- 1Institute for Food Safety and Hygiene, Vetsuisse Faculty, University of Zürich, Zurich, Switzerland
- 2Agroscope, Bern, Switzerland
A combination of phenotype microarrays, targeted stress resistance and virulence assays and comparative genome analysis was used to compare a set of Listeria monocytogenes strains including those involved in previous Swiss foodborne listeriosis outbreaks. Despite being highly syntenic in gene content these strains showed significant phenotypic variation in utilization of different carbon (C)-sources as well as in resistance of osmotic and pH stress conditions that are relevant to host and food associated environments. An outbreak strain from the 2005 Swiss Tomme cheese listeriosis outbreak (Lm3163) showed the highest versatility in C-sources utilized whereas the strain responsible for the 1983 to 1987 Vacherin Montd’or cheese listeriosis outbreak (LL195) showed the highest tolerance to both osmotic and pH stress conditions among the examined strains. Inclusion of L-norvaline led to enhanced resistance of acidic stress in all the examined strains and there were strain–strain-specific differences observed in the ability of other amino acids and urea to enhance acid stress resistance in L. monocytogenes. A strain dependent inhibition pattern was also observed upon inclusion of β-phenylethylamine under alkaline stress conditions. In targeted phenotypic analysis the strain-specific differences in salt stress tolerance uncovered in phenotypic microarrays were corroborated and variations in host cell invasion and virulence among the examined strains were also revealed. Outbreak associated strains representing lineage I serotype 4b showed superior pathogenicity in a zebrafish infection model whilst Lm3163 a lineage II serotype 1/2a outbreak strain demonstrated the highest cellular invasion capacity amongst the tested strains. A genome wide sequence comparison of the strains only revealed few genetic differences between the strains suggesting that variations in gene regulation and expression are largely responsible for the phenotypic differences revealed among the examined strains. Our results have generated data that provides a potential basis for the future design of improved Listeria specific media to enhance routine detection and isolation of this pathogen as well as provide knowledge for developing novel methods for its control in food.
Introduction
Listeria monocytogenes is an important foodborne pathogen that accounts for serious public health problems and food safety challenges since it causes severe clinical illnesses and high mortality in vulnerable human populations (European Food Safety Authority [EFSA], 2017; Centers for Disease Control [CDC], 2018; Radoshevich and Cossart, 2018). In high risk groups including young and old people as well as pregnant women infections can manifest as life-threatening meningitis, septicemia, and feto-maternal complications (European Food Safety Authority [EFSA], 2017; Radoshevich and Cossart, 2018). L. monocytogenes is a genetically diverse bacterial species that is subdivided into thirteen serotypes, four main evolutionary genetic lineages and numerous MLST clones (Orsi et al., 2011; Haase et al., 2014; Maury et al., 2016). All L. monocytogenes strains are presumed equally virulent although the molecular epidemiological evidence gathered to date suggests otherwise. A variable distribution of L. monocytogenes genotypes and serological subtypes in food products and processing environments as well as among human and animal clinical listeriosis cases has been reported (Liu, 2008; Orsi et al., 2011; Maury et al., 2016; Moura et al., 2016).
Natural stress resistance and virulence capacity both contribute to current challenges posed by L. monocytogenes to public health and food safety (Gandhi and Chikindas, 2007; Toledo-Arana et al., 2009; Allerberger and Wagner, 2010; Soni et al., 2011; Kocaman and Sarımehmetoğlu, 2016; European Food Safety Authority [EFSA], 2017; Radoshevich and Cossart, 2018). In addition, the ability of this pathogen to efficiently exploit various nutrient sources in food and infected host associated environments is crucial for its survival and growth in such environments (Deutscher et al., 2014). L. monocytogenes has evolved various systems necessary for nutrient acquisition and utilization, stress adaption and virulence responses that allow for stress survival and transmission along the food chain as well as subsequent host infection and pathogenicity processes (Nadon et al., 2002; Desvaux and Hebraud, 2006; Wilson et al., 2006; Toledo-Arana et al., 2009; Allerberger and Wagner, 2010; Soni et al., 2011; Deutscher et al., 2014; Kocaman and Sarımehmetoğlu, 2016; Radoshevich and Cossart, 2018). Such evolution has generated natural populations of this pathogen that are phenotypically heterogeneous. Nutrient utilization and stress resistance mechanisms documented in this bacterium include numerous enzymes, transporter systems and gene expression regulating proteins (Schmid et al., 2009; Toledo-Arana et al., 2009; Loepfe et al., 2010; Scharer et al., 2013; NicAogáin and O’Byrne, 2016; Chen et al., 2017). The virulence strategies employed in this bacterium involves various proteins that are primarily regulated through the transcription regulator PrfA (positive regulatory factor A), (de las Heras et al., 2011; Radoshevich and Cossart, 2018). PrfA activity is controlled by several environmental signals at the transcriptional and post-transcriptional level, which include temperature and the presence or absence of efficiently metabolized C-sources transported via the phosphoenolpyruvate (PEP): carbohydrate phosphotransferase system (PTS) (Johansson et al., 2002; Joseph et al., 2008). The uptake and metabolism of these carbohydrates leads to strong inhibition of PrfA activity without affecting prfA gene expression (Joseph et al., 2008). PrfA expression is also controlled through stress response regulatory proteins such as Sigma B and Csps (Ollinger et al., 2009; Scharer et al., 2013; Eshwar et al., 2017). Therefore, carbon source utilization and stress resistance profiles could be correlated to virulence. As such efforts to determine the global phenome of L. monocytogenes strains are important since virulence seems intricately connected to nutrient utilization and stress tolerance.
Several listeriosis outbreaks have been documented in Switzerland to date, which besides causing severe illnesses and claiming lives of several people have also been responsible for significant food safety problems and economic losses to the food industry (Bille, 1990; Bula et al., 1995; Bille et al., 2006; Schmid and Baumgartner, 2012; Hachler et al., 2013; Althaus et al., 2014; Ebner et al., 2015; Stephan et al., 2015; Althaus et al., 2017; Meier et al., 2017). Our current understanding of nutrient exploitation and stress resistance within the host and food-associated environments in outbreak-associated L. monocytogenes strains is still limited. A detailed study of metabolism and stress resilience in such strains might, however, provide more clues on the roles played by established and novel physiological and molecular response mechanisms of this bacterium in facilitating colonization, survival and proliferation in food and host associated environments. In this study, a selection of L. monocytogenes isolates that includes strains linked to Swiss listeriosis outbreaks and associated food sources were compared with respect to metabolism of different C-sources as well as pH and osmotic stress resistance profiles. A potential association between phenotypic diversity in carbon metabolism and stress resistance with virulence and the genome in such strains was also examined.
Materials and Methods
Ethics Statement
This study was carried out in accordance with the principles and recommendations of the “Ordinance on laboratory animal husbandry, the production of genetically modified animals and the methods of animal experimentation; Animal Experimentation Ordinance” (SR 455.163, April 12, 2010), Swiss Federal Food Safety and Veterinary Office (FSVO/BLV). The maximum age reached by the embryos during experimentation was 5 dpf for which no license was required from the cantonal veterinary office in Switzerland, since embryos had not yet reached free feeding stage. Husbandry and breeding of the adult zebrafishes was performed under the supervision of Prof. Stephan Neuhauss, Institute for Molecular Life Sciences, University of Zürich, Zurich, Switzerland. All animal protocols used were in compliance with internationally recognized and with Swiss legal ethical guidelines for the use of fish in biomedical research and experiments were approved by the local authorities (Veterinäramt Zürich Tierhaltungsnummer 150).
Overview of the Analyzed Strains
A set of strains that includes isolates from past Swiss listeriosis outbreaks as well as some food derived isolates and the L. monocytogenes EGDe reference strain was analyzed (Table 1). Outbreak strains included were responsible for previous Swiss Vacherin Montd’or cheese (1983–1987; LL195), Tomme cheese (2005; Lm3163 and Lm3136), ready-to-eat salad (2013–2014; N2306), and meat pâté (2016; N16-0044) listeriosis outbreaks (Bula et al., 1995; Bille et al., 2006; Stephan et al., 2015; Althaus et al., 2017). Two strains randomly isolated from Swiss milk products sampled in 2011 (N14-0435) and 2014 (N11-1515) were included as background strains (Ebner et al., 2015). Strains examined represent different L. monocytogenes genetic lineages I and II as well as different MLST clonal complexes and sequence types. LL195, N2306 and N16-0044 are lineage I, serotype 4b strains whist Lm3163 and Lm3136 are lineage II, serotype 1/2a strains. N14-0435 and N11-1515 included as background strains belong to lineage I, serotype 1/2b and lineage II, serotype 1/2a respectively. In addition, L. monocytogenes EGDe a lineage II, serotype 1/2a strain was included in the study as a reference strain and L. innocua J5051 was included as a negative control in some of the experiments (Guldimann et al., 2015).
Bacterial Culture and Growth Conditions
All strains were stored at -80°C in brain heart infusion medium (BHI, Oxoid, United Kingdom) supplemented with 20% glycerol. To prepare inoculum the frozen stock of each strain was streaked out on blood agar or BHI agar plates and incubated overnight at 37°C. Single colonies picked from each plate were then pre-cultured twice in BHI broth (37°C, 150 rpm) for 16 h. Secondary stationary phase cultures generated in this way were subsequently used in experiments unless otherwise stated.
Phenotype Microarray Analysis
Biolog phenotype microarrays (PMs) were used to compare metabolic and stress tolerance profiles among the strains1 (Bochner, 2009). The carbon source (C-source) utilization (PM01 and PM02) as well as osmotic and pH stress (PM09 and PM10) resistance phenotypic profiles were analyzed. PM experiments were done in accordance with standard Biolog Inc. protocols with a few modifications (Fox and Jordan, 2014; see text footnote 1). Bacteria from glycerol stocks stored at -80°C were grown overnight at 37°C on BHI agar. Single colonies of the bacteria grown on BHI agar were sub-cultured on BHI agar plates and incubated overnight at 37°C. A 81% transmittance cell suspension in Biolog solution IF-0a was then prepared by re-suspending the bacteria grown on BHI agar plates. This suspension was then diluted with Biolog additive solution for the specific plate at manufacturers prescribed ratio. One hundred microliter of the final mixture was then transferred to each of respective PM plate wells. The plates were incubated at 37°C with bacterial active metabolism being read every 15 min for 24 h using Omilog reader (Biolog, Hayward, CA, United States), a system that uses respiration as a universal reporter of active metabolism. Each experiment was performed in duplicate.
Growth Evaluation
Secondary stationary phase stage BHI cultures prepared as described above from each L. monocytogenes strain were diluted in BHI to 105 CFU/ml. To assess growth under NaCl stress, 100 μl volumes of BHI media supplemented with 0 and 16% NaCl were added in triplicate to wells of a 96-well microplate to which 100 μl of the different L. monocytogenes strains at 105 CFU/ml had been added. To compare growth in Eagle’s minimum essential medium (MEM), 20 μl of BHI secondary culture from each strain diluted to 106 CFU/ml was added in triplicate to respective wells containing 180 μl of MEM. Cultures were incubated for 24 h at 37°C with shaking in a Synergy HT OD reader (BioTek Instruments, GmbH, Switzerland) and the OD600 was measured every 30 min. Maximal growth rates were determined from the OD600 growth data using the program DMFit (Baranyi and Roberts, 1994).
Hemolysis Assays
For hemolysis analysis, sterile filtered 1M dithiothreitol (DTT) (Sigma-Aldrich, Buch, Switzerland) treated supernatants collected from early stationary phase cultures were used. These were collected from cultures grown using overnight cultures to make 1:100 dilutions in pre-heated BHI and incubated at 37°C for 5 h. The cultures were first standardized to the same optical density using BHI and centrifuged to collect the supernatants. To activate the hemolysin, a 1:200 dilution of 1M DTT and each of the supernatant was made and incubated for 1 h at 37°C. One hundred microliter of a 2% washed human red blood cells PBS solution was pipetted to respective 96 well plate wells. Then 100 μl of the DTT activated supernatant was added. The bacterial supernatant and RBC mixture was incubated at 37°C for 40 min to allow hemolysis to occur. After which it was centrifuged for 5 min at 3,100 × g and then 100 μl of each lysate was transferred to a new 96 well plate. The degree of hemolysis was assessed by measuring absorbance of the lysate at 420 nm using the Synergy HT OD reader (BioTek Instruments, GmbH, Switzerland). The internal control strains L. monocytogenes EGDe and L. innocua JF5051 were included in all experiments.
