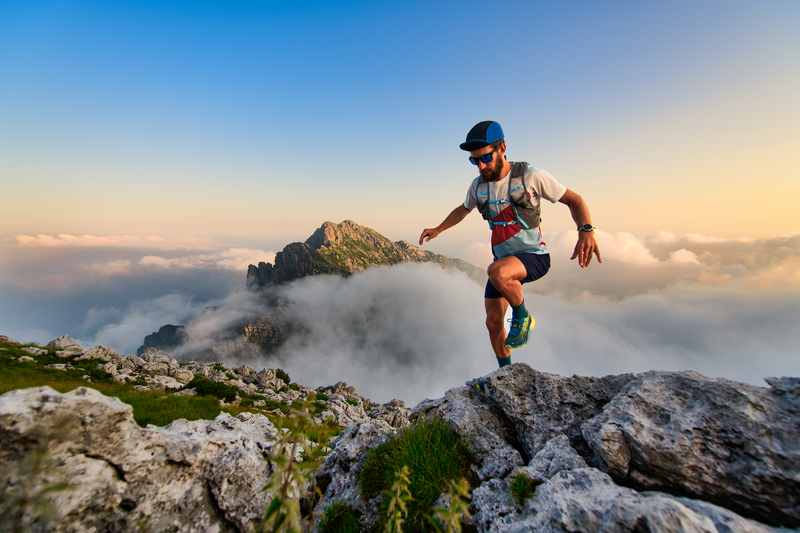
95% of researchers rate our articles as excellent or good
Learn more about the work of our research integrity team to safeguard the quality of each article we publish.
Find out more
ORIGINAL RESEARCH article
Front. Microbiol. , 26 April 2019
Sec. Food Microbiology
Volume 10 - 2019 | https://doi.org/10.3389/fmicb.2019.00903
Accumulating evidence has revealed the dysbiosis of gut/fecal microbiota induced by heat stress (HS) in mammals and poultry. However, the effects of HS on microbiota communities in different intestinal segments of Cherry-Valley ducks (a widely used meat-type breed) and their potential associations with growth performances, fat deposition, intestinal morphology, and antioxidant capacity have not been well evaluated yet. In this study, room temperature (RT) of 25°C was considered as control, and RT at 32°C for 8 h per day was set as the HS treatment. After 3 weeks, the intestinal contents of jejunum, ileum, and cecum were harvested to investigate the microbiota composition variations by 16S ribosomal RNA amplicon sequencing. And the weight gain, adipose indices, intestinal morphology, and a certain number of serum biochemical parameters were also measured and analyzed. The results showed the microbial species at different levels differentially enriched in duck jejunum and cecum under HS, while no significant data were observed in ileum. HS also caused the intestinal morphological changes (villus height and the ratio of villus height to crypt depth) and the reductions of growth speed (daily gain), levels of serum triglyceride (TG) and total cholesterol, and antioxidant activity (higher malondialdehyde (MDA) content and lower total antioxidant). The higher abdominal fat content and serum glucose level were also observed in HS ducks. The Spearman correlation analysis indicated that in jejunum the phyla Firmicutes and Proteobacteria were associated with average daily gain, feed/gain, serum TG and MDA levels, and villus height/crypt depth (P < 0.05). The phylum Firmicutes and genus Acinetobacter were significantly associated with fat deposition and serum glucose level (P < 0.05). The genus Lactobacillus was positively associated with serum total antioxidant (P < 0.05), while some other microbial species were found negatively associated, including order Pseudomonadales, genera Acinetobacter, and unidentified_Mitochondria. However, no significant correlations were observed in cecum. These findings imply the potential roles of duck gut microbiota in the intestinal injuries, fat deposition, and reductions of growth speed and antioxidant capacity caused by HS, although the molecular mechanisms requires further investigation.
Heat stress (HS) is a great threat to livestock industries, especially in the past decades due to the global warming and has induced major economic loss (St-Pierre et al., 2003; Hansen et al., 2010; Mack et al., 2013; Nawab et al., 2018). HS has a profound effect on animal health and production performance (Humphrey, 2006). HS causes multiple physiological disturbances, such as endocrine disorders, immune dysregulation, electrolyte imbalance, and so on (Teeter et al., 1985; Sohail et al., 2010). And also, HS could lead to the abnormalities of energy metabolism, fat deposition, and body oxidative status (Baziz et al., 1996; Küchenmeister et al., 1999; Rhoads et al., 2009; Faylon et al., 2015; He et al., 2018).
In recent years, gut microbiota, considered as a “microbial organ,” has become a central research focus because it has a symbiotic relationship with host health and plays an essential role in the nutrient digestion and absorption, immune system development, and host protection against pathogens (Leser and Molbak, 2009; Vasai et al., 2014b; Whiteside et al., 2015). Balanced intestinal microflora benefit host by excluding pathogens, improving intestinal barrier integrity, maintaining normal nutrient digestion and absorption, promoting other commensals, and so on (Burkholder et al., 2008; Song et al., 2013). However, it has been reported that HS negatively affects intestinal mucosa and microbiota composition (Liu et al., 2009; Kers et al., 2018). Damage to mucosal epithelium can directly affect intestinal barrier function, nutrient absorption and impair production performance (Elphick, 2005; Moeser et al., 2007). With inefficient heat dissipation and lack of sweat glands, poultry appears more susceptible to HS (Lara and Rostagno, 2013). In chicken, some reports are available about the influences of HS on gut microbiota in different intestinal segments and the use of dietary supplementations to improve intestinal morphology and microflora balance, like oligosaccharide (Song et al., 2013; Ghasemian and Jahanian, 2016), N-acetylcysteine (Yi et al., 2016), Artemisia annua (Song et al., 2017), and probiotics (Al-Fataftah and Abdelqader, 2014; Song et al., 2014). The data about gut microbiota in different duck breeds, including Peking, Muscovy, Sheldrake and Mule, are mainly on the application of overfeeding or functional dietary supplementation to promote production performances (Jiang et al., 2014; Vasai et al., 2014a,b). Similar data could also be found on zebrafish (Wang et al., 2019). Whereas, the effects of HS on the gut microbiota composition and its association with physiological changes have not been well evaluated.
In the present study, Cherry-Valley duck, a widely used meat-type breed, was selected to investigate the influences of HS on duck gut microbiota. After thermal treatment, the 16S rDNA sequencing analysis was performed to learn the variations of microflora compositions in different intestinal segments (jejunum, ileum, and cecum), and probe into their possible associations intestinal morphology, fat deposition, and oxidation status. The results help better understand the effects of HS on duck physiology and the probably involved bacterial species, and it might provide useful information for preventing or ameliorating the deficits of duck production caused by prolonged high-temperature environment.
