- 1Department of Bioorganic Chemistry, Max Planck Institute for Chemical Ecology, Jena, Germany
- 2Department of Plant Physiology, Matthias Schleiden Institute of Genetics, Bioinformatics and Molecular Botany, Friedrich-Schiller-University Jena, Jena, Germany
- 3Research Group Plant Defense Physiology, Max Planck Institute for Chemical Ecology, Jena, Germany
- 4Department of Genetics, Matthias Schleiden Institute of Genetics, Bioinformatics and Molecular Botany, Friedrich-Schiller-University Jena, Jena, Germany
- 5Department of Evolutionary Neuroethology, Max Planck Institute for Chemical Ecology, Jena, Germany
Auxin (indole-3-acetic acid, IAA) is an important phytohormone involved in root growth and development. Root-interacting beneficial and pathogenic fungi utilize auxin and its target genes to manipulate the performance of their hosts for their own needs. In order to follow and visualize auxin effects in fungi-colonized Arabidopsis roots, we used the dual auxin reporter construct DR5::EGFP-DR5v2::tdTomato and fluorescence microscopy as well as LC-MS-based phytohormone analyses. We demonstrate that the beneficial endophytic fungi Piriformospora indica and Mortierella hyalina produce and accumulate IAA in their mycelia, in contrast to the phytopathogenic biotrophic fungus Verticillium dahliae and the necrotrophic fungus Alternaria brassicicola. Within 3 h after exposure of Arabidopsis roots to the pathogens, the signals of the auxin-responsive reporter genes disappeared. When exposed to P. indica, significantly higher auxin levels and stimulated expression of auxin-responsive reporter genes were detected both in lateral root primordia and the root elongation zone within 1 day. Elevated auxin levels were also present in the M. hyalina/Arabidopsis root interaction, but no downstream effects on auxin-responsive reporter genes were observed. However, the jasmonate level was strongly increased in the colonized roots. We propose that the lack of stimulated root growth upon infection with M. hyalina is not caused by the absence of auxin, but an inhibitory effect mediated by high jasmonate content.
Introduction
Auxin plays a central role for root growth and participates in many aspects of root development, including cell elongation, differentiation (Rahman et al., 2007), lateral root, and root hair formation (Masucci and Schiefelbein, 1994, 1996; Pitts et al., 1998; Reed et al., 1998; Casimiro et al., 2001; Bhalerao et al., 2002), and gravitropic responses (Rashotte et al., 2000; Sukumar et al., 2009). Auxin action depends on its differential distribution within plant tissues, where it forms local maxima or gradients between cells. The different auxin levels arise from auxin metabolism (biosynthesis, degradation, and conjugation), long-distance transport and directional cell-to-cell translocation (Petrášek and Friml, 2009). Auxin interacts with other phytohormones and imbalances in the phytohormone levels have severe consequences. Well-studied examples are the auxin/cytokinin balance (De Rybel et al., 2014) and the control of lateral root growth by an interplay between auxin, abscisic acid, brassinosteroids, ethylene, and jasmonates (cf. Fukaki and Tasaka, 2009; Ishimaru et al., 2018). In addition, auxin produced by plant-associated microorganisms mediates phytostimulatory effects on plants. Pathogens can manipulate auxin biosynthesis, signaling, and transport pathways to promote host susceptibility. Auxin responses are also coupled to antagonistic and synergistic interactions with salicylic acid (SA)- and jasmonic acid (JA)-mediated defenses, respectively (Hoffmann et al., 2011; Naseem et al., 2015; Huang et al., 2017). Jasmonates participate in the regulation of primary root growth and reproductive development, thereby interacting with auxins. Crosstalk can occur at multiple levels, including hormone perception, since indole-acetic acid (IAA) and JA-isoleucine (JA-Ile) are perceived by SCF E3-ligases through the interaction of IAA- and JA-related regulators of gene expression and the modulation of each other's homeostasis (Hoffmann et al., 2011; Naseem et al., 2015). Auxin induces JA biosynthesis and JA controls the expression of some of the auxin biosynthetic genes (Dombrecht et al., 2007; Sun et al., 2009; Hentrich et al., 2013). Furthermore, high JA concentrations reduce the accumulation of the PIN-FORMED1 (PIN1) and PIN2 auxin transporters (Gutjahr et al., 2005; Hoffmann et al., 2011; Sun et al., 2011). IAA binds to the receptor AUXIN SIGNALING F-BOX PROTEIN TIR1 and inhibits a family of transcriptional repressors known as AUXIN/IAAs (Dharmasiri et al., 2005; Kepinski and Leyser, 2005; Salehin et al., 2015). In the presence of IAA, the SKP1-CULLIN1-F-BOX TIR1 ubiquitin ligase complex binds to AUXIN/IAAs and triggers their degradation (Calderon-Villalobos et al., 2010).
The vast majority of roots in the ecosystems is associated with beneficial fungi. They form mycorrhizal symbiosis or interact with endophytes. Plants benefit from these associations in many ways, such as better access to water and nutrients, promotion of growth and biomass production and resistance to biotic and abiotic stress. The fungi are supplied with reduced carbon from the host photosynthesis and live in a protected shelter. The beneficial symbiosis results in alterations of the host phytohormone levels. For example, beneficial microbes synthesize auxin or auxin precursors, interfere with the plant auxin biosynthesis and metabolism or manipulate auxin signaling and responses. In many cases, the microbes utilize the plant phytohormone machinery and reprogram it to their own needs (Xu et al., 2018, and references therein).
In this study, we used the previously described auxin-responsive reporter system DR5::EGFP-DR5v2::tdTomato (Ulmasov et al., 1997; Liao et al., 2015) to monitor how the root-interacting microbes Piriformospora indica, Mortierella hyalina, Verticillium dahlia, and Alternaria brassicicola manipulate the root auxin metabolism during early phases of the interaction with Arabidopsis roots. P. indica, a member of Sebacinales, grows inter- and intracellularly and forms pear shaped spores, which accumulate within the roots and on the root surface (Peškan-Berghöfer et al., 2004; Oelmüller et al., 2009; Camehl et al., 2011). The fungus promotes the growth of the host plants (Peškan-Berghöfer et al., 2004), induces early flowering (Pan et al., 2017) and confers resistance against abiotic and biotic stress (Narayan et al., 2017; Zhang et al., 2017; Vahabi et al., 2018). The endophyte produces indole derivatives but they are not required for growth promotion in barley roots (Hilbert et al., 2012). P. indica releases cellotriose that induces root-specific [Ca2+]cyt elevation required for the activation of a mild defense response (Vadassery et al., 2008; Johnson et al., 2018a; Oelmüller, 2018). Root-specific [Ca2+]cyt elevation is also induced by an exuded info-chemical from M. hyalina (Johnson et al., 2018b), a growth promoting fungus of Mortierellales (Shinmen et al., 1989) which results in defense gene activation. The fungus promotes growth of the aerial parts of Arabidopsis via a fungus-released volatile, while the growth behavior of colonized roots resembles that of un-colonized controls (Johnson et al., 2018b). V. dahliae is hemibiotroph with an initial biotrophic life phase in the root xylem, followed by a necrotrophic phase once the hyphae reach the aerial plant tissues. While infected roots show little or no disease symptom development during the biotrophic phase, the fungus blocks xylem transport and synthesizes a cocktail of toxins during the necrotrophic phase, which results in wilting and disease symptom development in the leaves of Brassicaceae species (Pemberton and Salmond, 2004; Gijzen and Nürnberger, 2006; Qutob et al., 2006; van der Does and Rep, 2007; Bolton et al., 2008; de Jonge and Thomma, 2009; van Esse et al., 2009; de Jonge et al., 2010, 2011; Oliva et al., 2010; Klosterman et al., 2011; Scholz et al., 2018). The necrotrophic fungus Alternaria causes black leaf spot disease in crucifers and was used in this study since it infects both leaves and roots. Root infection induces rapid [Ca2+]cyt elevations which results in host defense gene activation, jasmonate accumulation, ROS production and innate immunity. A few days after root infection, the Arabidopsis seedlings are dead (Johnson et al., 2018b).
In this study, we addressed the hypothesis that interactions of Arabidopsis roots with pathogenic and beneficial fungi are accompanied by different activation of auxin-responsive genes during very early phases of contact. Our results suggest that the activation of auxin-responsive genes and auxin-induced developmental programs in the colonized roots are controlled by the phytohormone balance, rather than by the auxin concentration alone.
Material and Methods
Plant Material and Growth Conditions
Seedlings of Arabidopsis thaliana containing the dual auxin reporter construct DR5::EGFP-DR5v2::tdTomato were generally described in Ulmasov et al. (1997) and Liao et al. (2015). Here, plants containing T-DNA comprising construct pGWB601-DR5::EGFP-DR5v2::tdTomato were used, which had been generated as described (Kirbis, 2017). The seeds were surface-sterilized and placed on petri dishes containing full Murashige-Skoog nutrient medium (MS) (Murashige and Skoog, 1962) supplemented with 40 mM sucrose, 2.6 mM 2-(N-morpholino)ethanesulfonic acid and 1% Kobe Agar (all supplies from Carl Roth, Karlsruhe, Germany). After a 48 h stratification at 4°C, plates were incubated vertically for 10 days at 22°C under long day conditions (16 h light/ 8 h dark; 80 μmol m−2 s−1).
Fungal Material and Growth Conditions
P. indica (syn. Serindipita indica) was grown on petri dishes with modified Kaefer's medium (KM) as previously described (Verma et al., 1998; Peškan-Berghöfer et al., 2004) and kept in the dark at room temperature for 3 weeks. A. brassicicola, M. hyalina, and V. dahliae were cultivated for 2 weeks at 22 ± 1°C on potato dextrose agar (PDA) medium as reported in Johnson et al. (2014). A. brassicicola, M. hyalina, and V. dahliae were obtained from the Jena Microbial Resource Center and P. indica was provided by Ajit Varma (Amity Institute of Microbial Technology, India).
Spore suspensions of the fungi were prepared according to Johnson et al. (2014) by rinsing the plates containing the fungi with sterile H2O, gently scraping the spores and hyphae off the plate followed by filtration through a sterilized nylon membrane (75 μm pore size). The spore concentration was determined using a hemocytometer and adjusted with sterile H2O containing 0.01 % Tween-20 to 2 × 106 spores/ml. As control the same solution was used without spores. Viability of the spores was routinely checked via germination tests.