Cell Invasion Assays
Cell invasion assays were performed in the human enterocyte-like Caco-2 (ATCC® HTB-37TM) cell line. Cells were grown to confluence in a 96-well cell culture plate overnight at 37°C, 5% CO2 in Eagle’s MEM, (Life Technologies, Switzerland) supplemented with 20% fetal bovine serum. The monolayers were washed with pre-warmed PBS (37°C) and then infected with L. monocytogenes strains at a multiplicity of infection (MOI) of 0.01 in MEM. Following 30 min of incubation the medium was removed, then cells were washed with PBS and overlaid with MEM medium containing 0.01 mg/ml gentamicin and incubated for another 60 min at 37°C to kill extracellular bacteria. At the end of the incubation the cells were washed five times with 100 μl warm PBS and then 100 μl of 40 mg/ml saponin was added to lyse the cells. The lysate was serially diluted and cell count was done to determine the number of L. monocytogenes recovered in comparison to the number used to infect the Caco-2 cells. Reference strain L. monocytogenes EGDe and L. innocua JF5051 were included in all experiments as positive and negative controls, respectively.
Zebra Fish Microinjection Assays
Zebrafish husbandry and assays were performed as previous described (Eshwar et al., 2017) with a few modifications. The Danio rerio wik zebrafish line strains were used in this study. All experiments were performed with the approval (no. 216/2012) from the Veterinary Office, Public Health Department, Canton of Zurich (Switzerland). Bacteria for microinjection experiments were harvested from stationary phase BHI bacteria cultures by centrifugation and washing with DPBS, then standardized to the same CFU through plate counts and appropriate dilutions. Two-day post fertilization embryos were injected with approximately 500 CFU in 1–2 nl volume of a bacterial suspension in DPBS into the blood circulation via the caudal vein. The number of CFU injected was determined by direct microinjection of a DPBS droplet on agar plates and confirmed by disintegrating five embryos individually immediately after microinjection and plating the lysates on BHI agar. Post-infection embryos were placed into 24-well plates (one embryo per well) in 1 ml E3 medium per well, incubated at 28°C and observed for signs of disease and survival under a stereomicroscope twice a day. The number of dead larvae was determined visually based on the absence of a heartbeat.
Genome Analysis
Genomes of L. monocytogenes EGDe, LL195, Lm3136, Lm3163, N2306, and N16-0044 are available in GenBank under accession numbers NC003210, HF558398, CP013722, CP013723, CP011004, and CP035187, respectively (Glaser et al., 2001; Weinmaier et al., 2013; Tasara et al., 2015, 2016). Rapid Annotation Subsystem Technology (RAST) and Seed Viewer standard settings2 were used for genome annotation and comparisons. Progressive Mauve was used to align the genomes and to derive the coordinates for the positions of the single nucleotide polymorphisms (SNPs), insertions and deletions (InDels) (Darling et al., 2010). Genomes were correlated with PM data using the DuctApe software (Galardini et al., 2014). Only those genes described in the Kyoto Encyclopedia of Genes and Genomes (KEGG) database were considered. Genes found in all strains were described as “core,” and the others as “dispensable”: Dispensable genes were further divided into “accessory,” when a gene is present in at least two strains, and “unique,” when a gene is present in exactly one strain as previously described (Galardini et al., 2014). Genes possibly linked to phenotypic differences were searched and compared between the genomes in CLC genomics Workbench (Qiagen, Prismet, Denmark) and using BLASTn and BLASTp in the National Center for Biotechnology Information (NCBI) platform (blast.ncbi.nlm.nih.gov/Blast.cgi). Relatedness of the strains was assessed by SNP comparisons. SNPs were identified using parsnp within the harvest suite (Treangen et al., 2014) using standard settings and nucleotide fasta files as input. Each strain was used as a reference strain and compared to the other strains. The output files were converted to variant calling files using harvesttools and a SNP matrix was constructed by taking the sum of the variants compared to the reference strain. The SNP matrix was visualized in a heatmap using clustvis (Metsalu and Vilo, 2015). Genome compositions analyses were performed by comparing the protein coding sequences using the script get_homologues (Contreras-Moreira and Vinuesa, 2013). A pangenome was constructed by using get_homologues with the option “-t 0” to obtain all proteins, a cut off of E < 1e-05 for blast searches, and a 75% minimum alignment coverage. Both a cluster of orthologous groups (COG) and an orthologous Markov clustering (OMCL) based pangenome was calculated and only genes presence in both OMCL and COG based pangenome were considered in further pangenome analysis. A presence-absence matrix of the pangenome was produced using the script compare clusters (Contreras-Moreira and Vinuesa, 2013). The pangenome was annotated by selecting the first protein of a cluster and annotating that protein using EggNOG 4.5 (Huerta-Cepas et al., 2016). Virulence genes were identified using get_homologues and the protein database VFDB_SetA_pro from the virulence factor database (Chen et al., 2016) as the reference set. Settings were identical to the settings for the pangenome analyses. The VFDB_SetA_pro contains the experimentally verified virulence factors and was downloaded in November 2018.
Statistical Analyses
All experiments presented were performed independently at least three times unless stated otherwise. JMP software (Version 12.1.0, SAS Institute Inc., NC, United States) was used for statistical analysis of data. One-way ANOVA with post hoc Tukey HSD tests were used to assess statistical significance of differences relative to the reference strains as well as between the strains. P-values of <0.05 were considered to be statistically significant. For PM data analysis DuctApe and opm version 1.3.64 software’s were used as previously described (Galardini et al., 2014; Göker et al., 2016). Briefly, for opm based analysis the reference parameter was area under the curve. Whilst for DuctApe the parameter, activity index (AV), was calculated to rank kinetic curves, providing information about the ability to be metabolically active under a specific culture condition. The AV parameter was obtained through k-means clustering on maximum metabolism, area under the curve, average height, lag time, and slope, whilst for opm based analysis k-means clustering was based on area under the curve as previously described (Galardini et al., 2014; Göker et al., 2016). For each compound tested, the final result was expressed as the mean of two replicates. The bacterium was not able to grow under conditions were AV value was equal to zero, whilst it was able to grow under conditions were the AV value was higher than zero.
Results
Phenotypic Microarray-Based Comparison of the Studied Strains
Using the Biolog phenotypic microarrays, we compared C-source utilization (PM01 and PM02), osmolyte (PM09) and pH (PM10) sensitivity profiles in a set comprising previous listeriosis outbreak and food-associated L. monocytogenes strains isolated in Switzerland (Table 1). An overview provided in Figure 1 shows that there were strain-specific differences observed in growth/metabolism activity detected on various C-sources and under different osmolyte and pH stress conditions (Figure 1). The total number of C-sources that were metabolized among the strains ranged from 34 to 51. Interestingly, two strains Lm3136 and Lm3163, that were recovered during the same 2005 Swiss Tomme cheese listeriosis outbreak showed the least and highest numbers of C-sources, respectively, that were metabolized among the tested strains (Table 2). Serotype 4b strains LL195 and N16-0044, that were responsible for the 1983–1987 Vacherin Montd’or cheese and 2016 meat pâté associated listeriosis outbreaks in Switzerland, respectively, were the most stress tolerant with respect to both osmotic and pH stress among the tested strains (Figure 2A). Overall whilst the strain Lm3163 showed highest overall metabolic activity on C-sources, LL195 was the most metabolically active strain under salt and pH stress conditions (Figure 2B). Meanwhile out of the 190 tested C-sources, 31 could be metabolized by all strains whereas another 22 were metabolized by at least one strain (Supplementary Table S1). A comparison of the C-source utilization profiles to those of L. monocytogenes EGDe revealed a mixture of metabolic activity capacities among the outbreak strains compared to the reference strain. For example, whilst the EGDe strain did not metabolize D-tagatose both Tomme cheese outbreak associated strains, Lm3136 and Lm3163 could efficiently utilize this C-source (Table 3). Of the 31 C-sources utilized by all strains, 10 C-sources including glycerol, ribose, inosine, maltose, arbutin, and thymidine were metabolized at varying rates by the different strains (Supplementary Figure S1). Meanwhile there were genetic lineage specific trends observed regarding β-D-allose utilization, which was metabolized by all lineage II but not any of the lineage I strains tested (Table 3). Only a single amino acid was utilized as a carbon source by one of the tested strains. Lm3163 was the only strain among the examined strains that utilized L-threonine as a C-source (Table 3 and Supplementary Table S1). An overall attempt to either cluster the strains based on their C-source or the pH and osmotic stress tolerance profiles on the other hand showed that the strains clustered independent of isolation source, serotype and evolutionary genetic lineage (Figure 3 and Supplementary Figures S2A–D).
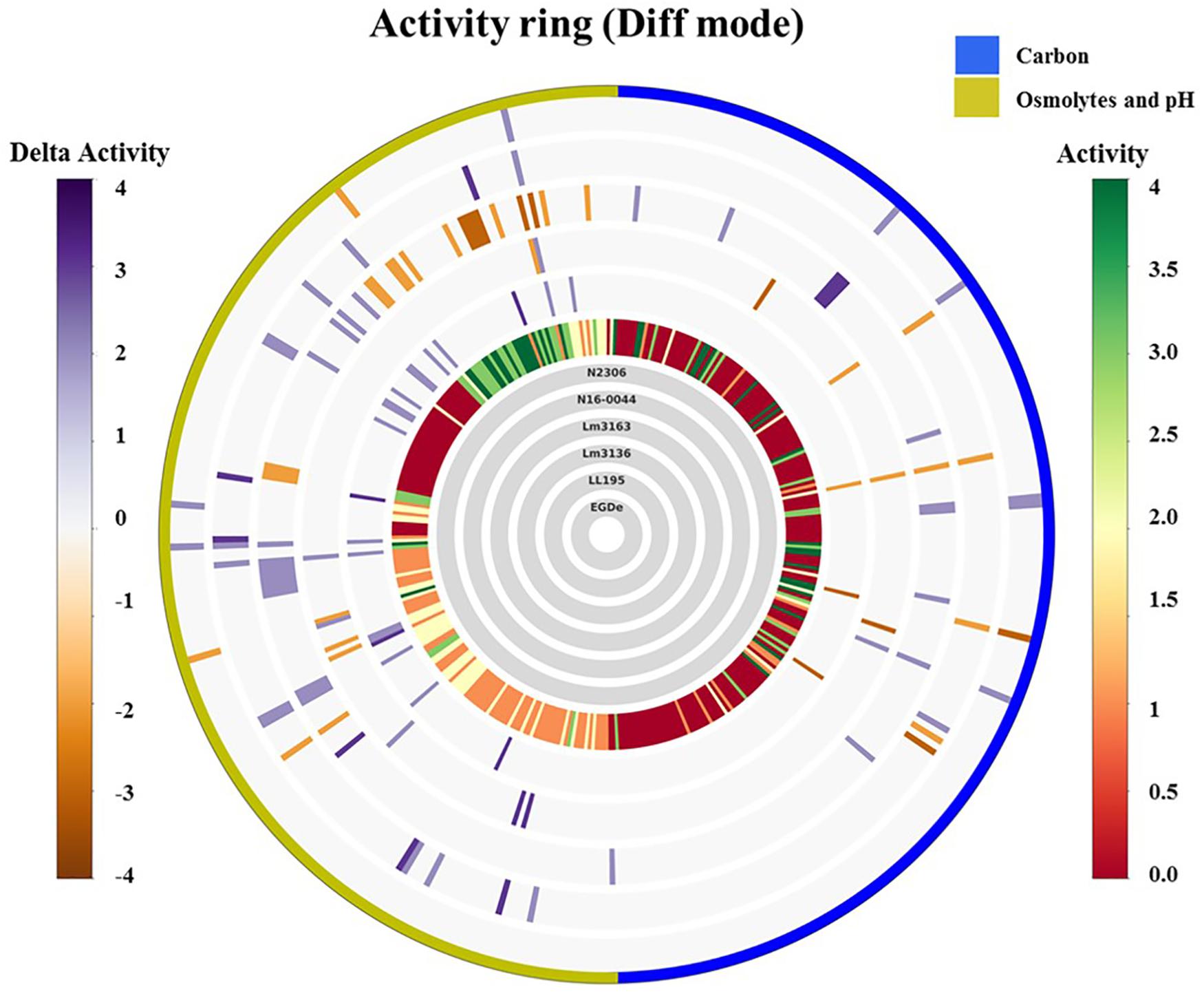
Figure 1. Overall growth/metabolic activity ring based on phenotype microarray comparison of the strains with respect to C-source utilization (PM01 and PM02) and stress (osmolytes and pH; PM09 and PM10) resistance: gray inner circles indicate the strains’ order; external circle indicates the PM categories. The activity index (AV) calculated for each strain and well is reported as color stripes going from red (AV = 0) to green (AV = 4). Delta activity: the difference with the AV value of the reference strain is reported when equal to or higher than 2 AV; gray is no difference; purple indicates a higher activity; orange color indicates a lower activity.