A total of 30 Cherry-Valley ducks (male, 5-week-old, 1.5∼1.8 kg) with the same genetic background were collected and randomly divided into 2 groups, control (C), and treatment (T). Each duck was housed in a separate cage. In the first week, the room temperature (RT) was controlled at 25°C for adaption. Then for the next 3 weeks, the RT of group T was promoted to 32°C and kept for 8 h per day (10:00 am to 18:00 pm), while no RT change was made to group C. Throughout the whole experimental period, all birds were watered and fed ad libitum. The diet ingredients and nutrient contents are listed in Table 1. After fasting for 12 h, the birds were weighed and the blood samples were harvested. Then 5 individuals of each group were randomly selected and euthanatized by jugular venesection. The intestinal contents of jejunum, ileum and cecum carefully sampled, immediately frozen into liquid nitrogen and lately stored at −70°C. The abdominal fat tissue was isolated and weighed. The content of abdominal fat was calculated as its proportion of the eviscerated carcass weight. Meanwhile, for each duck, a 2-cm section of each intestinal segment and a piece of abdominal fat tissue were separated and submersed in 10% neutral-buffered formalin for 24 h of fixation.
Total genomic DNA of the sampled intestinal contents was extracted using QIAamp DNA isolation kit (Qiagen, Hilden, Germany). The concentration and integrity of bacterial DNA were assessed with a Nanodrop (Thermo Fisher Scientific, United States) and 1.5% agarose gel electrophoresis, respectively. The diluted DNA (1.0 ng/μL) was used to amplify the V4 hypervariable regions the 16S rRNA gene amplicons with the barcoded primers (515F, 5′-GTGCCAGCMGCCGCGGTAA-3′;806R, 5′-GGACTACHVGGGTWTCTAAT-3′) and Phusion® High-Fidelity PCR Master Mix with GC Buffer (New England Biolabs). The PCR products were subjected to 2.0% agarose gel electrophoresis, recovered, and purified using GeneJET Gel Extraction Kit (Thermo Fisher Scientific, United States). Sequencing libraries were generated using Ion Plus Fragment Library Kit (Thermo Fisher Scientific, United States) according to the manufacturer’s recommendations. Library quality was assessed on the Qubit@ 2.0 Fluorometer (Thermo Fisher Scientific, United States) and Agilent Bioanalyzer 2100 system. Finally, the library was sequenced on Ion S5TM XL platform (Thermo Fisher Scientific, United States).
The low-quality reads of the original data were filtered with Cutadapt (V1.9.1) (Martin, 2011), and the data of each sample was separated by barcode. Then the barcode and primer sequences were cut off to obtain the Raw Reads, which further went through alignment with Gold database1 (Haas et al., 2011) using UCHIME Algorithm (Edgar et al., 2011) to remove the chimeric sequences and turned out the Clean Reads. The Clean Reads were then clustered as operational taxonomic units (OTUs) by scripts of Uparse software (version 7.0.1001) with a 97% similarity threshold.
The representative OTUs (high frequency) were screened and annotated with SILVA SSUrRNA database using Mothur (threshold 0.8∼1.0) (Wang et al., 2007; Quast et al., 2012). Then MUSCLE software (version 3.8.31) was applied to perform the multiple sequence alignment of the OTUs to generate their phylogenetic relationship. And the OTU abundances were normalized using a standard of sequence numbers corresponding to the sample with the least sequences, based on which the diversity analysis were performed. To estimate of the microbial community of the samples, the within-sample alpha-diversity was calculated according to the genera profiles. Beta-diversity was estimated by calculating Unweighted-Unifrac and Weighted-Unifrac distances, then visualized with principal coordinate analysis (PCoA) and non-metric multi-dimensional scaling (NMDS). Kyoto Encyclopedia of Genes and Genomes (KEGG) pathway enrichment analysis was performed to predict the microbial functions. Linear discrimination analysis coupled with effect size (LEfSe) was performed to identify the bacterial taxa differentially represented between/among groups at different taxonomy levels (Segata et al., 2011). The Spearman correlation analysis was applied to analyze the associations of the differential microbial species with the measured parameters.
According reported methods (Murugesan et al., 2015; Varlamov et al., 2017), after fixation and dehydration, samples of different intestinal segments and abdominal tissue were embedded (EG1150H, LEIC, Germany) in paraffin wax, sliced (rotary microtome, RM2016, LEIC, Germany) and stained with hematoxylin and eosin in preparation for examination by microscope (S4E, LEIC, Germany), and image analyzer (Image-ProPlus 6.0). For each intestinal sample, 5 villus were selected for Villus height (VH), crypt depth (CD), mucosal thickness determination. For each adipose section, at least 3 vision fields were chosen and for each a certain range (containing 20–50 adipocytes) was circled out. Then the area and number of each range was measured with the image analyzer to calculate the average area and density of adipocytes.
The collected blood samples were firstly incubated at RT for 1∼2 h, centrifuged at 3,000 rpm, 4°C for 15 min to obtain serum, and then stored at −80°C until analysis. The energy metabolism and oxidative status related parameters of the collected serum samples were determined, including levels of glucose (GLU), triglyceride (TG), high density lipoprotein-cholesterol (HDL-C), low density lipoprotein-cholesterol (LDL-C), total cholesterol (T-CHO), malondialdehyde (MDA), superoxide dismutase (SOD), and total antioxidant capacity (T-AOC). All the determinations were performed on a Multifunctional Microplate Reader (Infinite® M200PRO, TECAN, Switzerland) with related commercial reagent kits (Jiancheng, Nanjing, China) according to the manufacturer’s recommendations. Index measurements of each sample were replicated 3 times.
Body weight, serum biochemical parameters, intestinal and adipose traits were presented as mean ± standard deviation (SD). T-test was applied with SPSS software (Version 20.0) to analyze the differences between group C and T. The results were considered significantly different at P < 0.05.
Under HS for 3 weeks, the average daily gain of Cherry-Valley ducks were measured significantly less than control (P = 0.0098), with a higher Feed/Gain ratio (P = 0.0467) (Table 2). However, the abdominal fat content significantly increased in T, compared to C (P = 0.0396) (Figure 1A). And the morphology results of abdominal fat tissue also showed higher single adipocyte area (P = 0.0321) and lower cell density (P = 0.0240) in thermal-treated birds (Figures 1B,C).
Figure 1. Influences of heat stress (HS) on abdominal fat deposition in duck (n = 5 for each group). C and T stand for control and thermal treatment, respectively. The abdominal fat content (A), average adipocyte area (B), and cell density (C) were calculated, and the statistical differences between C and T were analyzed (∗P < 0.05). The adipose tissue sections (400×) of C (D) and T (E) were also presented here.