Co-cultivation for Fluorescence Microscopy
In order to investigate the impact of different fungi on the distribution of auxin maxima, Arabidopsis seedlings containing the reporter construct pGWB601-DR5::EGFP-DR5v2::tdTomato were co-cultivated with fungal plaques of P. indica, A. brassicicola, M. hyalina, or V. dahliae as described previously by Johnson et al. (2011, 2014) with modifications. For co-cultivation with subsequent fluorescence microscopy, 12 day-old plants were placed on microscope slides on top of a thin layer of full MS medium containing 1% Kobe agar and a plaque (5 mm diameter) of medium (PDA or KM, for control), or fungus agar cultures were applied (see Figure 1A). The seedling and fungal plug were coated with sterile tap water, covered with a cover slip and placed in a petri dish until microscopy was performed. The plates were then sealed with Parafilm (American National Can Company, Greenwich, USA) to prevent drying out of the sample and kept at 22°C under long day conditions (16 h light/8 h dark; 80 μmol m−2 s−1) for 1 to 3 days. The fluorescence of the reporter construct was analyzed by fluorescence microscopy (see below) after 24 h of incubation. To analyze the dose-dependent induction of fluorescence, the MS agar was supplemented with 0, 5, 10, or 20 μM of IAA (Merck, Darmstadt, Germany) or 0, 1, 5, or 10 μM of JA (Sigma-Aldrich, Taufkirchen, Germany).
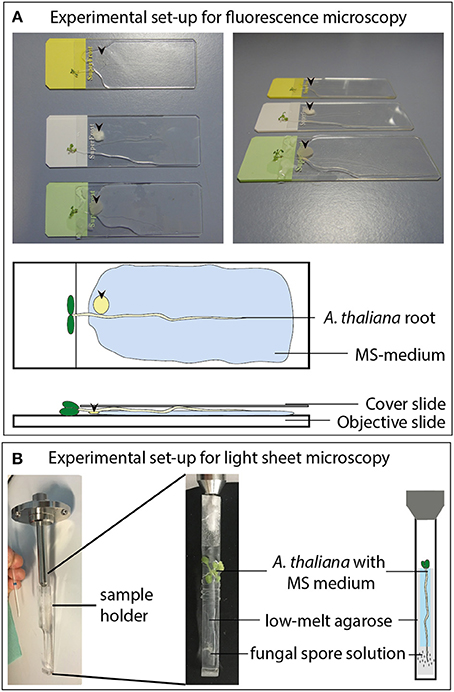
Figure 1. Experimental set-up for the fluorescence imaging measurements. (A) Co-cultivation of A. thaliana seedlings with different fungi on objective slides for fluorescence microscopy. Arrow heads indicate the position of the fungal plaques. (B) Co-cultivation of A. thaliana roots with fungal spore solutions (2 μl of 2 × 106 spores per ml) for light sheet microscopy.
Fluorescence Microscopy
The entire Arabidopsis roots, elongation zones, root tips and primordia were imaged using an AXIO Imager.M2 (Zeiss Microscopy GmbH, Jena, Germany) equipped with a 10x objective (N-Achroplan 10x/0.3). The bright field and fluorescence images (EX 545/25 and EM 605/70) were recorded with a color camera (AXIOCAM 503 color Zeiss, Jena, Germany) by use of an EGFP (EM 525/50 nm) and DsRED filter (EM 605/70 nm). Digital images were processed with the ZEN software (Zeiss, Jena, Germany), treated with Adobe® PhotoShop to optimize brightness, contrast and coloring and to overlay the photomicrographs. The quantification of fluorescence was measured using the ZEN software by analyzing a region of interest at the root tip and/or the whole root.
Light Sheet Fluorescence Microscopy
Twelve day-old Arabidopsis seedlings (grown as described above) were mounted on a custom plastic holder (see Figure 1B). Afterwards the root tip was infected with 2 μl of a P. indica, A. brassicicola, M. hyalina, or V. dahliae spore suspension (2 × 106 spores/ml) or sterile water containing 0.01 % Tween-20 as a control. The whole plant was fixed using 2% low melting agarose (Carl Roth, Karlsruhe, Germany) and Parafilm to prevent unspecific movement (see Figure 1B). The measuring chamber was flooded with full Murashige-Skoog nutrient medium to ensure optimal nutrient supply during the whole measurement. Time series of the root response to the spore treatments were recorded on a LightSheet.Z1 (ZEISS, Oberkochen, Germany) equipped with a W Plan Apochromat 20x/1.0 UV-VIS (ZEISS, Oberkochen, Germany) and two lasers (laser lines: Argon 488 nm and Helium-Neon 561 nm). The red fluorescence was visualized using the 561 nm Helium-Neon laser at 20% transmission with a LBF 405/488/561 emission filter BP 575-615. For all recordings an exposure time of 350 ms was used at a zoom of 0.36 and a light sheet thickness of 6.68 μm. Image stacks series were acquired at 1,920·pixels, 20 μm z-thickness and 16 bit every 30 min for 24 h. Representative intensity kinetics were generated with the ZEN software (Zeiss, Jena, Germany).
Co-cultivation for Phytohormone Analyses
For phytohormone analyses, 15 Arabidopsis seedlings were positioned on a full MS square plate on a sterilized nylon membrane, stratified for 2 days at 4°C and vertically grown for 10 days at 22°C under long day conditions (16 h light/8 h dark; 80 μmol m−2 s−1). In accordance with the previous plaque treatment, a 5 mm broad stripe of PDA medium with or without (control) fungal mycelium of A. brassicicola, M. hyalina, or V. dahliae was placed on the roots and incubated for 1 day. For co-cultivation with P. indica, a stripe of KM medium with or without fungus was used for treatment. The plates were co-cultured for 24 h before the entire root of each plate was collected, immediately frozen in liquid nitrogen and stored at −80°C before phytohormone extraction. Mycelium from each fungus grown alone was additionally collected from a whole plate, frozen and kept at −80°C.
Quantification of Phytohormones
Prior to phytohormone extraction, the collected fungal samples (40–200 mg fresh weight, n = 3) were freeze-dried in a Modulyo®D Freeze Dryer (Thermo Scientific, Waltham, USA) for 48 h. The freeze-dried fungal samples and the collected root tissue of co-cultivated seedlings (1 sample = 12–15 seedlings from the same plate; which corresponded to 30–150 mg fresh weight, n = 8) were weighed and homogenized using a Geno/Grinder® (Spex SamplePrep, Stanmore, UK) at 1,100 rounds per min for 1 min. As described in Almeida Trapp et al. (2014), 1 ml of methanol: water (7:3) containing 20 μg/ml of d4-SA and d5-IAA as well as 10 μg/ml d5-JA and d6-ABA was added to the powdered root and fungal material. After mixing, samples were shaken for 30 min and centrifuged at 16,000 g at 4°C for 5 min. Subsequently the supernatant was transferred into a new tube and concentrated for 4 h at 45°C in an Eppendorf Concentrator plus (Eppendorf AG, Hamburg, Germany). The concentrate was resuspended in 100 μl of 50% methanol with 0.05% formic acid, mixed and centrifuged at 16,000 g at 4°C for 10 min. Afterwards the supernatant was collected and measured on an Agilent 1100 HPLC system (Agilent Technologies, Böblingen, Germany) connected to a LTQ Orbitrap mass spectrometer (Thermo Scientific, Waltham, USA) (Almeida Trapp et al., 2014).
Statistics
Statistical analyzes of phytohormone data were performed in R studio (version 1.1.456), using one way ANOVA on log transformed data to ensure that the residues followed a normal distribution. Tukey's HSD test was used as post-hoc test to examine the differences between factor levels (treated plants vs. control) or multiple comparison for the amount of phytohormones present in fungi samples.
Results
The Reporter Construct DR5::EGFP-DR5v2::tdTomato Responds to Exogenously Applied IAA in a Concentration-Dependent Manner in Arabidopsis Roots
To test the expression of the auxin reporters to exogenously applied IAA, the roots of 12 day-old Arabidopsis seedlings were incubated with increasing IAA concentrations. After 24 h, the EGFP and tdTomato fluorescence was monitored (for experimental set-up see: Figures 1A, 2A,B). Without exogenously applied IAA, the fluorescence from both reporters was detectable in the quiescent center, columella, pericycle, and vascular system of the roots (Figure 2A, second image, EGFP; third image, tdTomato; fourth image, merged). Incubation with the lowest applied concentration of 5 μM IAA resulted in a significant increase in both fluorescence signals which were extended to the rhizodermal cell layers (Figure 2B). The intensity of the signals after the application of 5 μM IAA was twice as high as in the untreated controls and increased almost linear with increasing IAA concentrations (Figures 2B,C). Because the fluorescence signals were detectable throughout the entire root tissues (Figure 2B) exogenously applied IAA did not cause cell- or tissue-specific induction of the reporter genes. For these experiments and the pictures for the physiological experiments shown below, the fluorescence from both reporters was always measured; however, the overlay analysis never discovered meaningful differences (see Figure 2B). Since the fluorescence signal for the EGFP reporter was much lower than the one observed for the tdTomato reporter, the cell- and tissue-specific resolution was better for the latter reporter system. Therefore, for the presentation of the data, we show the results for the stronger fluorescent tdTomato reporter. Furthermore, the linear increase of the fluorescence signals with increasing exogenous applied IAA (Figure 2B) makes it likely that the fluorescence reports fungus-induced changes in the expression of auxin-responsive genes in the root tissues.