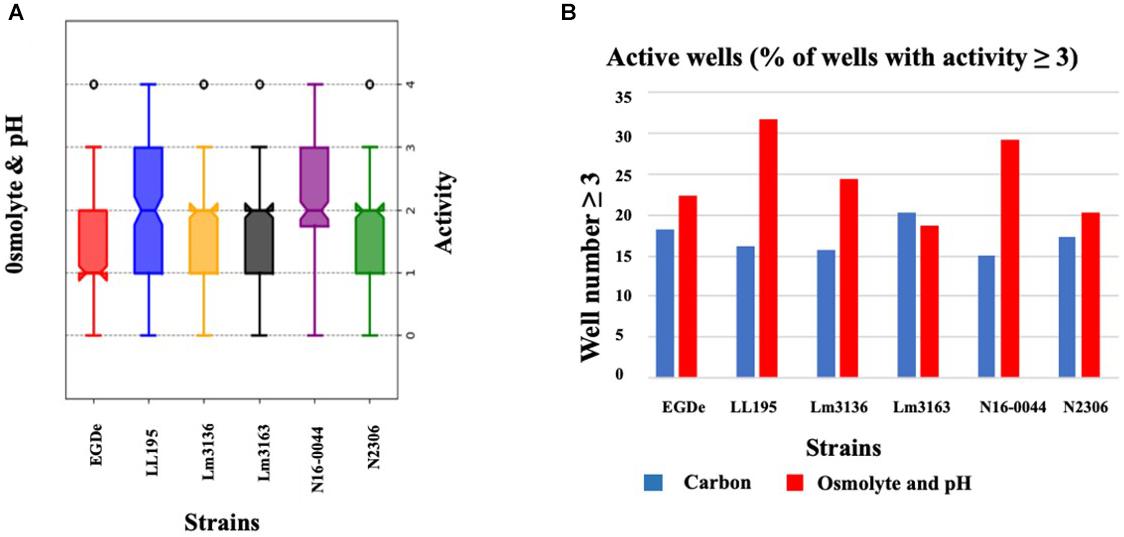
Figure 2. Variation of C-source utilization and stress tolerance profiles among L. monocytogenes strains. (A) Box-plot showing growth/metabolic activities observed among the study strains under pH and osmotic stress conditions. (B) Bar graph displaying the percentage of compounds under which each strain had an activity that equaled or was above 3 AV.
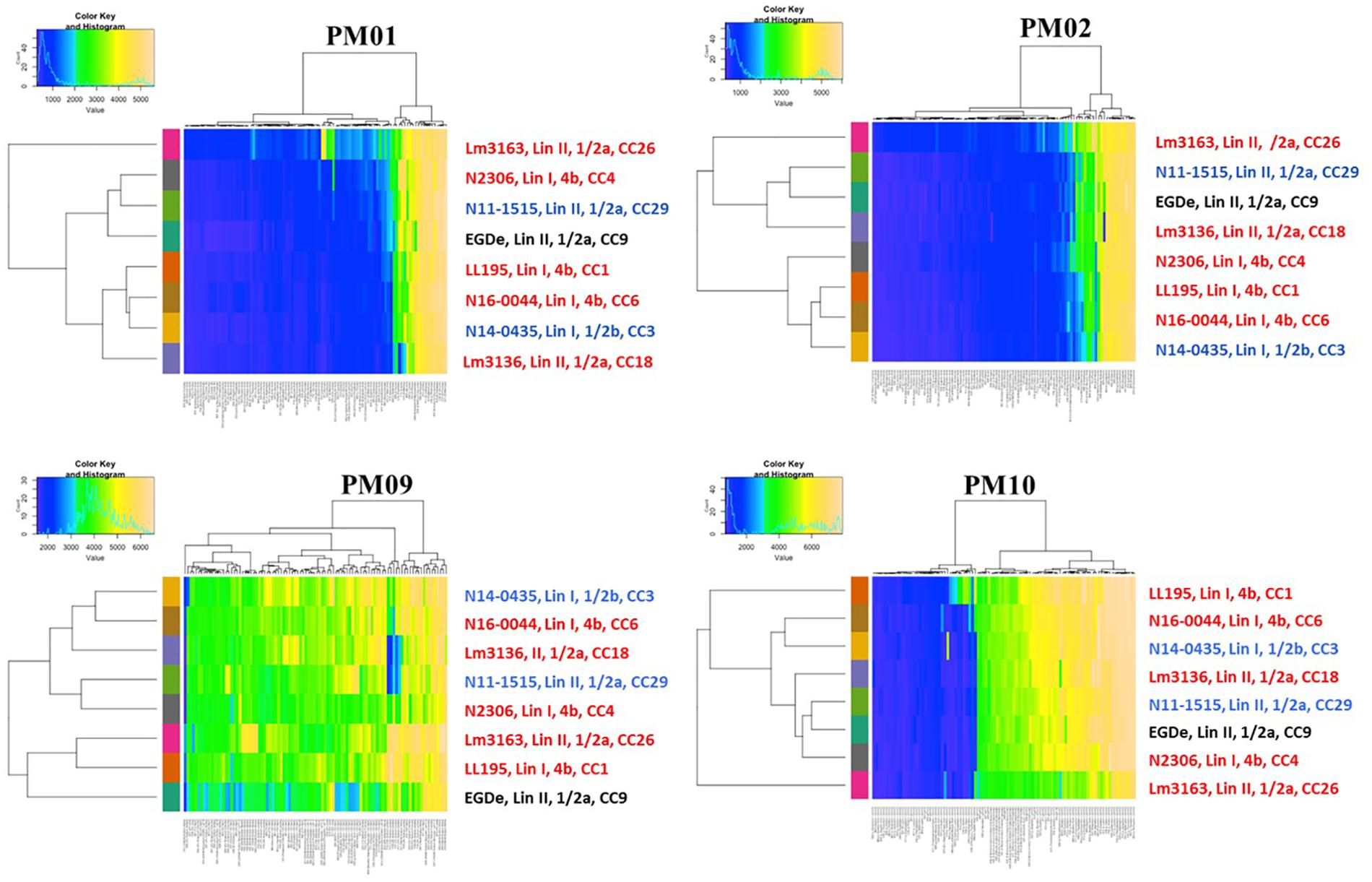
Figure 3. Heatmaps showing clustering of the strains based on PM01, PM02, PM09, and PM10 growth/metabolic activity results. Clinical listeriosis outbreak strains are shown in red, strains isolated from food during routine check are shown in blue and the L. monocytogenes EGDe reference strain is shown in black. Each of the colored bars identified on the x-axis represents the different PM test conditions applied and for detailed information on the reactions see Supplementary Figures S2A–D.
Outbreak Strains Vary in Metabolism of Host and Food Associated C-Sources
Strain-specific differences in the capacity to utilize some host and food relevant C-sources were also observed among the tested strains (Table 3). Intracellular C-sources nucleosides inosine and thymidine, as well as pyruvic acid and maltose were differentially metabolized by the strains. While Lm3163 was able to utilize all these C-sources, strain Lm3136 isolated from the same listeriosis outbreak was incapable of utilizing most such C-sources (Table 3). Furthermore, differences in metabolic activity on food relevant C-sources such as sucrose, lactose, pectin and D-tagatose were observed, with Lm3163 utilizing all such C-sources whereas most of the tested strains were unable to utilize them (Table 3). Strains Lm3163 and Lm3136 that were isolated from the 2005 Tomme cheese associated listeriosis outbreak were the only strains able to utilize D-tagatose. An ability of these two strains to metabolize a C-source that is often found in dairy products might be suggestive of their adaptation to dairy associated niches linked to milk and cheese.
Variation in Osmotic and pH Stress Sensitivity Is Observed Among Outbreak Strains
All the tested strains gave positive reactions on 92 of the 96 osmotic stress conditions tested on PM09 although they showed variable metabolic rates when exposed to higher concentrations of common food preservatives including NaCl and sodium lactate. At higher NaCl (8–10%) concentrations, the reference strain L. monocytogenes EGDe showed the lowest growth/metabolic activity compared to all the other strains (Figures 1, 2). In a validation of these PM observations a growth curve-based comparison showed that while the Swiss Vacherin Montd’or cheese listeriosis outbreak strain LL195 exhibits superior growth, L. monocytogenes EGDe was the most impaired strain during growth under salt stress (8% NaCl) in BHI (Figure 4). All tested strains were able to tolerate high concentrations of other common food preservative compounds such as sodium lactate and sodium benzoate (Table 4, Supplementary Table S2, and Supplementary Figure S3). Six strains showed growth/metabolic activity at the highest levels of sodium lactate (12%) and sodium nitrate (100 mM) tested. Strain Lm3136 involved in the Swiss Tomme cheese outbreak as well as N11-1515 isolated from milk in 2014, were the only strains inhibited by 11 and 12% sodium lactate, whereas other strains were not. At 80 mM sodium nitrate, the EGDe reference strain and the other Swiss Tomme cheese associated outbreak strain Lm3136 were inhibited, whilst all the other strains were metabolically active.
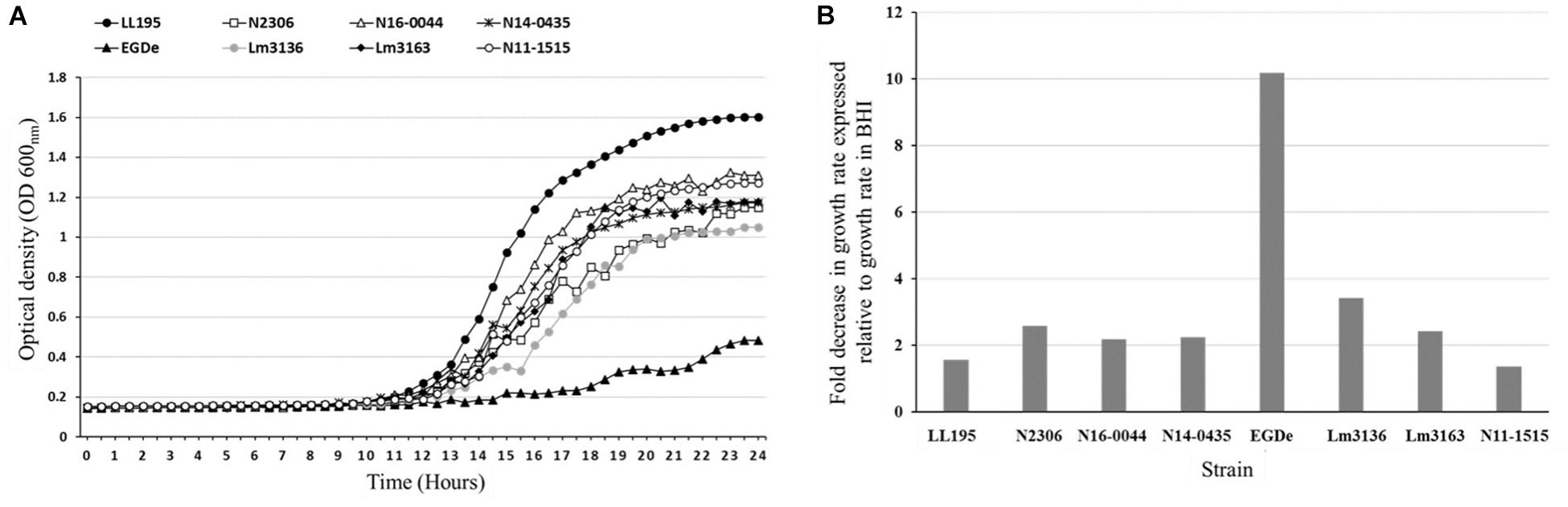
Figure 4. NaCl stress tolerance variation among the L. monocytogenes strains. Growth curves (A) and bar charts (B) showing growth kinetics and fold decrease in growth rate of the L. monocytogenes strains during growth in BHI under NaCl salt (8%) stress. LL195 and EGDe strains showed the highest and lowest tolerance to NaCl salt stress, respectively. The data presented represents three independent biological repeats.
