined by making gnotobiotic insects in this study. Collectively, we provided the evidence to show the promoting effects of gut microbiota on the development and body mass gain of RPW larvae by improving their nutrition metabolism. The generation of GF and gnotobiotic RPW larvae set the groundwork to facilitate the understanding of molecular physiological mechanisms that promote RPW microbial coexistence and the processes by which intestinal microbiota affect host fitness.
Materials and Methods
Insect Rearing and Maintaining
Lab RPW population were established and maintained from the adults which were trapped in Jinshan campus of Fujian Agriculture and Forestry university (119°30′ E, 26°08′ N) and Pingtan District (119°32′ E, 25°31′ N) of Fujian Province. The adults were fed with sugarcane stems in the incubator (Saifu ZRX-260, Ningbo Experimental Instrument Co., Ltd., China) at 27 ± 1°C, 75% relative humidity (RH) under the photoperiod of 12 h light/12 h dark, while larvae were reared under the following conditions: 27 ± 1°C, 75% RH and a photoperiod of 24 h dark. The fresh eggs, within 12 h after oviposition, were collected for dechorionation to generate GF insects. These collected eggs were divided into the following two groups: the dechorionated eggs which were processed to sequential washing by 10% sodium hypochlorite solution, 75% ethanol and sterilized water, others served as the control which was only treated with sterilized water. These treated eggs were moved to a sterilized Petri dish with autoclaved wet cottons for hatching and their hatching status were observed every 24 h. After dechorionation, the germfree individuals were always maintained in the laminar hood (Heal Force safe-1200LC) which can prevent the bacterial contamination from outside environment. The neonatal larvae were fed on sterile artificial diet (date palm tissue 8.0g, sucrose 8.0g, agar 6.0g, casein 8.0g, corn flour 10.0g, yeast extract 12.0g, avicel 5.0g, ascorbic acid as vitamin C 1.0g, potassium sorbate 0.4g, sodium p-hydroxybenzoate 0.2g, cholesterol 0.3g, choline chloride 0.25g, inositol 0.02g, and 220 ml distilled water) with or without antibiotic cocktail (Pu and Hou, 2016). Antibiotic cocktail (Kanamycin 150 mg/L, Tetracycline 150 mg/L, Gentamicin 150 mg/L, and Erythromycin 150 mg/L) and 1g ascorbic acid was added to the sterilized artificial diet when the temperature dropped to about 55°C. Each larva was provided with 3 g artificial food which was refreshed every 2 day. The axenic RPW individuals were generated as follows: the collected fresh eggs were washed in 10% sodium hypochlorite solution (NaClO) for 3–5 min, rinsed in 75% ethanol two times to remove the bacteria species on the egg surface, and then rinsed with sterilized water two times to exclude the potential effect of hypochlorite and ethanol on the following experiments. In the control group, the fresh eggs were only washed with sterilized distilled water by the same procedure. Subsequently, these treated eggs were transferred to the sterilized Petri dish (90 mm in diameter) containing autoclaved wet cottons for hatching. After dechorionation, the germfree individuals were always maintained in the laminar hood (Heal Force safe-1200LC) which can prevent the bacterial contamination from outside environment. All larvae were maintained at 27 ± 1°C, 75% relative humidity (RH) under the photoperiod of 24 h dark. However, the conventionally reared ones were maintained in the common incubator (Saifu ZRX-260, Ningbo Experimental Instrument Co., Ltd., China). Overall, there were four treatments: dechorionated eggs + food with antibiotics (GF), dechorionated eggs + food without antibiotics (DNA), non-dechorionated eggs + food with antibiotics (NDA), and non-dechorionated eggs + food without antibiotics (CR). Insects in the former three treatments were maintained in the laminar hood while the fourth group was reared in the incubator. To generate GF+B larvae through introducing the intestinal microbiota to GF larvae, 50 μl gut homogenates from CR larvae were poured on sterilized artificial food to feed them. Each treatment contained three replicates and three fifth instar larvae were used in a replicate.
The fifth instar GF larvae were employed to generate the gnotobiotic insects and they were transferred to sterilized food without antibiotics. E. cloacae RPWL3 and L. lactis RPWL8 were cultivated at 37°C overnight in nutrient broth (NB, tryptone 10 g/L, beef extract powder 5 g/L NaCl 10 g/L and pH 7.2). Then 50 μl bacterial suspensions (OD600 = 0.15) of two species were spread on sterilized food to feed the fifth instar GF RPW larvae. During the generation of gnotobiotic insects, their food was refreshed every 2 day. For the control groups, the same volume of NB was introduced on the food to feed insects. Seven day later, four insects were randomly selected from each group to verify the load of two introduced bacterial species. Gut homogenates of these insects were prepared, poured on nutrient agar (NA, tryptone 10.0g/L, beef extract powder 3.0g/L, NaCl 5.0g/L, 15.0g/L agar and pH 7.2) and incubated as above. Bacterial species was determined by 16S rRNA-based PCR diagnosis and the number of colony-forming units (CFUs) was counted 24 h after incubation.
Verification of Germfree RPW Individuals With Culture-Dependent and–Independent Assays
To verify if there were some commensal bacteria in the gut of RPW larvae, three Fifth-instar larvae were randomly dissected to collect guts. Before dissection, each individual surface was cleaned with 75% ethanol, followed by three rinses in the sterilized water. The whole gut of each insect was pulled out in a clean Petri dish with sterilized forceps. Each gut was put in 1 ml sterile PBS as a replicate and homogenized by the Scientz-48 tissue lyser (Ningbo Scientz BioTech. Co. Ltd, China). Each treatment comprises at least three replicates. In the culture-dependent assays, 100 μl gut homogenate was poured on the nutrient agar media in triplicate after serial dilution (10-1 to 10-4) and incubated aerobically at 37°C for 24 h. Finally, the number of bacterial colony forming units (CFUs) was counted for further analysis.
By using a DNeasy Blood & Tissue Kit (Qiagen), total gut bacterial DNA was extracted from RPW larvae in the four treatments according to the procedures as described by Muhammad et al. (2017). To detect if there were any bacteria in RPW gut with a highly sensitive way, PCR diagnosis with bacterial 16S rRNA primers (27F: 5′-AGAGTTTGATCATGGC TCAG-3′, 1492R: 5′-TACGGYTACCTTGTTACGACTT-3′) was performed with total reaction volume of 25 μl reaction system, being composed of 50 ng template DNA, 1 μl forward primer, 1 μl reverse primer and 12.5 μl of 2X Taq PCR Mastermix (Beijing Tiangen BioTech Co., Ltd., China). PCR reactions were run with 1 cycle of denaturation at 94°C for 3 min, 30 cycles at 94°C for 30 s, amplification at 55°C for 30 s, and a dissociation step. Finally, the PCR products were determined by electrophoresis on 1% agarose gel stained with ethidium bromide and visualized under UV light.
