- 1Institute of Microbiology of the CAS, v. v. i., Prague, Czechia
- 2Centrum für Biotechnologie, Universität Bielefeld, Bielefeld, Germany
- 3Institute of Physics, Faculty of Mathematics and Physics, Charles University, Prague, Czechia
Corynebacterium glutamicum ATCC 13032 harbors five sigma subunits of RNA polymerase belonging to Group IV, also called extracytoplasmic function (ECF) σ factors. These factors σC, σD, σE, σH, and σM are mostly involved in stress responses. The role of σD consists in the control of cell wall integrity. The σD regulon is involved in the synthesis of components of the mycomembrane which is part of the cell wall in C. glutamicum. RNA sequencing of the transcriptome from a strain overexpressing the sigD gene provided 29 potential σD-controlled genes and enabled us to precisely localize their transcriptional start sites. Analysis of the respective promoters by both in vitro transcription and the in vivo two-plasmid assay confirmed that transcription of 11 of the tested genes is directly σD-dependent. The key sequence elements of all these promoters were found to be identical or closely similar to the motifs -35 GTAACA/G and -10 GAT. Surprisingly, nearly all of these σD-dependent promoters were also active to a much lower extent with σH in vivo and one (Pcg0607) also in vitro, although the known highly conserved consensus sequence of the σH-dependent promoters is different (-35 GGAAT/C and -10 GTT). In addition to the activity of σH at the σD-controlled promoters, we discovered separated or overlapping σA- or σB-regulated or σH-regulated promoters within the upstream region of 8 genes of the σD-regulon. We found that phenol in the cultivation medium acts as a stress factor inducing expression of some σD-dependent genes. Computer modeling revealed that σH binds to the promoter DNA in a similar manner as σD to the analogous promoter elements. The homology models together with mutational analysis showed that the key amino acids, Ala 60 in σD and Lys 53 in σH, bind to the second nucleotide within the respective -10 promoter elements (GAT and GTT, respectively). The presented data obtained by integrating in vivo, in vitro and in silico approaches demonstrate that most of the σD-controlled genes also belong to the σH-regulon and are also transcribed from the overlapping or closely located housekeeping (σA-regulated) and/or general stress (σB-regulated) promoters.
Introduction
Most bacterial genes are transcribed by an RNA polymerase (RNAP) holoenzyme that includes a primary sigma subunit (σ factor) responsible for the transcription of housekeeping genes. In addition to the primary σ factor, a number of alternative sigma factors control various functions of the bacterial cells under specific nutritional, growth and environmental conditions. Corynebacterium glutamicum is a Gram-positive bacterium, which is applied in industrial biotechnology and also considered a model species for other corynebacteria as well as related actinobacterial genera such as Mycobacterium and Rhodococcus. The C. glutamicum genome encodes 7 σ factors: A primary σA, a primary-like σB and five σ factors of Group IV (Gruber and Gross, 2003) also called extracytoplasmic function (ECF) σ factors (σC, σD, σE, σH, and σM), which are involved in various stress responses.
Gene expression in bacteria is controlled by a complex regulatory network composed of protein transcription regulators, regulatory RNAs as well as low-molecular-weight metabolites. Sigma subunits of RNAP that are responsible for transcription initiation play an important role in this network. Groups of genes (σ factor regulons, called also sigmulons) controlled by different σ factors were found to constitute a modular network in Pseudomonas aeruginosa (Schulz et al., 2015). Individual separated sigmulons controlled by more than 25 σ factors display limited overlaps which probably allows this versatile bacterium to efficiently regulate cell processes and adapt to various environments as well as to the lifestyle of an opportunistic pathogen. The functions of the regulons of alternative σ factors in P. aeruginosa are significantly connected by the action of global transcriptional regulators which modulate transcription levels from the respective promoters (Binder et al., 2016). According to this hypothesis, modularity of regulatory networks may ensure rapid evolution (Binder et al., 2016). In contrast to previous notion concerning P. aeruginosa, considerable overlaps of σ factor regulons observed in many bacteria display regulatory redundancies and the ability to fine-tune responses to various environmental stimuli and combined stress conditions. Some genes belong to two or more sigmulons, since they are either transcribed from several promoters recognized by different alternative σ factors, or are transcribed with two or more different holoenzymes (RNAP core+σ) which drive transcription from the same promoter (Luo and Helmann, 2009). Microarray analysis revealed extensive coregulation of the genes involved in many cell processes, which are co-operatively controlled by four alternative σ factors (σB, σC, σH, and σL) in Listeria monocytogenes (Chaturongakul et al., 2011). Significant regulatory overlaps between the target genes of three σ factors (σM, σW, and σX) involved in cell surface stress responses were also found in Bacillus subtilis (Jordan et al., 2008). A single promoter of the sigB gene encoding an alternative σ in Mycobacterium tuberculosis was recognized by as many as three different ECF σ factors (σE, σH, and σL) (Dainese et al., 2006). Overlapping σ factor specificity in promoter recognition can be therefore considered to be a common feature of regulatory networks in bacteria.
Various types of stress conditions which bacteria encounter in the environment (e.g., heat, cell surface and oxidative stresses) often result in damaged proteins, which are then degraded by proteases or re-folded by chaperones during the stress response. Expression of some genes encoding proteases (clpB) and chaperones (dnaK and dnaJ2) was found to be activated by both σH (involved in heat and oxidative stress response) and σE (involved in cell surface stress response) in C. glutamicum (Šilar et al., 2016). The clgR gene encoding a heat-stress-responsive regulator was also found to be σH and σE-dependent (Šilar et al., 2016). ClgR controls expression of several genes involved in protein quality control, and the function of ECF σ factors and the transcriptional regulator is thus integrated into a network. The same σH/σE overlap was found in the transcription initiation of the C. glutamicum sigB gene, encoding the general stress response sigma factor σB (Dostálová et al., 2017). The possible activity of both σH and σM (involved in oxidative stress response) at the same C. glutamicum promoters was suggested, but has not yet been proven (Nakunst et al., 2007; Šilar et al., 2016; Dostálová et al., 2017), although the respective promoter consensus sequences seem very similar. Promoters of σC-regulated genes in C. glutamicum were found to be different from other promoters recognized by ECF σ factors, and σC regulon, which includes genes involved in the aerobic respiratory chain in C. glutamicum, seems to be separated from the other σ factor regulons (Toyoda and Inui, 2016).
Many stress response genes of C. glutamicum were found to be transcribed not only from stress promoters, but also from overlapping or closely located housekeeping promoters controlled by σA and/or σB (Engels et al., 2004; Ehira et al., 2009; Busche et al., 2012; Šilar et al., 2016). Such transcription of stress-response genes from multiple promoters also seems to be frequent in other bacteria (Seo et al., 2012; Cho et al., 2014). The activity of σA and/or σB under unstressed conditions probably ensures a basal level of expression of the respective genes which also play important roles in fast-growing bacterial cells.
We have recently found by using RNA-seq that σD-dependent genes are involved in the synthesis of components of the mycomembrane, which is a part of the cell wall in C. glutamicum ATCC 13032. The σD regulon, including 29 genes, thus contributes to the maintenance of cell wall integrity (Taniguchi et al., 2017). Similar group of σD-dependent genes was also detected in the strain C. glutamicum R using different techniques (Toyoda and Inui, 2018). In C. glutamicum R, the sigD overexpression conferred lysozyme resistance and the lppS gene (encoding L,D-transpeptidase) and probably some other σD-dependent genes were found to contribute to lysozyme resistance. No clear σD regulon induction was observed in this strain under any other stress conditions tested (treatment with sodium dodecyl sulfate, cetyl trimethylammonium bromide, ethambutol or ampicillin) (Toyoda and Inui, 2018).
Phenol was previously described to cause a significant oxidative stress at subtoxic concentrations. At higher concentrations, phenol affects bacterial cells by releasing cell wall components and causing non-specific increase in cell permeability (Denyer, 1995). At the level of the cytoplasmic membrane, phenol induces a loss of structural integrity by the leakage of potassium ions and various organic compounds and can also displace phospholipid molecules in the cell envelope (Denyer, 1995). Such damage to the cell wall might induce a similar stress response as lysozyme treatment. However, effects of toxic aromatic compounds, such as phenol, vanillin and ferulic acid, which are degraded by C. glutamicum (Chen et al., 2016, 2017, 2018), on σD regulon induction have not yet been tested.
Although the number of precisely localized promoters driving expression of σD-dependent genes was not high, it was possible to propose their consensus sequence. Considering the difference between σD-controlled promoters and other promoter classes, the σD regulon seemed to also be an insulated gene group.
In this study, we discovered that nearly all of the σD-dependent promoters found in C. glutamicum ATCC 13032 are also to a much lower extent active with RNAP+σH, although the known highly conserved consensus sequence of the σH-dependent promoters differs from that of the σD-dependent promoters. We have found that phenol in the cultivation medium acts as a stress factor, inducing the expression of some σD-dependent genes. To achieve reliable results, we combined the genome-wide technique (RNA-seq) and single-promoter analysis (in vitro transcription and an in vivo two-plasmid system for the assignment of σ factors to genes/promoters). In silico homology modeling completed the different approaches to analyzing the sigma-promoter relationships.
Materials and Methods
Bacterial Strains, Plasmids, Oligonucleotides and Growth Conditions
Escherichia coli DH5α (Hanahan, 1985) was cultivated aerobically in 500-ml flasks containing 70–100 ml of 2xYT medium (Green and Sambrook, 2012) at 37°C. Wild-type (WT) C. glutamicum ATCC 13032, its deletion derivative C. glutamicum ΔsigD (Taniguchi et al., 2017) and C. glutamicum sigD-overexpressing strain (Taniguchi et al., 2017) were used for DNA and RNA isolations, and as hosts for testing the activities of promoters cloned in the promoter-test vector pEPR1. C. glutamicum was cultivated aerobically in 500-ml flasks with 70–100 ml of complete 2xYT medium or in minimal CGXII medium (Keilhauer et al., 1993) at 30°C. Media were supplemented with antibiotics, when necessary: kanamycin (Km; 30 μg/ml), tetracycline (Tc; 10 μg/ml) or ampicillin (Ap; 100 μg/ml). The plasmid vectors used are listed in Table 1. The oligonucleotides used are listed in Supplementary Table S1.
DNA Manipulations
DNA isolation, PCR, cutting by restriction enzymes, ligation and transformation of E. coli were done using the standard techniques (Green and Sambrook, 2012). C. glutamicum cells were transformed by electroporation (van der Rest et al., 1999). DNA fragments for cloning promoters in pRLG770 and pEPR1 were assembled from the synthetized complementary oligonucleotides of around 75 nt in length, with overhangs ready for ligation. Their sequences are shown in Supplementary Table S1. Mutations in sigH were constructed using a Q5® Site-Directed Mutagenesis Kit (New England BioLabs® Inc.) according to the recommendations of the manufacturer. The specific oligonucleotide primers for mutagenesis were designed by NEBaseChangerTM v1.2.8 (New England BioLabs® Inc.).
RNA Isolation, cDNA Library Preparation and Sequencing
Corynebacterium glutamicum ATCC 13032 was cultivated in minimal medium CGXII with glucose (2%) or phenol (3.4 mM). The sigD-overexpressing C. glutamicum strain was used as described previously (Taniguchi et al., 2017). Total RNA was isolated from 3 biological replicates of C. glutamicum cells grown to the exponential phase using a Quick-RNA Miniprep Plus kit according to the manufacturer’s instructions (Zymo Research). After additional DNase treatment, RNA samples were purified with an RNA Clean&Concentrator-5 kit (Zymo Research) and quantified with a DropSense 16 (Trinean). The quality of total RNA was controlled with an RNA 6000 Nano kit in an Agilent 2100 Bioanalyzer (Agilent Technologies). To construct the whole transcriptome cDNA library, 2.5 μg total RNA (RIN > 9) was used for the depletion of rRNA with a Ribo-Zero rRNA Removal Kit (Bacteria) according to manufacturer’s instructions (Illumina). The rRNA removal was checked with an Agilent RNA Pico 6000 kit and the Agilent 2100 Bioanalyzer (Agilent Technologies). The mRNA obtained was converted to a cDNA library according to the TruSeq Stranded mRNA Sample Preparation guide (Illumina). The quality and quantity of the cDNA library was checked with an Agilent High Sensitivity DNA kit and the Agilent 2100 Bioanalyzer (Agilent Technologies). Sequencing was performed on an Illumina HiSeq 1500 using 70 bases read length (Illumina).