Out of the 96 pH conditions tested on PM10 a positive growth/metabolic activity reaction for at least one strain was detected in 71 assay conditions. The Vacherin Montd’or cheese associated outbreak strain LL195 generated the highest number of positive reactions under the tested range of pH stress conditions (Figures 1, 2, Table 4, and Supplementary Table S3). While all the tested strains gave negative results for active growth/metabolic activity at pH 4.5 and below, the inclusion of the compound L-norvaline enhanced their acidic pH tolerance allowing all the tested strains to grow or become metabolically active at pH 4.5 (Table 4 and Supplementary Table S3). Supplementation with some amino acids gave positive growth/metabolic activity reactions under acid stress at pH 4.5 with some but not all the tested strains indicating the existence of strain-specific differences in the capacity to use some amino acids for acid stress tolerance. LL195, N16-0044, and Lm3163 were for example able to grow at pH 4.5 when supplemented with L-hydroxyproline while the rest of the strains could not. LL195 was the only strain showing active growth/metabolism at pH 4.5 in presence of urea indicating urease dependent acid stress tolerance activity in this strain. Meanwhile all strains gave positive growth/metabolism reactions at pH 5–10 but there were strain associated differences observed in metabolic rates under such conditions (Supplementary Table S3). At pH 9.5 there was an inhibition of Lm3136 and Lm3163 strains detected in presence of β-phenylethylamine while the rest of the tested strains were not affected. None of the amino acids altering L. monocytogenes pH sensitivity were directly utilized as C-sources on PM01 and PM02 in the examined strains suggesting that the protective effects of these amino acids might not involve their metabolism as C-sources. Overall, the two lineage I and serotype 4b outbreak strains LL195 (1983–1987 Vacherin Montd’or cheese outbreak) and N16-0044 (Meat pâté outbreak) showed the greatest tolerance to both pH and osmotic stress in comparison to all the other tested strains (Figures 1, 2). Furthermore, the studied strains were able to metabolize all the chromogenic compounds tested on PM10, suggesting that these substances could be used in chromogenic L. monocytogenes detection or isolation media.
Outbreak Associated Strains Vary in Cell Invasion and Zebrafish Virulence
In order to examine if C-source metabolism and stress resistance variations could be related to pathogenicity we compared virulence between the Swiss listeriosis outbreak strains and the reference strain L. monocytogenes EGDe. Listeriosis outbreak strains showed significantly higher (P < 0.05) cell invasion (29- to 504-fold) capacities compared to L. monocytogenes EGDe when examined using the Caco-2 cell-based infection model (Table 5). Notably Lm3163 displaying highest levels of metabolic activity and C-source substrate diversity also showed highest cellular invasion levels compared to all other tested strains (Table 5). Comparison of strain virulence in vivo in a zebrafish embryo-based infection model further revealed strain specific variation in virulence based on the rate and mortality levels achieved. Lineage I, serotype 4b outbreak strains (LL195, N16-0044, N2306) caused significantly higher mortality compared to the lineage II, serotype 1/2a outbreak strains (Lm3136 and Lm3163) and L. monocytogenes EGDe (Figure 5A). In contrast, strain Lm3163 despite exhibiting a more diverse C-source metabolic capacity and high cell invasion capacity caused the lowest mortality than all other tested strains in this infection model (Figure 5A). L. monocytogenes EGDe despite exhibiting the lowest cell invasion levels in Caco-2 cells induced the highest levels of zebrafish embryo mortality compared to the lineage II outbreak strains Lm3136 and Lm3163. A comparison of hemolysis among the strains, however, did not show significant differences in hemolytic activity among the examined strains (data not shown). This observation rules out that differences in expression of this virulence function as a reason for the strain-specific differences observed in both cell invasion and zebrafish virulence. A possible explanation for the high invasiveness observed for Lm3163 could in part be due to the fact that this strain due to its high and diverse C-source metabolic capacity might outgrow other strains during incubation in MEM prior to invasion and inside cells post invasion. A notion supported by the observation that this strain grows better than all the other tested strains in BHI and MEM (Figures 5B,C). Another interesting observation is that in both media this strain exhibits a biphasic growth profile typical of changes in utilization efficiency of available nutrients such as C-sources as growth incubation time progresses.
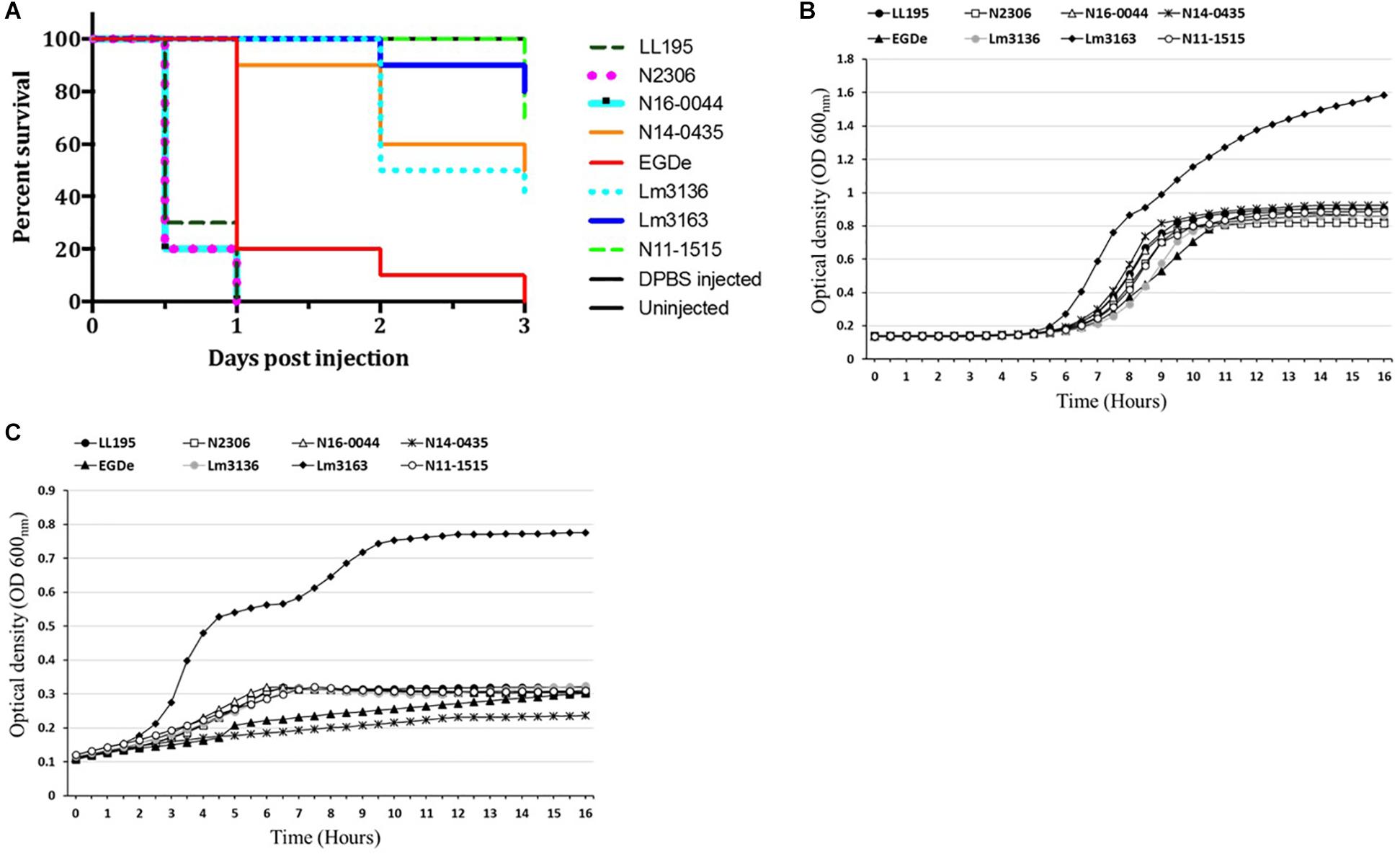
Figure 5. Strains vary in virulence and growth in BHI and MEM media. (A) Virulence was assessed using a zebrafish infection model. Zebrafish embryos (n = 10 per strain) infected (500 CFU) with different Listeria monocytogenes strains were monitored over 3 days. Zebra fish embryos more rapidly succumbed to serotype 4b compared to serotype 1/2a strains. Kinetic growth assays in (B) BHI and (C) MEM based on OD600 measurement showing varied growth of the examined L. monocytogenes strains with Lm3163 displaying the greatest growth capacity and biphasic growth in both media compared to the other strains.
Linking Phenotypic Variation to Genomic Differences Amongst the Listeriosis Outbreak Strains
A genome and phenome-based analysis linking variation in C-source metabolism phenotypes to the genomic information of the listeriosis outbreak strains was conducted using the program DuctApe. A comparison of the pangenome and metabolic pathway constructions revealed high conservation between genomes (76% conservation) and metabolic pathways in the examined outbreak strains and the L. monocytogenes EGDe reference strain (Figure 6 and Table 6). An observation suggesting that phenotypic variations observed between the outbreak strains in C-source metabolism phenotypes could be a function of minor differences affecting genes and metabolic pathways. A gene content comparison identified 81 lineage specific gene content differences that includes genes associated with transport, metabolism, transcription regulation, virulence, and cell envelope modification (Supplementary Table S4). One such gene content variation could be linked to D-allose metabolism differences detected between the genetic lineages. The genes of the lmo0734-0739 cassette encoding proteins involved in D-allose metabolism are found in genetic lineage II but not genetic lineage I listeriosis outbreak strains of our study (Supplementary Table S4). Consequently, all lineage I outbreak strains examined were unable to use D-allose as a C-source. Presence of numerous genes involved in the transport and metabolism of simple sugars, complex carbohydrates, amino acids and peptides was confirmed in the genomes of the examined strains (data not shown). In some cases, however, the number and composition of these nutrient transporters vary in a lineage and strain specific manner. A lineage specific trend in the distribution of several ATP-binding cassette (ABC transporters) and PTS transporter systems was detected (Supplementary Table S4). Notably one of such differences is associated with the lineage II specific D-allose metabolism as mentioned above. Overall a one-to-one assignment of nutrient and corresponding gene association in most cases was not always possible since the majority of C-sources tested have more than a single gene associated with their transport or metabolism. In another observation there were some C-sources that were metabolized but a complete pathway for their metabolism was not detected in the genome of the examined strains. The strain Lm3163 for example metabolized sucrose but no complete pathway for sucrose metabolism was detected in this strain based on the current genome annotations of L. monocytogenes EGDe applied as reference.