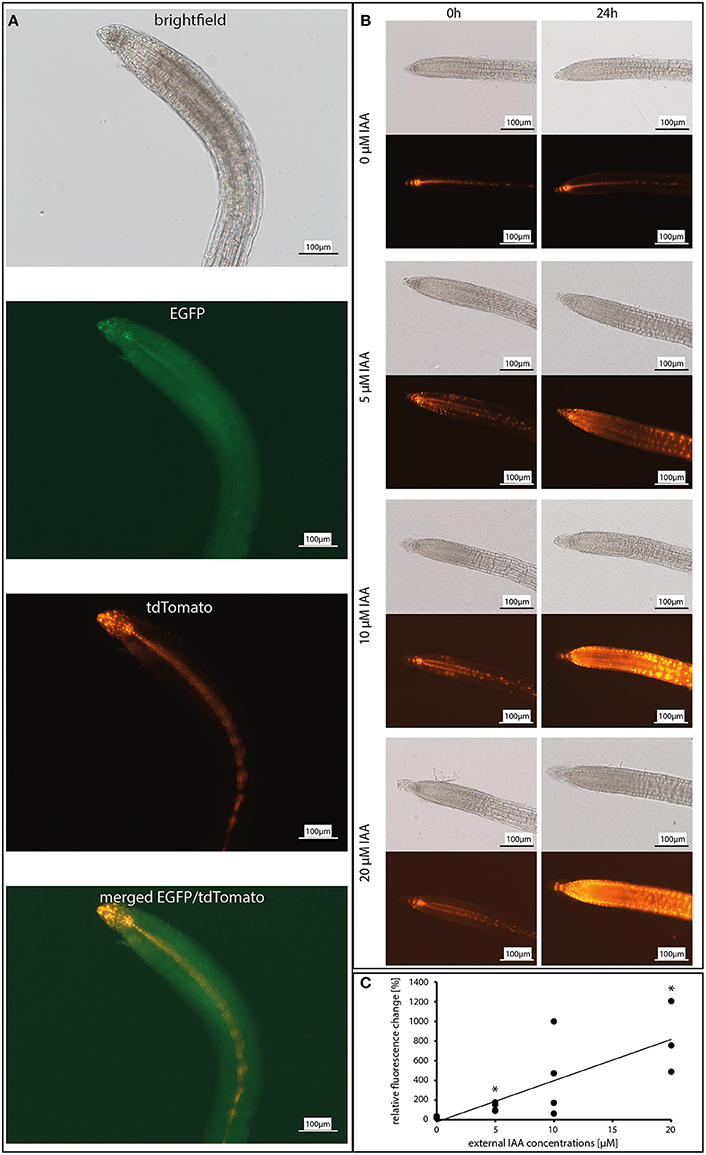
Figure 2. Dose-response curve of the reporter construct DR5::EGFP-DR5v2::tdTomato in response to incubation with 0, 5, 10, and 20 μM IAA (indole-3-acetic acid). (A) Co-localization of the EGFP and tdTomato expression in 12 day-old A. thaliana roots. Shown are the brightfield image, GFP channel (for EGFP), DS red channel (for tdTomato), and a merged image of the GFP and the DS red channel. (B) Fluorescence images of the tdTomato signal after 0 and 24 h. (C) Graphic presentation of the relative fluorescence change in response to exogenous IAA concentrations (n = 3–4). Asterisks indicate significant differences to 0 μM IAA (p < 0.05).
Live Imaging of Root Infection Reveals Fungus-Specific Redistribution of Auxin Maxima in A. thaliana
The auxin response was monitored over 24 h after spore application to the roots by measuring the fluorescence emitted from the DR5v2::tdTomato reporter using light sheet fluorescence microscopy (Figures 1B, 3A,B). Representative pictures from the movies (Supplementary Videos S1–S5) are shown in Figure 3A. Figure 3B shows quantified fluorescence signals of the entire roots and of the root tip separately. Without spores, the tdTomato-derived fluorescence displayed initially a stable signal in the quiescent center, columella, pericycle and vascular system (Figure 3A). After 10–12 h, the overall fluorescence in the entire root increased. In the root tip, we observed a continuous decrease of the fluorescence (see Figure 3B), while the fluorescence from cells located in the elongation zone and from rhizodermal cells increased. The measurements were stopped after 24 h because the reporter system showed the first bleaching symptoms at the root tip.
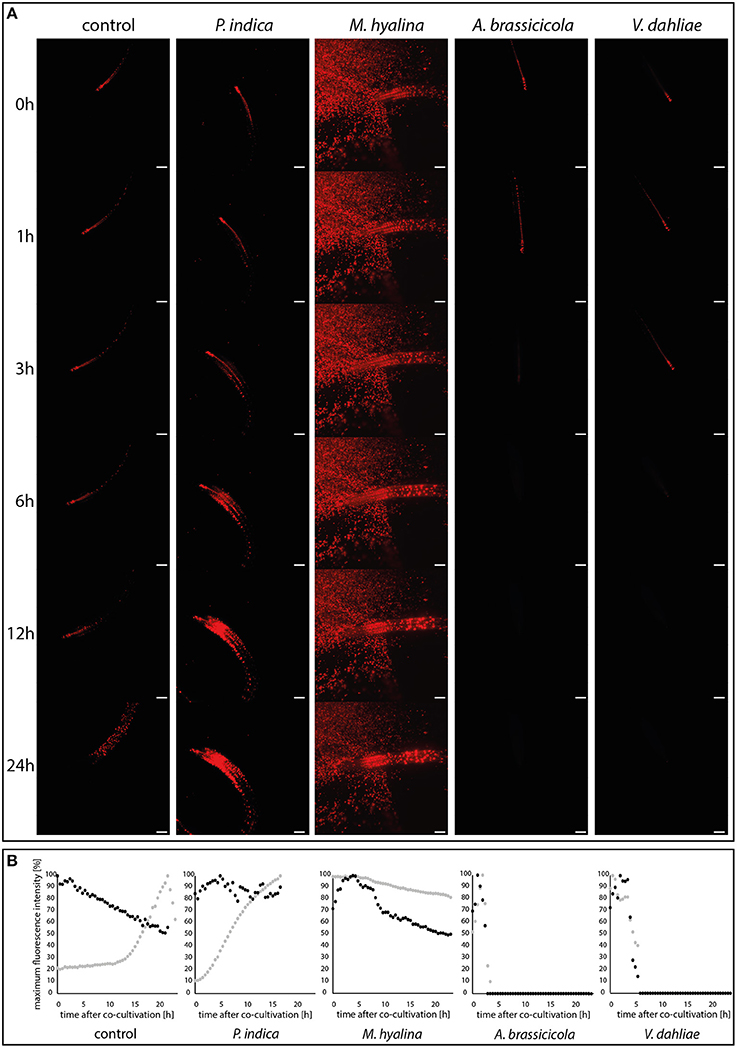
Figure 3. Visualization of auxin maxima using the reporter construct DR5::EGFP-DR5v2::tdTomato during plant-fungus interaction. (A) Light sheet images of 24 h-recordings of control and co-cultivation with spore solutions of P. indica, M. hyalina, A. brassicicola, and V. dahliae. Scale bar = 200 μm. Background fluorescence in M. hyalina co-culture is due to autofluorescence of spores. (B) Quantification curves of fluorescence maxima of each co-culture in a time-dependent manner. Black dots, root tip; gray dots, whole root (n = 3).
Application of spores from P. indica led to an almost linear increase of the fluorescence in the entire root which started after a lag phase of ~1 h. Most of the fluorescence was emitted from cells of the basal meristem and the transition zone. Furthermore, the decrease of the fluorescence emitted from the root tip, which was observed for uncolonized roots, was stopped. This clearly indicates that signals from P. indica activate the auxin-responsive reporter gene in Arabidopsis roots.
A quite different scenario was observed for M. hyalina. The overall emission from the entire root remained almost constant over the measuring period (<20% decrease over the 24 h period), indicating that the fungus inhibits the stimulation which is observed for uncolonized and P. indica-colonized roots (more than 70% increase over the 24 h period). Furthermore, after initial stimulation of the fluorescence emission, the activity also declines at the root tip, similar to the decline observed for the uncolonized control. These results demonstrate that M. hyalina prevents the activation of the reporter gene.
The two pathogenic fungi A. brassicicola and V. dahliae showed almost identical effects on the expression of the reporter gene, although the first fungus is a necrotroph and the second a biotroph. A few hours after spore application the fluorescence in the roots and root tips completely disappeared. Thus, signals from the fungi very likely inhibit the activation of the reporter gene.
In summary, compared to uncolonized roots, P. indica stimulated and the pathogens inhibited the expression of the reporter gene. Besides an initial positive effect on the root tip, the reporter gene activity is not altered by M. hyalina.
Phytohormone Levels in Colonized A. thaliana Roots
The phytohormone levels in Arabidopsis roots are strongly influenced by the 24 h incubation period with the different fungi (Figure 4). Compared to the uncolonized control, the IAA level in P. indica-, M. hyalina-, and A. brassicicola-colonized roots increased, whereas V. dahliae colonization had no effect. Interestingly, co-cultivation with M. hyalina resulted in the highest stimulation of the IAA level (~4-fold stimulation); P. indica and A. brassicicola showed a similar, but lower induction (~3-fold stimulation). These results are not consistent with expression data of the auxin reporter gene (Figures 2, 3).