A primary 5′-end specific cDNA library was synthesized using 2 × 2.5 μg total RNA (RIN > 9) as described previously (Kranz et al., 2018). Briefly, after rRNA depletion and quality control, as described for the whole transcriptome cDNA libraries, a terminator 5′-phosphate-dependent exonuclease treatment (TEN, Illumina) was carried out to digest non-primary transcripts. The remaining non-digested non-primary transcripts were tagged by the ligation of the RNA 5′-index adapter 5′-CCCUACACGACGCUCUUCCGAUCGAG-UACCCUAG (index in bold) to the 5′-monophosphorylated ends. The primary 5′-triphosphate ends were converted to 5′-mono-phosphate by RNA 5′-polyphosphatase (RPP) treatment (Epicenter) to ligate the 5′-adapter to the 5′-ends of primary transcripts. Finally, reverse transcription with a stem-loop DNA adapter and library amplification was performed. The quality and quantity of the cDNA library was checked in the same way as for the whole transcriptome cDNA library. Prior to sequencing, primary 5′-end cDNA libraries were purified and size-selected for fragments approximately 100–1000 bases long via gel electrophoresis, and quantified again. Paired end sequencing was performed in an Illumina MiSeq using MiSeq Reagent Kits v3 with a read length of 2 × 75 bases (Illumina).
Read Processing, Mapping and Visualization
Paired-end reads were mapped to the C. glutamicum ATCC 13032 reference genome sequence accession number BX927147 (Kalinowski et al., 2003) with bowtie2 v2.2.7 (Langmead and Salzberg, 2012) using the default settings for paired-end read mapping.
False-positive primary transcriptome cDNA reads containing the barcode sequence TACCCTAG at their 5′-ends were discarded. The remaining R1 cDNA reads were mapped to the C. glutamicum ATCC 13032 reference genome sequence accession number BX927147 (Kalinowski et al., 2003) with bowtie2 v2.2.7 (Langmead and Salzberg, 2012) using the default settings for single-end read mapping. Read Explorer v.2.2 (Hilker et al., 2014) was used for the visualization of short read alignments, transcription start site (TSS) detection and differential gene expression analysis.
Identification of Transcription Start Sites (TSS)
Transcription start sites (TSS) were detected essentially as described by Wittchen et al. (2018). Primary 5′-end cDNA library data were analyzed with the software ReadXplorer v2.2 (Hilker et al., 2016) using the Transcription analysis function. The parameters for TSS detection were chosen by ReadXplorer using its automatic parameter estimation. TSS were detected with at least 10 read starts and a minimal coverage increase of 100%. The resulting list of predicted TSS was manually checked for false-positives.
Differential Gene Expression Analysis
Differential gene expression analysis of C. glutamicum grown with and without phenol (3.4 mM), including normalization, was performed using the whole transcriptome data and Bioconductor package DESeq2 (Love et al., 2014) included in the software ReadXplorer v2.2 (Hilker et al., 2016). The signal intensity value (a-value) was calculated by the log2 mean of normalized read counts, and the signal intensity ratio (m-value) by log2 fold-change. The evaluation of the differential RNA-seq data was performed using an adjusted p-value cut-off of P ≤ 0.01 and a signal intensity ratio (m-value) cut-off of ≥1 or ≤-1.
In vitro Transcription
The in vitro transcription assay was carried out essentially as described previously (Holátko et al., 2012). The holo-RNAP was reconstituted from the RNAP core enzyme isolated from C. glutamicum and individual C. glutamicum sigma factors isolated as His-tagged recombinant proteins from E. coli. The RNAP core (100 nM) was mixed with the respective σ factor (σC, σD, σE, σH, or σM) in a molar ratio of 1:30. The holo-RNAP was assembled for 10 min at 37°C. The transcription mixture was incubated for 15 min at 37°C. The transcripts labeled with [α-32P]UTP were separated in 5.5% polyacrylamide gel. In vitro transcription assays were done 2 or 3 times for each promoter, with essentially the same results.
Promoter Activity Measurements Using the Two-Plasmid System
Sigma factors were assigned to individual promoters in vivo using the two-plasmid system for C. glutamicum described recently (Dostálová et al., 2017) and similar that developed for the identification of σE-dependent promoters in E. coli (Rezuchova and Kormanec, 2001). In principle, the sigma factors overproduced using the expression vector pEC-XT99A drove transcription from the individual promoters transcriptionally fused to the gfpuv reporter gene in the other plasmid present in the cell, the promoter-test vector pEPR1. Promoters were cloned to pEPR1 as approximately 75-nt fragments using the restriction sites PstI and BamHI. The same pEC-XT99A constructs as described previously (Dostálová et al., 2017) were used to overexpress the sig genes after the addition of isopropyl-β-thiogalactopyranoside (IPTG). Two-plasmid strains were cultivated in 2xYT medium (containing Km and Tc) for 24 h and the cell samples were collected. The cells were disrupted with a FastPrep homogenizer (MP Biomedicals). The fluorescence of the cell-free extracts was determined with a Saphire2 microplate spectrophotometer (Tecan; excitation wavelength 397 nm; emission wavelength 509 nm). Cells harboring the pEPR1 construct and the expression vector without a sig gene were used as a control. To determine the background fluorescence of C. glutamicum cells, the strain only carrying the promoter-less vector pEPR1 was used. Protein concentration in the extract was determined by Bradford assay, and promoter activity was expressed in arbitrary units/mg protein.
Homology Modeling and Molecular Dynamics (MD) Simulations
The homology models of the σD and σH domains which recognize the -10 and -35 sequences of the respective promoters were produced by using the Swiss-Model server (Waterhouse et al., 2018). The crystal structures of E. coli σE, PDBid: 4LUP (for -10 element GTC) (Campagne et al., 2014) and PDBid: 2H27 (for -35 element GGAAC) (Lane and Darst, 2006) were used as templates. The nucleotides within the E. coli σE consensus were replaced to match the consensus for C. glutamicum σH or σD, where necessary. Molecular dynamics simulations were done using the software package AMBER (Salomon-Ferrer et al., 2013) and Linux computer nodes with powerful NVIDIA GPUs that enable the accumulation of 50-ns MD trajectories at 280 K.
Results
Mapping of Transcription Start Sites of σD-Dependent Genes by RNA-seq
RNA-seq with a C. glutamicum WT strain, a strain overexpressing sigD and a sigD deletion (ΔsigD) strain was performed previously (Taniguchi et al., 2017). Using this approach, the 29 genes were identified, which showed the increased transcription in C. glutamicum overexpressing sigD and decreased transcription in the ΔsigD strain. The genes selected may be those which are directly under the control of σD, or the genes whose transcription is influenced by an indirect effect of sigD overexpression or deletion.
To further investigate these σD-dependent genes and to map their transcriptional start sites (TSS), RNA-seq of 5′-enriched primary transcripts of C. glutamicum overexpressing sigD was used. The sequencing of primary 5′-end specific cDNA library allows the exact mapping of TSS. To detect these TSS, the data obtained from the primary 5′-end cDNA sequencing were mapped to C. glutamicum ATCC 13032 reference genome sequence, accession number BX927147 (Kalinowski et al., 2003) and analyzed via ReadXplorer (Hilker et al., 2016). To distinguish between the background and actual TSS signal, we considered a position to be +1 (TSS) if the number of read starts here was 10 times higher than at position -1. In this way the 5′- ends of the transcripts of the above mentioned 29 putative σD-dependent genes were detected. Since several genes were cotranscribed in operons, the total number of TSS was 23 (Supplementary Table S2). The 50-nt sequences (-49 to +1) were aligned at the TSS, and the consensus motifs within the region of the supposed promoters were searched using the software Improbizer (Ao et al., 2004) as described previously (Albersmeier et al., 2017). The consensus sequence or closely similar motifs within the -35 and -10 regions were found in 11 sequences (Table 2). The products of the respective genes are involved in the synthesis of corynomycolic acid (fadD2, cmt1, cmt2, cmt3), peptidoglycan formation (lppS), inhibition of σD activity (rsdA) or have hypothetical functions (Table 3). The σD is thus involved in the control of cell wall integrity and cell envelope stress response, as described previously in the strains C. glutamicum ATCC 13032 (Taniguchi et al., 2017) and C. glutamicum R (Toyoda and Inui, 2018).
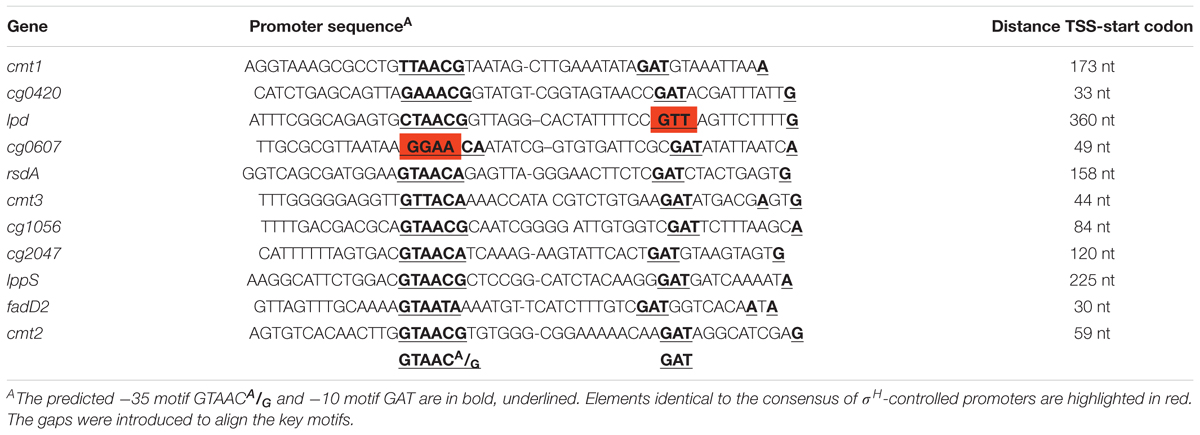
Table 2. Promoter sequences of σD-dependent genes in C. glutamicum ATCC 13032 determined by RNA sequencing specific for transcription start sites.
The sequences were used to generate a sequence logo with Weblogo3 (Figure 1). The consensus sequences GTAACA/G as the -35 motif and GAT as the -10 motif are apparent.

Figure 1. Sequence logo of the 11 studied σD-dependent promoters. The logo was made using Weblogo3 (Crooks et al., 2004).
In vitro Transcription From the σD-Controlled Promoters
The activity of the 11 proposed σD-dependent promoters, which were deduced from the TSS determination by RNA-seq, was tested using the in vitro transcription system (Holátko et al., 2012). The promoter sequence elements of the proposed σD-dependent promoters were apparently different from the consensus sequence of σA/σB-driven promoters (Pátek and Nešvera, 2011; Albersmeier et al., 2017) and therefore ECF σ factors (σC, σD, σE, σH, and σM) were only used. This assay may directly prove whether a promoter is specifically recognized by RNAP associated with the chosen σ factor, and thus distinguish between direct and indirect control by the tested σ. As shown in Figure 2, all but one promoter were found to be exclusively σD-specific. The only exception was the promoter of cg0607 (Pcg0607), which was active with both σD and σH. The in vitro transcript level with σH was even higher than with σD (Figure 2). It is worth mentioning that Pcg0607 is the only one out of the 11 analyzed promoters that has the -35 sequence element GGAAC which is a consensus for σH-dependent promoters (Busche et al., 2012). The ECF σ factors σC, σE, and σM did not provide any signal in the assays (data not shown). The σH-specific clpP1 promoter (Busche et al., 2012) was used as a control.