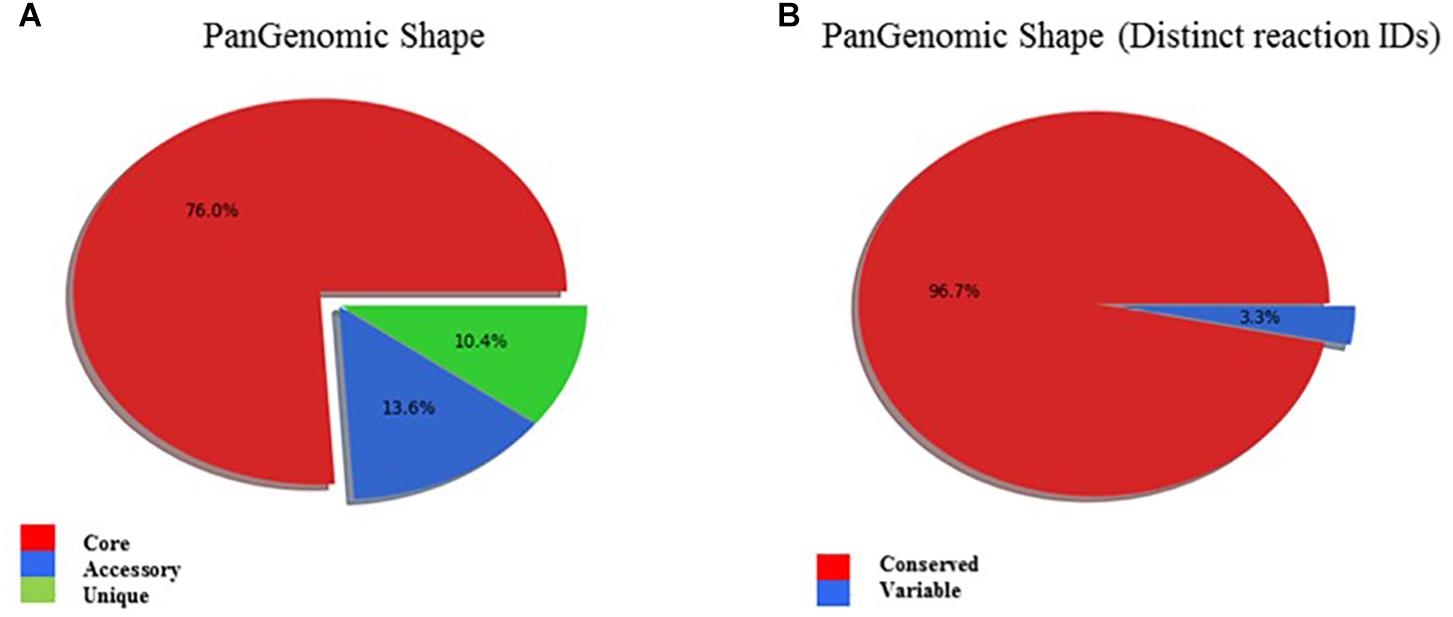
Figure 6. (A) PanGenomic shape showing high genomic conservation amongst outbreak associated L. monocytogenes strains. Genes found in all strains are labeled as “core,” and the others as “dispensable”: Dispensable genome is divided into “accessory,” when a gene is present in at least two strains, and “unique,” when a gene is present in exactly one strain. (B) PanGenomic Shape based on distinct reaction IDs demonstrates high metabolic pathway conservation amongst the outbreak associated L. monocytogenes strains.
Genome and phenome analysis also revealed various metabolic pathways where there was genetic and phenotypic variability among the strains leading in some cases to the identification of single reactions that were responsible for the observed metabolic variability. Examples include the starch and sucrose metabolism pathway shown in Figure 7. As indicated by the boxes highlighted in orange and yellow in Figure 7 there were variations associated with the presence of sucrose PTS permease (reaction 2.7.1.211) and maltose-6′-phosphate 6-phosphoglucohydrolase (reaction 3.2.1.122) proteins that are encoded by genes constituting the dispensable genome among strains. Genes encoding for the 2.7.1.211 protein were only detected in LL195, N16-0044, and N2306 strains whereas the 3.2.1.122 protein encoding gene was only detected in N2306. Notably, the latter also represents the only unique reaction identified by DuctApe amongst the studied strains (Table 6). The other C-sources also found to have a high phenotypic variability in their metabolism were cellobiose, dextrin and maltose (Figure 7). The analysis also indicated that most of the reactions are catalyzed by proteins encoded by genes which were not retrieved from the genomic data (Figure 6 and Table 6). The largest source of variation in genome content between the examined strains was related to the number and composition of the prophage elements (Supplementary Table S4). Although there are some strain specific differences, outbreak strains of lineage II contained more phage encoded elements than lineage I strains. The possible influence of such variations in these phage elements on C-source metabolism as well as stress response and virulence phenotypes observed here presently remains unknown.
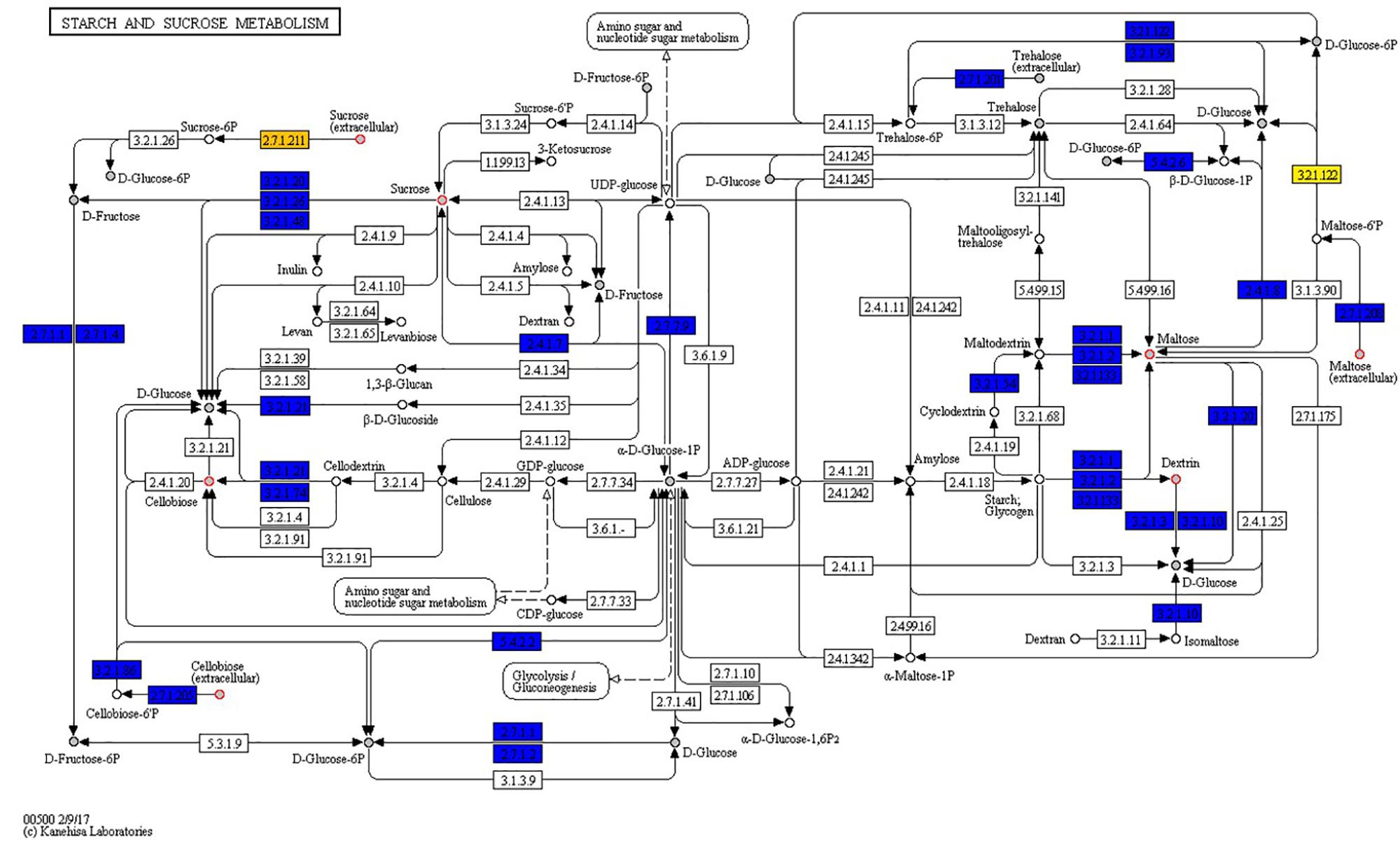
Figure 7. Strains show high phenotypic variability despite high genomic and metabolic pathway conservations. Starch and sucrose metabolism map: boxes represent reactions while circles represent compounds. Core reactions are colored blue, variable reactions are colored orange or yellow; compounds present in PM plates are filled with gray. Red circles around compounds highlight those compounds for which at least one strain has an AV difference with another strain equal or higher than 2 AV.
Meanwhile there were also several thousands (8,908–137,297 SNPs) of SNP differences detected between the studied outbreak strains (Figure 8). It is thus possible that some of these SNP differences are functionally relevant and contribute to differences observed not only in C-source utilization phenotypes but also to variations in stress resistance and virulence phenotypes that were detected between this set of listeriosis outbreak strains. A comparison with respect to genome virulence factor composition and sequence among the strains also revealed that despite high conservation in most virulence factors there were various minor lineage and strain specific genetic differences detected in some of the key virulence factors including some amino acid changing SNPs in internalin A (Supplementary Tables S5, S6). Therefore, our observation in this regard indicates that the differences observed in host cell invasion and virulence capacity amongst the examined outbreak strains might involve variation in a few genes and/or differences in overall virulence gene regulation and expression between strains. Uncovering such differences, however, will require further investigation in these strains in the future.
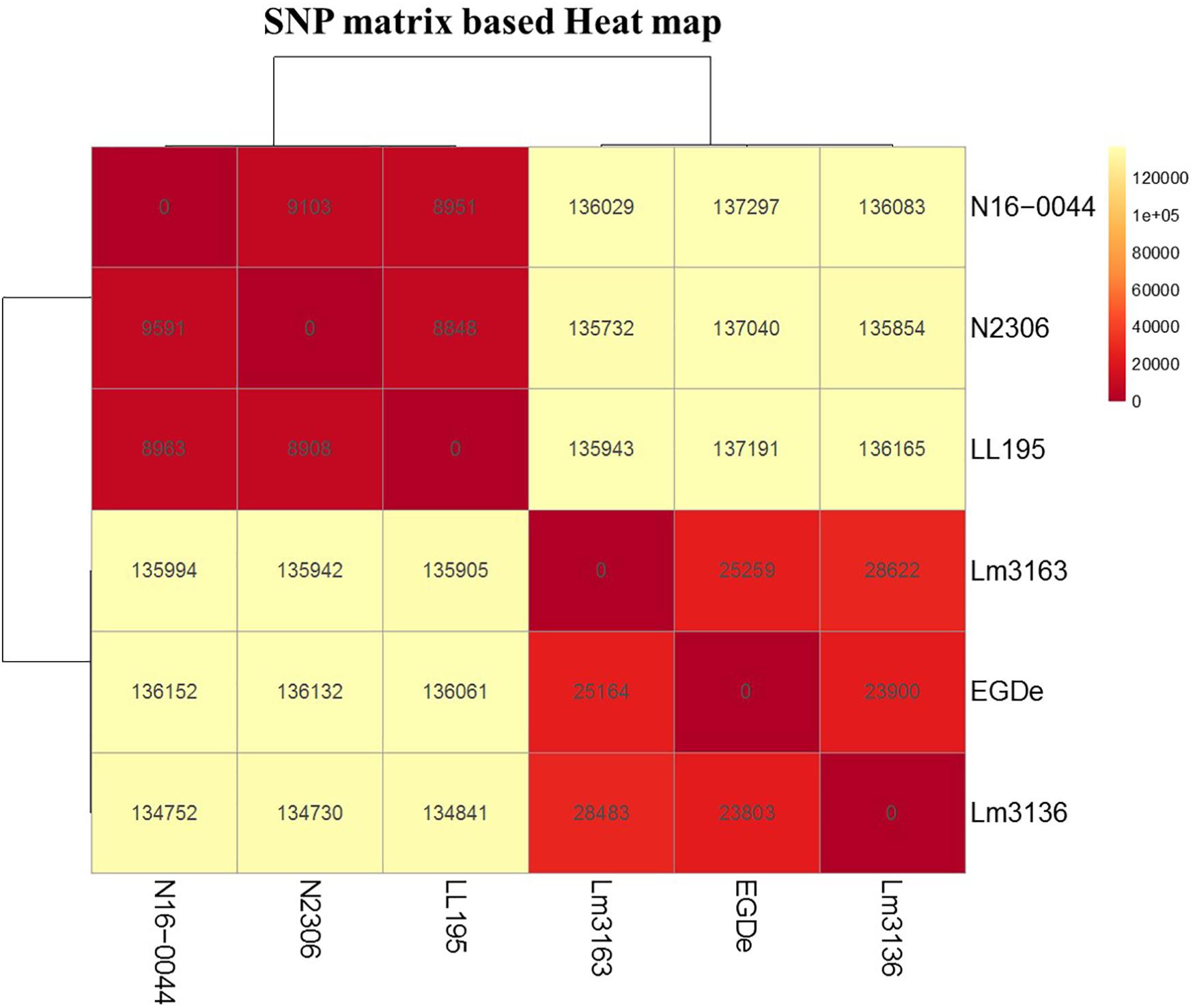
Figure 8. SNP matrix-based heatmap showing the variation in core genome SNPs between strains examined in this study.