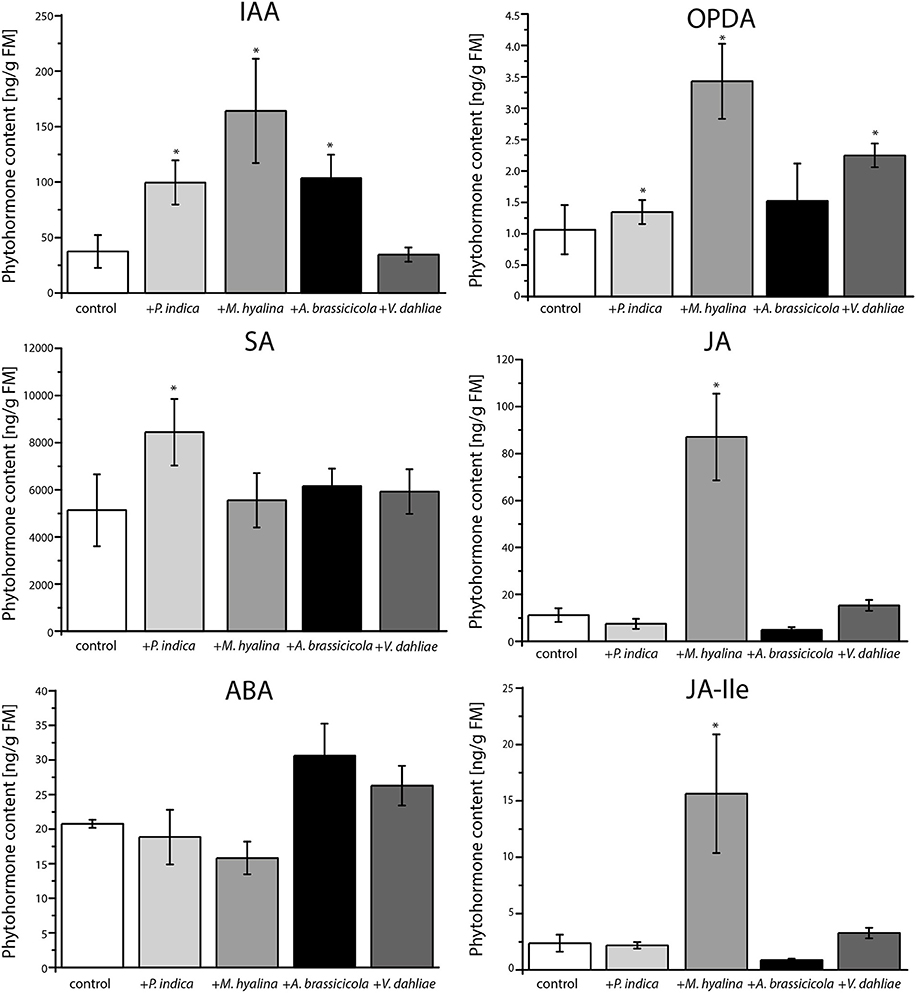
Figure 4. Phytohormone measurements of A. thaliana roots after one day of co-cultivation with P. indica, M. hyalina, A. brassicicola, and V. dahliae. (IAA, indole-3-acetic acid; ABA, abscisic acid; SA, salicylic acid; JA, jasmonic acid; OPDA, 12-oxophytodienoic acid (cis and trans); JA-Ile, jasmonoyl-isoleucine conjugate). Asterisks mark statistically significant differences against control treatment (n = 8; *p < 0.05) as determined by one–way ANOVA followed by Tukey's test.
However, quantification of the amounts of the defense-related phytohormones revealed that only M. hyalina, but not the other three fungi, stimulated the accumulation of the jasmonates JA and JA-Ile. In addition to M. hyalina, the JA precursor 12-oxophytodienoic acid (OPDA) showed a low, however significant increase after infection with P. indica and V. dahliae. The only other significant alteration induced by any of the fungi was a slight stimulation of the SA level in P. indica-infected roots. These results suggest the jasmonates play an important role during the early phase in the M. hyalina/Arabidopsis interaction, and that the sharp decline in the reporter gene expression in response to the two pathogens is probably not directly caused by the phytohormones but by factors influencing the cell fate in the symbiotic roots (cf. section Discussion).
Phytohormone Levels in the Fungal Mycelia
To investigate whether the observed differences in the regulation of the reporter gene in Arabidopsis roots are caused by differences in fungal auxin levels, the phytohormone concentrations were measured in the mycelia. Strikingly, the IAA contents in the four fungi varied tremendously. The highest level was found in P. indica, M. hyalina contained ~5 times less auxin, and the levels in the two pathogens was quite low (Figure 5). We also measured the amounts of the stress related JA, SA and abscisic acid. None of the mycelia contained JA and JA-Ile (data not shown). The lowest SA level was found in M. hyalina. P. indica contained twice as much and the pathogens 6–7 times more SA than M. hyalina. No abscisic acid was found in the two beneficial fungi, while the pathogens contained comparable levels (Figure 5). Taken together, the auxin levels that were detectable in the mycelia were consistent with the regulation of the auxin-responsive reporter gene in Arabidopsis roots.
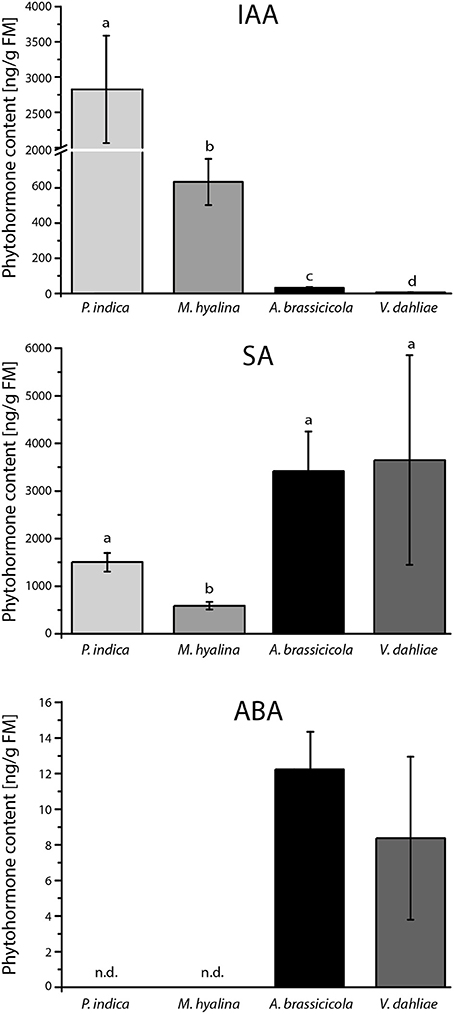
Figure 5. Phytohormone content of mycelia from P. indica cultivated on Kaefer's medium for three weeks and M. hyalina, A. brassicicola, and V. dahliae grown for 2 weeks on potato dextrose agar medium (IAA, indole-3-acetic acid; ABA, abscisic acid; SA, salicylic acid). Statistically significant differences between all fungal species were analyzed using one–way ANOVA. Different letters indicate significant differences among groups for p < 0.05, determined by Tukey's test (n = 3).
Jasmonate Impairs DR5::EGFP-DR5v2::tdTomato Fluorescence
Due to the elevated jasmonate contents found during the M. hyalina/Arabidopsis interaction (Figure 4), the question arose whether jasmonates can affect the expression of the auxin reporter gene. Therefore, roots of 12 day-old DR5::EGFP-DR5v2::tdTomato seedlings were incubated with increasing JA concentrations for 24 h and the fluorescence was monitored. Incubation with 1 and 5 μM JA resulted in a stable but decreased tdTomato fluorescence intensity compared to control samples (Figures 6A,B). Application of 10 μM JA showed the significantly highest detectable loss of fluorescence (~20%) in the roots, supporting the hypothesis that increased JA levels during early M. hyalina/Arabidopsis interaction impede the activation of the IAA-responsive reporter construct.
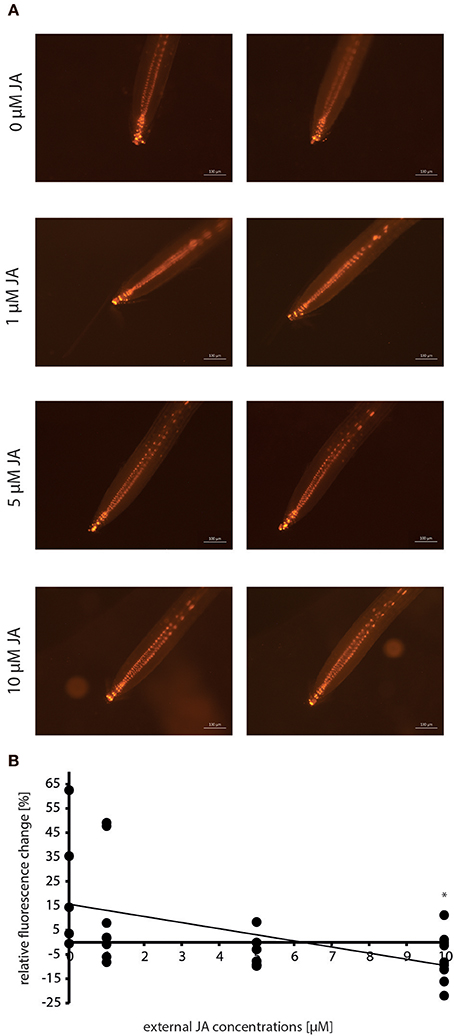
Figure 6. Dose-response curve of the reporter construct DR5::EGFP-DR5v2::tdTomato in response to incubation with 0, 1, 5, and 10 μM JA (jasmonic acid) before and after 24 h. (A) Fluorescence images of the tdTomato signal after 0 and 24 h. (B) Graphic presentation of the relative fluorescence change in response to exogenous JA concentrations (n = 6–10). Asterisks indicate significant differences to 0 μM JA (p < 0.05).
P. indica Induces the Formation of Lateral Root Primordia
The former results suggest that only P. indica can initiate processes which may result in the promotion of root development within the 24 h of experimentation. In accordance with this idea, we found that the initiation of lateral root primordia was stimulated by P. indica but not by M. hyalina (Figure 7).
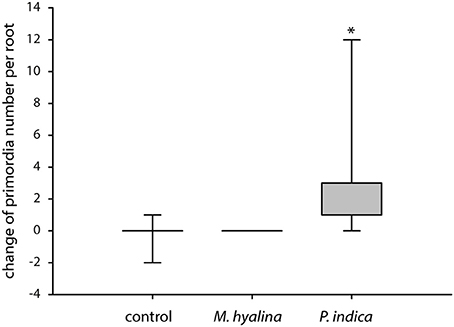
Figure 7. Measurement of lateral root primordia induction in A. thaliana after 1 day of co-cultivation with M. hyalina and P. indica. Graphical illustration of the absolute change of primordia number. The asterisk mark statistical significant differences against control and M. hyalina treatment (n = 5–7; *p < 0.05).