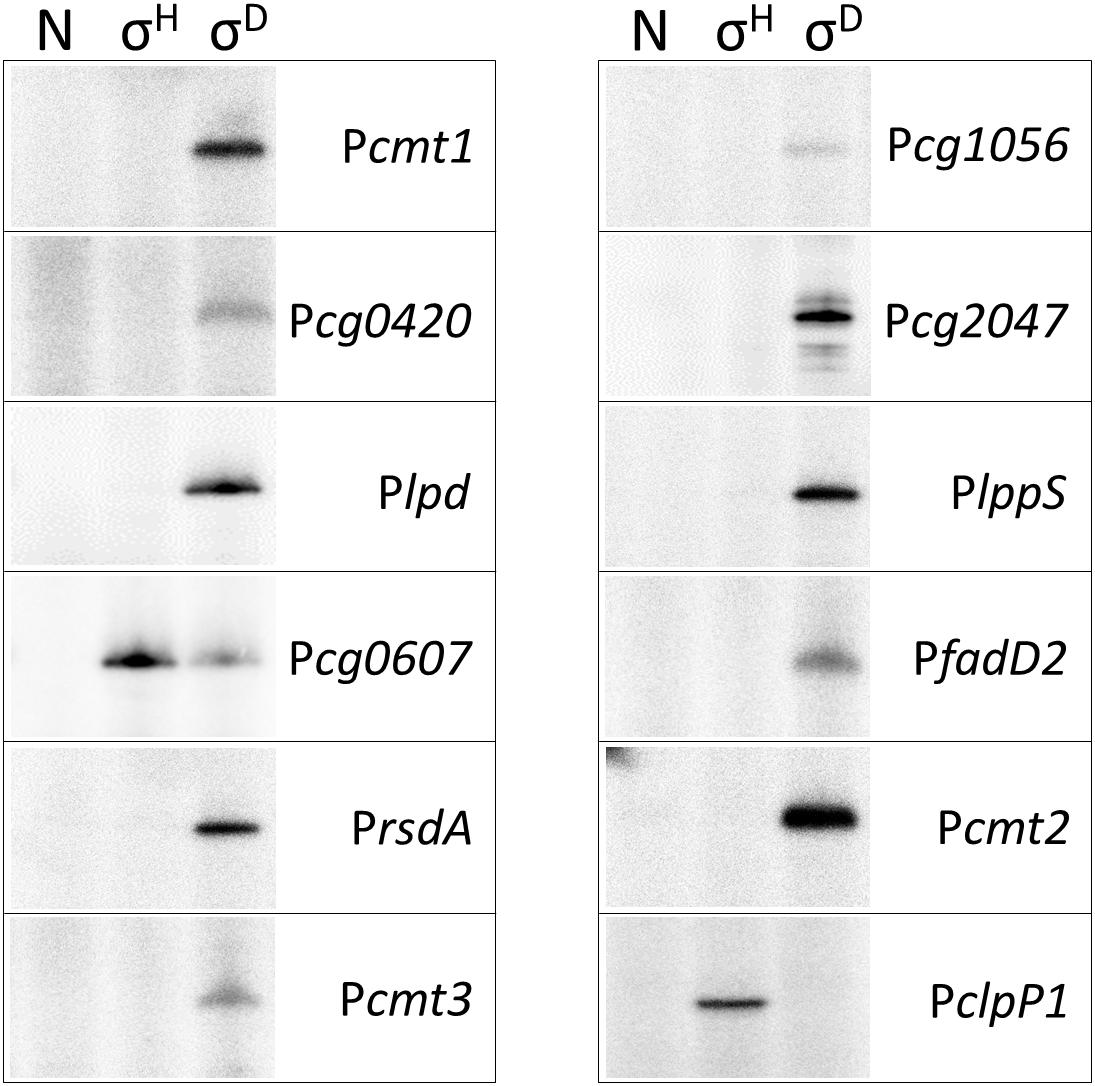
Figure 2. In vitro transcription driven from σD-dependent promoters with reconstituted C. glutamicum holo-RNAPs (autoradiogram of the SDS-PAGE gel). The lanes with no sigma (N), σH and σD are shown. In vitro transcription was carried out as described previously (Holátko et al., 2012). The derivatives of the plasmid vector pRLG770 with inserted promoter fragments (approximately 75 nt) were used as DNA templates, recombinant RNAP core was isolated from C. glutamicum, and sigma factors were isolated as His-tagged proteins from E. coli using the pET-22b(+) constructs carrying the respective inserted sig genes (Holátko et al., 2012; Šilar et al., 2016). The σH-specific promoter PclpP1 was used as a control. Representative results of 2–3 assays are shown.
Determination of Activity of σD-Controlled Promoters in vivo Using Two-Plasmid System
To confirm the results of in vitro transcription, the activity of σD-dependent promoters was determined in vivo using the two-plasmid C. glutamicum cells carrying a sig gene in the expression vector pEC-XT99A and the analyzed promoter in the promoter-test vector pEPR1 (Dostálová et al., 2017). The promoter activity was measured as the fluorescence intensity of the Gfp reporter protein. The results of the assays with 7 most felicitous example promoters which confirmed the activity of σH in transcription are presented in Figure 3. As shown in the left part of Figure 3, all promoters exhibited high activity with the overexpressed sigD. Results with Pcg1056 were included to document that not all σD-controlled promoters are also recognized by σH, although there is no apparent reason found by inspection of the promoter sequence only. Activity of Pcg2047 with σH was approximately twice higher after induction at time point 3 (T3) and time point 6 (T6) although it was at the same level of the control. It is an unusual feature of this two-plasmid system in some cases, which we described in our previous paper (Dostálová et al., 2017). We concluded, therefore, that there was an activity of Pcg2047 initiated by σH.
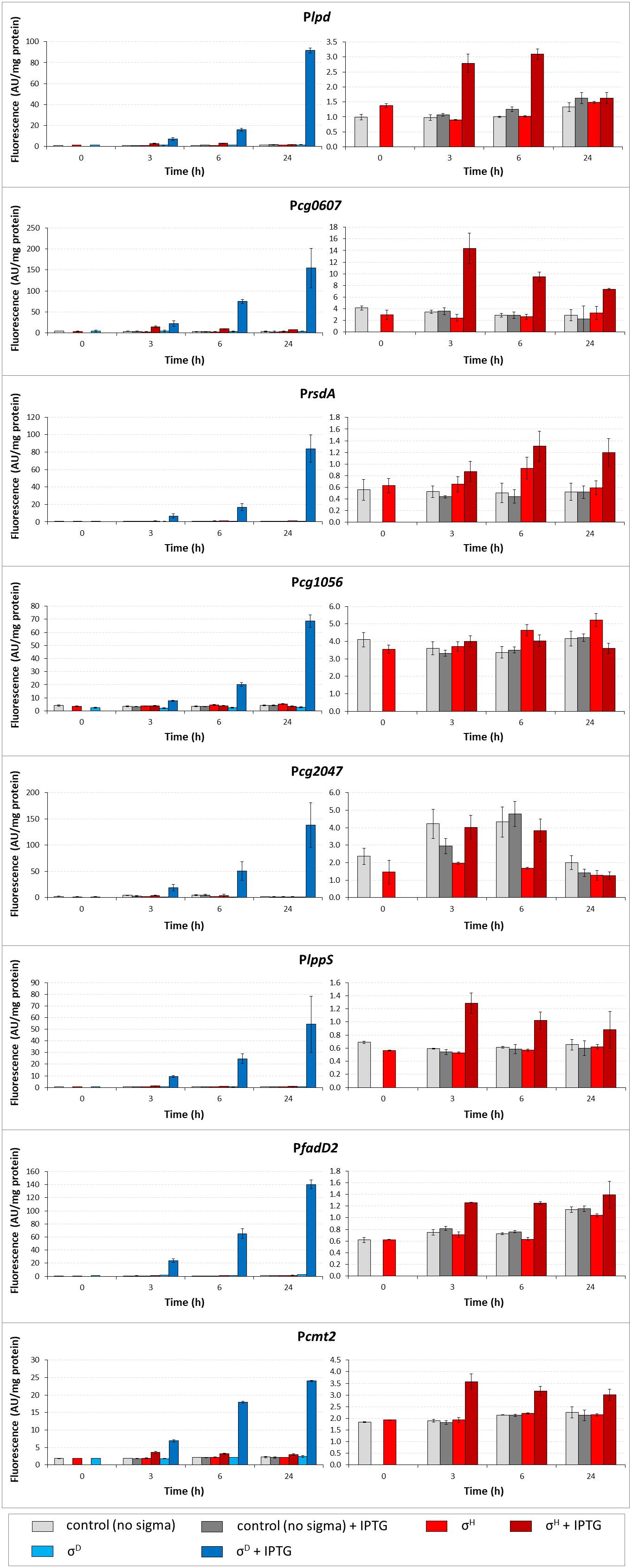
Figure 3. Determination of promoter activity under sigD or sigH overexpression conditions using the two-plasmid assay. Promoter activity was measured as Gfpuv fluorescence intensity of cell extracts shown as bars in colors representing respective sigma factors. The C. glutamicum strains carried the pEC-XT99A constructs overexpressing sigD (blue bars) or sigH (red bars) after IPTG addition (added at time point 0, dark bars) and the promoter-test vector pEPR1 carrying the reporter gfpuv gene downstream of the target promoter (Plpd, Pcg0607 PrsdA, Pcg1056, Pcg2047, PlppS, PfadD2, or Pcmt2). The strains harboring the pEPR1 with the target promoters and empty pEC-XT99A were used as the controls (gray bars). Only the σH-induced activity and controls are shown in the right panels to emphasize the minor recognition of σD-controlled promoters by σH. The background fluorescence intensity with control cells only carrying the empty vector pEPR1 was 0.25 ± 0.09 in all cases. AU, arbitrary units. The standard deviations of three biological replicates are depicted by error bars.
The level of Gfp reporter fluorescence was lowest 3 h (T3) after the addition of the inducer (IPTG) in all cases and highest after 24 h (T24) in 10 of the 11 tested promoters. The promoters Pcmt2, PfadD2, PrsdA, Pcg2047, PlppS, Plpd, and Pcg0607 were also found to be active with σH, as shown in the right part of Figure 3. Although the maximal σH-induced activities were 1.5- to 64-fold lower than those with σD under the conditions used, the effect was apparent (Figure 3, right part). The promoter Pcg1056 is an example of a promoter which did not demonstrate any increase in activity with sigH overexpression. Neither of the other overproduced σ factors supported a promoter activity increase with any of the promoters tested (data not shown). Interestingly, the trend of changes in the σH-directed promoter activity was mostly the opposite of that with σD: the activity with σH was highest 3 h after the addition of IPTG, then mostly decreased at T6, and the difference in Gfp level between the cells with IPTG-induced and non-induced σH-overproduction nearly vanished at T24 in most cases (Figure 3, right part). Due to this trend, σD- and σH-incited promoter activities were nearly comparable at T3. This effect was most apparent in Pcg0607, which was also found to be active with σH by in vitro assay (Figure 2): this ratio between promoter activity with σD/σH was 1.5 at T3 but 20.5 at T24 (Figure 3). The overall conclusion is that most of the σD-controlled promoters are also to a lesser extent σH-activated.
Promoter Activity in the sigD Deletion Strain (ΔsigD)
To prove that σH is directly responsible for the minor activity of σD-controlled promoters in vivo, we tried to overexpress sigH in the ΔsigD strain also harboring pEPR1 with the target promoters. The ΔsigD strain grew slightly slower than the wild type strain (Taniguchi et al., 2017). However, the ΔsigD clones carrying two vector constructs grew extremely poorly. Therefore, we only succeeded in testing the clones with Pcmt2, PlppS, and PrsdA (cloned in pEPR1), moreover, only on a solid agar medium. The increase in promoter activity, (induction ratio of WT and ΔsigD strains with sigH overexpressed), was 2.1 and 1.8, respectively, for Pcmt2, 2.2 and 3.5, respectively, for PlppS and 1.1 and 6.1, respectively, for PrsdA (Figure 4). Thus, RNAP+ σH was apparently directly active in transcription from all three tested σD-dependent promoters.
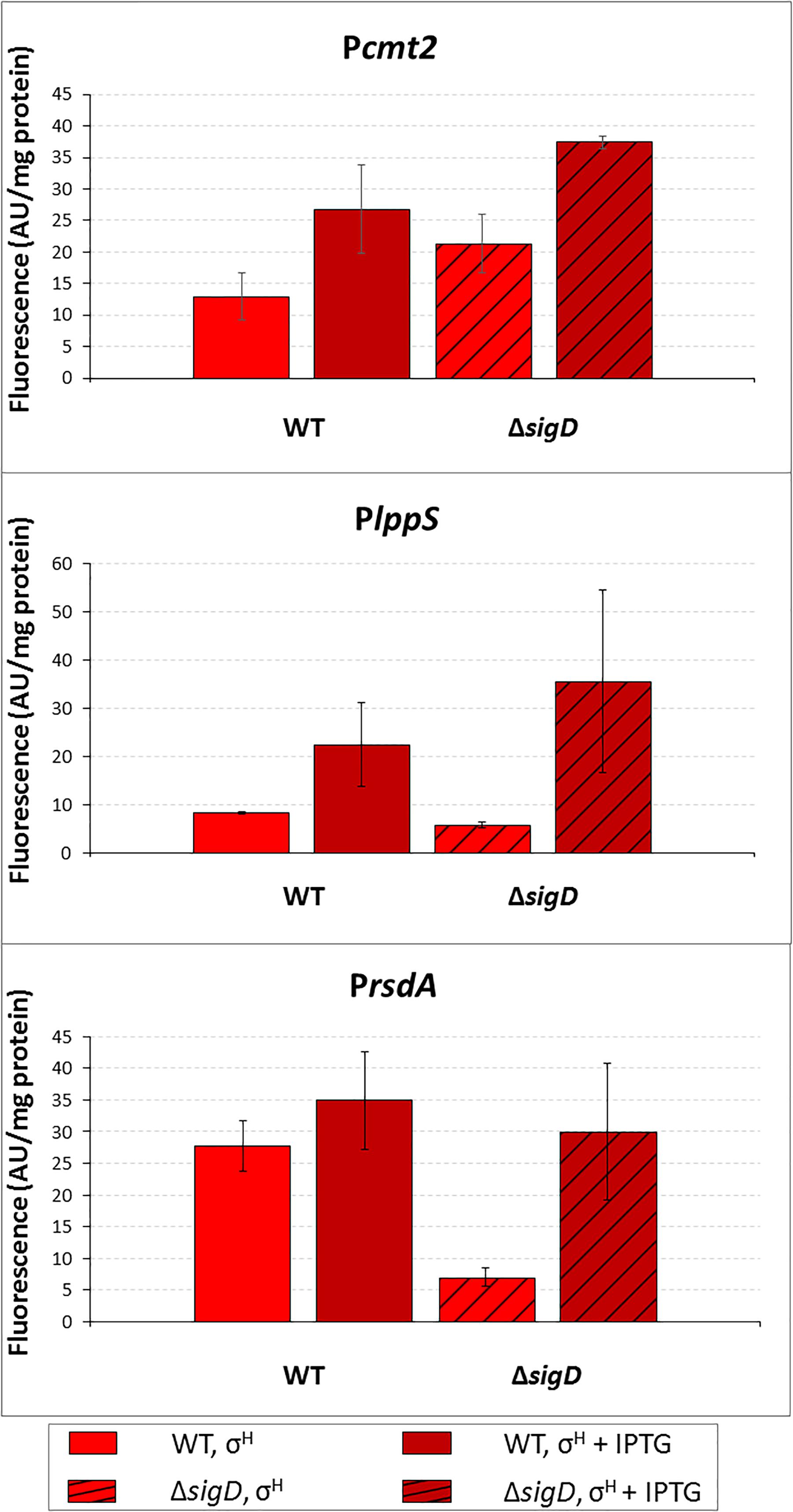
Figure 4. Determination of promoter activity in C. glutamicum WT and ΔsigD strains under sigH overexpression conditions using the two-plasmid assay. The C. glutamicum strains carried the vector pEC-XT99A construct overexpressing sigH with IPTG and the promoter-test vector pEPR1 with a target promoter Pcmt2, PlppS or PrsdA. The promoter activity was measured as the Gfpuv fluorescence intensity of the cell extracts after 6-h growth on complete agar plates with or without IPTG. Fluorescence of cultures without IPTG are shown as light bars; fluorescence of cultures with IPTG induction are shown as dark bars. Open bars depict fluorescence of the strains with WT background, hatched bars depict fluorescence of the strains with the ΔsigD background. AU, arbitrary units. The standard deviations of three biological replicates are depicted by error bars.