Effect of Gut Microbiota on RPW Physiological Traits and Nutrition Status
The instar and survival of each larva were observed daily and its body mass was also measured by an electric microbalance (Mettler Toledo AL104) to the accuracy of 0.01 μg. The seventh instar larvae were used to collect the hemolymph for the quantification of four nutritional indices, including protein, glucose, triglyceride (TAG) and trehalose concentration. Before hemolymph collection, each larva was washed with running water to remove excrement and food particles, and then anesthetized for 3–5 min on ice for immobilization. Subsequently, its epidermis was pierced by a fine sharp needle for collecting 50 μl of hemolymph per larva into a labeled 1.5 ml clean micro-centrifuge tube with 2 μl of 0.2% phenylthiourea (PTU) to inhibit the hemolymph coagulation. The protein content of each sample was analyzed with the BCA protein assay kit (Beijing Tiangen BioTech. Co., Ltd., China). Glucose was measured using Glucose Measurement Kit (Shanghai Rongsheng Biology Pharmaceutical Co., Ltd., China). TAG content was determined with Triglyceride assay kit (Zhejiang Dongou Diagnostic Products Co., Ltd., China). The concentration of trehalose was quantified with trehalose assay kit (Megazyme Bray, Co., Wicklow, Ireland), following the manufacturer’s instructions.
Statistical Analysis
The differences in body mass, development duration and eggs hatching success between GF and conventionally reared (CR) group was detected by independent t-test. The variations of nutrition indices across different groups were assessed by analysis of variance (ANOVA) and multiple comparisons were conducted with Tukey’s HSD post hoc analysis. Before ANOVA or t-test, Kolmogorov-Smirnov test and test of homogeneity of variances was run for determining if our data has normal distribution and equal variances, respectively. If not, the data were transformed to meet the prerequisite of parametric tests. All analyses were performed using IBM SPSS Statistics (22.0). Log rank test procedure was used to determine effects of microbiota on survival test. The significance level to threshold was set at P < 0.05.
Results
The Generation and Verification of Germfree RPW Larvae
The culture-dependent validations revealed that the greatest quantity of bacterial colonies was found in guts of CR group, followed by DNA and NDA, while no bacterial colony was detected in GF group (Figures 1A,B). Moreover, PCR diagnosis based on bacterial 16S rRNA uncovered that the expected bands, with the size of about 1500 bp, only missed in the GF group, indicating that the guts of GF RPW larvae was not colonized by any bacteria species (Figure 1C). Therefore, RPW larvae without any intestinal bacteria species were successfully generated and maintained with our protocols.
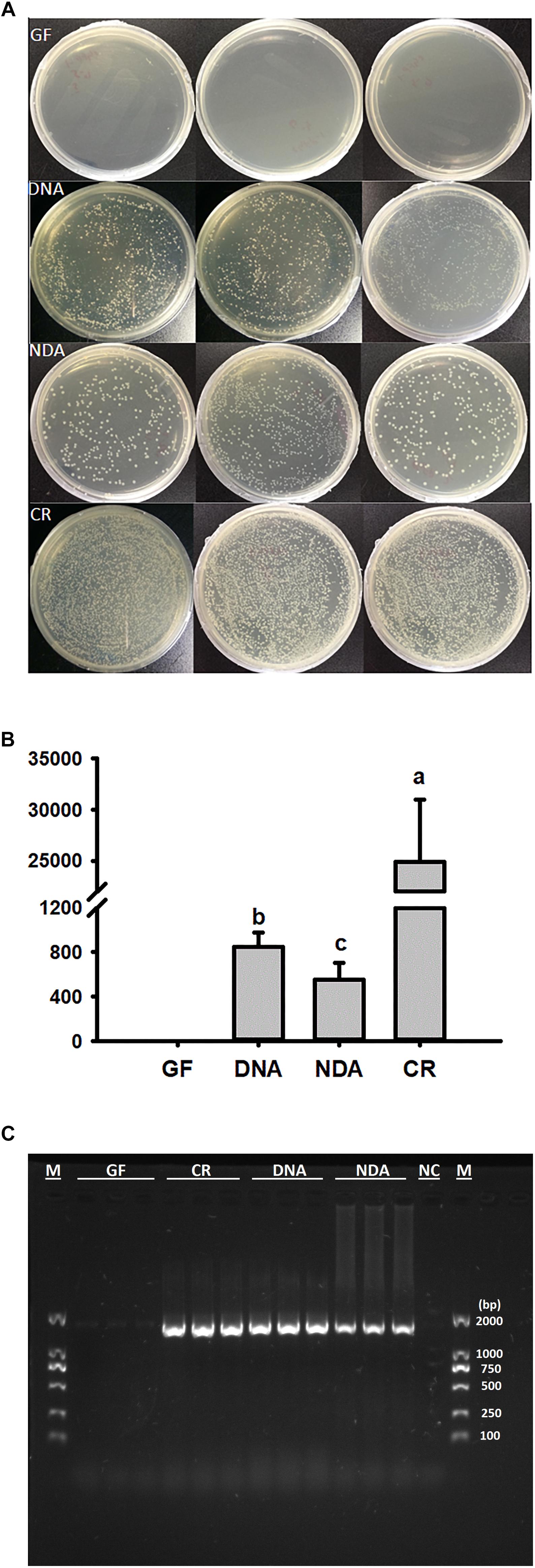
Figure 1. Verification of gut bacteria in the larvae of Rhynchophorus ferrugineus with culture-dependent (A,B) 10-4 dilution plating, and –independent methods (C). GF, dechorionated eggs + food with antibiotics; DNA, dechorionated eggs + food without antibiotics; NDA, non-dechorionated eggs + food with antibiotics; CR, non-dechorionated eggs + food without antibiotics.
Effect of Gut Microbiota on Insect on Hatching Success, Survival and Body Mass
No significant difference was detected in hatching success (t-test: t = 0.310, P = 0.91) and the time to hatching of dechorionated eggs and controls (2.59 ± 0.94 and 2.38 ± 0.61 day, t-test: t = -1.55, df = 26, P = 0.30, Table 1). The survival rate of RPW individuals to prepupa in the two groups were not significantly different from each other (log rank test P = 0.069; X2 = 3.2, Figure 2), indicating that the RPW-gut microbe symbiosis is facultative, not obligate. Furthermore, body mass of RPW GF larvae was significantly less than that of CR insects (t-test = -2.29, df = 22, P = 0.032). When compared to CR larvae, body mass of RPW GF individuals were decreased by 17.59% (Figure 3).
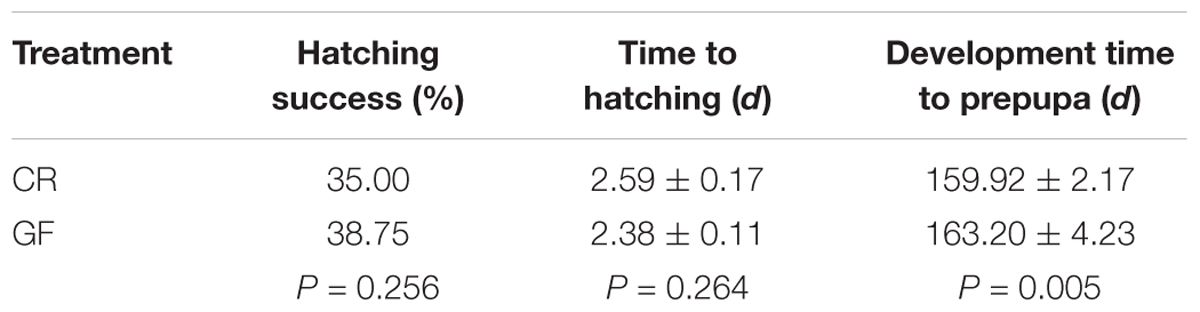
Table 1. Comparisons on the fitness parameters of conventionally reared (CR) and germfree (GF) larvae of Rhynchophorus ferrugineus Olivier.