Discussion
Expression of Auxin Reporter Is Dose-Dependent in Arabidopsis Roots
Liao et al. (2015) demonstrated that the auxin reporter construct DR5::EGFP-DR5v2::tdTomato induced a dose- and time-dependent fluorescence development as well as IAA-induced gene expression when Arabidopsis plants are treated with 0.0001–1.0 μM IAA. The construct was used to monitor the auxin level in different tissues of transformed A. thaliana, P. tremula × alba, H. vulgare, and N. benthamiana plants (Hilbert et al., 2012; Chen et al., 2013; Liao et al., 2015; Kirbis, 2017). A previous study showed that the EGFP signal was relatively low compared to the tdTomato signal (Kirbis, 2017). We observed the same for fungus-exposed and control Arabidopsis roots but did not observe differences in the expression for the two reporters (Figure 2A). Due to better resolution, we used the tdTomato fluorescence (Figures 2A,B) for our study and demonstrate that the expression is dose-dependent and shows expression in tissues and cells which are known to be involved in root growth (Figures 2B,C). With an increase in the applied IAA concentration, the fluorescence appeared also in tissues which are located shootwards of the elongation zone in the roots. The expression pattern matches known polar auxin transport mechanisms where IAA is translocated to the root apex and toward the root–shoot junction (Bennett et al., 1998; Reed et al., 1998; Rashotte et al., 2000; Casimiro et al., 2001). Therefore, the construct was used to monitor rapid changes in the auxin distribution in Arabidopsis roots in response to different root-interacting fungi.
P. indica, but Not M. hyalina and the Pathogenic Fungi Activate the DR5v2 Promoter in Arabidopsis Roots
The endophytic fungus P. indica promotes the growth of A. thaliana by enhancing both root and shoot biomass production (Peškan-Berghöfer et al., 2004; Camehl et al., 2011; Lee et al., 2011; Das et al., 2012). In Chinese cabbage it was demonstrated that colonization by P. indica also strongly enhances lateral root development (Lee et al., 2011; Dong et al., 2013). It is known that auxin plays a role in the beneficial interaction and that the fungus produces auxin and auxin precursors which interfere with hormones inside the plant (Sirrenberg et al., 2007; Vadassery et al., 2008; Hilbert et al., 2012; Xu et al., 2018). The auxin reporter was utilized to analyze the auxin kinetic in the early infection stages of P. indica-colonized roots as well as in roots colonized by M. hyalina, A. brassicicola and V. dahliae (Figure 3). Colonization with P. indica showed a strong, 10-fold increase in fluorescence in the entire root while the expression at the root tip stayed at a rather constant level (Figure 3B). Already 3 h after co-culture, the increase in the fluorescence (Figure 3A) indicates a higher amount of available IAA in the root tissue, which may be provided by the fungus or is produced by the plant. A similar (but only 3-fold) elevation of free IAA was observed in barley roots co-cultured with P. indica for 3 d (Hilbert et al., 2012). At later time points (5 and 14 days after infection) this effect was gone in barley which was also observed for Arabidopsis seedlings where the IAA level in colonized seedlings was not different from uncolonized controls 7, 10 and 14 days after infection (Vadassery et al., 2008; Hilbert et al., 2012). Our data suggests that P. indica induces a rapid increase in auxin levels during early recognition phases which might be crucial for reprogramming root development. Initiation of the formation of lateral root primordia can already be detected 24 h after co-cultivation with P. indica, but not with M. hyalina (Figure 7).
Phytohormones controlling the development of colonized roots can be of fungal or plant origin. Hilbert et al. (2012) showed that indole derivative production by P. indica is not required for growth promotion but for biotrophic colonization of barley roots. In other symbiotic interactions, promotion of root or plant growth was proposed to be caused by microbial phytohormones—in particular auxin (e.g., Contreras-Cornejo et al., 2009; Dudeja et al., 2012; Khan et al., 2014; Lee et al., 2015; Liao et al., 2017, and references therein). The examples demonstrate that fungal auxin or metabolites which can be converted to auxin in the plant, participate in growth regulatory effects in the host. Apparently, phytohormones that are active in the roots can be of either plant or microbial origin. Which one of the two sources might play a more important role in the symbiotic interaction and how these processes are related to circuits, which manipulate plant's phytohormone metabolism and function is probably symbiosis-specific.
M. hyalina did not induce such an auxin increase in the entire root during the first 24 h of co-culture, in fact, the level even decreased slightly about 20% (Figure 3B). Five h after co-cultivation, the fluorescence at the root tip increased by 30% and decreased again afterwards to half of the initial level. The initial increase in fluorescence during the recognition of the fungus by the plant could be caused by fungus-derived auxin which is later on stopped by other processes (cf. below). These results match the phenotype observed for M. hyalina-colonized Arabidopsis seedlings: we did not observe any significant increase in the root biomass while the aerial parts of the colonized seedlings became bigger (Johnson et al., 2018b).
In contrast, the fluorescence in the entire root decreased in response to the two pathogenic fungi V. dahliae and A. brassicicola after short periods of co-cultivation (Figure 3A). For V. dahliae, this was unexpected since it is well known that its interaction starts with a biotrophic phase associated with the stimulation of growth which is followed by a necrotrophic phase later on (Schnathorst, 1981; Reusche et al., 2014; Cho, 2015). In the A. brassicicola co-culture, the fluorescence was no longer detectable after 3 h while V. dahliae-infected plants lost the fluorescence ~6 h after co-cultivation (Figure 3B). This is accompanied by visible destruction of plant tissue and the root tip (data not shown). Both pathogens colonized the roots rapidly. For V. dahliae, the roots were covered with conidia already 6 h after infection (Zhao et al., 2014). Downregulation of the auxin response is likely caused by toxic effects: V. dahliae produces different phytotoxins and other molecules that induce the hosts' cell death associated with the degradation of host tissue (Fradin and Thomma, 2006). A. brassicicola produces the AB-toxin which is released by the germinating spores on host tissue (Oka et al., 2005).
M. hyalina Induces Jasmonate and P. indica SA Accumulation in the Host Roots
Arabidopsis plants respond to colonization by different fungi with the accumulation of different phytohormones. Beneficial fungi like P. indica often induce SA, while necrotrophic fungi induce jasmonates (Schäfer et al., 2009; Sun et al., 2014; Johnson et al., 2018b; Scholz et al., 2018; Vahabi et al., 2018; Xu et al., 2018). To analyze whether the observed IAA-induced fluorescence changes during root colonization (Figure 3) are exclusively mediated by auxin changes or whether other phytohormones might also be involved, we measured the phytohormone profiles in the roots (Figure 4). Compared to uncolonized control roots, both beneficial fungi showed a significant accumulation of IAA within the first 24 h of co-culture. For later time points, quite different results for IAA accumulation were reported for P. indica-colonized roots (Vadassery et al., 2008; Lee et al., 2011; Hilbert et al., 2012; Hua et al., 2017). M. elongata-colonized maize roots and M. alpina-colonized Crocus sativus L. plants also showed significantly elevated IAA contents (Wani et al., 2017; Li et al., 2018). However, expression of auxin-responsive genes has never been investigated at the early time points studied here (Figure 3). Apparently, although P. indica and M. hyalina colonization results in the accumulation of auxin, the outcome is quite different. While P. indica induces fluorescence, M. hyalina does not. This suggests that auxin in the M. hyalina-colonized roots cannot (fully) activate the downstream IAA signaling cascade. A possible explanation could be that IAA signaling is antagonistically regulated by other hormones or elicitors.
Furthermore, V. dahliae did not induce IAA accumulation in Arabidopsis roots which matches the observed fluorescence. However, the IAA increase was similar for A. brassicicola and P. indica, although the pathogen did not induce the fluorescence from the auxin reporter (Figure 3). Similar observations were made in previous studies (Qi et al., 2012; Riet et al., 2016). This might be due to the rapidly induced cell death by the fast growing pathogen and its release of toxins. The importance of auxins for resistance against different pathogens has been repeatedly demonstrated: For instance, auxin biosynthesis defective mutants are more susceptible to A. brassicicola infection compared to wild-type plants (Bari and Jones, 2009; Kazan and Manners, 2009; Qi et al., 2012). Furthermore, A. brassicicola infection leads to the degradation of AUX/ IAA proteins indicating that the fungus activates the auxin signal transduction pathway (Qi et al., 2012). Since the hormone data in previous studies were measured for entire roots or even plants, it is difficult to compare them with locally occurring infections and generation of auxin maxima.
Recent studies have demonstrated an antagonistic crosstalk between IAA and SA, which in turn regulates plants' resistance to different fungi. It has been hypothesized that downregulation of auxin signaling is part of the SA-mediated disease-resistance mechanism (Navarro et al., 2006; Chen et al., 2007; Wang et al., 2007; Kazan and Manners, 2009). Our analysis of the SA content of colonized roots is consistent with this assumption, since roots with high IAA content did not show a strong accumulation of SA upon fungal infection at least with A. brassicicola and M. hyalina (Figure 4). However, P. indica-colonized roots showed a small increase in both—IAA and SA levels; the latter was also observed in our previous studies (Sun et al., 2014; Vahabi et al., 2018). This seemingly contradiction was addressed in a previous study showing that SA does not directly affect the IAA concentration but the auxin-dependent signaling (Wang et al., 2007). Colonization by M. hyalina did not result in elevated SA levels as already shown by Johnson et al. (2018b). The two pathogenic fungi did not induce SA. Similar results were obtained in previous studies where SA content was not elevated and SA mutants did not show a different susceptibility (Botanga et al., 2012; Sun et al., 2014; Scholz et al., 2018). Apparently, significant amounts of SA accumulate only during early phases of the P. indica/Arabidopsis interaction.
The class of jasmonates is mainly induced by wounding of plant tissue and plays a major role in defense against necrotrophic fungi (Thaler et al., 2004; Kachroo and Kachroo, 2009; Acosta and Farmera, 2010). Our study revealed that after 24 h of co-culture, the JA and JA-Ile contents were not significantly up-regulated in the roots colonized by the two pathogens (Figure 4). A previous study with V. dahliae showed the same result (Scholz et al., 2018), although a precursor of JA, OPDA, was stimulated by V. dahliae. In this study, we could not distinguish between the active cis- and inactive trans-OPDA, so a statement about the relevance of this JA precursor would not be appropriate. Interestingly, there was a clear difference in the induction of jasmonates between the two beneficial fungi. While P. indica-colonized roots contained the same levels of JA and JA-Ile as the uncolonized control roots, M. hyalina colonization resulted in a significant increase in the OPDA (3-fold), JA and JA-Ile levels (8-fold) (Figure 4). This appears to be restricted to the early recognition phase of the two partners, since we did not detect an increase of jasmonates in M. hyalina-colonized plants during later time points (Johnson et al., 2018b). A recent study in Arabidopsis indicated that JA interferes with auxin signaling independent of the JA-receptor complex COI1. The expression of auxin-inducible genes and lateral rooting is inhibited in Arabidopsis seedlings treated with JA (Ishimaru et al., 2018). These findings are supported by our experiment showing that the incubation with increasing concentrations of JA impairs the expression of the IAA-responsive reporter construct (Figures 6A,B) in spite of the presence of elevated IAA levels in M. hyalina colonized roots (Figure 4). Finally, although ABA is involved in plant defense (Figure 4), we did not find a significant accumulation of this hormone during the early phase of interaction for any of the four fungi with Arabidopsis roots.