Analysis of C. glutamicum σD and σH Protein Sequence Similarity
To explain the observed function of σH in transcription from some σD-dependent promoters, the similarity of the amino acid sequences of C. glutamicum σD and σH proteins was analyzed. The level of overall similarity of the σD and σH sequences is very low (<25% identical AA), in contrast to C. glutamicum ECF sigma factors σH, σE, and σM, which exhibit a 25–38% mutual identity of AAs. However, when the regions which are thought to interact with the -35 and -10 promoter sequences (Lane and Darst, 2006; Campagne et al., 2014) were compared, some AAs were found to be identical or similar in σD and σH (shown in green and yellow, respectively, in Figure 5). As shown in Figure 5, majority of these AAs were also found to be conserved in the model ECF sigma factor, σE from E. coli, whose crystal structure was previously used for studies on sigma-promoter DNA interactions (Lane and Darst, 2006; Campagne et al., 2014).
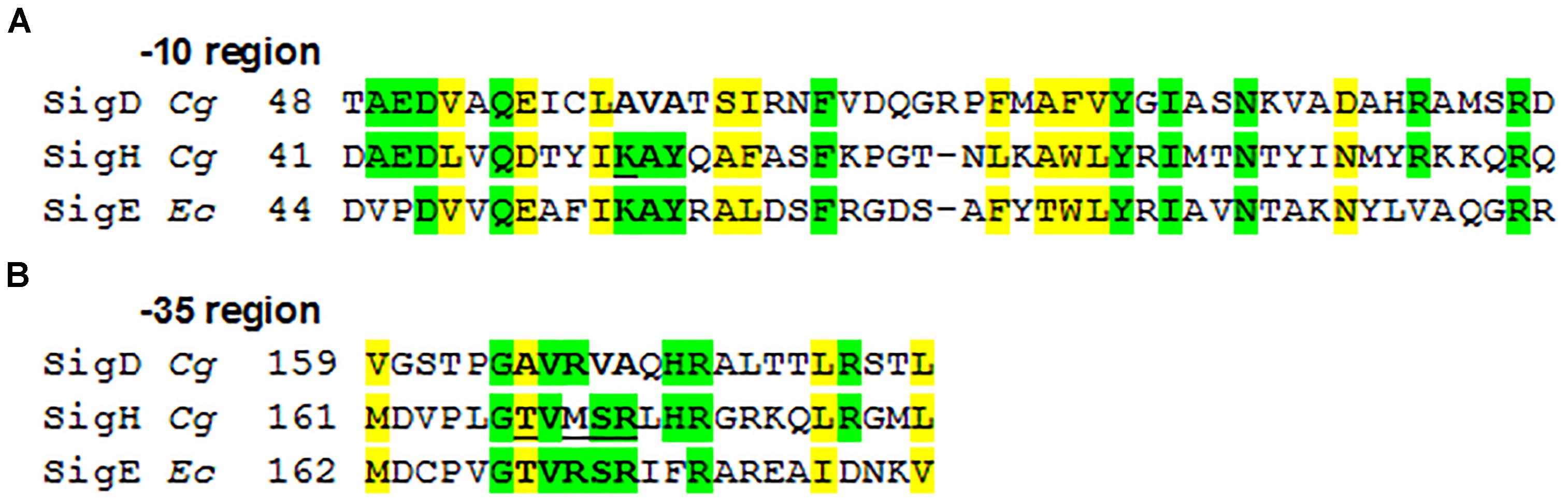
Figure 5. Alignment of the σ AA sequences binding promoter –35 and –10 elements in C. glutamicum. E. coli σE, which was analyzed using its crystal structure (Lane and Darst, 2006; Campagne et al., 2014), is aligned for comparison. The AAs which are supposed to interact with the –10 (A) and –35 (B) elements (Lane and Darst, 2006; Campagne et al., 2014) are in bold. The σH AAs which we replaced with the corresponding AAs from σD by mutagenesis are underlined. Identical AAs are shown in green, similar in yellow; Cg, C. glutamicum; Ec, E. coli.
Computer Modeling of σD and σH in Complexes With -10 and -35 Elements of σD- and σH-Controlled Promoters
We have shown that the consensus sequences of the C. glutamicum -10 and -35 elements recognized by σD (this study) and σH (Busche et al., 2012) differ (i.e., GAT vs. GTT at -10 and GTAACA/G vs. GGAAT/C at -35). To identify the key AAs responsible for recognizing different nucleotides at the second position of these consensus sequences and to understand why σH is, to a certain extent, able to initiate transcription from the σD-dependent promoters, we used computer modeling tools. First, we created homology models of those σD and σH domains, which recognize the -10 and -35 sequences of the respective promoters. The structure of E. coli σE based on the crystal protein in complex with promoter DNA was used as a template (Lane and Darst, 2006; Campagne et al., 2014). It should be noted that the DNA promoter sequences recognized by E. coli σE are closer to the C. glutamicum consensus of the σH-specific -10 promoter element (GTC in E. coli σE, i.e., T at the second position in both cases) as well as the -35 element (GGAAC in E. coli σE, i.e., G in the second position in both cases). This also corresponds to the similarity of the AA sequences of E. coli σE and C. glutamicum σH which are supposed to interact with these nucleotides (Lane and Darst, 2006; Campagne et al., 2014) (shown in bold in Figure 5).
As for the -10 element, there is no X-ray or cryo-electron microscopy structure of the RNAP complex with any of the group IV (ECF) sigma factors described, and a model of the complete DNA transcription bubble is not yet available. The key nucleotide at the second position of the C. glutamicum -10 elements (i.e., A in σD and T in σH) is in close contact with the surface of the σ subunit in our homology models based on the E. coli σE crystal structure 4LUP (Figure 6). According to these models, the presence of Ala 60 in σD and Lys 53 in σH seems to be crucial for the recognition of the nucleotide at the second position of the -10 element. Ala 60 with the minimal side chain creates space for a larger adenine at the second position in the -10 element at σD (Figure 6A). In contrast, Lys 53 with a larger side chain fills the free space made by a smaller base, thymine, at the second position of the -10 consensus element at σH (Figure 6B).
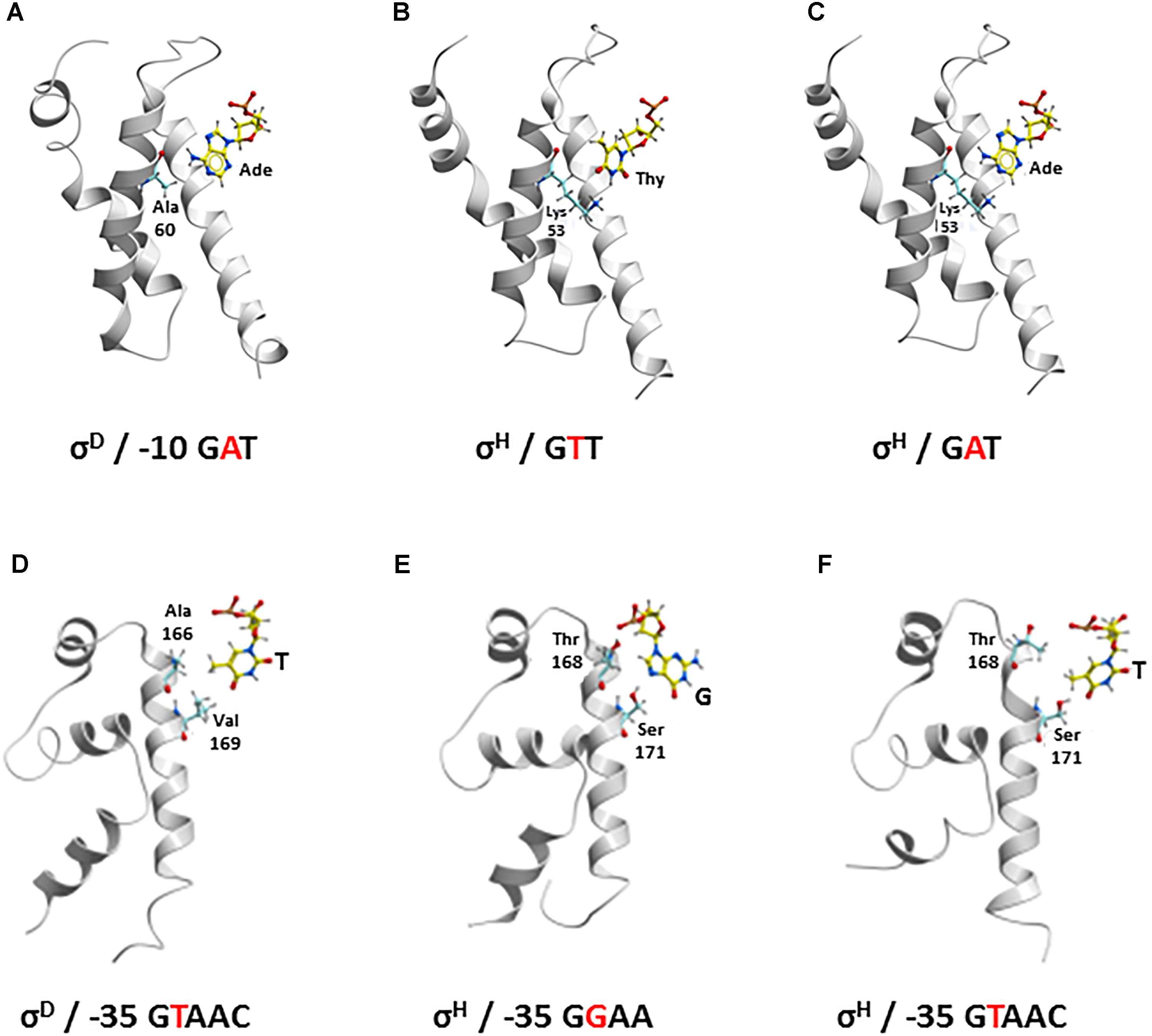
Figure 6. Recognition of the nucleotide at second position of –10 element (yellow represents carbon; red, oxygen; blue, nitrogen; white, hydrogen, Ade, adenine; Thy, thymine) (A–C) by Ala 60 in σD and Lys 53 in σH (cyan, carbon) and at second position of –35 element (yellow, carbon) (D–F) by Ala 166 and Val 169 in σD and Thr 168 and Ser 171 in σH (cyan, carbon). –10: (A) σD and σD-dependent promoter; (B) σH and σH-dependent promoter; (C) σH and σD-dependent promoter –35: (D) σD and σD-dependent promoter; (E) σH and σH-dependent promoter; (F) σH and σD-dependent promoter.