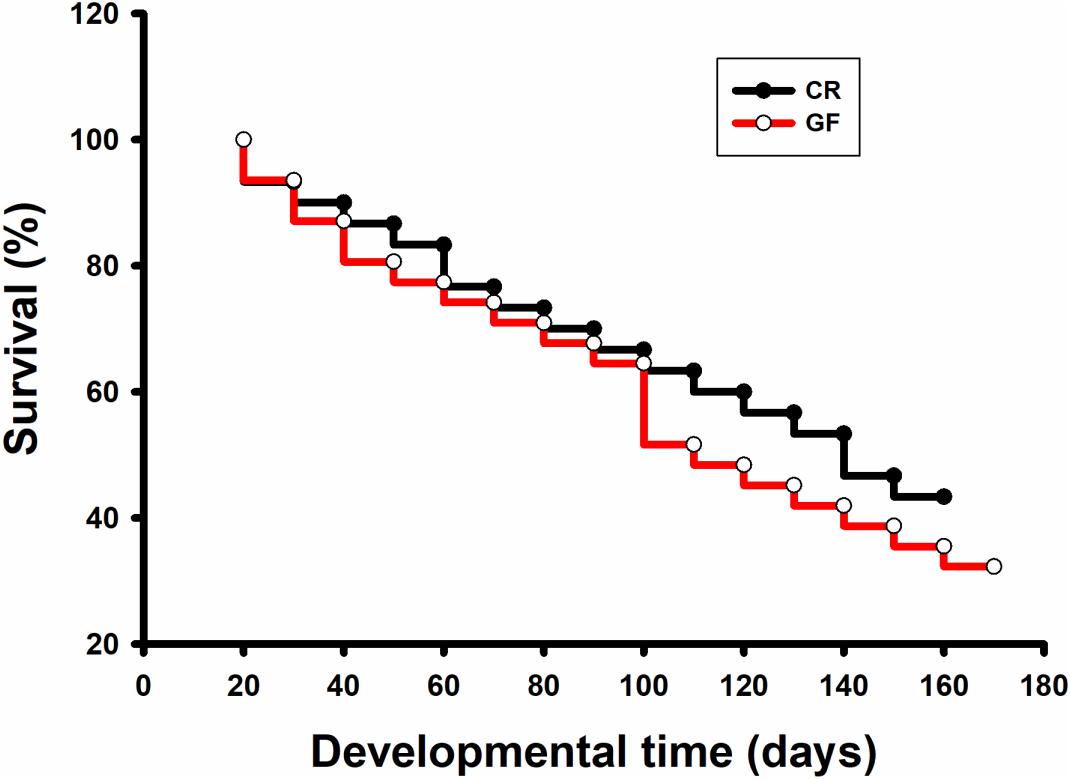
Figure 2. Survival analysis of conventionally reared (CR) and germfree (GF) Rhynchophorus ferrugineus larvae.
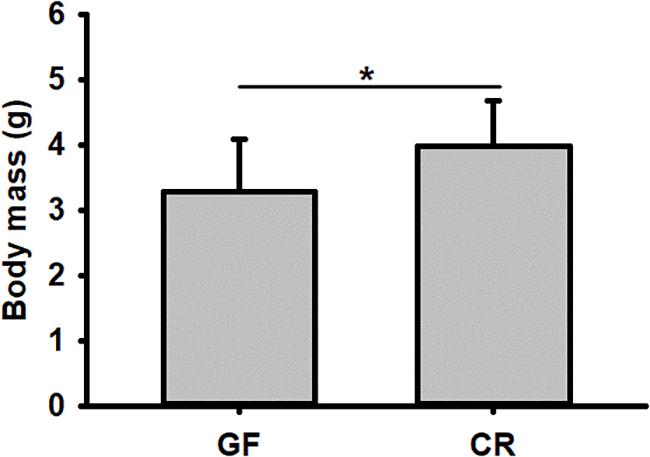
Figure 3. Body mass of conventionally reared (CR) and germfree (GF) Rhynchophorus ferrugineus larvae. ∗Indicates the significance between two groups.
Effect of Gut Microbiota on the Concentration of Hemolymph Protein, Glucose, TG and Trehalose in RPW Larvae
No significant differences were found in our assayed nutrient indices in RPW larvae from NDA and CR group (Figure 4), revealing that the introduction of antibiotic into artificial food had no significant effects on RPW nutrient indices. Interestingly, the concentration of hemolymph protein (ANOVA: F2,20 = 38.02, P < 0.001, Figure 4A), glucose (ANOVA: F4,20 = 615.31, P < 0.001, Figure 4B) and TAG (ANOVA: F4,20 = 527.84, P < 0.001, Figure 4C) in the CR individuals were significantly higher than those of GF individuals.
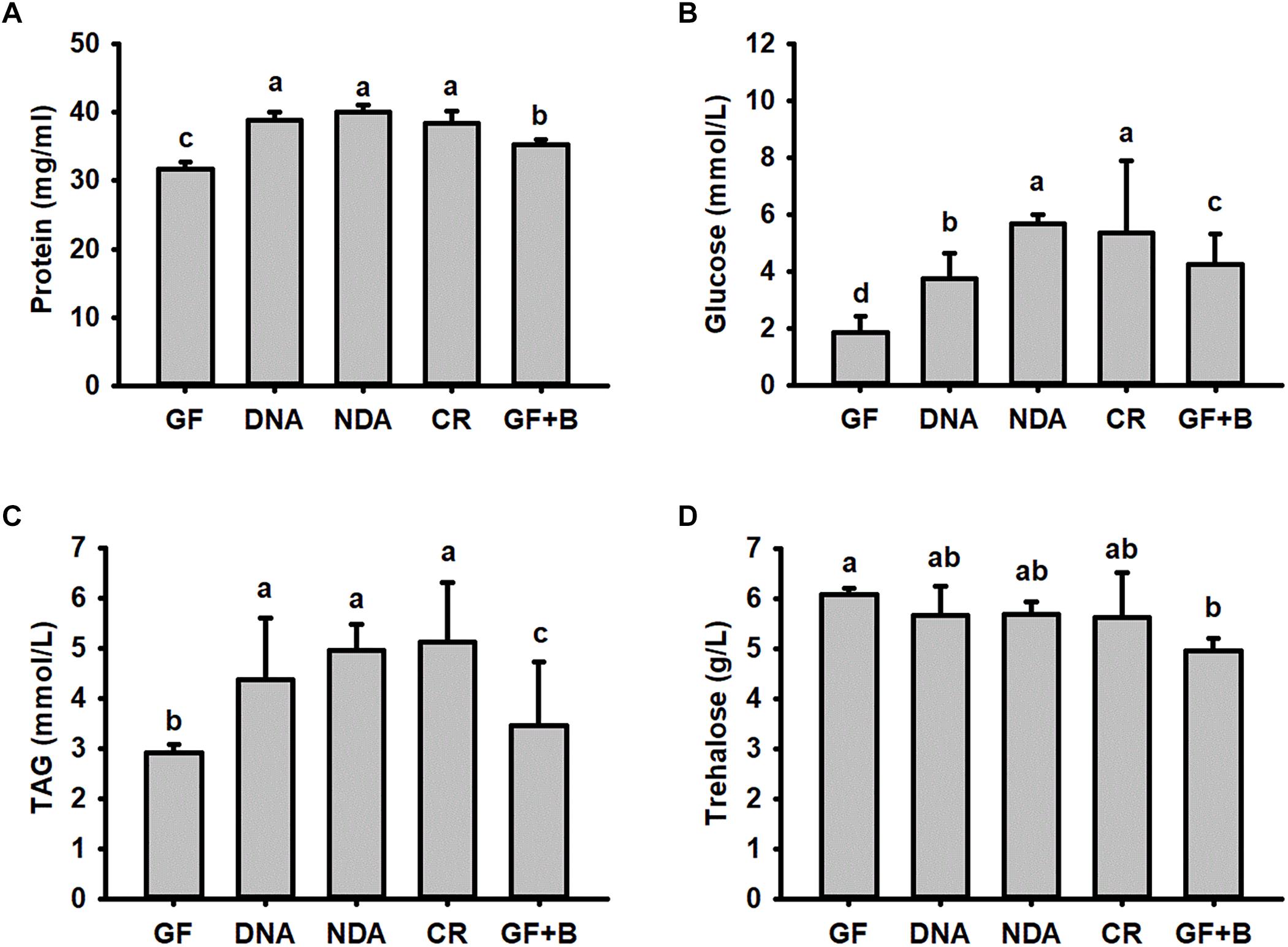
Figure 4. The content of hemolymph protein (A), glucose (B), triglyceride (C) and trehalose (D) in Rhynchophorus ferrugineus larvae with different treatments. GF, dechorionated eggs + food with antibiotics; DNA, dechorionated eggs + food without antibiotics; NDA, non-dechorionated eggs + food with antibiotics; CR, non-dechorionated eggs + food without antibiotics; GF+B, GF larvae established the interactions with gut bacteria by feeding gut homogenates of CR. The different letters above each graph indicate the significance across groups.