For both groups, the morphological changes of jejunum, ileum, and cecum were presented in Table 3 and Figure 2. It showed that VHs in jejunum and ileum were both shorter in T, compared with C (P < 0.05). No significant differences of the CDs in all the three intestinal segments were found (P > 0.05). Then the VH/CD values were calculated higher in both jejunum and ileum of C (P < 0.05).
Figure 2. Tissue sections (100×) of intestinal mucosa in jejunum, ileum, and cecum in ducks with/without HS (n = 5 for each group). Cj, Ci, and Cc stand for the jejunum, ileum, and cecum of control. Tj, Ti, and Tc stand for the jejunum, ileum, and cecum of the thermal treated group, respectively. This also applies to the following figures.
The levels of serum Glu, TG, HDL-C, LDL-C, T-CHO, MDA, SOD, and T-AOC were determined and listed in Table 4. It indicated that compared to group C, the level of Glu was significantly higher (P = 0.0228), while the levels of TG, T-CHO, MDA, and T-AOC were all found significantly lower (P < 0.05) in group T. And no significant difference was detected on HDL-C, LDL-C or SOD between C and T (P > 0.05).
By 16S rRNA gene sequencing, the gut microbiota composition at phylum level of C (Cj, Cj, and Cc) and T (Tj, Ti, and Tc) was calculated (Figure 3A) and the dominant species were analyzed and shown in ternary plot (Figures 3B,C). It indicated that Firmicutes and Proteobacteria were major microbiota communities in Cj (mean, 84.5%), Ci (mean, 96.6%), Tj (mean, 84.7%), and Ti (mean, 91.3%). Firmicutes, Proteobacteria, Fusobacteria, and Bacteroidetes altogether comprised 98.0 and 98.9% of microbiota in Cc and Tc, respectively. Top 10 microbiota relative abundances at levels of class, order, family, and genus were also analyzed and presented in Supplementary Figure S1. The shared and specific OTUs in the two groups (Supplementary Figures S2A,B) and in the 3 segments with different treatments (Supplementary Figures S2C–E) were also calculated. The result of KEGG pathway analysis was listed in Figure 4.
Figure 3. Microbiota compositions in different intestinal segments of ducks with/without HS (n = 5 for each group). Top 10 bacterial phyla in jejunum, ileum and cecum of the used Cherry-Valley ducks in this study (A). And the ternary analysis of the 3 intestinal positions in group C and T are shown in (B,C), respectively.
Figure 4. The KEGG pathway enrichment analysis of the gut microbiota in ducks. The different colors represent the distance between the raw score and the mean population of the standard deviation.
Two indexes that reflecting species richness and diversity (Shannon and Simpson) decreased in Ci compared with Cc (P < 0.01) (Figure 5), while no significant difference was found between any other two segments in C or T. The Binary-Jaccard based PCoA results showed that the cecal microbiota was separated clearly from that in jejunum or ileum of both C and T (Adonis analysis, P < 0.05), and for both the 2 groups, the jejunum microbiota was well separated from that in ileum (Adonis analysis, P < 0.05) except for one sample spot (Figures 6A,B). To identify bacterial taxa that significantly differentiated among jejunum, ileum and cecum, a metagenomic biomarker discovery approach (LEfSe) was applied (LDA score >4) and the results were listed in Figures 6C,D. Moreover, The Binary-Jaccard based PCoA also showed that Cj displayed a distinct microbiota community that clustered separately from Tj (Adonis analysis, P < 0.05) (Figure 7A), while neither Ci-Ti nor Cc-Tc were separated into different clusters (Adonis analysis, P > 0.05) (Figures 7B,C). The NMDS analysis showed similar results to PCoA (Supplementary Figure S3). By LEfSe analysis, the differentially enriched microbiota (LDA score >4) at different classification levels were found in jejunum and cecum. Firmicutes (phylum), Bacilli (class), Lactobacillales (order), Lactobacillaceae (family), and Lactobacillus (genus) were more abundant in Cj. Proteobacteria (phylum), Pseudomonadales (order), Moraxellaceae (family), Acinetobacter (genus), and an unclassified member of Mitochondria (genus) were significantly enriched in Tj (Figure 7D). The relative abundances of Rickettsiales (order) and Mitochondria (family) markedly increased in Tc, while Negativicutes (class), and Selenomonadales (order) were more prevalent in Cc (Figure 7E). However, no significant differences of microbiota abundance were detected between Ci and Ti.
Figure 5. Bacterial species richness and diversity in the 3 intestinal segments of group C and T. The Shannon index and Simpson index were used to assess the species richness and diversity (∗∗P < 0.01).
Figure 6. Differential analysis of microbiota community among the 3 intestinal segments within group C and T. The principal coordinate analysis (PCoA) based on Binary-Jaccard distance was applied to observe the separation of the samples from C (A) and T (B). The Linear discrimination analysis (LDA) coupled with effect size (LEfSe) was used to identify the most differentially abundant taxa among the 3 intestinal segments within group C (C) and T (D). Only the results meeting an LDA significant threshold of >4 were shown.
Figure 7. Effects of HS on the microbiota compositions in the 3 intestinal segments. The Binary-Jaccard based PCoA analysis revealed the different effects of HS on the samples from jejunum (A), ileum (B), and cecum (C). The LEfSe analysis identified the differentially abundant (LDA score >4) bacterial species induced by HS in jejunum (D) and cecum (E), but not in ileum.
The Spearman correlation analysis results were presented in Figure 8. The results in jejunum showed that ADG and VH/CD were associated with phyla Firmicutes (positive, P < 0.05) and Proteobacteria (negative, P < 0.05), and Feed/Gain represented the opposite associations (P < 0.05). Abdominal fat content and serum GLU level were positively associated with genus Acinetobacter and negatively associated with phylum Firmicutes (P < 0.05). TG and T-CHO levels represented the opposite associations to GLU and abdominal fat content, and also negatively associated with phylum Proteobacteria, order Pseudomonadales, and family Moraxellacese (P < 0.05). Two positive associations and five negative associations were observed between serum MDA content and bacterial species (P < 0.05). T-AOC was positively associated with genus Lactobacillus (R = 0.47, P = 0.016), and negatively associated with order Pseudomonadales (R = −0.71, P = 0.022), genera Acinetobacter (R = −0.66, P = 0.039), and unidentified_Mitochondria (R = −0.59, P = 0.035). Whereas, no significant associations were found in cecum microbiota (P > 0.05).