As for the -35 element, template and non-template DNA strands clearly form a duplex. There is no potential conformational polymorphism that could appear with the -10 element, where the transcription bubble opens. Therefore, our homology models of σD and σH in complex with the respective -35 elements can be considered highly plausible. To verify the stability and reliability of these homology models, we introduced a water envelope into the models and carried out 50-ns MD simulations. It was found, that the hydrophobic interactions of the methyl group of T at the second position of the σD-consensus of the -35 element with the side chains of Val 169 and Ala 166 in σD are crucial for its recognition (Figure 6D). Conversely, a hydrogen bond with the side chain of Ser 171 is most important for the recognition of G at the second position of the σH-consensus of the -35 element (Figure 6E). The hydrogen bond between the hydroxyl group of Thr 168 and the phosphate group of the sugar-phosphate DNA backbone provides another stabilizing interaction.
We used the established models to investigate why σH is, to some degree, capable of recognizing σD-controlled promoters.
-10 Element
The larger side chain of Lys 53 in σH must interact with the larger adenine at the second position of the GAT consensus instead of the small side chain of Ala 60 in σD, if we consider the recognition of the -10 GAT motif of a σD-controlled promoter by σH. This is possible since the Lys 53 side chain is highly flexible and therefore probably does not represent a major barrier to the larger adenine (Figure 6C). To test whether Ala 60 in σD and Lys 53 in σH play key roles in recognizing the -10 element in σD-controlled promoters, we designed the mutant σHLys-53-Ala for in vivo analysis.
-35 Element
With the -35 element within the σD-dependent promoter, thymine at the second position of the σD-promoter consensus (GTAAC), whose methyl group interacts with the side chains of the hydrophobic AAs Val 169 and Ala 166 in σD (Figure 6D), interacts with Thr 168 and Ser 171 in σH (Figure 6F). To assess these interactions in complex (σH/-35 element of σD-dependent promoter) in detail, we again performed an extensive 50-ns MD simulation. It was found that the side chain of Thr 168 in σH can rotate, and its methyl group then interacts with the methyl group of the thymine within GTAAC, which undoubtedly leads to a hydrophobic stabilization. Moreover, the hydroxyl group from the Ser 171 side chain forms a hydrogen bond with the oxygen atom of the thymine. To test whether the replacement of the AA sequence 168-ThrValMetSerArg-172 with AlaValArgValAla from σD can improve the recognition of σD-controlled promoters by σH, we designed the mutant σH168-AlaValArgValAla-172 for the in vivo analysis.
Activity of σH-σD Mutants With the σD-Controlled Promoters
Most of the σD-controlled promoters were to a lesser extent also activated by σH, although the highly conserved sequences of the σH-specific promoters (-35 GGAAT/C, -10 GTT) differ from those of the identified σD-specific promoters (-35 GTAAC, -10 GAT). Computer modeling showed that σH can bind to the key regions of promoter DNA in a similar manner as σD to the analogous elements. Based on the results of the sequence similarity analysis and computer modeling, we designed mutations to make σH more σD-like and possibly more efficient at transcription from σD-dependent promoters. We introduced the mutations into the sigH gene cloned in the expression vector pEC-XT99A, which resulted in the production of the mutant proteins σHLys-53-Ala or σH168-AlaValArgValAla-172. These mutant genes were used in the two-plasmid assays with the promoters which exhibited the highest activity with σH: Pcg0607, Plpps, Pcmt2, and PrsdA. We assumed that the overproduced σHLys-53-Ala and σH168-AlaValArgValAla-172 would trigger higher promoter activity in two-plasmid assays with the chosen σD-dependent promoters than σH. This assumption was only confirmed with σHLys-53-Ala and Pcg0607 (Figure 7). This effect was particularly apparent at T24, when promoter activity with σHLys-53-Ala was 1.7-fold higher than that with σH. This higher activity compared to other promoters could be explained by the unparalleled presence of the GGAA sequence in the -35 region of Pcg0607, which is a consensus sequence of -35 elements in σH-dependent promoters. This result indicated that Lys 53 in σH and Ala 53 in σHLys-53-Ala (and Ala 60 in σD) most likely interact with the -10 promoter elements.
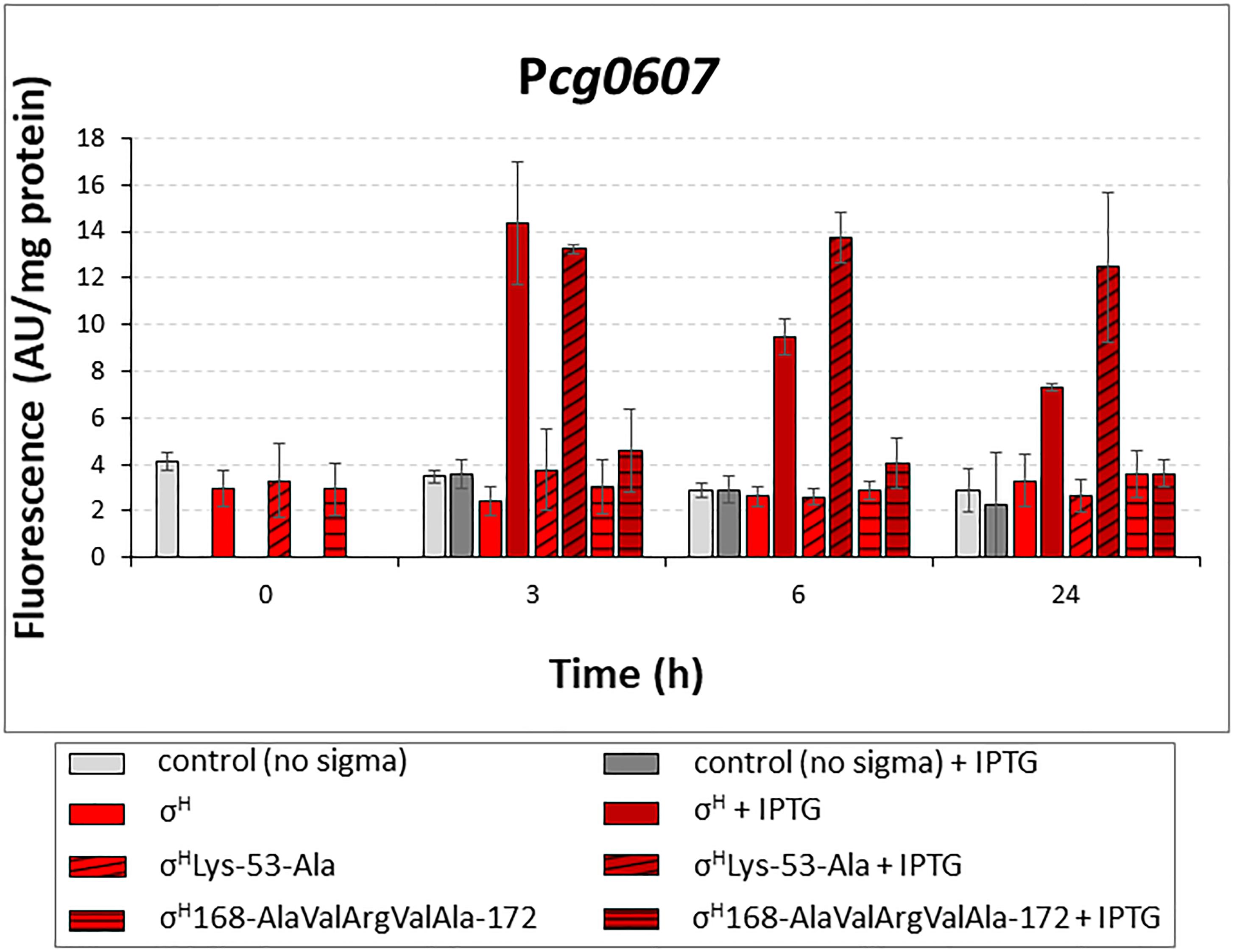
Figure 7. Determination of promoter activity in C. glutamicum WT with overexpressed sigH or its mutants σHLys-53-Ala and σH168-AlaValArgValAla-172 by using two-plasmid assay. The C. glutamicum strains carried the vector pEC-XT99A overexpressing sigH (open bars), σHLys-53-Ala (cross-hatched bars) or σH168-AlaValArgValAla-172 (horizontally hatched bars) after IPTG addition and the promoter-test vector pEPR1 with the target promoter Pcg0607. The strain with the empty expression vector pEC-XT99A was used as a control (gray bars). The promoter activity was measured as the Gfpuv fluorescence intensity of the cell extracts. Fluorescence of cultures without IPTG is shown as light bars; fluorescence of cultures with IPTG induction is shown as dark bars. AU, arbitrary units. The standard deviations of three biological replicates are depicted by error bars.
Contrary to the expectation, σH168-AlaValArgValAla-172 recognized Pcg0607 less efficiently. To assess how the mutant σH168-AlaValArgValAla-172 could interact with the σD-controlled promoters, we analyzed the homology model with this mutant σ factor in more detail. Using a 50-ns MD simulation, we found that the complex of σH168-AlaValArgValAla-172 with the -35 element of the σD-controlled promoter is probably not stable. This was something of a surprise given that the native σH was able to efficiently bind σD-controlled promoter DNA in previous MD simulations. A detailed analysis of the MD trajectories showed that this instability appeared to be due to the absence of Arg at the final position of the AA sequence AlaValArgValAla (ThrValMetSerArg in σH). The positively charged Arg 172 side chain of σH was found to create a significant stabilizing interaction (a salt bridge) with the negatively charged backbone of promoter DNA. This hypothesis, which might explain the low efficiency of the mutant σH168-AlaValArgValAla-172, may be tested in the future by creating additional mutations suggested by the in silico analysis.
Multiple Overlapping Promoters Upstream of the σD-Controlled Genes
In addition to the TSS ascribed to the σD-controlled promoters (Table 2), start sites of transcription driven from different overlapping or nearby promoters were detected by RNA-seq for 8 of the 11 analyzed genes. We suggest that most of these promoters are activated by σA and/or σB, and two are activated by σH, according to the upstream sequences (Figure 8A, in green and red, respectively). The putative housekeeping promoters are always located upstream of the σD-dependent promoters if there is a single additional σA- and/or σB-dependent promoter (upstream of the cg0420, cg0607, cg2047, and cmt2 genes). Promoters which we consider to be σH-dependent according to the sequences of the putative -35 (GGAA) and -10 (GTT) elements were found upstream of lppS and fadD2 genes. The detection of all TSS in the upstream regions of the σD-dependent genes was important to avoid effects of transcription started from the additional promoters. The position of the cloned promoter fragments for in vivo and in vitro assays could thus be chosen in such a way, that only the activity of the supposed σD-controlled promoters was determined. The organization of the multiple promoters within the upstream regions of the 8 genes is shown in Figure 8B. In the case of fadD2, the studied σD-dependent P1fadD2 and the additional σH-dependent P2fadD2 partially overlap and the activity of the additional σH-specific promoter might thus contribute to the overall σH-dependent activity of the fragment. However, such activity was not detected by in vitro transcription. In vivo, the σH-dependent activity was similarly low as in other cases. The σA- and/or σB-dependent promoters P3cmt1, P2cg0420, P2cg0607, P2cg2047, P3lppS, and P2cmt2 (Figures 8A,B) were found to partially (mostly by the -10 region) reside on the 70-nt fragments with the analyzed σD-dependent promoters. However, their possible activity most probably did not interfere with the in vivo measurements of the effects of σD and σH overexpression.