Discussion
In this study, we describe the comparative analysis in a set of clinical and food related L. monocytogenes strains including those responsible for previous Swiss listeriosis outbreaks. Despite high levels of synteny in gene content and conservation of metabolic pathways our analysis uncovered significant phenotypic differences among strains with respect to metabolism of host cell and food relevant C-sources as well as resistance of some common food preservatives. Strain Lm3163 that caused the 2005 Tomme cheese Swiss listeriosis outbreak exhibited highest C-source metabolic activity and versatility among the tested strains. It efficiently utilized several food and host relevant C-sources that other tested strains could not metabolize including lactose, sucrose, pyruvic acid and nucleosides. All in all, the examined strain set varied with respect to C-source utilization, osmotic and pH stress tolerance, virulence capacity, and growth in nutrient rich bacterial and cell culture media. The various phenotypic diversities uncovered could provide selective advantages to some of the strains in natural and food-associated environments leading to increased survival and expanded niche range for such strains in the environment as well as infected hosts. Genome sequence comparison in outbreak associated strains revealed minor gene content differences between the strains including lineage specific distribution of some genes. One notable such genetic variation with an impact on C-source utilization involves the D-allose metabolism associated lmo0734 to lmo0739 gene cassette found in genetic lineage II but not in lineage I strains. Similar observations were reported from the examination of an even larger L. monocytogenes strain collection from China (Liu et al., 2017). Overall our observations indicate that minor genetic changes possibly induced through SNPs as well as variations in gene expression regulation amongst strains are probably behind the bulk of the phenotypic differences detected in our study.
Listeria monocytogenes has evolved to survive and replicate in the cytosol of eukaryotic cells including specific metabolic adaptations to optimize survival of host-derived stresses while promoting nutrient acquisition and proliferation within host cells (Grubmüller et al., 2014; Chen et al., 2017). Glycerol and glucose-6-phosphate play an important role as major intracellular carbon substrates for energy generation and anabolism respectively for this bacterium (Grubmüller et al., 2014). In agreement with this all analyzed strains used glycerol but not glucose-6P as a C-source. Despite presence of complete glycolytic and pentose-phosphate pathways based on genome analysis these strains were unable to utilize glucose-6P. This is consistent with repressed uptake of this C-source under extracellular assay conditions as applied in our study (Kreft and Vazquez-Boland, 2001). This repression arises from extracellular suppression of prfA expression, which positively regulates the expression of the hpt gene encoding the Hpt transporter required for the uptake of glucose-6P (Chico-Calero et al., 2002). Most of the amino acids imported by L. monocytogenes inside host cells are directly used for protein biosynthesis and are hardly catabolized (Grubmüller et al., 2014; Chen et al., 2017). Consistent with such observations the examined strains only used a single amino acid Threonine as a carbon source, albeit at a slow rate. Previous work on the EGDe strain suggested that L. monocytogenes is not able to utilize pyruvic acid (Grubmüller et al., 2014). While most examined strains could not utilize pyruvic acid as a C-source, Lm3163 was an exception it was able to use it as a C-source.
Survival and adaptation against acidic stress is crucial for host pathogenicity since L. monocytogenes encounters acidic challenge in the gastrointestinal tract and phagocytic vacuole during infection (Sleator and Hill, 2002; Begley and Hill, 2015). Observed differences in pH stress sensitivity amongst strains indicate differences in acid stress response mechanisms or their functional efficiencies in examined strains. Variable acid stress adaptation capacities among the examined strains could also have an impact on host pathogenicity since some acid tolerance systems are also linked to virulence in L. monocytogenes (Bowman et al., 2010; Begley and Hill, 2015). Serovars specific differences in distribution of acid stress survival genes such as those of the gadD1T1 operon among non-serotype four strains have been reported (Cotter and Hill, 2003). In our case, however, serotype 4b outbreak strains LL195 and N16-0044 showed higher acid stress tolerance than others indicating the contribution of other mechanism besides gadD1T1 operon to observed acid stress tolerance differences. Previous studies by others also noted increased organic acid stress exposure tolerance in some outbreak related serotype 4b isolates but mechanisms underlying such an enhanced acid tolerance among the serotype 4b strains remain unknown (Lianou et al., 2006).
Our findings here also showed that the availability of some amino acids enhances L. monocytogenes resistance to acidic pH (Table 4). Growth at pH 4.5 was restored in all tested strains in presence of L-norvaline suggesting that this amino acid enhances acidic pH stress resistance in L. monocytogenes. Protective mechanisms underlying such a phenomenon are not yet clear, but it appears to be not connected with an ability to metabolize this amino acid. Inclusion of β-phenylethylamine on the other hand inhibited growth/metabolism in strains Lm3136 and Lm3163 at pH 9.5, indicating a strain specific ability of this amino acid to inhibit L. monocytogenes growth under alkaline conditions. Interestingly, similar observations were reported in E. coli where β-phenylethylamine was used to reduce its growth on meat and biofilm production (Lynnes et al., 2014). Our observations suggest that β-phenylethylamine can also potentially be exploited in developing novel ways to control L. monocytogenes in foods.
Osmolytes including NaCl are widely used as food preservatives against bacteria and L. monocytogenes encounters osmotic stress within human gastrointestinal tracts (Bergholz et al., 2010; Schuppler and Loessner, 2010; Begley and Hill, 2015). Increased osmotic stress tolerance can thus improve survival of this pathogen in environmental niches associated with foods and human gastrointestinal tracts. As most of our study strains were associated with foods where elevated salt concentrations are encountered, their enhanced osmo-tolerance properties could have been an important factor in enabling survival and growth at elevated salt concentrations in such foods. An association between salt stress adaptation and virulence in L. monocytogenes was previously highlighted and an osmotic stress sensitive mutant deleted in the osmotic stress protection gene opuC showed poor colonization of the upper small intestine and a reduced ability to cause systemic infection in mice (Sleator et al., 2001; Begley and Hill, 2015). We presume that some outbreak strains in our study showing high tolerance to elevated NaCl salt levels could be better equipped for survival and growth in food as well as in subsequent human gastrointestinal colonization and infection. Strain LL195 isolated during the 1983–1987 Vacherin Montd’or cheese associated listeriosis outbreak showed enhanced salt and acid stress tolerance. Such features could have aided in long-term survival and proliferation of this strain in cheese and associated processing environment where both salt and acid stress conditions are encountered. The strains LL195 and N16-0044 that showed the highest acid and osmotic stress tolerance were also the most pathogenic strains as evaluated in the zebrafish infection model.
Our study also uncovered variable growth among some of the examined strains even in nutrient rich media such as BHI and MEM. The Tomme cheese outbreak-associated strain Lm3163 grew more rapidly than all the other strains and showed biphasic growth curves in both media (Figure 5). One possible explanation for the biphasic growth curves could therefore be the strain switching carbon source utilization during growth when one of the more highly metabolizable source gets exhausted. This effect might be explained through the carbon catabolite repression (Kreft and Vazquez-Boland, 2001). As earlier indicated, this strain has an expanded carbon source metabolic profile. When a strain achieves such increased capacity to utilize a wide spectrum of nutrient sources, it is more likely to grow rapidly on contaminated food. This might also explain why this strain was isolated during an outbreak where CFU counts as high as 3.2 × 104 CFU/g were observed on the contaminated cheese (Bille et al., 2006). Although it might appear trivial, the ability of this strains to use some nutrients such as xylose and pectin could also have had a profound effect on survival and dissemination in the environment.
During infection, L. monocytogenes re-encounters many of the same stresses it experiences in food matrices meaning mechanisms essential for survival in foods can also influence host virulence potential. Certain environmental conditions such as exposure to mild acid stress might adapt foodborne pathogens to life within the host (Conte et al., 2000; Begley and Hill, 2015). In a bid to assess for possible relationship between the phenotypic diversity uncovered in carbon source metabolism and stress resistance to host pathogenicity we also evaluated our strains with respect to their virulence phenotypes. L. monocytogenes virulence and survival within host cells is dependent on a variety of virulence proteins including the hemolysin listeriolysin O (LLO) that are regulated through PrfA (Chatterjee et al., 2006; Desvaux and Hebraud, 2006; Cossart and Lebreton, 2014; Radoshevich and Cossart, 2018). Despite LLO production as judged by hemolysis levels being similar among strains, listeriosis outbreak strains showed superior epithelial cell invasion ability than the reference strain L. monocytogenes EGDe. The cell invasion capacity of Lm3163 was significantly higher compared to all the other tested strains. We hypothesize that the high invasiveness of this strain might be due to its ability to multiply more rapidly than other strains within the cell culture media and infected cells. Lm3163 has an expanded and more flexible metabolic profile that includes metabolism of intracellular located substrates such as pyruvic acid suggesting that it might have multiplied faster during the invasion assay in comparison to the other strains in the media and inside the cell after invasion. In addition, there were amino acid changing SNPs detected in some virulence factors including Internalin A, which could also have contributed to host cell invasion differences observed between strains.
Listeria monocytogenes strains vary in virulence capacity as indicated by the variable distribution of genetic and serological subtypes in food associated environments compared to human and animal clinical listeriosis cases (Liu, 2008; Orsi et al., 2011; Maury et al., 2016; Moura et al., 2016). Virulence analysis using zebrafish embryos indicated genetic lineage and serotype related trends with lineage I and serotype 4b strains being more virulent than lineage II and serotype 1/2a strains. Interestingly Lm3163 the strain that utilized most of the C-sources while highly invasive in Caco-2 cells was the least virulent in this model. Reasons for this remain unknown although it might be tempting to speculate that its ability to metabolize some C-sources found in the zebrafish host environment could also have a repressive effect on virulence gene expression. It is known that metabolism of some carbohydrates taken up via the PTS strongly repress the expression of virulence genes (Deutscher et al., 2014). PrfA, the main regulator of virulence genes is repressed by some PTS substrates although mechanisms of such repressions are not yet fully understood (de las Heras et al., 2011; Cossart and Lebreton, 2014; Deutscher et al., 2014; Radoshevich and Cossart, 2018). Notably Lm3163 was the only strain that could metabolize PTS sugars such as mannitol, lactose, and sucrose, which could in part have contributed to virulence repression in this strain (Kreft and Vazquez-Boland, 2001). Although genome virulence factor composition analysis showed a high conservation of most virulence factors there were also some lineage specific virulence genes detected. Such lineage specific virulence gene distribution coupled with some amino acid changing SNPs in conserved virulence genes and differences gene expression regulation could all contribute to observed lineage associated strain variability in pathogenicity.
Genome comparisons between the strains showed high genome conservation consistent with previous observations among L. monocytogenes strains (Orsi et al., 2011). Despite this some minor differences that could contribute to phenotypic differences amongst strains were observed including the lineage specific distribution of the lmo0734 to lmo0739 gene cassette in lineage II but not lineage I strains, which explains lineage specific difference in allose metabolism as described recently (Zhang et al., 2018). This could in part explain the predominance of lineage II amongst food isolates when isolation media that employ D-allose supplemented enrichment broths are used to improve L. monocytogenes isolation and reduce the growth of non-target organisms (Liu et al., 2017). The araBAD operon that directs arabinose catabolism in E. coli is dynamically activated in the presence of arabinose and the absence of glucose (Desai and Rao, 2010). Lm3163 utilized both D- and L-arabinose whilst the Lm3136 and N11-1515 strains utilized the former suggesting that they might possess a similar catabolic mechanism. Isolation and identification protocols often describe L. monocytogenes as incapable of utilizing L-arabinose and D-mannitol (Gawade et al., 2011; Orsi and Wiedmann, 2016), the demonstration that Lm3163 can metabolize both sugars bring into question whether some isolates could be miss classified as non-L. monocytogenes. Genomes of the study strains also contain varying numbers and composition of phage-related elements with lineage II containing more elements than lineage I strains. Prophages have been demonstrated to both detract from and add to an organism’s fitness, by disrupting the function of the gene into which they have incorporated or, by encoding genes aiding faster cell growth (Milillo et al., 2012). It is thus plausible that some of these elements might be contributing to phenotypic variability observed amongst strains with respect to growth capacity and virulence phenotypes.