Figure 8. The Spearman correlation analysis between the differential microbial species and measured parameters in jejunum (A) and cecum (B). ADG, average daily gain; AF, abdominal fat; Glu, glucose; TG, triglyceride; T-CHO, total cholesterol; MDA, malondialdehyde; T-AOC, total antioxidant; VH, villus height; CD, crypt depth. The red represents positive correlation and the blue represents negative correlation, respectively (∗P < 0.05, ∗∗P < 0.01).
To learn the gut microbiota community structure in Cherry-Valley duck and evaluate the effects of HS on it, a 16S rRNA sequencing analysis of the intestinal contents from jejunum, ileum, and cecum was performed in the current study. It has been reported that Firmicutes and Bacteroidetes were identified as the dominant phyla in avian gut microbiota, suggesting their importance in metabolism, and host physiology (Kohl, 2012). Firmicutes was the major phylum in feces of Canada geese (Lu et al., 2009) and Muscovy duck (Vasai et al., 2014a), whereas phylum Bacteroidetes was less enriched in some avian species (Lu et al., 2007). It is consistent with the present results that Firmicutes was the most abundant phylum in all the 3 selected intestinal segments, jejunum (45.4%), ileum (88.7%), and cecum (38.4%). In cecum, the second abundant phylum was Bacteroidetes (30.6%). And together with Fusobacteria (17.2%) and Proteobacteria (12.6%), the four phyla accounted for over 98% of the cecal microbiota composition of Cherry-Valley ducks under normal feeding conditions. However, instead of Bacteroidetes, the second most enriched bacterial phylum turned out to be Proteobacteria in both jejunum (39.0%), and ileum (7.9%). At class level, Clostridia and bacteroidia (obligate anaerobes) were reported to dominate in both ileum and cecum of mule ducks (Vasai et al., 2014b). Similar data could be found in this study (Supplementary Figure S1A) except little abundance of bacteroidia (0.44%) in ileum. Gut microbiota is associated with nutrient digestion/absorption and host protection, and these differences might be due to different avian species/breeds or intestinal positions.
In this study, experimental animals were exposed to high ambient temperature (32°C, 8 h per day) for 3 weeks to investigate the effects of HS on gut microbiota in different intestinal segments. Although with alteration of relative abundances, the dominant bacterial phyla in ileum and cecum were found just the same as in control. In jejunum, the major phylum came out to be Proteobacteria (72.7%) under HS, followed by Firmicutes (12.0%), the second most common phylum. This compositional reshape in microbial community indicated that among the 3 intestinal segments, the most significant impacts of HS on microbiota community probably located in jejunum. HS was reported to increase intestinal permeability, decrease intestinal blood circulation and further cause damages to its integrity, which were believed to affect the colonized microbial composition (Lambert, 2009; Song et al., 2014). Some morphological changes, including VH, VH/CD or intestinal sections, were observed in all the 3 intestinal segments. However, based on LEfSe analysis, the differentially enriched bacterial at different classification levels under HS were observed in jejunum and cecum (Figures 7D,E), while no significant differences were detected in ileum. It indicated that there might be some other factors involved in the intestinal microbiota changes under HS, e.g., different segments and original microbial composition.
For example, the Firmicutes abundance significantly decreased in jejunum when exposed to HS. Firmicutes is thought to be correlated with host energy metabolism. The increase of Firmicutes/Bacteroidetes ratio was considered to be a typical characteristic of obesity-driven dysbiosis in humans and animals (Mozes et al., 2008; Cotillard et al., 2013). Here, HS caused the significant decreases of duck growth performances and serum T-CHO level. T-CHO level reflects the interaction of lipid metabolism between liver and other tissues (Heyer and Lebret, 2007). And the correlation analysis showed that the abundance of jejunum Firmicutes was positively associated with ADG (R = 0.648, P = 0.043) and serum T-CHO level (R = 0.539, P = 0.034). It was consistent with previous studies. On the other hand, the negative association of phylum Proteobacteria abundance with ADG (R = −0.624, P = 0.029) was also found. The order selenomonadales was reported as an acetate producer (Vargas et al., 2017) which could induce secretion of ghrelin, a “hunger hormone,” and promote food intake (Perry et al., 2016). The abundance of selenomonadales in cecum was observed significantly decreased under HS. But the correlation analysis suggested that its association with ADG was not significant (R = 0.602, P = 0.054). These findings still needed to be further investigated. Moreover, it was found that the serum GLU level in duck significantly increased under HS. Analogs findings have been reported in Japanese quails (Ozbey and Ozcelik, 2004). It was probably regulated by hormones to satisfy the larger body energy demand to maintain a new physiological balance, e.g., nervous system, respiratory system and fat deposition.
Heat stress was demonstrated that could promote the differentiation of adipose tissues in cattle (Rhoads et al., 2009),swine (Kouba et al., 1999), and chicken (Baziz et al., 1996). HS could increase membrane fluidity and expressions of protective proteins (Hooper and Hooper, 2005; Jiang et al., 2007; Deng et al., 2017; Zhang et al., 2018). It would further improve the insulin resistance of adipose tissue and enhance its GLU uptake to promote the TG synthesis and storage (Yu et al., 2008; O’Brien et al., 2010; Hooper et al., 2014). It was believed to be a temporary body defense mechanism to HS. The data of the abdominal fat sections showed that the adipocytes in group T appeared more differentiated than in group C, which was consistent with previous studies. The correlation analysis (Figure 8A) found the increased abundance of genus Acinetobacter was positively associated with the abdominal fat content (R = 0.729, P = 0.017) and abdominal fat content and serum GLU level (R = 0.632, P = 0.049), and negatively associated with the serum TG level (R = −0.657, P = 0.039), respectively. Meanwhile, the reduced abundance of phylum Firmicutes represented the opposite associations to Acinetobacter. These data indicated that jejunum microbiota might contribute to the fat deposition in duck for defending again HS, although the mechanism still needed to be further explored.