The crosstalk of phytohormones during recognition and early phases of plant/fungus interaction is not well understood and most studies focus on defense-related hormones. The presented results and measuring techniques open new research fields, which will shine more light on the first steps in symbiotic interactions.
Data Availability
All datasets generated for this study are included in the manuscript and/or the supplementary files.
Author Contributions
AKM, AF, SS, and RO designed the experiments. AKM, AF, SÖ, and MA-T performed the experiments. AKM, AF, and MA-T analyzed the data. VG and BH provided and assisted with the light sheet microscope. AK, TL, and GT generated and provided the transgenic seed material. AKM, AF, SS, AM, and RO wrote the manuscript with contributions from all authors.
Conflict of Interest Statement
The authors declare that the research was conducted in the absence of any commercial or financial relationships that could be construed as a potential conflict of interest.
Acknowledgments
We thank Frank Müller and Daniel Veit for technical and Anindya Majumder for experimental support. The research was supported by the CRC1127 to SS and RO; AKM was supported by the International Max Planck Research School and MA-T by the Alexander von Humboldt Foundation.
Supplementary Material
The Supplementary Material for this article can be found online at: https://www.frontiersin.org/articles/10.3389/fmicb.2019.00380/full#supplementary-material
Supplementary Video S1. Visualization of auxin maxima using the reporter construct DR5::EGFP-DR5v2::tdTomato during plant-fungus interaction. Light sheet videos of 17 h-recordings of control without addition of fungal spores.
Supplementary Video S2. Visualization of auxin maxima using the reporter construct DR5::EGFP-DR5v2::tdTomato during plant-fungus interaction. Light sheet video of 17 h-recording of co-cultivation with P. indica spore solution.
Supplementary Video S3. Visualization of auxin maxima using the reporter construct DR5::EGFP-DR5v2::tdTomato during plant-fungus interaction. Light sheet video of 17 h-recording of co-cultivation with M. hyalina spore solution. Background fluorescence in M. hyalina co-culture is due to autofluorescence of spores.
Supplementary Video S4. Visualization of auxin maxima using the reporter construct DR5::EGFP-DR5v2::tdTomato during plant-fungus interaction. Light sheet video of 17 h-recording of co-cultivation with A. brassicicola spore solution.
Supplementary Video S5. Visualization of auxin maxima using the reporter construct DR5::EGFP-DR5v2::tdTomato during plant-fungus interaction. Light sheet video of 17 h-recording of co-cultivation with V. dahliae spore solution.
References
Acosta, I. F., and Farmer, E. E. (2010). Jasmonates. Arabidopsis Book 8:e0129. doi: 10.1199/tab.0129
Almeida Trapp, M., De Souza, G. D., Rodrigues-Filho, E., Boland, W., and Mithöfer, A. (2014). Validated method for phytohormone quantification in plants. Front. Plant Sci. 5:417. doi: 10.3389/fpls.2014.00417
Bari, R., and Jones, J. D. (2009). Role of plant hormones in plant defence responses. Plant Mol. Biol. 69, 473–488. doi: 10.1007/s11103-008-9435-0
Bennett, M. J., Marchant, A., May, S. T., and Swarup, R. (1998). Going the distance with auxin: unravelling the molecular basis of auxin transport. Philos. Trans. R Soc. Lond. B Biol. Sci. 353, 1511–1515. doi: 10.1098/rstb.1998.0306
Bhalerao, R. P., Eklöf, J., Ljung, K., Marchant, A., Bennett, M., and Sandberg, G. (2002). Shoot-derived auxin is essential for early lateral root emergence in Arabidopsis seedlings. Plant J. 29, 325–332. doi: 10.1046/j.0960-7412.2001.01217.x
Bolton, M. D., van Esse, H. P., Vossen, J. H., de Jonge, R., Stergiopoulos, I., Dekker, H. L., et al. (2008). The novel Cladosporium fulvum lysin motif effector Ecp6 is a virulence factor with orthologues in other fungal species. Mol. Microbiol. 69, 119–136. doi: 10.1111/j.1365-2958.2008.06270.x
Botanga, C. J., Bethke, G., Chen, Z., Gallie, D. R., Fiehn, O., and Glazebrook, J. (2012). Metabolite profiling of Arabidopsis inoculated with Alternaria brassicicola reveals that ascorbate reduces disease severity. Mol. Plant Microbe Interact. 12, 1628–1638. doi: 10.1094/MPMI-07-12-0179-R
Calderon-Villalobos, L. I., Tan, X., Zheng, N., and Estelle, M. (2010). Auxin perception—structural insights. Cold Spring Harb. Perspect. Biol. 2:a005546. doi: 10.1101/cshperspect.a005546
Camehl, I., Drzewiecki, C., Vadassery, J., Shahollari, B., Sherameti, I., Forzani, C., et al. (2011). The OXI1 kinase pathway mediates Piriformospora indica-induced growth promotion in Arabidopsis. PLoS Pathog. 7:e1002051. doi: 10.1371/journal.ppat.1002051
Casimiro, I., Marchant, A., Bhalerao, R. P., Beeckman, T., Dhooge, S., Swarup, R., et al. (2001). Auxin transport promotes Arabidopsis lateral root initiation. Plant Cell 13, 843–852. doi: 10.1105/tpc.13.4.843
Chen, Y., Yordanov, Y. S., Ma, C., Strauss, S., and Busov, V. B. (2013). DR5 as a reporter system to study auxin response in Populus. Plant Cell Rep. 32, 453–463. doi: 10.1007/s00299-012-1378-x
Chen, Z., Agnew, J. L., Cohen, J. D., He, P., Shan, L., Sheen, J., et al. (2007). Pseudomonas syringae type III effector AvrRpt2 alters Arabidopsis thaliana auxin physiology. Proc. Natl. Acad. Sci. U.S.A. 104, 20131–20136. doi: 10.1073/pnas.0704901104
Cho, Y. (2015). How the necrotrophic fungus Alternaria brassicicola kills plant cells remains an enigma. Eukaryot. Cell 14, 335–344. doi: 10.1128/EC.00226-14
Contreras-Cornejo, H. A., Macías-Rodríguez, L., Cortés-Penagos, C., and López-Bucio, J. (2009). Trichoderma virens, a plant beneficial fungus, enhances biomass production and promotes lateral root growth through an auxin-dependent mechanism in Arabidopsis. Plant Physiol. 149, 1579–1592. doi: 10.1104/pp.108.130369
Das, A., Kamal, S., Shakil, N. A., Sherameti, I., Oelmüller, R., Dua, M., et al. (2012). The root endophyte fungus Piriformospora indica leads to early flowering, higher biomass and altered secondary metabolites of the medicinal plant, Coleus forskohlii. Plant Signal. Behav. 7, 103–112. doi: 10.4161/psb.7.1.18472
de Jonge, R., Bolton, M. D., and Thomma, B. P. H. J. (2011). How filamentous pathogens co-opt plants: the ins and outs of fungal effectors. Curr. Opin. Plant Biol. 14, 400–406. doi: 10.1016/j.pbi.2011.03.005
de Jonge, R., and Thomma, B. P. H. J. (2009). Fungal LysM effectors: extinguishers of host immunity? Trends Microbiol. 17, 151–157. doi: 10.1016/j.tim.2009.01.002
de Jonge, R., van Esse, H. P., Kombrink, A., Shinya, T., Desaki, Y., Bours, R., et al. (2010). Conserved fungal LysM effector Ecp6 prevents chitin-triggered immunity in plants. Science 329, 953–955. doi: 10.1126/science.1190859
De Rybel, B., Adibi, M., Breda, A. S., Wendrich, J. R., Smit, M. E., Novák, O., et al. (2014). Plant development. Integration of growth and patterning during vascular tissue formation in Arabidopsis. Science 345:1255215. doi: 10.1126/science.1255215
Dharmasiri, N., Dharmasiri, S., and Estelle, M. (2005). The F-box protein TIR1 is an auxin receptor. Nature 435, 441–445. doi: 10.1038/nature03543
Dombrecht, B., Xue, G. P., Sprague, S. J., Kirkegaard, J. A., Ross, J. J., Reid, J. B., et al. (2007). MYC2 differentially modulates diverse jasmonate-dependent functions in Arabidopsis. Plant Cell 19, 2225–2245. doi: 10.1105/tpc.106.048017
Dong, S., Tian, Z., Chen, P. J., Senthil Kumar, R., Shen, C. H., Cai, D., et al. (2013). The maturation zone is an important target of Piriformospora indica in Chinese cabbage roots. J. Exp. Bot. 64, 4529–4540. doi: 10.1093/jxb/ert265
Dudeja, S. S., Giri, R., Saini, R., Suneja-Madan, P., and Kothe, E. (2012). Interaction of endophytic microbes with legumes. J. Basic Microbiol. 52, 248–260. doi: 10.1002/jobm.201100063
Fradin, E. F., and Thomma, B. P. (2006). Physiology and molecular aspects of Verticillium wilt diseases caused by V. dahliae and V. albo-atrum. Mol. Plant Pathol. 7, 71–86. doi: 10.1111/j.1364-3703.2006.00323.x
Fukaki, H., and Tasaka, M. (2009). Hormone interactions during lateral root formation. Plant Mol. Biol. 69, 437–449. doi: 10.1007/s11103-008-9417-2
Gijzen, M., and Nürnberger, T. (2006). Nep1-like proteins from plant pathogens: recruitment and diversification of the NPP1 domain across taxa. Phytochemistry 67, 1800–1807. doi: 10.1016/j.phytochem.2005.12.008
Gutjahr, C., Riemann, M., Müller, A., Düchting, P., Weiler, E. W., and Nick, P. (2005). Cholodny-Went revisited: a role for jasmonate in gravitropism of rice coleoptiles. Planta 222, 575–585. doi: 10.1007/s00425-005-0001-6
Hentrich, M., Böttcher, C., Düchting, P., Cheng, Y., Zhao, Y., Berkowitz, O., et al. (2013). The jasmonic acid signaling pathway is linked to auxin homeostasis through the modulation of YUCCA8 and YUCCA9 gene expression. Plant J. 74, 626–637 doi: 10.1111/tpj.12152
Hilbert, M., Voll, L. M., Ding, Y., Hofmann, J., Sharma, M., and Zuccaro, A. (2012). Indole derivative production by the root endophyte Piriformospora indica is not required for growth promotion but for biotrophic colonization of barley roots. New Phytol. 196, 520–534. doi: 10.1111/j.1469-8137.2012.04275.x
Hoffmann, M., Hentrich, M., and Pollmann, S. (2011). Auxin-oxylipin crosstalk: relationship of antagonists. J. Integr. Plant Biol. 53, 429–444. doi: 10.1111/j.1744-7909.2011.01053.x
Hua, M. S., Kumar, R. S., Shyur, L. F., Cheng, Y. B., Tian, Z., Oelmüller, R., et al. (2017). Metabolomic profiles delineate symbiotic functions of the root endophytic fungus Piriformospora indica colonizing Chinese cabbage roots. Sci. Rep. 7:9291. doi: 10.1038/s41598-017-08715-2
Huang, H., Liu, B., Liu, L., and Song, S. (2017). Jasmonate action in plant growth and development. J. Exp. Bot. 68, 1349–1359. doi: 10.1093/jxb/erw495
Ishimaru, Y., Hayashi, K., Suzuki, T., Fukaki, H., Prusinska, J., Meester, C., et al. (2018). Jasmonic acid inhibits auxin-induced lateral rooting independently of the CORONATINE INSENSITIVE1 receptor. Plant Physiol. 177, 1704–1716. doi: 10.1104/pp.18.00357
Johnson, J. M., Ludwig, A., Furch, A. C. U., Mithöfer, A., Scholz, S. S., Reichelt, M., et al. (2018b). The beneficial root-colonizing fungus Mortierella hyalina promotes the aerial growth of Arabidopsis and activates calcium-dependent responses which restrict Alternaria brassicae-induced disease development in roots. Mol. Plant Microbe Interact. doi: 10.1094/MPMI-05-18-0115-R
Johnson, J. M., Reichelt, M., Vadassery, J., Gershenzon, J., and Oelmüller, R. (2014). An Arabidopsis mutant impaired in intracellular calcium elevation is sensitive to biotic and abiotic stress. BMC Plant Biol. 14:162. doi: 10.1186/1471-2229-14-162
Johnson, J. M., Sherameti, I., Ludwig, A., Nongbri, P. L., Sun, C., Lou, B., et al. (2011). Protocols for Arabidopsis thaliana and Piriformospora indica co-cultivation - a model system to study plant beneficial traits. Endocyt. Cell Res. 21, 101–113.