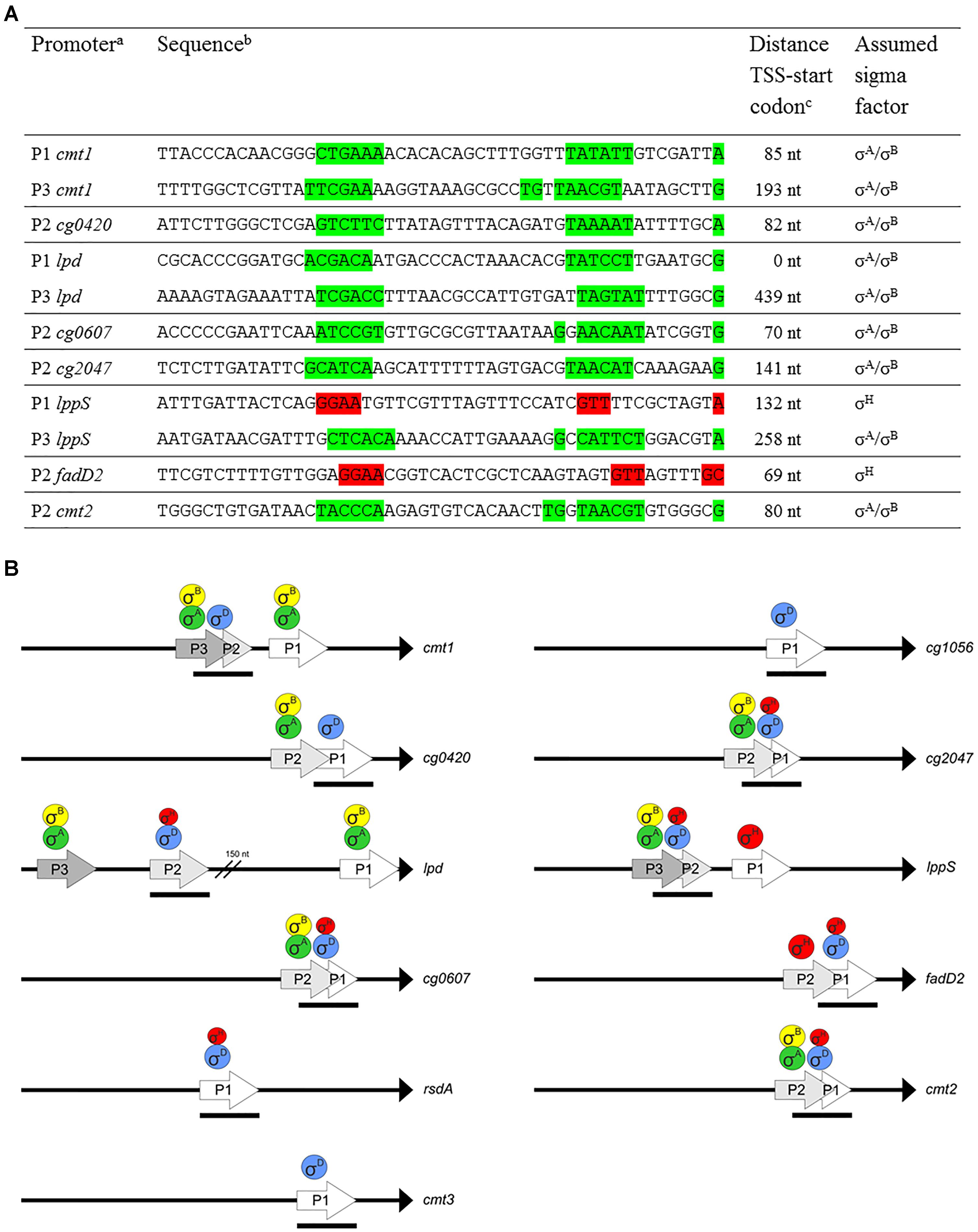
Figure 8. Multiple promoters within upstream region of σD-dependent genes. (A) Sequences of putative σA/σB- or σH-dependent promoters within upstream region of σD-dependent genes. The promoters identified closest to the translation start codon were designated P1. The suggested –35 and –10 (or extended –10) promoter elements and the respective TSS are highlighted in green (σA/σB-dependent) or red (σH-dependent). TSS were detected by RNA-seq specific for transcription start sites. (B) Schematic representation of upstream regions of the genes with multiple promoters. To complete the studied promoter set, the fragments only carrying the σD-dependent promoters PrsdA, Pcmt3, and Pcg1056 are also included. The proposed sigma factors regulating transcription from the individual promoters are shown above. Transcriptional starts are at the tips of the arrows. The length and positions of the bars below the arrows depict approximately the length and positions of the promoter-carrying DNA fragments (70 nt) used for both in vitro and in vivo assays. Translation initiation points are shown as black triangles.
Phenol as a Stressor
We have tested a number of stress conditions (heat shock, SDS, penicillin G, glycine, mitomycin C, phenol and limitation by glucose) to detect a slower growth of the C. glutamicum ΔsigD strain or to induce transcription from PsigD or from the promoters found to be σD-dependent (data not shown). Out of the conditions tested, only phenol exhibited a stronger inhibitory effect on the growth of the ΔsigD strain carrying the vector pEC-XT99A than on the growth of the analogous WT strain. We found that growth rate of C. glutamicum WT culture in 2xYT medium after the addition of 7.5 mM phenol decreased from 0.88 to 0.46 h-1 (1.9-fold), whereas the growth of the C. glutamicum ΔsigD decreased from 0.68 h-1 to 0.22 (3.1-fold). A difference in the effect of phenol was not significant in plasmidless strains.
To analyze the transcriptional response of C. glutamicum to phenol stress, we performed RNA-seq using the RNA isolated from the C. glutamicum WT cells grown in minimal medium with glucose (2%) or phenol (3.4 mM). Differential gene expression analysis including normalization, was performed using the whole transcriptome data and Bioconductor package DESeq2 (Love et al., 2014) included in the software ReadXplorer v2.2 (Hilker et al., 2014). The evaluation of the differential RNA-seq data was performed using an adjusted p-value cut-off of P ≤ 0.01 and a signal intensity ratio (m-value) cut-off of ≥1 or ≤-1. According to the results (Supplementary Tables S3, S4), five genes previously found to be σD-dependent were more highly expressed on phenol than on glucose: cg0607, lppS, rsdA, lpd, and cg2047 (Supplementary Table S4). The respective TSS identified by RNA-seq of 5′-enriched primary transcripts using the cultures grown on phenol were identical with those found using the sigD overexpressing strain.
The effect of phenol on the activity of the promoters Pcg0607, PlppS, PrsdA, Plpd, and Pcg2047 was tested in vivo with the two-plasmid system. The strains carrying sigD or sigH inserted in pEC-XT99A and the promoters in pEPR1 were cultivated in minimal medium with glucose (2%) or phenol (3.4 mM). The promoter activity was measured in the extract of the cells before the addition of IPTG and at the end of exponential growth phase.
The activity of four promoters (Pcg0607, PlppS, PrsdA, and Plpd) in the strains with overexpressed sigD was clearly higher after cultivation with phenol (Figure 9). The activity of the σC-dependent Pcg2556 used as a control was much lower in the medium with phenol under sigC overexpression conditions. The overexpression of sigH in the presence of phenol only resulted in a small increase in the Plpd activity. These results confirmed the data from RNA-seq (with the exception of Pcg2047 which was induced on phenol according to RNA-seq, but not in the two-plasmid assay). This is one of a few examples of the stress response to the action of phenolic compounds regulated by the ECF sigma factors in C. glutamicum (Chen et al., 2016, 2017). The phenol stress response mediated by ECF sigma factors will be further studied in C. glutamicum on the basis of the large amount of data produced by RNA-seq.
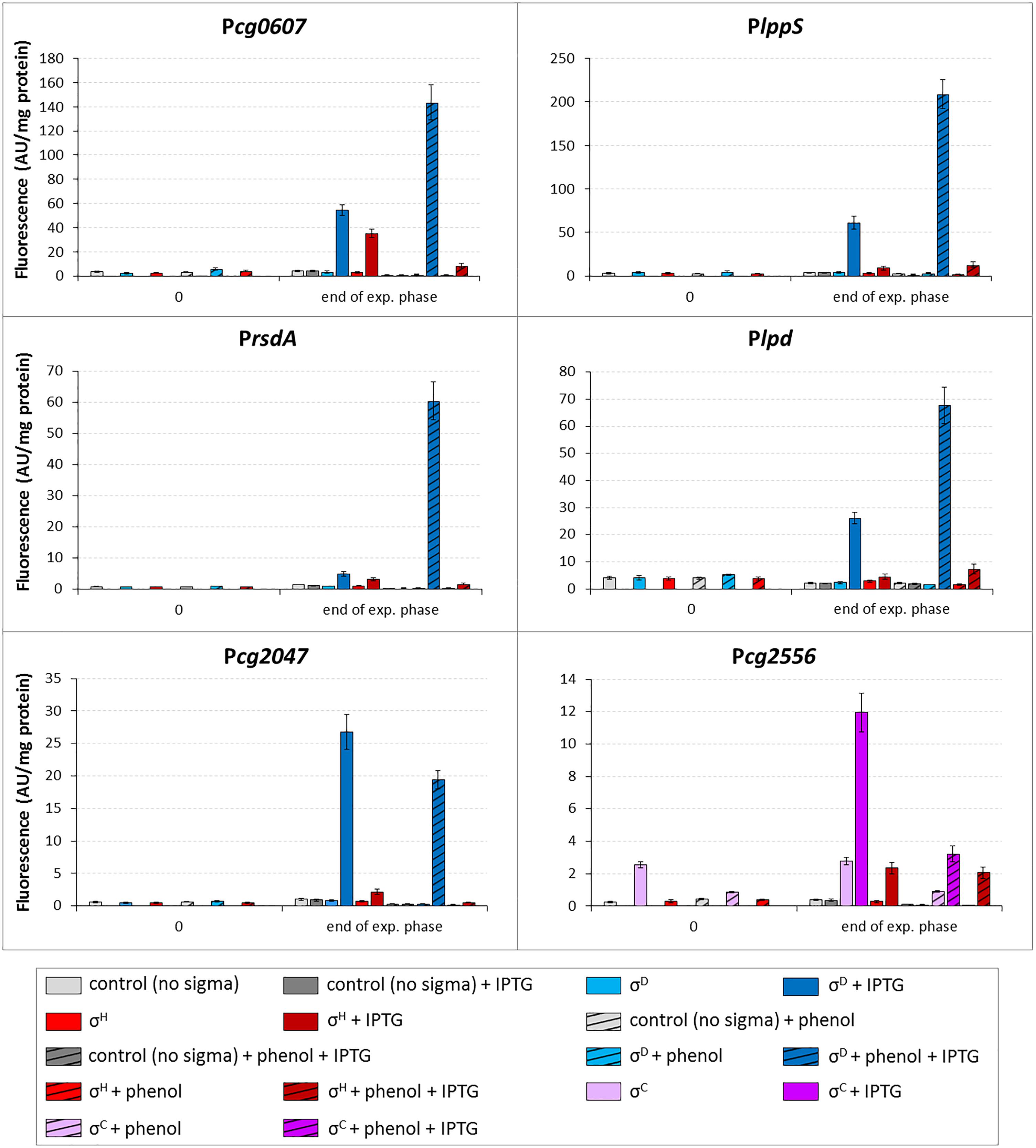
Figure 9. Effect of phenol on activity of σD-dependent promoters. The C. glutamicum strains carried the vector pEC-XT99A overexpressing sigH (red bars), sigD (blue bars) or sigC (purple bars) after the addition of IPTG (added at OD600 = 1; dark bars) and the promoter-test vector pEPR1 constructs carrying the tested promoters (above the graphs). The strain overexpressing sigC and harboring pEPR1 with the σC-dependent promoter Pcg2556 (Dostálová et al., 2017), which is not activated by phenol, was used as a control (purple bars). Phenol (3.4 mM) was present in media from the beginning of cultivation (hatched bars). Plasmids carrying the pEPR1 construct and empty pEC-XT99A were used as controls (gray bars). The samples were taken at OD600 = 1 (0) and at the end of exponential growth phase. AU, arbitrary units. The standard deviations of three biological replicates are depicted by error bars.
Discussion
We recently began to study the function of C. glutamicum σD and its regulon for the first time (Taniguchi et al., 2017). The ECF sigma factor σD was found to be crucial for the envelope stress response, the synthesis of the mycomembrane and formation of the cell wall (Taniguchi et al., 2017; Toyoda and Inui, 2018). The genes of the σD regulon are involved in the synthesis of mycolic acids, the modification of peptidoglycan and other cell envelope-related functions. A subgroup of these genes which control mycolate metabolism are specific to the Mycolata group of bacteria, which include Corynebacterium, Mycobacterium, Rhodococcus, and Nocardia as the most important genera.
Sigma factors exhibiting a high level of similarity to C. glutamicum σD have been found in many bacteria. The highest level of AA identity with C. glutamicum σD was detected in σD proteins deduced from the respective genes in species closely related to C. glutamicum [C. deserti (94%), C. callunae (93%) and C. efficiens (90%)]. A lower level of identity (70–79%) was found with σD proteins from most of the phylogenetically more distant Corynebacterium species. The identity of C. glutamicum σD with σD proteins from other bacteria of the Mycolata group (e.g., genera Mycobacterium, Rhodococcus, and Nocardia) exhibited a range of 54–57%. All bacteria with a σD identity level higher than 45% (e.g., streptomycetes) belong to Actinobacteria. However, the functions of the genes of the σD regulons in these related bacteria are partially different. In addition to mycolate synthesis, the M. tuberculosisσD regulon is involved in virulence, lipid metabolism and gene expression under starvation conditions (Raman et al., 2004; Calamita et al., 2005).
A comparison of the promoter sequences of the putative σD-dependent genes among various bacteria was limited by the fact that either homologous genes were only found in a small number of Corynebacterium species (e.g., the genes cg0420 and cg2047 only occur in the genomes of three C. glutamicum strains) or no consensus sequences suggested for σD-dependent promoters were identified upstream of the homologous genes in the vast majority of other Corynebacterium species. Conservation of the putative σD-dependent promoter sequences among various bacteria was only found in the genes cmt1 and cmt2, encoding trehalose corynomycolyl transferases.
Almost total conservation (37/38) of the -10 and -35 sequences (GTAAC - 15 nt – CTCGAT) in the PrsdA promoter was found in 38 Corynebacterium species encoding the anti-sigma factor RsdA. In other Actinobacteria (genera Rhodococcus, Nocardia, and Mycobacterium), a putative σD-dependent promoter is not present upstream of the rsdA gene but upstream of the sigD gene. Such an arrangement indicates that the sigD-rsdA operon is autoregulated by σD in these bacteria, in contrast to C. glutamicum, where σD seems to only control rsdA gene expression but not its own synthesis. Only two vegetative promoters were localized upstream of the C. glutamicum ATCC 13032 sigD gene (Pfeifer-Sancar et al., 2013).