Heat stress usually induces oxidation alteration (Slawinska et al., 2019), which is closely related to intestinal barrier integrity (Song et al., 2011). And gut microbiota is believed to be closely related with gut barrier (Yang et al., 2019; Paraskeuas and Mountzouris, 2019). MDA is a product of lipid oxidation. And the serum T-AOC index was measured using FeSO4 as standard substance by catalyzing the reduction of Fe3+-TPTZ (tripyridyl-triazine) to Fe2+-TPTZ. In the present study, the results of MDA and T-AOC indicated the decreased antioxidative capacity in duck induced by HS. The damaged intestinal mucosa, lower VH, and VH/CD values were observed in ducks under HS. The Spearman analysis showed that the MDA content and VH/CD value were significantly associated with the abundances of jejumun Firmicutes and Proteobacteria (Figure 8A). It also showed that the abundance of Lactobacillus was positively associated with the T-AOC (R = 0.47, P = 0.016). It was consistent with previous reports. Lactobacillus, a member of the class Bacilli (subordinate to Firmicutes phylum), has been reported negatively affected by HS in broilers (Al-Fataftah and Abdelqader, 2014; Zhang et al., 2017). Lactobacillus was proved to be of antioxidant activity (Lin and Chang, 2000; Lee et al., 2005b), and had the capacity of scavenging free radical and reactive oxygen species (ROS) to alleviate damages induced by oxidative stress (Lee et al., 2005a; Xin et al., 2014), which could be caused by HS (Altan et al., 2003). It indicated that the reduction of genus Lactobacillus probably weakened the antioxidant activity of duck under HS. However, its interaction with the other 3 bacterial species (negatively associated with T-AOC) was still unclear. Lactobacillus was also known as amylolytic bacteria and frequently increased when fed with high-starch diets in pigs (Regmi et al., 2011). No significant association was detected between Lactobacillus abundance and GLU content in the present study. It was probably due to different kind of stresses or animal species.
Lactobacillus consists of gram-positive and facultative anaerobes that produce lactic acid, which can create low pH environment and inhibit the growth of pathogen (Rodriguez-Cabezas et al., 2010). With the damaged intestinal integrity, the less abundance of Lactobacillus in jejunum of group T probably enlarged the risk of pathogen amplification and invasion. For example, order Pseudomonadales and family Moraxellaceae, containing known zoonotic pathogens (George, 2005), were detected significantly increased in jejunum under HS. With respect to cecum, the microbiota compositional variation also indicated some unfavorable influences of HS. The class negativicutes was demonstrated negatively associated with colonitis (Warner et al., 2016), which was observed significantly decreased under HS. Moreover, the order Rickettsiales, which contains major species that pathogenic to animals and human (Darby et al., 2007), was also found significantly increased in HS cecum. These information indicated that more attention should be paid to the prevention of pathogen invasion in HS ducks, which has been reported in HS broilers (Alhenaky et al., 2017).
After all, there are still massive works required to be further carried out on gut microbiota involved in HS ducks. For example, the effects of the obtained associated microbial species should be carefully and respectively, validated. Under HS, the interactions between duck gut microbiota and body regulatory factors needed to be further explored and discussed, e.g., inflammatory proteins, ghrelin, peroxisome proliferators-activated receptor γ (PPARγ) or mammalian target of rapamycin (mTOR).
The impacts of HS on gut microbiota community and their possible relationships with the physiological changes in Cherry-Valley ducks were investigated. The results showed that the significant microbiota compositional differences occurred in jejunum and cecum under HS, accompanied by the changes of weight gain, fat content, intestinal morphology, and oxidative indices. By Spearman correlation analysis, significant associations were found between microbiota alteration and these parameters in jejunum, but not in cecum. These data indicated that HS induced intestinal injuries, abnormal fat deposition, and reductions of growth performances and antioxidant capacity in duck, which probably have potential relationships with the gut microbiota dysbiosis.
This study was carried out in accordance with the recommendations of the Guidelines for Experimental Animals established by the Ministry of Science and Technology (Beijing, China). The protocol was approved by the Institutional Animal Care and Use Committee of Ningbo University (Ningbo, China).
DP and JC conceived of and designed the experiments. YH performed the sampling and serum index measurement. YS wrote the tissue section. XZ and JH performed the 16S rRNA sequencing experiments. JH, DP, and JC analyzed the data. JH wrote the manuscript. All authors contributed to refining the text and approved the submitted version.
This work was supported by National Natural Science Foundation of China (31801570), China Postdoctoral Science Foundation (2017M621894), and Modern Agricultural Technical Foundation of China (CARS-42-25).
The authors declare that the research was conducted in the absence of any commercial or financial relationships that could be construed as a potential conflict of interest.
Great thanks to Institute of Animal Husbandry and Veterinary Science, Zhejiang Academy Agricultural Sciences for their support of this research.
The Supplementary Material for this article can be found online at: https://www.frontiersin.org/articles/10.3389/fmicb.2019.00903/full#supplementary-material
Al-Fataftah, A. R., and Abdelqader, A. (2014). Effects of dietary Bacillus subtilis on heat-stressed broilers performance, intestinal morphology and microflora composition. Anim. Feed Sci. Tech. 198, 279–285. doi: 10.1016/j.anifeedsci.2014.10.012
Alhenaky, A., Abdelqader, A., Abuajamieh, M., and Al-fataftah, A. R. (2017). The effect of heat stress on intestinal integrity and Salmonella invasion in broiler birds. J. Therm. Biol. 70, 9–14. doi: 10.1016/j.jtherbio.2017.10.015
Altan, O., Pabuccuoglu, A., Altan, A., Konyalioglu, S., and Bayraktar, H. (2003). Effect of heat stress on oxidative stress, lipid peroxidation and some stress parameters in broilers. Brit. Poult. Sci. 44, 545–550. doi: 10.1080/00071660310001618334
Baziz, H. A., Geraert, P. A., Padilha, J. C. F., and Guillaumin, S. (1996). Chronic heat exposure enhances fat deposition and modifies muscle and fat partition in broiler carcasses. Poult. Sci. 75, 505–513. doi: 10.3382/ps.0750505
Burkholder, K. M., Thompson, K. L., Einstein, M. E., Applegate, T. J., and Patterson, J. A. (2008). Influence of stressors on normal intestinal microbiota, intestinal morphology, and susceptibility to Salmonella enteritidis colonization in broilers. Poult. Sci. 87, 1734–1741. doi: 10.3382/ps.2008-00107
Cotillard, A., Kennedy, S. P., Kong, L. C., Prifti, E., Pons, N., Le Chatelier, E., et al. (2013). Corrigendum: dietary intervention impact on gut microbial gene richness. Nature 502:580. doi: 10.1038/nature12738
Darby, A. C., Cho, N. H., Fuxelius, H. H., Westberg, J., and Andersson, S. G. (2007). Intracellular pathogens go extreme: genome evolution in the Rickettsiales. Trends Genet. 23, 511–520. doi: 10.1016/j.tig.2007.08.002
Deng, S. L., Sun, T. C., Yu, K., Wang, Z. P., Zhang, B. L., Zhang, Y., et al. (2017). Melatonin reduces oxidative damage and upregulates heat shock protein 90 expression in cryopreserved human semen. Free Radic. Biol. Med. 113, 347–354. doi: 10.1016/j.freeradbiomed.2017.10.342
Edgar, R. C., Haas, B. J., Clemente, J. C., Quince, C., and Knight, R. (2011). UCHIME improves sensitivity and speed of chimera detection. Bioinformatics 27, 2194–2200. doi: 10.1093/bioinformatics/btr381
Elphick, D. A. (2005). Paneth cells: their role in innate immunity and inflammatory disease. Gut 54, 1802–1809. doi: 10.1136/gut.2005.068601
Faylon, M. P., Baumgard, L. H., Rhoads, R. P., and Spurlock, D. M. (2015). Effects of acute heat stress on lipid metabolism of bovine primary adipocytes. J. Dairy Sci. 98, 8732–8740. doi: 10.3168/jds.2015-9692
George, M. G. (2005). Bergey’s Manual of Systematic Bacteriology, Vol. 2. New York, NY: Auflage Springer Press.