The concentrations of protein, glucose and TAG in GF+B larvae, with introduced gut microbiota, were still markedly lower than those of CR insects. But their contents were significantly increased in contrast with GF larvae (Figure 4). Therefore, these data confirmed that introducing intestinal bacteria into the guts of GF larvae could restore their nutritional status dramatically. Significant difference was also determined in the content of trehalose across different groups (ANOVA: F4,20 = 3.26, P = 0.033, Figure 4D). However, the content of trehalose of GF+B larvae was significantly lower than that of GF individuals. Taken together, these data suggested that intestinal commensal bacteria have profound effects on the nutrition metabolism of RPW larvae.
Impact of Lactococcus lactis and Enterobacter cloacae Monoassciation With RPW Larvae on the Concentration of Hemolymph Protein, Glucose, TAG and Trehalose
The number of E. cloacae and L. lactis, being harvested from gut homogenate of gnotobiotic larvae was 30,317.66 ± 1913.98 CFUs/ml and 24150.00 ± 4084.42 CFUs/ml (Figure 5), respectively. These data indicated that gnotobiotic RPW larvae with E. cloacae and L. lactis were successfully established. Furthermore, we also found that gnotobiotic RPW larvae with L. lactis exhibited markedly higher protein content as compared to that of GF insects (ANOVA: F3,12 = 8.459, P = 0.003, Figure 6A), suggesting that L. lactis could restore the protein level to that of CR larvae. In contrast, the level of TAG (ANOVA: F3,12 = 12.357, P = 0.001, Figure 6B) and glucose (ANOVA: F3,12 = 13.215, P < 0.001, Figure 6C) in gnotobiotic larvae with E. cloacae were significantly improved in contrast to the GF individuals. But no significant difference was detected in trehalose content between gnotobiotic and GF groups (ANOVA: F3,12 = 1.985, P = 0.304, Figure 6D). Consequently, we provided the evidence to show that two gut bacteria species, E. cloacae and L. lactis, could play different roles in regulating the nutrition status of RPW larvae.
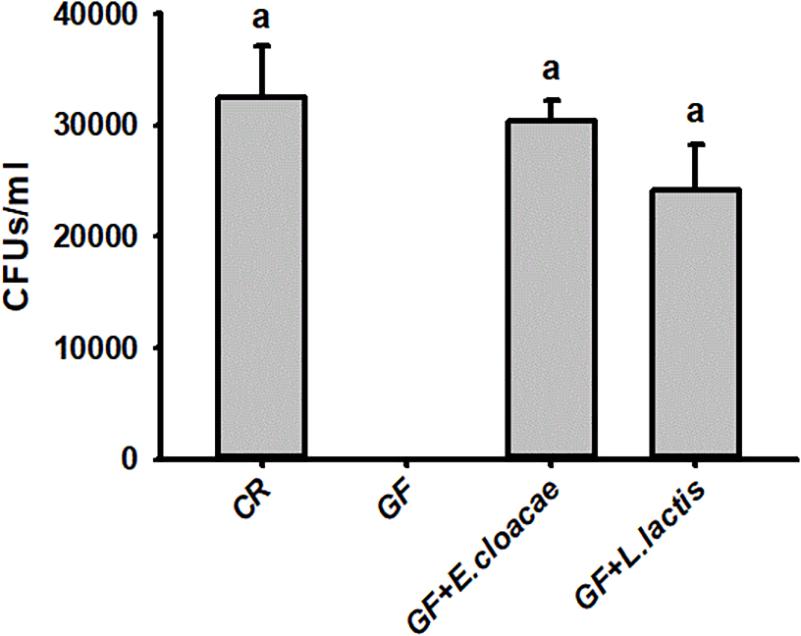
Figure 5. The CFU number of Enterobacter cloacae and Lactococcus lactis were harvested from the guts of Rhynchophorus ferrugineus gnotobiotic larvae.