Phenome genome link analysis with DuctApe revealed some metabolic pathways showing genetic and phenotypic variability among the analyzed strains. It revealed that some alternate pathways for certain carbon source metabolism might exist and are yet to be discovered. This is exemplified by the starch and sucrose metabolism pathway, which highlighted that Lm3163 lacks a transporter (sucrose PTS permease) required for sucrose uptake but still metabolizes sucrose. Whereas LL195, N16-0044, and N2306 strains carrying genes encoding the sucrose transporter could not utilize sucrose. It also revealed that strains with similar cellobiose metabolism gene content patterns had different cellobiose metabolism capacities a phenomenon that might be a result of epigenetics. It also highlighted the presence of genes encoding maltose-6′-phosphate 6-phosphoglucohydrolase on N2306 genome. However, the importance of this Listeria pathogenicity island 4 (LIP4) associated protein regarding maltose metabolism could not be established as this strain had similar maltose metabolic capacity like other strains lacking this enzyme (Maury et al., 2016). Two strains could not utilize maltose despite having complete pathways for its metabolism, which might also be attributed to differences in the epigenetic regulation of gene expression among strains. Meanwhile the differences in genetic makeup amongst the strains and between the lineages such as the variability in number and composition of transporters and pumps amongst the strains and between lineages could in some cases be associated with lineage and strain specific phenotypic differences such as lineage II specific D-allose metabolism or the ability of Lm3163 to utilize the most diverse set of C-sources.
All bacteria require nutrients and minerals for growth. For saprophytic or pathogenic bacterium, these nutrients can be derived from decaying vegetation, host body fluids, host cells or other resident or dietary microbes (Fischbach and Sonnenburg, 2011). Knowing the full metabolic requirements of a bacterium can lead to a better understanding of the conditions under which it is likely to thrive in food vehicles and infected hosts as well as enable the design of interventions to prevent this. Overall the C-source utilization and stress resistance profiles defined might provide a basis for developing improved media for L. monocytogenes isolation and detection. Alternatively, such knowledge can also be exploited to come up with novel protocols for L. monocytogenes control in foods, for example, by limiting nutrient availability through the use of natural inhibitors or chemical mimics that block uptake of certain key C-sources. This data forms the basis for further studies of such nature with more strains to understand the extent of these phenotypic differences and possibly apply the knowledge gained to improve food safety.
Author Contributions
TT designed and supervised the study. FM and AE performed the experiments. UvA assisted with the PM experiments. FM, TT, AE, UvA, and MS analyzed the data and wrote the manuscript.
Funding
This project was funded by the University of Zürich and done in collaboration with Agroscope, Bern, Switzerland.
Conflict of Interest Statement
The authors declare that the research was conducted in the absence of any commercial or financial relationships that could be construed as a potential conflict of interest.
Acknowledgments
The authors would like to thank Anne Guisolan (Agroscope, Bern, Switzerland) for providing technical assistance with the PM experiments and Dieter Weik (Agroscope, Bern, Switzerland) for availing his lab for part of the PM experiments done at Agroscope.
Supplementary Material
The Supplementary Material for this article can be found online at: https://www.frontiersin.org/articles/10.3389/fmicb.2019.00957/full#supplementary-material
Footnotes
References
Allerberger, F., and Wagner, M. (2010). Listeriosis: a resurgent foodborne infection. Clin. Microbiol. Infect 16, 16–23. doi: 10.1111/j.1469-0691.2009.03109.x
Althaus, D., Jermini, M., Giannini, P., Martinetti, G., Reinholz, D., Nüesch-Inderbinen, M., et al. (2017). Local outbreak of Listeria monocytogenes serotype 4b sequence type 6 due to contaminated meat pâté. Foodborne Pathog. Dis. 14, 219–222. doi: 10.1089/fpd.2016.2232
Althaus, D., Lehner, A., Brisse, S., Maury, M., Tasara, T., and Stephan, R. (2014). Characterization of Listeria monocytogenes strains isolated during 2011-2013 from human infections in Switzerland. Foodborne Pathog. Dis. 11, 753–758. doi: 10.1089/fpd.2014.1747
Baranyi, J., and Roberts, T. A. (1994). A dynamic approach to predicting bacterial-growth in food. Int. J. Food Microbiol. 23, 277–294. doi: 10.1016/0168-1605(94)90157-0
Begley, M., and Hill, C. (2015). Stress adaptation in foodborne pathogens. Annu. Rev. Food Sci. Technol. 6, 9.1–9.20. doi: 10.1146/annurev-food-030713-092350
Bergholz, T. M., den Bakker, H. C., Fortes, E. D., Boor, K. J., and Wiedmann, M. (2010). Salt stress phenotypes in Listeria monocytogenes vary by genetic lineage and temperature. Foodborne Pathog. Dis. 7, 1537–1549. doi: 10.1089/fpd.2010.0624
Bille, J. (1990). “Epidemiology of human listeriosis in Europe with special reference to the Swiss outbreak,” in Foodborne Listeriosis, eds A. J. Miller, J. L. Smith, and G. A. Somkuti (New York, NY: Elsevier), 71–74.
Bille, J., Blanc, D. S., Schmid, H., Boubaker, K., Baumgartner, A., Siegrist, H. H., et al. (2006). Outbreak of human listeriosis associated with tomme cheese in northwest Switzerland, 2005. Euro. Surveill. 11, 91–93.
Bochner, B. R., (2009). Global phenotypic characterization of bacteria. FEMS Microbiol. Rev. 33, 191–205. doi: 10.1111/j.1574-6976.2008.00149.x
Bowman, J. P., Chang, K. J. L., Pinfold, T., and Ross, T. (2010). Transcriptomic and phenotypic responses of Listeria monocytogenes strains possessing different growth efficiencies under acidic conditions. Appl. Environ. Microbiol. 76, 4836–4850. doi: 10.1128/AEM.00315-10
Bula, C. J., Bille, J., and Glauser, M. P. (1995). An epidemic of food-borne listeriosis in western switzerland: description of 57 cases involving adults. Clin. Infect. Dis 20, 66–72.
Centers for Disease Control [CDC] (2018). Centre for disease control and prevention. Preliminary incidence and trends of infections with pathogens transmitted commonly through food. Foodborne Diseases Active Surveillance Network, 10 U.S. Sites, 2006-2017. MMWR Morb. Mortal. Wkly Rep. 67, 324–328.
Chatterjee, S. S., Hossain, H., Otten, S., Kuenne, C., Kuchmina, K., Machata, S., et al. (2006). Intracellular gene expression profile of Listeria monocytogenes. Infect. Immun. 74, 1323–1338. doi: 10.1128/IAI.74.2.1323-1338.2006
Chen, G. Y., Pensinger, D. A., and Sauer, J. D. (2017). Listeria monocytogenes cytosolic metabolism promotes replication, survival and evasion of innate immunity. Cell Microbiol. 19:e12762. doi: 10.1111/cmi.12762
Chen, L. H., Zheng, D. D., Liu, B., Yang, J., and Jin, Q. (2016). VFDB 2016: hierarchical and refined dataset for big data analysis—10 years on. Nucleic Acids Res. 44, D694–D697. doi: 10.1093/nar/gkv1239
Chico-Calero, I., Suarez, M., Gonzalez-Zorn, B., Scortti, M., Slaghuis, J., Goebel, W., et al. (2002). Hpt, a bacterial homolog of the microsomal glucose-6-phosphate translocase, mediates rapid intracellular proliferation in Listeria. Proc. Natl. Acad. Sci. U.S.A. 99, 431–436. doi: 10.1073/pnas.012363899
Conte, M. P., Petrone, G., Di Biase, A. M., Ammendolia, M. G., Superti, F., and Seganti, L. (2000). Acid tolerance in Listeria monocytogenes influences invasiveness of enterocyte-like cells and macrophage-like cells. Microb. Pathog. 29, 137–144. doi: 10.1006/mpat.2000.0379
Contreras-Moreira, B., and Vinuesa, P. (2013). GET_HOMOLOGUES, a versatile software package for scalable and robust microbial pangenome analysis. Appl. Environ. Microbiol. 79, 7696–7701. doi: 10.1128/AEM.02411-13
Cossart, P., and Lebreton, A. (2014). A trip in the “New Microbiology” with the bacterial pathogen Listeria monocytogenes. FEBS Lett. 588, 2437–2445. doi: 10.1016/j.febslet.2014.05.051
Cotter, P. D., and Hill, C. (2003). Surviving the acid test: responses of gram-positive bacteria to low pH. Microbiol. Mol. Biol. Rev. 67, 429–453. doi: 10.1128/MMBR.67.3.429-453.2003
Darling, A. E., Mau, B., and Perna, N. T. (2010). Progressive mauve: multiple genome alignment with gene gain, loss and rearrangement. PLoS One 5:e11147. doi: 10.1371/journal.pone.0011147
de las Heras, A., Cain, R. J., Bielecka, M. K., and Vázquez-Boland, J. A. (2011). Regulation of listeria virulence: PrfA master and commander. Curr. Opin. Microbiol 14, 118–127. doi: 10.1016/j.mib.2011.01.005
Desai, T. A., and Rao, C. V. (2010). Regulation of arabinose and xylose metabolism in Escherichia coli. Appl. Environ. Microbiol. 76, 1524–1532. doi: 10.1128/AEM.01970-09
Desvaux, M., and Hebraud, M. (2006). The protein secretion systems in Listeria: inside out bacterial virulence. FEMS Microbiol. Rev. 30, 774–805. doi: 10.1111/j.1574-6976.2006.00035.x
Deutscher, J., Aké, F. M. D., Zébré, A. C., Cao, T. N., Kentache, T., Monniot, C., et al. (2014). “Carbohydrate utilization by Listeria monocytogenes and its influence on virulence gene expression,” in Listeria monocytogenes: Food Sources, Prevalence and Management Strategies, ed. E. C. Hambrick (New York, NY: Nova Science Publishers), 49–76.
Ebner, R., Stephan, R., Althaus, D., Brisse, S., Maury, M., and Tasara, T. (2015). Phenotypic and genotypic characteristics of Listeria monocytogenes strains isolated during 2011-2014 from different food matrices in Switzerland. Food Control. 57, 321–326. doi: 10.1016/j.foodcont.2015.04.030
Eshwar, A. K., Guldimann, C., Oevermann, A., and Tasara, T. (2017). Cold-shock domain family proteins (Csps) are involved in regulation of virulence, cellular aggregation, and flagella-based motility in Listeria monocytogenes. Front. Cell. Infect. Microbiol. 7:453. doi: 10.3389/fcimb.2017.00453
European Food Safety Authority [EFSA] (2017). The European Union summary report on trends and sources of zoonoses, zoonotic agents and food-borne outbreaks in 2016. EFSA J. 15:5077. doi: 10.2903/j.efsa.2017.5077
Fischbach, M. A., and Sonnenburg, J. L. (2011). Eating for two: how metabolism establishes interspecies interactions in the gut. Cell Host. Microbe. 10, 336–347. doi: 10.1016/j.chom.2011.10.002
Fox, E. M., and Jordan, K. (2014). “High-throughput characterization of Listeria monocytogenes using the omnilog phenotypic microarray,” in Listeria monocytogenes: Methods in Molecular Biology (Methods and Protocols), Vol. 1157, eds K. Jordan, E. Fox, and M. Wagner (New York, NY: Humana Press), 103–108. doi: 10.1007/978-1-4939-0703-8_9
Galardini, M., Mengoni, A., Biondi, E. G., Semeraro, R., Florio, A., Bazzicalupo, M., et al. (2014). DuctApe: a suite for the analysis and correlation of genomic and OmniLogTM phenotype microarray data. Genomics 103, 1–10. doi: 10.1016/j.ygeno.2013.11.005
Gandhi, M., and Chikindas, M. L. (2007). Listeria: a foodborne pathogen that knows how to survive. Int. J. Food Microbiol. 113, 1–15. doi: 10.1016/j.ijfoodmicro.2006.07.008
Gawade, L., Barbuddhe, S. B., and Bhosle, S. (2011). Isolation and confirmation of listeria species from seafood off goa region by polymerase chain reaction. Indian J Microbiol. 50, 385–389. doi: 10.1007/s12088-011-0064-y
Glaser, P., Frangeul, L., Buchrieser, C., Rusniok, C., Amend, A., Baquero, F., et al. (2001). Comparative genomics of Listeria species. Science 294, 849–852. doi: 10.1126/science.1063447
Göker, M., Hofner, B., Montero Calasanz, M. C., Sikorski, J., and Vaas, L. A. I. (2016). opm: An R Package for Analysing Phenotype Microarray and Growth Curve Data. 1–68. Available at: http://www.goeker.org/opm/opm_doc/doc/opm-tutorial.pdf (accessed September 14, 2016).