Ghasemian, M., and Jahanian, R. (2016). Dietary mannan-oligosaccharides supplementation could affect performance, immunocompetence, serum lipid metabolites, intestinal bacterial populations, and ileal nutrient digestibility in aged laying hens. Anim. Feed Sci. Tech. 213, 81–89. doi: 10.1016/j.anifeedsci.2015.12.012
Haas, B. J., Gevers, D., Earl, A. M., Feldgarden, M., Ward, D. V., Giannoukos, G., et al. (2011). Chimeric 16S rRNA sequence formation and detection in Sanger and 454-pyrosequenced PCR amplicons. Genome Res. 21, 494–504. doi: 10.1101/gr.112730.110
Hansen, J., Ruedy, R., Sato, M., and Lo, K. (2010). Global surface temperature change. Rev. Geophys. 48:RG4004. doi: 10.1029/2010rg000345
He, C., Cheng, D., Peng, C., Li, Y., Zhu, Y., and Lu, N. (2018). High-fat diet induces dysbiosis of gastric microbiota prior to gut microbiota in association with metabolic disorders in mice. Front. Microbiol. 9:639. doi: 10.3389/fmicb.2018.00639
Heyer, A., and Lebret, B. (2007). Compensatory growth response in pigs: effect on growth performance, composition of weight gain at carcass and muscle levels, and meat quality. J. Anim. Sci. 85, 769–778. doi: 10.2527/jas.2006-164
Hooper, P. L., Balogh, G., Rivas, E., Kavanagh, K., and Vigh, L. (2014). The importance of the cellular stress response in the pathogenesis and treatment of type 2 diabetes. Cell Stress Chaperon. 19, 447–464. doi: 10.1007/s12192-014-0493-8
Hooper, P. L., and Hooper, J. J. (2005). Loss of defense against stress: diabetes and heat shock proteins. Diabetes Technol. Ther. 7, 204–208. doi: 10.1089/dia.2005.7.204
Humphrey, T. (2006). Are happy chickens safer chickens? Poultry welfare and disease susceptibility. Brit. Poult. Sci. 47, 379–391. doi: 10.1080/00071660600829084
Jiang, H., He, J., Pu, S., Tang, C., and Xu, G. (2007). Heat shock protein 70 is translocated to lipid droplets in rat adipocytes upon heat stimulation. Biochim. Biophys. Acta 1771, 66–74. doi: 10.1016/j.bbalip.2006.10.004
Jiang, J. F., Song, X. M., Wu, J. L., and Jiang, Y. Q. (2014). Effects of alfalfa meal on the intestinal microbial diversity and immunity of growing ducks. J. Anim. Physiol. Anim. Nutr. 98, 1039–1046. doi: 10.1111/jpn.12167
Kers, J. G., Velkers, F. C., Fischer, E. A. J., Hermes, G. D. A., Stegeman, J. A., and Smidt, H. (2018). Host and environmental factors affecting the intestinal microbiota in chickens. Front. Microbiol. 9:235. doi: 10.3389/fmicb.2018.00235
Kohl, K. D. (2012). Diversity and function of the avian gut microbiota. J. Comp. Physiol. B 182, 591–602. doi: 10.1007/s00360-012-0645-z
Kouba, M., Hermier, D., and Le Dividich, J. (1999). Influence of a high ambient temperature on stearoyl-CoA-desaturase activity in the growing pig. Comp. Biochem. Physiol. B 124, 7–13. doi: 10.1016/S0305-0491(99)00090-5
Küchenmeister, U., Nürnberg, K., Fiedler, I., Kuhn, G., Nürnberg, G., and Ender, K. (1999). Cell injury and meat quality of pig in the time period post mortem from two genotypes susceptible or resistant to malignant hyperthermia. Eur. Food Res. Technol. 209, 97–103. doi: 10.1007/s002170050465
Lambert, G. P. (2009). Stress-induced gastrointestinal barrier dysfunction and its inflammatory effects. J. Anim. Sci. 87, E101–E108. doi: 10.2527/jas.2008-1339
Lara, L. J., and Rostagno, M. H. (2013). Impact of heat stress on poultry production. Animals 3, 356–369. doi: 10.3390/ani3020356
Lee, J., Hwang, K. T., Chung, M. Y., Cho, D. H., and Park, C. S. (2005a). Resistance of Lactobacillus casei KCTC 3260 to reactive oxygen species (ROS): role for a metal ion chelating effect. J. Food Sci. 70, m388–m391. doi: 10.1111/j.1365-2621.2005.tb11524.x
Lee, J., Hwang, K. T., Heo, M. S., Lee, J. H., and Park, K. Y. (2005b). Resistance of Lactobacillus plantarum KCTC 3099 from kimchi to oxidative stress. J. Med. Food 8, 299–304. doi: 10.1089/jmf.2005.8.299
Leser, T. D., and Molbak, L. (2009). Better living through microbial action: the benefits of the mammalian gastrointestinal microbiota on the host. Environ. Microbiol. 11, 2194–2206. doi: 10.1111/j.1462-2920.2009.01941.x
Lin, M. Y., and Chang, F. J. (2000). Antioxidative effect of intestinal bacteria Bifidobacterium longum ATCC 15708 and Lactobacillus acidophilus ATCC 4356. Dig. Dis. Sci. 45, 1617–1622. doi: 10.1023/A:1005577330695
Liu, F., Yin, J., Du, M., Yan, P., Xu, J., Zhu, X., et al. (2009). Heat-stress-induced damage to porcine small intestinal epithelium associated with downregulation of epithelial growth factor signaling. J. Anim. Sci. 87, 1941–1949. doi: 10.2527/jas.2008-1624
Lu, J., Santo Domingo, J., and Shanks, O. C. (2007). Identification of chicken-specific fecal microbial sequences using a metagenomic approach. Water Res. 41, 3561–3574. doi: 10.1016/j.watres.2007.05.033
Lu, J., Santo Domingo, J. W., Hill, S., and Edge, T. A. (2009). Microbial diversity and host-specific sequences of Canada goose feces. Appl. Environ. Microbiol. 75, 5919–5926. doi: 10.1128/AEM.00462-09
Mack, L. A., Felver-Gant, J. N., Dennis, R. L., and Cheng, H. W. (2013). Genetic variations alter production and behavioral responses following heat stress in 2 strains of laying hens. Poult. Sci. 92, 285–294. doi: 10.3382/ps.2012-02589