Johnson, J. M., Thürich, J., Petutschnig, E. K., Altschmied, L., Meichsner, D., Sherameti, I., et al. (2018a). Poly(A) ribonuclease controls cellotriose-based interaction of Piriformospora indica and its host Arabidopsis. Plant Physiol. 176, 2496–2514. doi: 10.1104/pp.17.01423
Kachroo, A., and Kachroo, P. (2009). Fatty acid-derived signals in plant defense. Ann. Rev. Phytopathol. 47, 153–176. doi: 10.1146/annurev-phyto-080508-081820
Kazan, K., and Manners, J. M. (2009). Linking development to defense: auxin in plant-pathogen ineractions. Trends Plant Sci. 14, 373–382. doi: 10.1016/j.tplants.2009.04.005
Kepinski, S., and Leyser, O. (2005). The Arabidopsis F-box protein TIR1 is an auxin receptor. Nature 435, 446–451. doi: 10.1038/nature03542
Khan, A. L., Waqas, M., Kang, S. M., Al-Harrasi, A., Hussain, J., Al-Rawahi, A., et al. (2014). Bacterial endophyte Sphingomonas sp. LK11 produces gibberellins and IAA and promotes tomato plant growth. J Microbiol. 52, 689–95. doi: 10.1007/s12275-014-4002-7
Kirbis, A. (2017). Monitoring Auxin Distribution in the Fruits of Lepidium and Aethionema Employing Transgenic Reporter Constructs. Masterthesis, FSU, Jena.
Klosterman, S. J., Subbarao, K. V., Kang, S., Veronese, P., Gold, S. E., Thomma, B. P. H. J., et al. (2011). Comparative genomics yields insights into niche adaptation of plant vascular wilt pathogens. PLoS Pathog. 7:e1002137. doi: 10.1371/journal.ppat.1002137
Lee, K. E., Radhakrishnan, R., Kang, S. M., You, Y. H., Joo, G. J., Lee, I. J., et al. (2015). Enterococcus faecium LKE12 cell-free extract accelerates host plant growth via gibberellin and indole-3-acetic acid secretion. J. Microbiol. Biotechnol. 25, 1467–1475. doi: 10.4014/jmb.1502.02011
Lee, Y. C., Johnson, J. M., Chien, C. T., Sun, C., Cai, D., Lou, B., et al. (2011). Growth promotion of Chinese cabbage and Arabidopsis by Piriformospora indica is not stimulated by mycelium-synthesized auxin. Mol. Plant Microbe Interact. 24, 421–431. doi: 10.1094/MPMI-05-10-0110
Li, F., Chen, L., Redmile-Gordon, M., Zhang, J., Zhang, C., Ning, Q., et al. (2018). Mortierella elongata's roles in organic agriculture and crop growth promotion in a mineral soil. Land Degrad. Dev. 29, 1642–1651. doi: 10.1002/ldr.2965
Liao, C. Y., Smet, W., Brunoud, G., Yoshida, S., Vernoux, T., and Weijers, D. (2015). Reporters for sensitive and quantitative measurement of auxin response. Nat. Methods 12, 207–210. doi: 10.1038/nmeth.3279
Liao, X., Lovett, B., Fang, W., and St Leger, R. J. (2017). Metarhizium robertsii produces indole-3-acetic acid, which promotes root growth in Arabidopsis and enhances virulence to insects. Microbiology 163, 980–991. doi: 10.1099/mic.0.000494
Masucci, J. D., and Schiefelbein, J. W. (1994). The rhd6 mutation of Arabidopsis thaliana alters root-hair initiation through an auxin- and ethylene-associated process. Plant Physiol. 106, 1335–1346. doi: 10.1104/pp.106.4.1335
Masucci, J. D., and Schiefelbein, J. W. (1996). Hormones act downstream of TTG and GL2 to promote root hair outgrowth during epidermis development in the Arabidopsis root. Plant Cell 8, 1505–1517. doi: 10.1105/tpc.8.9.1505
Murashige, T., and Skoog, F. (1962). A revised medium for rapid growth and bioassay with tobacco cultures. Physiol. Plant. 15, 473–497. doi: 10.1111/j.1399-3054.1962.tb08052.x
Narayan, O. P., Verma, N., Singh, A. K., Oelmüller, R., Kumar, M., Prasad, D., et al. (2017). Antioxidant enzymes in chickpea colonized by Piriformospora indica participate in defense against the pathogen Botrytis cinerea. Sci. Rep. 7:13553. doi: 10.1038/s41598-017-12944-w
Naseem, M., Srivastava, M., Tehseen, M., and Ahmed, N. (2015). Auxin crosstalk to plant immune networks: a plant-pathogen interaction perspective. Curr. Protein Pept. Sci. 16, 389–394. doi: 10.2174/1389203716666150330124911
Navarro, L., Dunoyer, P., Jay, F., Arnold, B., Dharmasiri, N., Estelle, M., et al. (2006). A plant miRNA contributes to antibacterial resistance by repressing auxin signaling. Science 312, 436–439. doi: 10.1126/science.1126088
Oelmüller, R. (2018). Sensing environmental and developmental signals via cellooligomers. J. Plant Physiol. 229, 1–6. doi: 10.1016/j.jplph.2018.06.010
Oelmüller, R., Sherameti, I., Tripathi, S., and Varma, A. (2009). Piriformospora indica: a novel multifunctional symbiotic fungus. Symbiosis 49, 1–18. doi: 10.1007/s13199-009-0009-y
Oka, K., Akamatsu, H., Kodama, M., Nakajima, H., Kawada, T., and Otanib, H. (2005). Host-specific AB-toxin production by germinating spores of Alternaria brassicicola is induced by a host-derived oligosaccharide. Physiol. Mol. Plant Pathol. 66, 12–19. doi: 10.1016/j.pmpp.2005.03.005
Oliva, R., Win, J., Raffaele, S., Boutemy, L., Bozkurt, T. O., Segretin, M. E., et al. (2010). Recent developments in effector biology of filamentous plant pathogens. Cell. Microbiol. 12, 705–715. doi: 10.1111/j.1462-5822.2010.01471.x
Pan, R., Xu, L., Wie, Q., Wu, C., Tang, W., Oelmüller, R., et al. (2017). Piriformospora indica promotes early flowering in Arabidopsis through regulation of the photoperiod and gibberellin pathways. PLoS ONE 12:e0189791. doi: 10.1371/journal.pone.0189791
Pemberton, C. L., and Salmond, G. P. C. (2004). The Nep1-like proteins - a growing family of microbial elicitors of plant necrosis. Mol. Plant Pathol. 5, 353–359. doi: 10.1111/j.1364-3703.2004.00235.x
Peškan-Berghöfer, T., Shahollari, B., Giang, P. H., Hehl, S., Markert, C., Blanke, V., et al. (2004). Association of Piriformospora indica with Arabidopsis thaliana roots represents a novel system to study beneficial plant-microbe interactions and involves early plant protein modifications in the endoplasmatic reticulum and at the plasma membrane. Physiol. Plant. 122, 465–477. doi: 10.1111/j.1399-3054.2004.00424.x
Petrášek, J., and Friml, J. (2009). Auxin transport routes in plant development. Development 136, 2675–2688. doi: 10.1242/dev.030353
Pitts, R. J., Cernac, A., and Estelle, M. (1998). Auxin and ethylene promote root hair elongation in Arabidopsis. Plant J. 16, 553–560. doi: 10.1046/j.1365-313x.1998.00321.x
Qi, L., Yan, J., Li, Y., Jiang, H., Sun, J., Chen, Q., et al. (2012). Arabidopsis thaliana plants differentially modulate auxin biosynthesis and transport during defense responses to the necrotrophic pathogen Alternaria brassicicola. New Phytol. 195, 872–882. doi: 10.1111/j.1469-8137.2012.04208.x
Qutob, D., Kemmerling, B., Brunner, F., Kufner, I., Engelhardt, S., Gust, A. A., et al. (2006). Phytotoxicity and innate immune responses induced by Nep1-like proteins. Plant Cell 18, 3721–3744. doi: 10.1105/tpc.106.044180
Rahman, A., Bannigan, A., Sulaman, W., Pechter, P., Blancaflor, E. B., and Baskin, T. I. (2007). Auxin, actin and growth of the Arabidopsis thaliana primary root. Plant J. 50, 514–528. doi: 10.1111/j.1365-313X.2007.03068.x
Rashotte, A. M., Brady, S. R., Reed, R. C., Ante, S. J., and Muday, G. K. (2000). Basipetal auxin transport is required for gravitropism in roots of Arabidopsis. Plant Physiol. 122, 481–490. doi: 10.1104/pp.122.2.481
Reed, R. C., Brady, S. R., and Muday, G. K. (1998). Inhibition of auxin movement from the shoot into the root inhibits lateral root development in Arabidopsis. Plant Physiol. 118, 1369–1378. doi: 10.1104/pp.118.4.1369