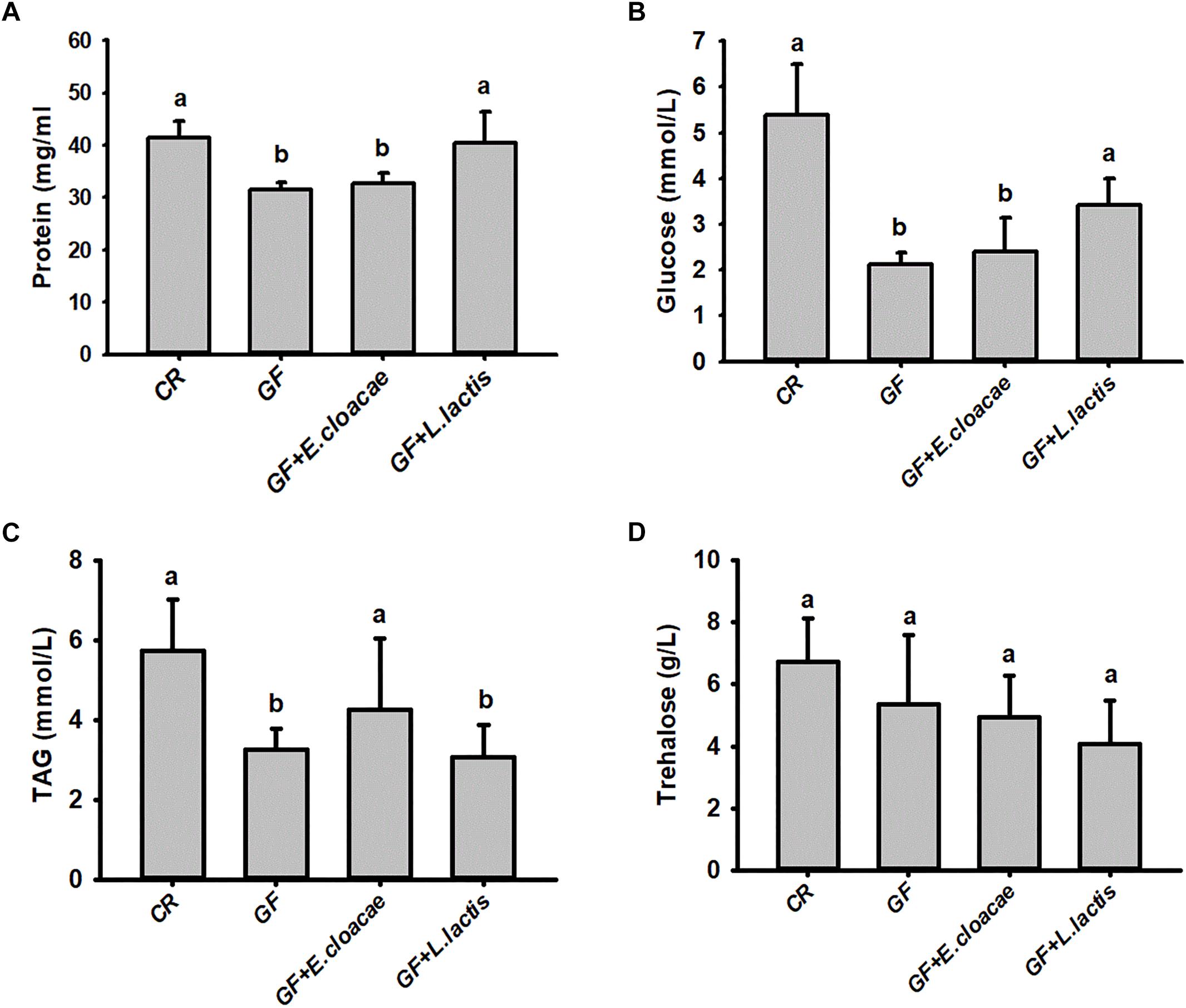
Figure 6. The level of hemolymph protein (A), glucose (B), triglyceride (C) and trehalose (D) in the gnotobiotic larvae inoculated with Lactococcus lactis and Enterobacter cloacae. The data on glucose (B) and triglyceride (C) were transformed by Log10 and Square root to meet the prerequisite of ANOVA, respectively. The different letters above each graph indicate the significance across groups.
Discussion
Recent evidence demonstrated that RPW harbor a complex gut microbiota with profound effects on food digestion and nutrition assimilation of this pest (Butera et al., 2012; Jia et al., 2013; Montagna et al., 2015; Muhammad et al., 2017). However, it is still unknown whether the specific bacterial community or the interactions between different bacterial groups mediate these physiological traits and the molecular dialogue that shapes host-microbiota mutualism because of the complexity of gut microbiota. To this end, GF RPW larvae were generated and maintained by our protocols reported here. Previous investigations have revealed that insect gut microbiota can be transferred vertically via depositing gut bacteria on the egg surface (Behar et al., 2008). Embryo dechorionation is an important step as it has been confirmed that eggshell layer carries microbes from adults (Sabat et al., 2015; Kolye et al., 2016; Kietz et al., 2018). Recently, it has been verified that D. melanogaster must consume bacteria from its food to establish and maintain its microbiome (Blum et al., 2013). In the present study, we provide the evidence to show that dechorionation and sterilized food with antibiotics are used together to successfully make RPW GF larvae. Dechorionation can remove the bacteria on egg surface, while antibiotics are able to prevent the proliferation of gut bacteria in the sterilized food. The generation of GF RPW larvae provides a tool for dissecting the different aspects of host-microbe interplay. More importantly, it has also laid a strong foundation for determining the precise role of specific bacteria on host by generating gnotobiotic model organism.
We found body mass of RPW GF larvae was less than that of CR insects and the development rate from eggs to GF prepupae was prolonged significantly as well, suggesting the promoting effect of gut microbiota on the growth and development of this pest. Additionally, our data uncovered that the content of hemolymph protein, glucose and triglyceride of GF larvae were significantly lower than that of CR insects. Candidate bacterial products are acetic acid and lactic acid, which are derived from Acetobacter and Lactobacillus species, and are known to reduce the digestibility of starch and other carbohydrates by mammals (Johnston et al., 2010; Matzkin et al., 2011). L. plantarum in D. melanogaster can enhance its dietary protein digestion and amino acid intake by upregulating the expression of intestinal peptidases (Erkosar et al., 2015). Therefore, introducing gut bacteria into RPW GF larvae could restore the above nutrition indices markedly. In this way, these data suggest that less body mass gain of GF larvae is the resulting effect of gut microbiota absence-mediated nutrition metabolic defects. This is consistent with the recent reports on the impact of gut microbiota on D. melanogaster (Shin et al., 2011; Storelli et al., 2011, 2018) and honeybee A. mellifera (Zheng et al., 2017). To note, previous investigations have also revealed that gut microbiota can enhance body mass gain and development via modulating insulin signaling (Shin et al., 2011; Storelli and Leulier, 2016; Zheng et al., 2017). Unfortunately, what gut microbiota-derived chemical signals result in the promoting effects on insect host phenotype remains largely unknown. In mammals, it has been verified that gut microbiota-derived short-chain fatty acids, such as acetate, butyrate and propionate, serve as the key metabolites to contribute to host health and disease via providing energy and modifying chromatin status (Donohoe et al., 2011; Lee and Hase, 2014; Koh et al., 2016; Krautkramer et al., 2016; Fellows et al., 2018). More recently, Zheng et al. (2017) found that the honeybee gut bacteria produce short-chain fatty acids, being dominated by acetate and propionate, affects bee growth, gut physicochemical conditions and hormonal signaling. Yet the profile of gut microbiota-derived short-chain fatty acids and molecular mechanisms behind these short-chain fatty acids on the physiological traits of insects need further extensive elucidation.
In this study, we uncovered that feeding L. lactis to RPW GF larvae could elevate the protein content significantly. L. lactis, being involved in the production of fermented dairy products containing lactic acid (Bolotin et al., 2001), is present as a dominant species in RPW gut in July (Jia et al., 2013; Tagliavia et al., 2014). Lactobacillus has been used as model lactic acid bacteria because they are widely recognized as the potential health beneficial microbes in human gastrointestinal tract (Perpetuini et al., 2013; Drissi et al., 2014). More interestingly, L. plantarum can promote the growth of Drosophila under chronic undernutrition via enhancing the expression of intestinal peptidases and then increasing host dietary protein digestion and amino acid intake (Erkosar et al., 2015). Furthermore, L. plantarum-derived D-alanylated teichoic acids can ensure the optimal intestinal peptidase expression (Matos et al., 2017). However, the mechanisms underlying the promoting effects of L. lactis on the nitrogen nutrition assimilation of this pest remain elusive. Because L. lactis is a lactic acid bacterium as well, we postulate that this commensal bacterium possibly enhance host physiology via the similar actions.