Martin, M. (2011). Cutadapt removes adapter sequences from high-throughput sequencing reads. EMBnet J. 17, 10–12. doi: 10.14806/ej.17.1.200
Moeser, A. J., Klok, C. V., Ryan, K. A., Wooten, J. G., Little, D., Cook, V. L., et al. (2007). Stress signaling pathways activated by weaning mediate intestinal dysfunction in the pig. Am. J. Physiol. Gastrointest. Liver Physiol. 292, G173–G181. doi: 10.1152/ajpgi.00197.2006
Mozes, S., Bujnakova, D., Sefcikova, Z., and Kmet, V. (2008). Developmental changes of gut microflora and enzyme activity in rat pups exposed to fat-rich diet. Obesity 16, 2610–2615. doi: 10.1038/oby.2008.435
Murugesan, G. R., Syed, B., Haldar, S., and Pender, C. (2015). Phytogenic feed additives as an alternative to antibiotic growth promoters in broiler chickens. Front. Vet. Sci. 2:21. doi: 10.3389/fvets.2015.00021
Nawab, A., Ibtisham, F., Li, G., Kieser, B., Wu, J., Liu, W., et al. (2018). Heat stress in poultry production: mitigation strategies to overcome the future challenges facing the global poultry industry. J. Therm. Biol. 78, 131–139. doi: 10.1016/j.jtherbio.2018.08.010
O’Brien, M. D., Rhoads, R. P., Sanders, S. R., Duff, G. C., and Baumgard, L. H. (2010). Metabolic adaptations to heat stress in growing cattle. Domest. Anim. Endocrinol. 38, 86–94. doi: 10.1016/j.domaniend.2009.08.005
Ozbey, O., and Ozcelik, M. (2004). The effect of high environmental temperature on growth performance of japanese quails with different body weights. Int. J. Poult. Sci. 3, 468–470. doi: 10.3923/ijps.2004.468.470
Paraskeuas, V., and Mountzouris, K. C. (2019). Broiler gut microbiota and expressions of gut barrier genes affected by cereal type and phytogenic inclusion. Anim. Nutr. 5, 22–31. doi: 10.1016/j.aninu.2018.11.002
Perry, R. J., Peng, L., Barry, N. A., Cline, G. W., Zhang, D., Cardone, R. L., et al. (2016). Acetate mediates a microbiome-brain-beta-cell axis to promote metabolic syndrome. Nature 534, 213–217. doi: 10.1038/nature18309
Quast, C., Pruesse, E., Yilmaz, P., Gerken, J., Schweer, T., Yarza, P., et al. (2012). The SILVA ribosomal RNA gene database project: improved data processing and web-based tools. Nucleic Acids Res. 41, D590–D596. doi: 10.1093/nar/gks1219
Regmi, P. R., Metzler-Zebeli, B. U., Ganzle, M. G., Van Kempen, T. A., and Zijlstra, R. T. (2011). Starch with high amylose content and low in vitro digestibility increases intestinal nutrient flow and microbial fermentation and selectively promotes bifidobacteria in pigs. J. Nutr. 141, 1273–1280. doi: 10.3945/jn.111.140509
Rhoads, M. L., Rhoads, R. P., Vanbaale, M. J., Collier, R. J., Sanders, S. R., Weber, W. J., et al. (2009). Effects of heat stress and plane of nutrition on lactating Holstein cows: I. Production, metabolism, and aspects of circulating somatotropin. J. Dairy Sci. 92, 1986–1997. doi: 10.3168/jds.2008-1641
Rodriguez-Cabezas, M. E., Camuesco, D., Arribas, B., Garrido-Mesa, N., Comalada, M., Bailon, E., et al. (2010). The combination of fructooligosaccharides and resistant starch shows prebiotic additive effects in rats. Clin. Nutr. 29, 832–839. doi: 10.1016/j.clnu.2010.05.005
Segata, N., Izard, J., Waldron, L., Gevers, D., Miropolsky, L., Garrett, W. S., et al. (2011). Metagenomic biomarker discovery and explanation. Genome Biol. 12:R60. doi: 10.1186/gb-2011-12-6-r60
Slawinska, A., Mendes, S., Dunislawska, A., Siwek, M., Zampiga, M., Sirri, F., et al. (2019). Avian model to mitigate gut-derived immune response and oxidative stress during heat. Biosystems 178, 10–15. doi: 10.1016/j.biosystems.2019.01.007
Sohail, M. U., Ijaz, A., Yousaf, M. S., Ashraf, K., Zaneb, H., Aleem, M., et al. (2010). Alleviation of cyclic heat stress in broilers by dietary supplementation of mannan-oligosaccharide and Lactobacillus-based probiotic: dynamics of cortisol, thyroid hormones, cholesterol, C-reactive protein, and humoral immunity. Poult. Sci. 89, 1934–1938. doi: 10.3382/ps.2010-00751
Song, J., Jiao, L. F., Xiao, K., Luan, Z. S., Hu, C. H., Shi, B., et al. (2013). Cello-oligosaccharide ameliorates heat stress-induced impairment of intestinal microflora, morphology and barrier integrity in broilers. Anim. Feed Sci. Tech. 185, 175–181. doi: 10.1016/j.anifeedsci.2013.08.001
Song, J., Xiao, K., Ke, Y. L., Jiao, L. F., Hu, C. H., Diao, Q. Y., et al. (2014). Effect of a probiotic mixture on intestinal microflora, morphology, and barrier integrity of broilers subjected to heat stress. Poult. Sci. 93, 581–588. doi: 10.3382/ps.2013-03455
Song, P., Zhang, R., Wang, X., He, P., Tan, L., and Ma, X. (2011). Dietary grape-seed procyanidins decreased postweaning diarrhea by modulating intestinal permeability and suppressing oxidative stress in rats. J. Agric. Food Chem. 59, 6227–6232. doi: 10.1021/jf200120y
Song, Z., Cheng, K., Zhang, L., and Wang, T. (2017). Dietary supplementation of enzymatically treated Artemisia annua could alleviate the intestinal inflammatory response in heat-stressed broilers. J. Therm. Biol. 69, 184–190. doi: 10.1016/j.jtherbio.2017.07.015
St-Pierre, N. R., Cobanov, B., and Schnitkey, G. (2003). Economic losses from heat stress by US livestock industries. J. Dairy Sci. 86, E52–E77. doi: 10.3168/jds.S0022-0302(03)74040-5