Grubmüller, S., Schauer, K., Goebel, W., Fuchs, M. T., and Eisenreich, W. (2014). Analysis of carbon substrates used by Listeria monocytogenes during growth in J774A.1 macrophages suggests a bipartite intracellular metabolism. Front. Cell. Infect. Microbiol. 4:156. doi: 10.3389/fcimb.2014.00156
Guldimann, C., Bärtschi, M., Frey, J., Zurbriggen, A., Seuberlich, T., and Oevermann, A. (2015). Increased spread and replication efficiency of Listeria monocytogenes in organotypic brain-slices is related to multilocus variable number of tandem repeat analysis (MLVA) complex. BMC Microbiol. 15:134. doi: 10.1186/s12866-015-0454-0
Haase, J. K., Didelot, X., Lecuit, M., Korkeala, H., and Achtman, M. (2014). The ubiquitous nature of Listeria monocytogenes clones: a large-scale multilocus sequence typing study. Environ. Microbiol. 16, 405–416. doi: 10.1111/1462-2920.12342
Hachler, H., Marti, G., Giannini, P., Lehner, A., Jost, M., Beck, J., et al. (2013). Outbreak of listerosis due to imported cooked ham, Switzerland 2011. Euro. Surveill. 18:20469. doi: 10.2807/ese.18.18.20469-en
Huerta-Cepas, J., Szklarczyk, D., Forslund, K., Cook, H., Heller, D., Mathias, C., et al. (2016). eggNOG 4.5: a hierarchical orthology framework with improved functional annotations for eukaryotic, prokaryotic and viral sequences. Nucleic Acids Res. 44, D286–D293. doi: 10.1093/nar/gkv1248
Johansson, J., Mandin, P., Renzoni, A., Chiaruttini, C., Springer, M., and Cossart, P. (2002). An RNA thermosensor controls expression of virulence genes in Listeria monocytogenes. Cell 110, 551–561.
Joseph, B., Mertins, S., Stoll, R., Schär, J., Umesha, K. R., Luo, Q., et al. (2008). Glycerol metabolism and PrfA activity in Listeria monocytogenes. J. Bacteriol. 190, 5412–5430. doi: 10.1128/JB.00259-08
Kocaman, N., and Sarımehmetoğlu, B. (2016). Stress responses of Listeria monocytogenes. Ankara Üniv. Vet. Fak. Derg. 63, 421–427.
Kreft, J., and Vazquez-Boland, J. A. (2001). Regulation of virulence genes in Listeria. Int. J. Med. Microbiol. 291, 145–157. doi: 10.1078/1438-4221-00111
Lianou, A., Stopforth, J. D., Yoon, Y., Wiedmann, M., and Sofos, J. N. (2006). Growth and stress resistance variation in culture broth among Listeria monocytogenes strains of various serotypes and origins. J. Food Prot. 69, 2640–2647.
Liu, D., Wang, Y., Wang, Y., Zhang, L., Luo, L., Liu, K., et al. (2017). Development of a novel Listeria enrichment broth for the isolation of pathogenic Listeria. J. Food Prot. 80, 1768–1776. doi: 10.4315/0362-028X.JFP-16-529
Loepfe, C., Raimann, E., Stephan, R., and Tasara, T. (2010). Reduced host cell invasiveness and oxidative stress tolerance in double and triple csp gene family deletion mutants of Listeria monocytogenes. Foodborne Pathog. Dis. 7, 775–783. doi: 10.1089/fpd.2009.0458
Lynnes, T., Horne, S. M., and Pruss, B. M. (2014). ß-phenylethylamine as a novel nutrient treatment to reduce bacterial contamination due to Escherichia coli O157: H7 on beef meat. Meat Sci. 96, 165–171. doi: 10.1016/j.meatsci.2013.06.030
Maury, M. M., Tsai, Y., Charlier, C., Touchon, M., Chenal-Francisque, V., Leclercq, A., et al. (2016). Uncovering Listeria monocytogenes hypervirulence by harnessing its biodiversity. Nat. Genet. 48, 308–313. doi: 10.1038/ng.3501
Meier, A. B., Guldimann, C., Markkula, A., Pöntinen, A., Korkeala, H., and Tasara, T. (2017). Comparative phenotypic and genotypic analysis of swiss and finnish Listeria monocytogenes isolates with respect to benzalkonium chloride resistance. Front. Microbiol. 8:397. doi: 10.3389/fmicb.2017.00397
Metsalu, T., and Vilo, J. (2015). ClustVis: a web tool for visualizing clustering of multivariate data using principal component analysis and heatmap. Nucleic Acids Res. 43, W566–W570. doi: 10.1093/nar/gkv468
Milillo, R. S., Friedly, E. C., Saldivar, J. C., Muthaiyan, A., O’bryan, C., and Crandall, P. G. (2012). A Review of the Ecology, Genomics, and stress response of Listeria innocua and Listeria monocytogenes. Crit. Rev. Food Sci. Nutr. 52, 712–725. doi: 10.1080/10408398.2010.507909
Moura, A., Criscuolo, A., Pouseele, H., Maury, M. M., Leclercq, A., Tarr, C., et al. (2016). Whole genome-based population biology and epidemiological surveillance of Listeria monocytogenes. Nat. Microbiol. 2:16185. doi: 10.1038/nmicrobiol.2016.185
Nadon, C. A., Bowen, M. B., Wiedmann, M., and Boor, K. J. (2002). Sigma B contributes to PrfA-mediated virulence in Listeria monocytogenes. Infect. Immun. 70, 3948–3952. doi: 10.1128/IAI.70.7.3948-3952.2002
NicAogáin, K., and O’Byrne, C. P. (2016). The role of stress and stress adaptations in determining the fate of the bacterial pathogen Listeria monocytogenes in the food chain. Front. Microbiol. 7:1865. doi: 10.3389/fmicb.2016.01865
Ollinger, J., Bowen, B., Wiedmann, M., Boor, J. B. J., and Bergholz, T. M. (2009). Listeria monocytogenes σB modulates PrfA-mediated virulence factor expression. Infect. Immun. 77, 2113–2124. doi: 10.1128/IAI.01205-08
Orsi, R. H., den Bakker, H. C., and Wiedmann, M. (2011). Listeria monocytogenes lineages: genomics, evolution, ecology, and phenotypic characteristics. Int. J. Med. Microbiol. 301, 79–96. doi: 10.1016/j.ijmm.2010.05.002
Orsi, R. H., and Wiedmann, M. (2016). Characteristics and distribution of Listeria spp., including Listeria species newly described since 2009. Appl. Microbiol. Biotechnol. 100, 5273–5287. doi: 10.1007/s00253-016-7552-2
Radoshevich, L., and Cossart, P. (2018). Listeria monocytogenes: towards a complete picture of its physiology and pathogenesis. Nat. Rev. Microbiol. 16, 32–46. doi: 10.1038/nrmicro.2017.126
Scharer, K., Stephan, R., and Tasara, T. (2013). Cold shock proteins contribute to the regulation of listeriolysin O production in Listeria monocytogenes. Foodborne Path. Dis. 10, 1023–1029. doi: 10.1089/fpd.2013.1562
Schmid, B. J., Klumpp, J., Raimann, E., Loessner, M. J., Stephan, R., and Tasara, T. (2009). Role of cold shock proteins in growth of Listeria monocytogenes under cold and osmotic stress conditions. Appl. Environ. Microbiol. 75, 1621–1627. doi: 10.1128/AEM.02154-08
Schmid, H., and Baumgartner, A. (2012). Foodborne Outbreaks in Switzerland – Current Statistics, Future Developments, Practical Guideline for the Investigation of Outbreaks and a Historical Review. Berne: Federal Office of Public Health.
Schuppler, M., and Loessner, M. J. (2010). The opportunistic pathogen Listeria monocytogenes: pathogenicity and interaction with the mucosal immune system. Int. J. Inflam. 2010: 704321. doi: 10.4061/2010/704321
Sleator, R. D., and Hill, C. (2002). Bacterial osmoadaptation: the role of osmolytes in bacterial stress and virulence. FEMS Microbiol. Rev. 26, 49–71.
Sleator, R. D., Wouters, J., Gahan, C. G. M., Abee, T., and Hill, C. (2001). Analysis of the role of OpuC, an osmolyte transport system, in salt tolerance and virulence potential of Listeria monocytogenes. Appl. Environ. Microbiol. 67, 2692–2698. doi: 10.1128/AEM.67.6.2692-2698.2001
Soni, K. A., Nannapaneni, R., and Tasara, T. (2011). An overview of stress response proteomes in Listeria monocytogenes. Agric. Food Anal. Bacteriol. 1, 66–85. doi: 10.5167/uzh-60457
Stephan, R., Althaus, D., Kiefer, S., Lehner, A., Hatz, C., Schmutz, C., et al. (2015). Food- borne transmission of Listeria monocytogenes via ready-to-eat salad: a nationwide outbreak in Switzerland, 2013-2014. Food Control. 57, 14–17. doi: 10.1016/j.foodcont.2015.03.034
Tasara, T., Ebner, R., Klumpp, J., and Stephan, R. (2015). Complete genome sequence of Listeria monocytogenes N2306, a strain associated with the 2013-2014 listeriosis outbreak in Switzerland. Genome Announc. 3:e553-15. doi: 10.1128/genomeA.00553-15
Tasara, T., Klumpp, J., Bille, J., and Stephan, R. (2016). Genome sequences of Listeria monocytogenes strains responsible for cheese- and cooked ham product-associated Swiss listeriosis outbreaks in 2005 and 2011. Genome Announc. 4:e106-16. doi: 10.1128/genomeA.00106-16
Toledo-Arana, A., Dussurget, O., Nikitas, G., Nina Sesto, N., Guet-Revillet, H., Balestrino, D., et al. (2009). The Listeria transcriptional landscape from saprophytism to virulence. Nature 459, 950–956. doi: 10.1038/nature08080
Treangen, T. J., Ondov, B. D., Koren, S., and Phillippy, A. M. (2014). The harvest suite for rapid core-genome alignment and visualization of thousands of intraspecific microbial genomes. Genome Biol. 15, 1–15. doi: 10.1186/s13059-014-0524-x
Weinmaier, T., Riesing, M., Rattei, T., Bille, J., Arguedas-Villa, C., Stephan, R., et al. (2013). Complete genome sequence of Listeria monocytogenes LL195, a serotype 4b strain from the 1983–1987 listeriosis epidemic in Switzerland. Genome Announc. 1:e152-12. doi: 10.1128/genomeA.00152-12
Wilson, R. L., Brown, L. L., Kirkwood-Watts, D., Warren, T. K., Lund, S. A., King, D. S., et al. (2006). Listeria monocytogenes 10403S HtrA is necessary for resistance to cellular stress and virulence. Infect. Immun. 74, 765–768. doi: 10.1128/IAI.74.1.765-768.2006
Keywords: Listeria monocytogenes, phenotype microarray, phenotype, genome, carbon source, stress, virulence
Citation: Muchaamba F, Eshwar AK, Stevens MJA, von Ah U and Tasara T (2019) Variable Carbon Source Utilization, Stress Resistance, and Virulence Profiles Among Listeria monocytogenes Strains Responsible for Listeriosis Outbreaks in Switzerland. Front. Microbiol. 10:957. doi: 10.3389/fmicb.2019.00957
Received: 01 February 2019; Accepted: 16 April 2019;
Published: 03 May 2019.
Edited by:
Rosalba Lanciotti, University of Bologna, ItalyReviewed by:
Ricardo Calderón González, Queen’s University Belfast, United KingdomKimon Andreas Karatzas, University of Reading, United Kingdom
Copyright © 2019 Muchaamba, Eshwar, Stevens, von Ah and Tasara. This is an open-access article distributed under the terms of the Creative Commons Attribution License (CC BY). The use, distribution or reproduction in other forums is permitted, provided the original author(s) and the copyright owner(s) are credited and that the original publication in this journal is cited, in accordance with accepted academic practice. No use, distribution or reproduction is permitted which does not comply with these terms.
*Correspondence: Taurai Tasara, dGFzYXJhdEBmc2FmZXR5LnV6aC5jaA==; dGF1cmFpLnRhc2FyYUB1emguY2g=