Enterobacteriaceae has been determined as the dominant populations in the RPW gut (Jia et al., 2013; Tagliavia et al., 2014; Muhammad et al., 2017). Members of Enterobacteriaceae are known as nitrogen fixers (Behar et al., 2005; Morales-Jiménez et al., 2009; Ben-Yosef et al., 2014). Here, we also found that RPW gnotobiotic larvae with E. cloacae exhibited the dramatically recapitulated level of hemolymph glucose and triglyceride, being consistent with the observed effects of E. cloacae B29 in gnotobiotic mice (Fei and Zhao, 2013). E. cloacae, producing various carbohydrate modifying and glycolytic enzymes like cellulases, trehalases and other glucosidase to facilitate insect nutrition acquisition, was found to be the most abundant species of gut bacteria in P. xylostella larva (Xia et al., 2017). Recently, it has been unveiled that E. cloacae can assist in trehalose hydrolysis by secreting trehalase to modulate energy metabolism in P. xylostella (Adhav et al., 2019). In this context, it can be deduced that E. cloacae might release some carbohydrate hydrolytic enzymes into RPW larva gut to facilitate the digestion of food polymers to regulate its energy metabolism. Collectively, the mechanisms behind how E. cloacae and L. lactis affect nutrition metabolism of RPW need be defined further with these gnotobiotic models.
Conclusion
In summary, we determined that RPW has established facultative mutualistic interplays with its gut microbiota. Gut bacteria promote the development and growth of RPW via modulating nutrition metabolism of this pest. Additionally, the monoassociation of two dominant gut bacteria, L. lactis and E. cloacae, could restore the content of some nutrition in contrast to GF RPW larvae. Given the importance of gut microbiota on RPW physiology, it is a very promising alternative way to control this pest by disrupting the interactions between RPW and its gut microbiota.
Ethics Statement
Rhynchophorus ferrugineus is exempted from above mentioned requirements.
Author Contributions
ZS conceived and designed this project. PH, AM, TJ, RX, and XY performed the experiments. ZS, PH, AM and YH performed the analysis and wrote this manuscript. All authors have approved the final version of the manuscript.
Funding
This work is granted by the National Key Research and Development Program of China (2017YFC1200605), National Natural Science Foundation of China (31470656) and Natural Science Foundation of Fujian Province (2018J01705).
Conflict of Interest Statement
The authors declare that the research was conducted in the absence of any commercial or financial relationships that could be construed as a potential conflict of interest.
References
Adhav, A., Harne, S., Bhide, A., Giri, A., Pananghat, G., and Joshi, R. (2019). Mechanistic insights into enzymatic catalysis by trehalase from the insect gut endosymbiont Enterobacter cloacae. FEBS J. 286, 1700–1716. doi: 10.1111/febs.14760
Behar, A., Jurkevitch, E., and Yuval, B. (2008). Bringing back the fruit into fruit fly-bacteria interactions. Mol. Ecol. 17, 1375–1386. doi: 10.1111/j.1365-294X.2008.03674.x
Behar, A., Yuval, B., and Jurkevitch, E. (2005). Enterobacteria-meidated nitrogen fixation in natural populations of the fruit fly Ceratitis capitata. Mol. Ecol. 14, 2637–2643. doi: 10.1111/j.1365-294X.2005.02615.x
Ben-Yosef, M., Pasternak, Z., Jurkevitch, E., and Yuval, B. (2014). Symbiotic bacteria enable olive flies (Bacterocera oleae) to exploit intractable sources of nitrogen. J. Evol. Biol. 27, 2695–2705. doi: 10.1111/jeb.12527
Blum, J. E., Fischer, C. N., Miles, J., and Handelsman, J. (2013). Frequent replenishment sustains the beneficial microbiome of Drosophila melanogaster. mBio 4:e0860. doi: 10.1128/mBio.00860-13
Bolognini, D., Tobin, A. B., Milligan, G., and Moss, C. E. (2016). The pharmacology and function of receptors for short-chain fatty acids. Mol. Pharmacol. 89, 388–398. doi: 10.1124/mol.115.102301
Bolotin, A., Wincker, P., Mauger, S., Jaillon, O., Malarme, K., Weissenbach, J., et al. (2001). The complete genome sequence of the lactic acid bacterium Lactococcus lactis ssp. Lactis IL1403. Genome Res. 11, 731–775. doi: 10.1101/gr.169701
Butera, G., Ferraro, C., Colazza, S., Alonzo, G., and Quatrini, P. (2012). The cultural bacterial community of frass produced by larvae of Rhynchophorus ferrugineus Olivier (Coleoptera: Curculionidae) in the Canary island date palm. Lett. Appl. Microbiol. 54, 530–536. doi: 10.1111/j.1472-765X.2012.03238.x
Canfora, E., Jocken, J., and Blaak, E. (2015). Short-chain fatty acids in control of body weight and insulin sensitivity. Nat. Rev. Endocrinol. 11, 577–591. doi: 10.1038/nrendo.2015.128
Chen, B., Teh, B., Sun, C., Hu, S., Lu, X., Boland, W., et al. (2016). Biodiversity and activity of the gut microbiota across the life history of the insect herbivore Spodoptera littoralis. Sci. Rep. 6:29505. doi: 10.1038/srep29505
Cheng, C., Wickham, J. D., Chen, L., Xu, D., Lu, M., and Sun, J. (2018). Bacterial microbiota protect an invasive bark beetle from a pine defensive compound. Microbiome 6:132. doi: 10.1186/s40168-018-0518-0
Dawadi, B., Wang, X., Xiao, R., Muhammad, A., Hou, Y., and Shi, Z. (2018). PGRP-LB homolog acts as a negative modulator of immunity in maintaining the gut-microbe symbiosis of red palm weevil, Rhynchophorus ferrugineus Olivier. Dev. Comp. Immunol. 86, 65–77. doi: 10.1016/j.dci.2018.04.021
Donohoe, R., Garge, N., Zhang, X., Sun, W., O’Connell, T. M., Bunger, M. K., et al. (2011). The microbiome and butyrate regulate energy metabolism and autophagy in the mammalian colon. Cell Metabol. 13, 517–526. doi: 10.1016/j.cmet.2011.02.018
Drissi, F., Merhej, V., Angelakis, E., El Kaoutari, A., Carrière, F., Henrissat, B., et al. (2014). Comparative genomics analysis of Lactobacillus species associated with weight gain or weight protection. Nutr. Diabetes 4:e109. doi: 10.1038/nutd.2014.6
Erkosar, B., Storelli, G., Mitchell, M., Bozonnet, L., Bozonnet, N., and Leulier, F. (2015). Pathogen virulence impedes mutualist-mediated enhancement of host juvenile growth via inhibition of protein digestion. Cell Host Microbe 18, 445–455. doi: 10.1016/j.chom.2015.09.001
Fei, N., and Zhao, L. (2013). An opportunistic pathogen isolated from the gut of an obese human causes obesity in germfree mice. ISME J. 7, 880–884. doi: 10.1038/ismej.2012.153
Fellows, R., Denizot, J., Stellato, C., Cuomo, A., Jain, P., Stoyanova, E., et al. (2018). Microbiota derived short chain fatty acids promote histone crotonylation in the colon through histone deacetylases. Nat. Commun. 9:105. doi: 10.1038/s41467-017-02651-5
Ge, X. Z., He, S. Y., Wang, T., Yan, W., and Zong, S. X. (2016). Potential distribution predicted for Rhynchophorus ferrugineus in China under different climate warming scenarios. PLoS One 10:e141111. doi: 10.1371/journal.pone.0141111
Giblin-Davis, R. M., Faleiro, J. R., Jacas, J. A., Penã, J. E., and Vidyasagar, P. (2013). “Biology and management of the Red Palm Weevil, Rhynchophorus ferrugineus,” in Potential Invasive Pests of Agricultural Crops, ed. J. E. Penã (Gainesville, FL: University of Florida), 1–34. doi: 10.1079/9781845938291.0001
Jia, S., Zhang, X., Zhan, G., Yin, A., Zhang, S., Li, F., et al. (2013). Seasonally variable intestinal metagenomes of the red palm weevil (Rhynchophorus ferrugineus). Environ. Microbiol. 15, 3020–3029. doi: 10.1111/1462-2920.12262
Johnston, C. S., Steplewska, I., Long, C. A., Harris, L. N., and Ryals, R. H. (2010). Examination of the antiglycemic properties of vinegar in healthy adults. Ann. Nutr. Metab. 56, 74–79. doi: 10.1159/000272133
Kamareddine, L., Robins, W. P., Berkey, C. D., Mekalanos, J. J., and Watnick, P. I. (2018). The Drosophila immune deficiency pathway modulates enteroendocrine function and host metabolism. Cell Metab. 28, 1–14. doi: 10.1016/j.cmet.2018.05.026
Kietz, C., Pollari, V., and Meinander, A. (2018). Generating germ-free Drosophila to study gut-microbe interactions: protocol to rear Drosophila under axenic conditions. Curr. Prot. Toxicol. doi: 10.1002/cptx.52 [Epub ahead of print].