We showed that σD played a dominant role in the transcription from all promoters tested in this study, however, low σH activity with most of these promoters was detected by the in vivo two-plasmid assay. This activity of the promoters was not observed when using in vitro transcription (with one exception). The promoter Pcg0607 was an exception and its efficiency documented by in vitro transcription was even higher with σH than with σD (Figure 2). The reason for this is probably that the -35 sequence GGAA within Pcg0607 is identical to the consensus of the -35 element of σH-controlled promoters. Similarly, the -10 element GTT, typical for σH-controlled promoters, was found in Plpd. Its activity with σH in vivo was also higher than that of other promoters (Figure 3). We can consider Pcg0607 and Plpd to be natural σD/σH-dependent hybrid promoters. However, it is apparent that the promoter efficiencies determined in vivo and in vitro do not correlate perfectly.
SigH plays a prominent role in the sigma regulatory network. Promoters controlled by σH were found to drive the transcription of sigA (Toyoda and Inui, 2015), sigB (Dostálová et al., 2017) and sigM (Nakunst et al., 2007). SigH, which also controls the expression of several genes encoding transcriptional regulators (clgR, sufR, and hspR), is therefore considered to be a candidate for a global regulatory molecule (Schröder and Tauch, 2010). Overlap of the recognition specificities of σH and σE was described for some promoters (Šilar et al., 2016). In this study, an unexpected overlap of σH and σD in recognizing σD-dependent promoters has been proven.
To prove that the promoters are recognized directly with RNAP+σH, we measured the activities of Pcmt2, PlppS, and PrsdA with σH in the ΔsigD strain. All three promoters were found to be active (Figure 4). The transcription driven by σH was apparently stronger in the absence of σD in the cell, probably because there was no competition with σD. Upstream regions of cmt2 and rsdA carried no potential σH-specific promoter and the possible σH-specific promoter P1lppS was not included within the tested 70-nt P2lppS fragment (distance between TSS1 and TSS2 was 93 nt). Thus, it was apparently σH, which drove the detected activity of the σD-dependent promoter P2lppS in the ΔsigD mutant. Thus, σH was able to partially substitute for missing σD.
We have investigated the σD-dependent genes by different methods in C. glutamicum ATCC 13032 than those used in C. glutamicum R (Toyoda and Inui, 2018). In our study, differential gene expression analysis based on the obtained RNA-seq data of the whole transcriptome libraries (Illumina TruSeq stranded mRNA libraries) and RNA-seq of the 5′-enriched primary transcripts of C. glutamicum ATCC 13032 overexpressing sigD enabled us to not only discover the σD-regulated genes, but also precisely identify the TSS of the genes studied. Moreover, individual σD-dependent promoters were analyzed using in vitro transcription and in vivo two-plasmid assay. The in vitro assay revealed that Pcg0607 can directly drive transcription with RNAP+σH (Figure 2). Two σD-dependent genes of C. glutamicum ATCC 13032, cg0420 and cg2047 (encoding glycosyltransferase and a secreted protein, respectively), are not present on the C. glutamicum R chromosome. Another gene, lpd was not found to be σD-dependent in C. glutamicum R. The promoter P2lpd was localized 360 nt upstream of the translation initiation codon. There is a possibility that a potential small RNA, which was detected by RNA-seq, is transcribed from this promoter. P2lpd has a GTT sequence in the -10 region which is the consensus of σH-dependent promoters. The lpd gene (encoding dihydrolipoamide dehydrogenase) is known to play a role in the oxidative stress response in bacteria (Krisko et al., 2014). In M. tuberculosis lpd contributes to survival against host-generated reactive oxygen species (Daly et al., 2007). The activity of P2lpd was found to increase with sigD and, to a lesser extent, with sigH overexpression in the presence of phenol in C. glutamicum (Figure 9).
Based on the in silico analysis, the sigH mutants resulting in the production of the mutant proteins σHLys-53-Ala or σH168-AlaValArgValAla-172 were constructed. The mutation Lys-53-Ala within the -10 sequence-binding region of σH improved the σH-controlled activity of Pcg0607, which is in agreement with the suggested crucial role of Ala in this position in recognizing the -10 element of σD-controlled promoters. The low promoter activity with σH mutant carrying 168-AlaValArgValAla-172 was probably due to the missing Arg 172, which may establish important stabilizing salt bridges with the sugar-phosphate backbone of DNA according to the in silico homology modeling.
Differential gene expression analysis of the transcriptome of C. glutamicum ATCC 13032 grown with or without phenol revealed that some σD-dependent genes (cg0607, lppS, rsdA, and cg2047) were higher transcribed under the phenol treatment. The promoters Pcg0607, PlppS, Prsd and in addition Plpd, were also found to be induced by phenol in minimal medium by two-plasmid analysis (Figure 9). In C. glutamicum R, differential gene expression analyzed by microarrays showed that the σD-dependent genes play a role in the response to the lysozyme treatment (Toyoda and Inui, 2018). The changes in gene expression seem to be induced by the cell envelope stress exerted by the action of phenol or lysozyme.
Phenol in complete medium did not significantly slow the growth of the ΔsigD strain compared to the WT strain. However, when the replication of a single or even two plasmids placed a burden on the cell growth, these plasmid-harboring ΔsigD strains grew slower or did not grow at all. This extremely poor growth of ΔsigD strain with two plasmids was probably due to deficiencies in the cell envelope synthesis in combination with detrimental effects of supplemented antibiotics (Km and Tc) necessary for plasmid maintenance.
The function of σD-dependent genes and the organization of their transcription suggest that these genes play an important role not only in stress response, but also in cell homeostasis and in building a cell envelope during rapid growth under optimum conditions. Under optimal growth conditions, the transcription of the genes involved in corynomycolate synthesis is probably driven from additional σA- and/or σB-dependent promoters or σH-dependent promoters, which we detected upstream of many σD-dependent genes (Figure 8A). The transcription of σD-dependent genes from multiple promoters controlled by other sigma factors and the transcription from the σD-dependent promoters by σH may partially ensure the expression of these genes if the σD function is damaged or eliminated. However, these promoters are probably not strong enough to enable the cell to cope with the combined stress conditions if σD is missing. The low σH-triggered activity of the largely σD-controlled promoters, which is probably induced by modulation of the σH recognition specificity under particular physiological conditions, may contribute to fine-tuning transcription under various stresses, especially under the cell envelope stress.
Several genes of the M. tuberculosis σD regulon were found to be active in the stationary growth phase (Raman et al., 2004). Gfpuv fluorescence, which should reflect the activity of C. glutamicum σD-dependent promoters in our study, increased during cultivation and was found to be maximal at T24 (Figure 3). Interestingly, activity of the same promoters driven by σH exhibited the opposite trend. It therefore seems that σH may partially substitute for σD activity during the exponential phase.
The possible global regulator σH has been proven to play a crucial role in the hierarchy of σ factors, since it drives the transcription of at least three of them (σA, σB, and σM). Moreover, the regulatory overlaps of σH with transcriptional regulators(Toyoda and Inui, 2015) and with several sigma factors including σD seem to control a number of physiological functions in C. glutamicum including heat, SOS, oxidative, chemical and cell surface stress responses. The accumulated evidence indicates that overlaps between the regulons of C. glutamicum ECF sigma factors are rather a common regulatory strategy how to cope with complex environmental stresses than an unusual exception.
Author Contributions
MP and JK conceived the project and led the studies performed in Prague and Bielefeld, respectively. HD, TB, LR, and VŠ carried out the most experiments. TB and HD carried out RNA-seq and processed the data. JH did the in vitro transcription assays. JN performed the sequence analyses. IB carried out in silico analyses. All authors analyzed the results. MP and JN drafted the initial manuscript. All authors contributed to writing the final manuscript, reading, and approving the submitted version.
Funding
This work was supported by Grant 17-06991S from the Czech Science Foundation, Mobility Grant DAAD-18-11 from Czech Academy of Sciences (CAS) and Deutscher Akademischer Austauschdienst.
Conflict of Interest Statement
The authors declare that the research was conducted in the absence of any commercial or financial relationships that could be construed as a potential conflict of interest.
Acknowledgments
Hironori Taniguchi (Wendisch Lab, Bielefeld University) is acknowledged for providing sigD-overexpressing C. glutamicum cultures for RNA isolation.
Supplementary Material
The Supplementary Material for this article can be found online at: https://www.frontiersin.org/articles/10.3389/fmicb.2018.03287/full#supplementary-material
TABLE S1 | Oligonucleotides used.
TABLE S2 | List of identified TSSs of SigD-upregulated genes and the respective sequences as detected by RNA-seq of transcriptome of C. glutamicum with overexpressed sigD cultivated on glucose.
TABLE S3 | List of identified TSSs of and the respective sequences as detected by RNA-seq of transcriptome of C. glutamicum WT cultivated on glucose.
TABLE S4 | List of identified SigD-dependent TSSs of SigD-upregulated genes and the respective sequences as detected by RNA-seq of transcriptome of C. glutamicum WT cultivated on phenol.
References
Albersmeier, A., Pfeifer-Sancar, K., Rückert, C., and Kalinowski, J. (2017). Genome-wide determination of transcription start sites reveals new insights into promoter structures in the actinomycete Corynebacterium glutamicum. J. Biotechnol. 257, 99–109. doi: 10.1016/j.jbiotec.2017.04.008
Ao, W., Gaudet, J., Kent, W. J., Muttumu, S., and Mango, S. E. (2004). Environmentally induced foregut remodeling by PHA-4/FoxA and DAF-12/NHR. Science 305, 1743–1746. doi: 10.1126/science.1102216
Binder, S. C., Eckweiler, D., Schulz, S., Bielecka, A., Nicolai, T., Franke, R., et al. (2016). Functional modules of sigma factor regulons guarantee adaptability and evolvability. Sci. Rep. 6:22212. doi: 10.1038/srep22212
Busche, T., Šilar, R., Pičmanová, M., Pátek, M., and Kalinowski, J. (2012). Transcriptional regulation of the operon encoding stress-responsive ECF sigma factor SigH and its anti-sigma factor RshA, and control of its regulatory network in Corynebacterium glutamicum. BMC Genomics 13:445. doi: 10.1186/1471-2164-13-445
Calamita, H., Ko, C., Tyagi, S., Yoshimatsu, T., Morrison, N. E., and Bishai, W. R. (2005). The Mycobacterium tuberculosis SigD sigma factor controls the expression of ribosome-associated gene products in stationary phase and is required for full virulence. Cell. Microbiol. 7, 233–244. doi: 10.1111/j.1462-5822.2004.00454.x
Campagne, S., Marsh, M. E., Capitani, G., Vorholt, J. A., and Allain, F. H. (2014). Structural basis for -10 promoter element melting by environmentally induced sigma factors. Nat. Struct. Mol. Biol. 21, 269–276. doi: 10.1038/nsmb.2777
Chaturongakul, S., Raengpradub, S., Palmer, M. E., Bergholz, T. M., Orsi, R. H., Hu, Y., et al. (2011). Transcriptomic and phenotypic analyses identify coregulated, overlapping regulons among PrfA, CtsR, HrcA, and the alternative sigma factors σB, σC, σH, and σL in Listeria monocytogenes. Appl. Environ. Microbiol. 77, 187–200. doi: 10.1128/AEM.00952-10
Chen, C., Pan, J., Yang, X., Guo, C., Ding, W., Si, M., et al. (2016). Global transcriptomic analysis of the response of Corynebacterium glutamicum to vanillin. PLoS One 11:e0164955. doi: 10.1371/journal.pone.0164955
Chen, C., Pan, J., Yang, X., Xiao, H., Zhang, Y., Si, M., et al. (2017). Global transcriptomic analysis of the response of Corynebacterium glutamicum to ferulic acid. Arch. Microbiol. 199, 325–334. doi: 10.1007/s00203-016-1306-5
Chen, C., Zhang, Y., Xu, L., Zhu, K., Feng, Y., Pan, J., et al. (2018). Transcriptional control of the phenol hydroxylase gene phe of Corynebacterium glutamicum by the AraC-type regulator PheR. Microbiol. Res. 209, 14–20. doi: 10.1016/j.micres.2018.02.001
Cho, B. K., Kim, D., Knight, E. M., Zengler, K., and Palsson, B. O. (2014). Genome-scale reconstruction of the sigma factor network in Escherichia coli: topology and functional states. BMC Biol. 12:4. doi: 10.1186/1741-7007-12-4
Crooks, G. E., Hon, G., Chandonia, J. M., and Brenner, S. E. (2004). WebLogo: a sequence logo generator. Genome Res. 14, 1188–1190. doi: 10.1101/gr.849004
Dainese, E., Rodrigue, S., Delogu, G., Provvedi, R., Laflamme, L., Brzezinski, R., et al. (2006). Posttranslational regulation of Mycobacterium tuberculosis extracytoplasmic-function sigma factor sigma L and roles in virulence and in global regulation of gene expression. Infect. Immun. 74, 2457–2461. doi: 10.1128/IAI.74.4.2457-2461.2006
Daly, M. J., Gaidamakova, E. K., Matrosova, V. Y., Vasilenko, A., Zhai, M., Leapman, R. D., et al. (2007). Protein oxidation implicated as the primary determinant of bacterial radioresistance. PLoS Biol. 5:e92. doi: 10.1371/journal.pbio.0050092
Denyer, S. P. (1995). Mechanisms of action of antibacterial biocides. Int. Biodeterior. Biodegr. 36, 221–225. doi: 10.1016/0964-8305(96)00015-7
Dostálová, H., Holátko, J., Busche, T., Rucká, L., Rapoport, A., Halada, P., et al. (2017). Assignment of sigma factors of RNA polymerase to promoters in Corynebacterium glutamicum. AMB Express 7:133. doi: 10.1186/s13568-017-0436-8
Ehira, S., Teramoto, H., Inui, M., and Yukawa, H. (2009). Regulation of Corynebacterium glutamicum heat shock response by the extracytoplasmic-function sigma factor SigH and transcriptional regulators HspR and HrcA. J. Bacteriol. 191, 2964–2972. doi: 10.1128/JB.00112-09
Engels, S., Schweitzer, J. E., Ludwig, C., Bott, M., and Schaffer, S. (2004). Clpc and clpP1P2 gene expression in Corynebacterium glutamicum is controlled by a regulatory network involving the transcriptional regulators ClgR and HspR as well as the ECF sigma factor σH. Mol. Microbiol. 52, 285–302. doi: 10.1111/j.1365-2958.2003.03979.x
Green, M. R., and Sambrook, J. (2012). Molecular Cloning: A Laboratory Manual, Fourth Edn. Cold Spring Harbor, NY: Cold Spring Harbor Laboratory Press.