Teeter, R. G., Smith, M. O., Owens, F. N., Arp, S. C., Sangiah, S., and Breazile, J. E. (1985). Chronic heat stress and respiratory alkalosis: occurrence and treatment in broiler chicks. Poult. Sci. 64, 1060–1064. doi: 10.3382/ps.0641060
Vargas, J. E., Andres, S., Snelling, T. J., Lopez-Ferreras, L., Yanez-Ruiz, D. R., Garcia-Estrada, C., et al. (2017). Effect of sunflower and marine oils on ruminal microbiota, in vitro fermentation and digesta fatty acid profile. Front. Microbiol. 8:1124. doi: 10.3389/fmicb.2017.01124
Varlamov, O., Bishop, C. V., Handu, M., Takahashi, D., Srinvasan, S., White, A., et al. (2017). Combined androgen excess and western-style diet accelerates adipose tissue dysfunction in young adult, female nonhuman primates. Hum. Reprod. 32, 1892–1902. doi: 10.1093/humrep/dex244
Vasai, F., Brugirard Ricaud, K., Bernadet, M. D., Cauquil, L., Bouchez, O., Combes, S., et al. (2014a). Overfeeding and genetics affect the composition of intestinal microbiota in Anas platyrhynchos (Pekin) and Cairina moschata (Muscovy) ducks. FEMS Microbiol. Ecol. 87, 204–216. doi: 10.1111/1574-6941.12217
Vasai, F., Ricaud, K. B., Cauquil, L., Daniel, P., Peillod, C., Gontier, K., et al. (2014b). Lactobacillus sakei modulates mule duck microbiota in ileum and ceca during overfeeding. Poult. Sci. 93, 916–925. doi: 10.3382/ps.2013-03497
Wang, Q., Garrity, G. M., Tiedje, J. M., and Cole, J. R. (2007). Naive bayesian classifier for rapid assignment of rRNA sequences into the new bacterial taxonomy. Appl. Environ. Microb. 73, 5261–5267. doi: 10.1128/aem.00062-07
Wang, X., Shen, M., Zhou, J., and Jin, Y. (2019). Chlorpyrifos disturbs hepatic metabolism associated with oxidative stress and gut microbiota dysbiosis in adult zebrafish. Comp. Biochem. Phys. C Toxicol. Pharmacol. 216, 19–28. doi: 10.1016/j.cbpc.2018.11.010
Warner, B. B., Deych, E., Zhou, Y., Hall-Moore, C., Weinstock, G. M., Sodergren, E., et al. (2016). Gut bacteria dysbiosis and necrotising enterocolitis in very low birthweight infants: a prospective case-control study. Lancet 387, 1928–1936. doi: 10.1016/s0140-6736(16)00081-7
Whiteside, S. A., Razvi, H., Dave, S., Reid, G., and Burton, J. P. (2015). The microbiome of the urinary tract–a role beyond infection. Nat. Rev. Urol. 12, 81–90. doi: 10.1038/nrurol.2014.361
Xin, J., Zeng, D., Wang, H., Ni, X., Yi, D., Pan, K., et al. (2014). Preventing non-alcoholic fatty liver disease through Lactobacillus johnsonii BS15 by attenuating inflammation and mitochondrial injury and improving gut environment in obese mice. Appl. Microbiol. Biot. 98, 1–13. doi: 10.1007/s00253-014-5752-1
Yang, C., Deng, Q., Xu, J., Wang, X., Hu, C., Tang, H., et al. (2019). Sinapic acid and resveratrol alleviate oxidative stress with modulation of gut microbiota in high-fat diet-fed rats. Food Res. Int. 116, 1202–1211. doi: 10.1016/j.foodres.2018.10.003
Yi, D., Hou, Y., Tan, L., Liao, M., Xie, J., Wang, L., et al. (2016). N -acetylcysteine improves the growth performance and intestinal function in the heat-stressed broilers. Anim. Feed Sci. Tech. 220, 83–92. doi: 10.1016/j.anifeedsci.2016.07.014
Yu, W., Chen, Z., Zhang, J., Zhang, L., Ke, H., Huang, L., et al. (2008). Critical role of phosphoinositide 3-kinase cascade in adipogenesis of human mesenchymal stem cells. Mol. Cell. Biochem. 310, 11–18. doi: 10.1007/s11010-007-9661-9
Zhang, C., Zhao, X. H., Yang, L., Chen, X. Y., Jiang, R. S., Jin, S. H., et al. (2017). Resveratrol alleviates heat stress-induced impairment of intestinal morphology, microflora, and barrier integrity in broilers. Poult. Sci. 96, 4325–4332. doi: 10.3382/ps/pex266
Keywords: gut microbiota, heat stress, intestinal injury, antioxidant capacity, 16S rRNA, Cherry-Valley duck
Citation: He J, He Y, Pan D, Cao J, Sun Y and Zeng X (2019) Associations of Gut Microbiota With Heat Stress-Induced Changes of Growth, Fat Deposition, Intestinal Morphology, and Antioxidant Capacity in Ducks. Front. Microbiol. 10:903. doi: 10.3389/fmicb.2019.00903
Received: 05 September 2018; Accepted: 09 April 2019;
Published: 26 April 2019.
Edited by:
Aldo Corsetti, University of Teramo, ItalyReviewed by:
Ana Cláudia Coelho, University of Trás-os-Montes and Alto Douro, PortugalCopyright © 2019 He, He, Pan, Cao, Sun and Zeng. This is an open-access article distributed under the terms of the Creative Commons Attribution License (CC BY). The use, distribution or reproduction in other forums is permitted, provided the original author(s) and the copyright owner(s) are credited and that the original publication in this journal is cited, in accordance with accepted academic practice. No use, distribution or reproduction is permitted which does not comply with these terms.
*Correspondence: Daodong Pan, ZGFvZG9uZ3BhbkAxNjMuY29t
Disclaimer: All claims expressed in this article are solely those of the authors and do not necessarily represent those of their affiliated organizations, or those of the publisher, the editors and the reviewers. Any product that may be evaluated in this article or claim that may be made by its manufacturer is not guaranteed or endorsed by the publisher.
Research integrity at Frontiers
Learn more about the work of our research integrity team to safeguard the quality of each article we publish.