Koh, A., Vadder, F. D., Kovatcheva-Datchary, P., and Bäckhed, F. (2016). From dietary fiber to host physiology: short-chain fatty acids as key bacterial metabolites. Cell 165, 1332–1345. doi: 10.1016/j.cell.2016.05.041
Kolye, M. L., Veloz, M., Judd, A. M., Wong, A. C., Newell, P. D., Douglas, A. E., et al. (2016). Rearing the fruitfly Drosophila melanogaster under axenic and gnotobiotic conditions. J. Vis. Exp. 113:e54219. doi: 10.3791/54219
Krautkramer, K. A., Kreznar, J. H., Romano, K. A., Vivas, E. I., Barrett-Wilt, G. A., Rabaglia, M. E., et al. (2016). Diet-microbiota interactions mediate global epigenetic programming in multiple host tissues. Mol. Cell. 64, 982–992. doi: 10.1016/j.molcel.2016.10.025
Lee, W., and Hase, K. (2014). Gut microbiota-generated metabolites in animal health and disease. Nat. Chem. Biol. 10, 416–424. doi: 10.1038/nchembio.1535
Matos, R. C., Schwarzer, M., Gervais, H., Courtin, P., Joncour, P., Gillet, B., et al. (2017). D-Alanylation of teichoic acids contributes to Lactobacillus plantarum-mediated Drosophila growth during chronic undernutrition. Nat. Microbiol. 2, 1635–1647. doi: 10.1038/s41564-017-0038-x
Matzkin, L. M., Johnson, S., Paight, C., Bozinovic, G., and Markow, T. A. (2011). Dietary protein and sugar differentially affect development and metabolic pools in ecologically diverse Drosophila. J. Nutr. 141, 1127–1133. doi: 10.3945/jn.111.138438
Miyamoto, J., Hasegawa, S., Kasubuchi, M., Ichimura, A., Nakajima, A., and Kimura, I. (2016). Nutritional signaling via free fatty acid receptors. Int. J. Mol. Sci. 17:450. doi: 10.3390/ijms17040450
Montagna, M., Chouaia, B., Mazza, G., Prosdocimi, E. M., Crotti, E., Mereghetti, V., et al. (2015). Effects of the diet on the microbiota of the red palm weevil (Coleoptera: Dryophthoridae). PLoS One 10:e0117439. doi: 10.1371/journal.pone.0117439
Morales-Jiménez, L., Zuniga, G., Villa-Tanaca, L., and Hernandez-Rodriguez, C. (2009). Bacterial community and nitrogen fixation in the red turpentine beetle, Dendroctonus valens LeConte (Coleoptera: Curculionidae: Scolytinae). Microb. Ecol. 58, 879–891. doi: 10.1007/s00248-009-9548-2
Muhammad, A., Fang, Y., Hou, Y., and Shi, Z. (2017). The gut entomotype of red palm weevil Rhynchophorus ferrugineus Olivier (Coleoptera: Dryophthoridae) and their effect on host nutrition metabolism. Front. Microbiol. 8:2291. doi: 10.3389/fmicb.2017.02291
Nagnan, P., Cain, A. H., and Rochat, D. (1992). Extraction et identification des composés volatils de la sève de palmier à huìle fermentée (vin de palme) attractifs potentiels pour le charancon du palmier. Oléagineux 47, 135–142.
Newell, P. D., and Douglas, A. E. (2014). Interspecies interactions determine the impact of the gut microbiota on nutrient allocation in Drosophila melanogaster. Appl. Environ. Microbiol. 80, 788–796. doi: 10.1128/AEM.02742-13
Nicholson, J. K., Holmes, E., Kinross, J., Burcelin, R., Gibson, G., Jia, W., et al. (2012). Host-gut microbiota metabolic interactions. Science 336, 1262–1268. doi: 10.1126/science.1223813
Passerini, D., Coddeville, M., Le Bourgeois, P., Loubière, P., Ritzenthaler, P., Fontagné-Faucher, C., et al. (2013). The carbohydrate metabolism signature of Lactococcus lactis strain A12 reveals its sourdough ecosystem origin. Appl. Environ. Microbiol. 79, 5844–5852. doi: 10.1128/AEM.01560-13
Perpetuini, G., Scornec, H., Tofalo, R., Serror, P., Schirone, M., Suzzi, G., et al. (2013). Identification of critical genes for growth in olive brine by transposon mutagenesis of Lactobacillus pentosus C11. Appl. Environ. Microbiol. 79, 4568–4575. doi: 10.1128/AEM.01159-13
Pu, Y. C., and Hou, Y. M. (2016). Isolation and identification of bacterial strains with insecticidal activities from Rhynchophorus ferrugineus Oliver (Coleoptera: Curculionidae). J. Appl. Entomol. 140, 617–626. doi: 10.1111/jen.12293
Raymann, K., and Moran, N. A. (2018). The role of the gut microbiome in health and disease of adult honey bee workers. Curr. Opin. Insect. Sci. 26, 97–104. doi: 10.1016/j.cois.2018.02.012
Ridley, E. V., Wong, A. C.-N., Westmiller, S., and Douglas, A. E. (2012). Impact of the resident microbiota on the nutritional phenotype of Drosophila melanogaster. PLoS One 7:e36765. doi: 10.1371/journal.pone.0036765
Sabat, D., Johnson, E. M., Abhinay, A., Jayabalan, R., and Mishra, M. (2015). A protocol to generate germ free Drosophila for microbial interaction studies. Adv. Tech. Biol. Med. S1:001. doi: 10.4172/2379-1764.S1-001
Sharon, G., Segal, D., Zilber-Rosenberg, I., and Rosenberg, E. (2011). Symbiotic bacteria are responsible for diet-induced mating preference in Drosophila melanogaster, providing support for the hologenome concept of evolution. Gut Microbes 2, 190–192. doi: 10.4161/gmic.2.3.16103
Shi, Z., Lin, Y., and Hou, Y. (2014). Mother-derived trans-generational immune priming in the red palm weevil, Rhynchophorus ferrugineus Olivier (Coleoptera, Dryophthoridae). Bull. Eentomol. Res. 104, 742–750. doi: 10.1017/S0007485314000583
Shi, Z., Wang, L., and Zhang, H. (2012). Low diversity bacterial community and the trapping activity of metabolites from cultivable bacteria species in the female reproductive system of the oriental fruit fly, Bactrocera dorsalis Hendel (Diptera: Tephritidae). Int. J. Mol. Sci. 13, 6266–6278. doi: 10.3390/ijms13056266