Reusche, M., Truskina, J., Thole, K., Nagel, L., Rindfleisch, S., Tran, V. T., et al. (2014). Infections with the vascular pathogens Verticillium longisporum and Verticillium dahliae induce distinct disease symptoms and differentially affect drought stress tolerance of Arabidopsis thaliana. Environ. Exp. Bot. 108, 23–37. doi: 10.1016/j.envexpbot.2013.12.009
Riet, K. B., Ndlovu, N., Piater, L. A., and Dubery, I. A. (2016). Simultaneous analysis of defense-related phytohormones in Arabidopsis thaliana responding to fungal infection. Appl. Plant Sci. 4:1600013. doi: 10.3732/apps.1600013
Salehin, M., Bagchi, R., and Estelle, M. (2015). SCFTIR1/AFB-based auxin perception: mechanism and role in plant growth and development. Plant Cell 27, 9–19. doi: 10.1105/tpc.114.133744
Schäfer, P., Pfiffi, S., Voll, L. M., Zajic, D., Chandler, P. M., Waller, F., et al. (2009). Phytohormones in plant root-Piriformospora indica mutualism. Plant Signal. Behav. 4, 669–671. doi: 10.4161/psb.4.7.9038
Schnathorst, W. C. (1981). “Life cycle and epidemiology of verticillium,” in Fungal Wilt Diseases of Plants, eds M. E. Mace, A. A. Bell, and C. H. Beckman (NewYork, NY: Academic Press Inc), 81–111.
Scholz, S. S., Schmidt-Heck, W., Guthke, R., Furch, A. C. U., Reichelt, M., Gershenzon, J., et al. (2018). Verticillium dahliae-Arabidopsis interaction causes changes in gene expression profiles and jasmonate levels on different time scales. Front. Microbiol. 9:217. doi: 10.3389/fmicb.2018.00217
Shinmen, Y., Shimizu, S., Akimoto, K., Kawashima, H., and Yamada, H. (1989). Production of arachidonic acid by Mortierella fungi: selection of a potent producer and optimization of culture for large-scale production. Appl. Microbiol. Biotechnol. 31, 11–16. doi: 10.1007/BF00252518
Sirrenberg, A., Göbel, C., Grond, S., Czempinski, N., Ratzinger, A., Karlovsky, P., et al. (2007). Piriformospora indica affects plant growth by auxin production. Physiol. Plant. 131, 581–589. doi: 10.1111/j.1399-3054.2007.00983.x
Sukumar, P., Edwards, K. S., Rahman, A., Delong, A., and Muday, G. K. (2009). PINOID kinase regulates root gravitropism through modulation of PIN2-dependent basipetal auxin transport in Arabidopsis. Plant Physiol. 150, 722–735. doi: 10.1104/pp.108.131607
Sun, C., Shao, Y., Vahabi, K., Lu, J., Bhattacharya, S., Dong, S., et al. (2014). The beneficial fungus Piriformospora indica protects Arabidopsis from Verticillium dahliae infection by downregulation plant defense responses. BMC Plant Biol. 14, 268–283. doi: 10.1186/s12870-014-0268-5
Sun, J., Chen, Q., Qi, L., Jiang, H., Li, S., Xu, Y., et al. (2011). Jasmonate modulates endocytosis and plasma membrane accumulation of the Arabidopsis PIN2 protein. New Phytol. 191, 360–375. doi: 10.1111/j.1469-8137.2011.03713.x
Sun, J., Xu, Y., Ye, S., Jiang, H., Chen, Q., Liu, F., et al. (2009). Arabidopsis ASA1 is important for jasmonate-mediated regulation of auxin biosynthesis and transport during lateral root information. Plant Cell 21, 1495–1511. doi: 10.1105/tpc.108.064303
Thaler, J. S., Owen, B., and Higgins, V. J. (2004). The role of the jasmonate response in plant susceptibility to diverse pathogens with a range of lifestyles. Plant Physiol. 135, 530–538. doi: 10.1104/pp.104.041566
Ulmasov, T., Murfett, J., Hagen, G., and Guilfoyle, T. J. (1997). Aux/IAA proteins repress expression of reporter genes containing natural and highly active synthetic auxin response elements. Plant Cell 9, 1963–1971. doi: 10.1105/tpc.9.11.1963
Vadassery, J., Ritter, C., Venus, Y., Camehl, I., Varma, A., Shahollari, B., et al. (2008). The role of auxins and cytokinins in the mutualistic interaction between Arabidopsis and Piriformospora indica. Mol. Plant Microbe Interact. 21, 1371–1383. doi: 10.1094/MPMI-21-10-1371
Vahabi, K., Reichelt, M., Scholz, S. S., Furch, A. C. U., Matsuo, M., Johnson, J. M., et al. (2018). Alternaria Brassicae induces systemic jasmonate responses in Arabidopsis which travel to neighboring plants via a Piriformospora indica hyphal network and activate abscisic acid responses. Front. Plant Sci. 9:626. doi: 10.3389/fpls.2018.00626
van der Does, H. C., and Rep, M. (2007). Virulence genes and the evolution of host specificity in plant-pathogenic fungi. Mol. Plant Microbe Interac. 20, 1175–1182 doi: 10.1094/MPMI-20-10-1175
van Esse, H. P., Fradin, E. F., de Groot, P. J., de Wit, P. J., and Thomma, B. P. (2009). Tomato transcriptional responses to a foliar and a vascular fungal pathogen are distinct. Mol. Plant Microbe Interact. 22, 245–258. doi: 10.1094/MPMI-22-3-0245
Verma, S., Varma, A., Rexer, K.-H., Hassel, A., Kost, G., Sarbhoy, A., et al. (1998). Piriformospora indica, gen. et sp. nov., a new root-colonizing fungus. Mycologia 90, 898–905. doi: 10.1080/00275514.1998.12026983
Wang, D., Pajerowska-Mukhtar, K., Culler, A. H., and Dong, X. (2007). Salicylic acid inhibits pathogen growth in plants through repression of the auxin signaling pathway. Curr. Biol. 17, 1784–1790. doi: 10.1016/j.cub.2007.09.025
Wani, Z. A., Kumar, A., Sultan, P., Bindu, K., Riyaz-Ul-Hassan, S., and Ashraf, N. (2017). Mortierella alpina CS10E4, an oleaginous fungal endophyte of Crocus sativus L. enhances apocarotenoid biosynthesis and stress tolerance in the host plant. Sci. Rep. 7:8598. doi: 10.1038/s41598-017-08974-z
Xu, L., Wu, C., Oelmüller, R., and Zhang, W. (2018). Role of phytohormones in Piriformospora indica-induced growth promotion and stress tolerance in plants: more questions than answers. Front. Microbiol. 9:1646. doi: 10.3389/fmicb.2018.01646
Zhang, W., Wang, J., Xu, L., Wang, A., Huang, L., Du, H., et al. (2017). Drought stress responses in maize are diminished by Piriformospora indica. Plant Signal. Behav. 13:e1414121. doi: 10.1080/15592324.2017.1414121
Keywords: auxin, phytohormones, plant-fungus interaction, Piriformospora indica, Mortierella hyalina, Alternaria brassicicola, Verticillium dahliae, light sheet fluorescence microscopy
Citation: Meents AK, Furch ACU, Almeida-Trapp M, Özyürek S, Scholz SS, Kirbis A, Lenser T, Theißen G, Grabe V, Hansson B, Mithöfer A and Oelmüller R (2019) Beneficial and Pathogenic Arabidopsis Root-Interacting Fungi Differently Affect Auxin Levels and Responsive Genes During Early Infection. Front. Microbiol. 10:380. doi: 10.3389/fmicb.2019.00380
Received: 08 November 2018; Accepted: 13 February 2019;
Published: 12 March 2019.
Edited by:
Eloise Foo, University of Tasmania, AustraliaReviewed by:
Jason Liang Pin Ng, Australian National University, AustraliaAntonio Figueira, University of São Paulo, Brazil
Philip B. Brewer, University of Adelaide, Australia
Copyright © 2019 Meents, Furch, Almeida-Trapp, Özyürek, Scholz, Kirbis, Lenser, Theißen, Grabe, Hansson, Mithöfer and Oelmüller. This is an open-access article distributed under the terms of the Creative Commons Attribution License (CC BY). The use, distribution or reproduction in other forums is permitted, provided the original author(s) and the copyright owner(s) are credited and that the original publication in this journal is cited, in accordance with accepted academic practice. No use, distribution or reproduction is permitted which does not comply with these terms.
*Correspondence: Ralf Oelmüller, YjdvZXJhQHVuaS1qZW5hLmRl