Gruber, T. M., and Gross, C. A. (2003). Multiple sigma subunits and the partitioning of bacterial transcription space. Annu. Rev. Microbiol. 57, 441–466. doi: 10.1146/annurev.micro.57.030502.090913
Hanahan, D. (1985). “Techniques for transformation of E. coli,” in DNA Cloning. A Practical Approach, Vol. 1, ed. D. M. Glover (Oxford: IRL),109–135.
Hilker, R., Stadermann, K. B., Doppmeier, D., Kalinowski, J., Stoye, J., Straube, J., et al. (2014). ReadXplorer–visualization and analysis of mapped sequences. Bioinformatics 30, 2247–2254. doi: 10.1093/bioinformatics/btu205
Hilker, R., Stadermann, K. B., Schwengers, O., Anisiforov, E., Jaenicke, S., Weisshaar, B., et al. (2016). ReadXplorer 2-detailed read mapping analysis and visualization from one single source. Bioinformatics 32, 3702–3708. doi: 10.1093/bioinformatics/btw541
Holátko, J., Šilar, R., Rabatinová, A., Šanderová, H., Halada, P., Nešvera, J., et al. (2012). Construction of in vitro transcription system for Corynebacterium glutamicum and its use in the recognition of promoters of different classes. Appl. Microbiol. Biotechnol. 96, 521–529. doi: 10.1007/s00253-012-4336-1
Jordan, S., Hutchings, M. I., and Mascher, T. (2008). Cell envelope stress response in Gram-positive bacteria. FEMS Microbiol. Rev. 32, 107–146. doi: 10.1111/j.1574-6976.2007.00091.x
Kalinowski, J., Bathe, J., Bartels, D., Bischoff, N., Bott, M., Burkovski, A., et al. (2003). The complete Corynebacterium glutamicum ATCC 13032 genome sequence and its impact on the production of L-aspartate-derived amino acids and vitamins. J. Biotechnol. 104, 5–25. doi: 10.1016/S0168-1656(03)00154-8
Keilhauer, C., Eggeling, L., and Sahm, H. (1993). Isoleucine synthesis in Corynebacterium glutamicum: molecular analysis of the ilvB-ilvN-ilvC operon. J. Bacteriol. 175, 5595–5603. doi: 10.1128/jb.175.17.5595-5603.1993
Kirchner, O., and Tauch, A. (2003). Tools for genetic engineering in the amino acid-producing bacterium Corynebacterium glutamicum. J. Biotechnol. 104, 287–299. doi: 10.1016/S0168-1656(03)00148-2
Knoppová, M., Phensaijai, M., Veselý, M., Zemanová, M., Nešvera, J., and Pátek, M. (2007). Plasmid vectors for testing in vivo promoter activities in Corynebacterium glutamicum and Rhodococcus erythropolis. Curr. Microbiol. 55, 234–239. doi: 10.1007/s00284-007-0106-1
Kranz, A., Busche, T., Vogel, A., Usadel, B., Kalinowski, J., Bott, M., et al. (2018). RNAseq analysis of alpha-proteobacterium Gluconobacter oxydans 621H. BMC Genomics 19:24. doi: 10.1186/s12864-017-4415-x
Krisko, A., Copic, T., Gabaldon, T., Lehner, B., and Supek, F. (2014). Inferring gene function from evolutionary change in signatures of translation efficiency. Genome Biol. 15:R44. doi: 10.1186/gb-2014-15-3-r44
Lane, W. J., and Darst, S. A. (2006). The structural basis for promoter -35 element recognition by the group IV sigma factors. PLoS Biol. 4:e269. doi: 10.1371/journal.pbio.0040269
Langmead, B., and Salzberg, S. L. (2012). Fast gapped-read alignment with Bowtie 2. Nat. Methods 9, 357–359. doi: 10.1038/nmeth.1923
Love, M. I., Huber, W., and Anders, S. (2014). Moderated estimation of fold change and dispersion for RNA-seq data with DESeq2. Genome Biol. 15:550. doi: 10.1186/s13059-014-0550-8
Luo, Y., and Helmann, J. D. (2009). Extracytoplasmic function sigma factors with overlapping promoter specificity regulate sublancin production in Bacillus subtilis. J. Bacteriol. 191, 4951–4958. doi: 10.1128/JB.00549-09
Nakunst, D., Larisch, C., Hüser, A. T., Tauch, A., Pühler, A., and Kalinowski, J. (2007). The extracytoplasmic function-type sigma factor SigM of Corynebacterium glutamicum ATCC 13032 is involved in transcription of disulfide stress-related genes. J. Bacteriol. 189, 4696–4707. doi: 10.1128/JB.00382-07
Pátek, M., and Nešvera, J. (2011). Sigma factors and promoters in Corynebacterium glutamicum. J. Biotechnol. 154, 101–113. doi: 10.1016/j.jbiotec.2011.01.017
Pfeifer-Sancar, K., Mentz, A., Rückert, C., and Kalinowski, J. (2013). Comprehensive analysis of the Corynebacterium glutamicum transcriptome using an improved RNAseq technique. BMC Genomics 14:888. doi: 10.1186/1471-2164-14-888
Raman, S., Hazra, R., Dascher, C. C., and Husson, R. N. (2004). Transcription regulation by the Mycobacterium tuberculosis alternative sigma factor SigD and its role in virulence. J. Bacteriol. 186, 6605–6616. doi: 10.1128/JB.186.19.6605-6616.2004
Rezuchova, B., and Kormanec, J. (2001). A two-plasmid system for identification of promoters recognized by RNA polymerase containing extracytoplasmic stress response σE in Escherichia coli. J. Microbiol. Methods 45, 103–111. doi: 10.1016/S0167-7012(01)00237-8
Ross, W., Thompson, J. F., Newlands, J. T., and Gourse, R. L. (1990). E. coli Fis protein activates ribosomal RNA transcription in vitro and in vivo. EMBO J. 9, 3733–3742. doi: 10.1002/j.1460-2075.1990.tb07586.x
Salomon-Ferrer, R., Case, D. A., and Walker, R. C. (2013). An overview of the amber biomolecular simulation package. WIREs Comput. Mol. Sci. 3, 198–210. doi: 10.1002/wcms.1121
Schröder, J., and Tauch, A. (2010). Transcriptional regulation of gene expression in Corynebacterium glutamicum: the role of global, master and local regulators in the modular and hierarchical gene regulatory network. FEMS Microbiol. Rev. 34, 685–737. doi: 10.1111/j.1574-6976.2010.00228.x
Schulz, S., Eckweiler, D., Bielecka, A., Nicolai, T., Franke, R., Dotsch, A., et al. (2015). Elucidation of sigma factor-associated networks in Pseudomonas aeruginosa reveals a modular architecture with limited and function-specific crosstalk. PLoS Pathog. 11:e1004744. doi: 10.1371/journal.ppat.1004744
Seo, J. H., Hong, J. S., Kim, D., Cho, B. K., Huang, T. W., Tsai, S. F., et al. (2012). Multiple-omic data analysis of Klebsiella pneumoniae MGH 78578 reveals its transcriptional architecture and regulatory features. BMC Genomics 13:679. doi: 10.1186/1471-2164-13-679
Šilar, R., Holátko, J., Rucká, L., Rapoport, A., Dostálová, H., Kadeøabková, P., et al. (2016). Use of in vitro transcription system for analysis of Corynebacterium glutamicum promoters recognized by two sigma factors. Curr. Microbiol. 73, 401–408. doi: 10.1007/s00284-016-1077-x
Taniguchi, H., Busche, T., Patschkowski, T., Niehaus, K., Pátek, M., Kalinowski, J., et al. (2017). Physiological roles of sigma factor SigD in Corynebacterium glutamicum. BMC Microbiol. 17:158. doi: 10.1186/s12866-017-1067-6
Toyoda, K., and Inui, M. (2015). Regulons of global transcription factors in Corynebacterium glutamicum. Appl. Microbiol. Biotechnol. 100, 45–60. doi: 10.1007/s00253-015-7074-3
Toyoda, K., and Inui, M. (2016). The extracytoplasmic function sigma factor σC regulates expression of a branched quinol oxidation pathway in Corynebacterium glutamicum. Mol. Microbiol. 100, 486–509. doi: 10.1111/mmi.13330
Toyoda, K., and Inui, M. (2018). Extracytoplasmic function sigma factor σD confers resistance to environmental stress by enhancing mycolate synthesis and modifying peptidoglycan structures in Corynebacterium glutamicum. Mol. Microbiol. 107, 312–329. doi: 10.1111/mmi.13883
van der Rest, M. E., Lange, C., and Molenaar, D. (1999). A heat shock following electroporation induces highly efficient transformation of Corynebacterium glutamicum with xenogeneic plasmid DNA. Appl. Microbiol. Biotechnol. 52, 541–545. doi: 10.1007/s002530051557
Waterhouse, A., Bertoni, M., Bienert, S., Studer, G., Tauriello, G., Gumienny, R., et al. (2018). SWISS-MODEL: homology modelling of protein structures and complexes. Nucleic Acids Res. 46, W296–W303. doi: 10.1093/nar/gky427
Wittchen, M., Busche, T., Gaspar, A. H., Lee, J. H., Ton-That, H., and Kalinowski, J. (2018). Transcriptome sequencing of the human pathogen Corynebacterium diphtheriae NCTC 13129 provides detailed insights into its transcriptional landscape and into DtxR mediated transcriptional regulation. BMC Genomics 19:82. doi: 10.1186/s12864-018-4481-8
Keywords: Corynebacterium glutamicum, stress response, RNA-seq, in vitro transcription, sigma factor, promoter
Citation: Dostálová H, Busche T, Holátko J, Rucká L, Štěpánek V, Barvík I, Nešvera J, Kalinowski J and Pátek M (2019) Overlap of Promoter Recognition Specificity of Stress Response Sigma Factors SigD and SigH in Corynebacterium glutamicum ATCC 13032. Front. Microbiol. 9:3287. doi: 10.3389/fmicb.2018.03287
Received: 13 September 2018; Accepted: 17 December 2018;
Published: 09 January 2019.
Edited by:
Daniela De Biase, Sapienza University of Rome, ItalyReviewed by:
Andreas Burkovski, Friedrich-Alexander-Universität Erlangen-Nürnberg, GermanySaori Kosono, The University of Tokyo, Japan
Copyright © 2019 Dostálová, Busche, Holátko, Rucká, Štěpánek, Barvík, Nešvera, Kalinowski and Pátek. This is an open-access article distributed under the terms of the Creative Commons Attribution License (CC BY). The use, distribution or reproduction in other forums is permitted, provided the original author(s) and the copyright owner(s) are credited and that the original publication in this journal is cited, in accordance with accepted academic practice. No use, distribution or reproduction is permitted which does not comply with these terms.
*Correspondence: Miroslav Pátek, cGF0ZWtAYmlvbWVkLmNhcy5jeg==
†Present address: Tobias Busche, Institute of Biology and Microbiology, Freie Universität Berlin, Berlin, Germany