Shin, S. C., Kim, S. H., You, H., Kim, B., Kim, A. C., Lee, K. A., et al. (2011). Drosophila microbiome modulates host developmental and metabolic homeostasis via insulin signaling. Science 334, 670–674. doi: 10.1126/science.1212782
Storelli, G., Defaye, A., Erkosar, B., Hols, P., Royet, J., and Leulier, F. (2011). Lactobacillus plantarum promotes Drosophila systemic growth by modulating hormonal signals through TOR-dependent nutrient sensing. Cell Metabol. 14, 403–414. doi: 10.1016/j.cmet.2011.07.012
Storelli, G., and Leulier, F. (2016). The role of the microbial environment in Drosophila post-embryonic development. Dev. Comp. Immunol. 64, 39–52. doi: 10.1016/j.dci.2016.01.017
Storelli, G., Strigini, M., Grenier, T., Bozonnet, L., Schwarzer, M., Daniel, C., et al. (2018). Drosophila perpetuates nutritional mutualism by promoting the fitness of its intestinal symbiont Lactobacillus plantarum. Cell Metabol. 27, 362–377. doi: 10.1016/j.cmet.2017.11.011
Strigini, M., and Leulier, F. (2016). The role of the microbial environment in Drosophila post-embryomic development. Dev. Comp. Immunol. 64, 39–52. doi: 10.1016/j.dci.2016.01.017
Tagliavia, M., Messina, E., Manachini, B., Cappello, S., and Quatrini, P. (2014). The gut microbiota of larvae of Rhynchophorus ferrugineus Oliver (Coleoptera: Curculionidae). BMC Microbiol. 14:e136. doi: 10.1186/1471-2180-14-136
Venu, I., Durisko, Z., Xu, J., and Dukas, R. (2014). Social attraction mediated by fruit flies’ microbiome. J. Exp. Biol. 217, 1346–1352. doi: 10.1242/jeb.099648
Wang, G. H., Zhang, X., Hou, Y. M., and Tang, B. Z. (2015). Analysis of the population genetic structure of Rhynchophorus ferrugineus in Fujian, China, revealed by microsatellite loci and mitochondrial COI sequences. Entomol. Exp. Appl. 155, 28–38. doi: 10.1111/eea.12282
Wong, A. C., Dobson, A. J., and Douglas, A. E. (2014). Gut microbiota dictates the metabolic response of Drosophila to diet. J. Exp. Biol. 217, 1894–1901. doi: 10.1242/jeb.101725
Wong, A. C., Wang, Q., Morimoto, J., Senior, A. M., Lihoreau, M., Neely, G. G., et al. (2017). Gut microbiota modifies olfactory-guided microbial preferences and foraging decisions in Drosophila. Curr. Biol. 27, 1–8. doi: 10.1016/j.cub.2017.07.022
Xia, X., Gurr, G. M., Vasseur, L., Zheng, D., Zhong, H., Qin, B., et al. (2017). Metagenomic sequencing of diamondback moth gut microbiome unveils key holobiont adaptations for herbivory. Front. Microbiol. 8:663. doi: 10.3389/fmicb.2017.00663
Xia, X., Zheng, D., Zhong, H., Qin, B., Gur, G. M., Vasseur, L., et al. (2013). DNA sequencing reveals the midgut microbiota of Diamondback moth, Plutella xylostella L. and a possible relationship with insecticide resistance. PLoS One 8:e68852. doi: 10.1371/journal.pone.0068852
Xiang, H., Wei, G., Jia, S., Huang, J., Miao, X., Zhou, Z., et al. (2006). Microbial communities in the larval midgut of laboratory and field populations of cotton bollworm Helicoverpa armigera. Can. J. Microbiol. 52, 1085–1092. doi: 10.1139/w06-064
Xiao, R., Wang, X., Xie, E., Ji, T., Li, X., Muhammad, A., et al. (2019). An IMD-like pathway mediates the intestinal immunity to modulate the homeostasis of gut microbiota in Rhynchophorus ferrugineus Olivier (Coleoptera: Dryophthoridae). Dev. Comp. Immunol. 97, 20–27. doi: 10.1016/j.dci.2019.03.013
Zhang, R. Z., Ren, L., Sun, J. H., Wu, J., and Zeng, R. (2003). Morphological differences of the coconut pest insect, Rhynchophorus ferrugineus (Olivier) and its related species (Coleoptera: Curculionidae). Chin. For. Pest Dis. 22, 3–6. doi: 10.1673/031.013.9101
Zheng, H., Powell, J. E., Steele, M. I., Dietrich, C., and Moran, N. A. (2017). Honeybee gut microbiota promotes host weight gain via bacterial metabolism and hormonal signaling. Proc. Natl. Acad. Sci. U.S.A. 114, 4775–4780. doi: 10.1073/pnas.1701819114
Zhou, F., Xu, L., Wang, S., Wang, B., Lou, Q., Lu, M., et al. (2017). Bacterial volatile ammonia regulates the consumption sequence of D-pinitol and D-glucose in a fungus associated with an invasive bark beetle. ISME J. 11, 2809–2820. doi: 10.1038/ismej.2017.131
Keywords: Rhynchophorus ferrugineus, gut microbiota, insect symbiosis, symbiotic invasion, nutrition metabolism, germ-free larvae
Citation: Habineza P, Muhammad A, Ji T, Xiao R, Yin X, Hou Y and Shi Z (2019) The Promoting Effect of Gut Microbiota on Growth and Development of Red Palm Weevil, Rhynchophorus ferrugineus (Olivier) (Coleoptera: Dryophthoridae) by Modulating Its Nutritional Metabolism. Front. Microbiol. 10:1212. doi: 10.3389/fmicb.2019.01212
Received: 07 January 2019; Accepted: 14 May 2019;
Published: 29 May 2019.
Edited by:
David William Waite, The University of Auckland, New ZealandReviewed by:
Daniel B. Schwab, Indiana University Bloomington, United StatesBessem Chouaia, Università Ca’ Foscari, Italy
Copyright © 2019 Habineza, Muhammad, Ji, Xiao, Yin, Hou and Shi. This is an open-access article distributed under the terms of the Creative Commons Attribution License (CC BY). The use, distribution or reproduction in other forums is permitted, provided the original author(s) and the copyright owner(s) are credited and that the original publication in this journal is cited, in accordance with accepted academic practice. No use, distribution or reproduction is permitted which does not comply with these terms.
*Correspondence: Youming Hou, eW1ob3VAZmFmdS5lZHUuY24=; Zhanghong Shi, c2hpemhAZmFmdS5lZHUuY24=
†These authors have contributed equally to this work