- 1Departamento de Genética e Evolução, Universidade Federal de São Carlos, São Carlos, Brazil
- 2Laboratório Central de Saúde Pública do Tocantins, Palmas, Brazil
- 3School of Dentistry, University of Ribeirão Preto, Ribeirão Preto, Brazil
- 4Department of Cell Cycle and Cancer Biology, Oklahoma Medical Research Foundation, Oklahoma City, OK, United States
Klebsiella pneumoniae is an important opportunistic pathogen that commonly causes nosocomial infections and contributes to substantial morbidity and mortality. We sought to investigate the antibiotic resistance profile, pathogenic potential and the clonal relationships between K. pneumoniae (n = 25) isolated from patients and sources at a tertiary care hospital’s intensive care units (ICUs) in the northern region of Brazil. Most of K. pneumoniae isolates (n = 21, 84%) were classified as multidrug resistant (MDR) with high-level resistance to β-lactams, aminoglycosides, quinolones, tigecycline, and colistin. All the 25 isolates presented extended-spectrum beta-lactamase-producing (ESBL), including carbapenemase producers, and carried the blaKPC (100%), blaTEM (100%), blaSHV variants (n = 24, 96%), blaOXA-1 group (n = 21, 84%) and blaCTX-M-1 group (n = 18, 72%) genes. The K2 serotype was found in 4% (n = 1) of the isolates, and the K1 was not detected. The virulence-associated genes found among the 25 isolates were mrkD (n = 24, 96%), fimH-1 (n = 22, 88%), entB (100%), iutA (n = 10, 40%), ybtS (n = 15, 60%). The genes related with efflux pumps and outer membrane porins found were AcrAB (100%), tolC (n = 24, 96%), mdtK (n = 22, 88%), OmpK35 (n = 15, 60%), and OmpK36 (n = 7, 28%). ERIC-PCR was employed to determine the clonal relationship between the different isolated strains. The obtained ERIC-PCR patterns revealed that the similarity between isolates was above 70%. To determine the sequence types (STs) a multilocus sequence typing (MLST) assay was used. The results indicated the presence of high-risk international clones among the isolates. In our study, the wide variety of MDR K. pneumoniae harboring β-lactams and virulence genes strongly suggest a necessity for the implementation of effective strategies to prevent and control the spread of antibiotic resistant infections.
Introduction
Klebsiella pneumoniae is a Gram-negative opportunistic bacterium that causes infections in hospitalized or otherwise immunocompromised individuals (Gorrie et al., 2017). Currently, K. pneumoniae is showing a high resistance to a broad spectrum of drugs including beta-lactam antibiotics, fluoroquinolones, and aminoglycosides (Fair and Tor, 2014; Dsouza et al., 2017). This resistance is resulting in a growing worldwide problem regarding the choice of effective antibiotic treatment for hospital-acquired infections (Davies and Davies, 2010).
Antibiotics of the β-lactam group are commonly prescribed worldwide and include penicillins, cephalosporins, monobactams, and carbapenems (Samaha-Kfoury and Araj, 2003; Ur Rahman et al., 2018). The production of β-lactamase enzymes by the presence of β-lactam-insensitive cell wall transpeptidases, or the active expulsion of β-lactam molecules from Gram-negative bacteria represent the main indications of β-lactam antibiotic resistance (Wilke et al., 2005). Carbapenems are the β-lactams of choice for the treatment of infections caused by extended-spectrum beta-lactamase (ESBL)-producing bacteria (Karuniawati et al., 2013; Okoche et al., 2015), such as K. pneumoniae. These antibiotics are also considered the last resort for the management of life-threatening health-care-associated infections (Amjad et al., 2011). Unfortunately, bacterial resistance to carbapenems has been increased and is well documented (Paterson and Bonomo, 2005; World Health Organization [WHO], 2014), and has also been further complicated by the production of β-lactamases, efflux pumps, and mutations that alter the expression and/or function of porins and penicillin-binding proteins (PBPs) (Papp-Wallace et al., 2011).
Antimicrobial resistance is commonly related to the spread of transmissible plasmids and the acquisition of resistance genes that normally occur by horizontal gene transfer, which may also carry virulence determinants (Derakhshan et al., 2016). For pathogen survival, the acquisition of resistance and virulent traits is necessary (Da Silva and Mendonça, 2012), and some reports suggest that such may have an essential role in the pathogenesis of K. pneumoniae infections (Vila et al., 2011). Capsule, lipopolysaccharide (LPS), fimbriae (types 1 and 3), and siderophores are virulence factors that contribute to the pathogenicity of K. pneumoniae. K. pneumoniae strains can synthesize capsules of any of the serotypes K1 to K78; however, K1 and K2 can also be associated with increased pathogenicity (Paczosa and Mecsas, 2016).
Here, we show the antibiotic resistance profile, pathogenic potential, and clonal relationships among K. pneumoniae isolated from patients and sources at a tertiary care hospital’s intensive care units (ICUs) in the northern region of Brazil.
Materials and Methods
Bacterial Strains
Twenty-five K. pneumoniae clinical isolates were collected from patients and devices at a tertiary care hospital’s ICUs in the state of Tocantins, located in the northern region of Brazil, between January 2014 and May 2015. All K. pneumoniae were collected at the bed-side, and then transported to the microbiology laboratory immediately for inoculation on proper culture media and preliminary analysis. Thereafter, the bacterial cultures were sent to the Central Laboratory of Public Health of Tocantins (LACEN/TO), a reference unit from the Brazilian Ministry of Health that receives samples for surveillance of antimicrobial resistance and which is usually located in the capital city of each federal state of Brazil. Strains were isolated from the following sources: tracheal aspirate, rectal swab, surgical drain, wound, catheter tip, cerebrospinal fluid, abscess, urine, and sputum.
Ethics Statement
In this work, all K. pneumoniae and the anonymous archival data related patient age, gender, and sample type were obtained from LACEN/TO (data’s owner). The study was approved by the Committee of Ethics in Human Research of the Federal University of São Carlos (no. 1.088.936). Permission to conduct the present study was obtained from the Health Department of the State of Tocantins (Secretaria da Sauìde do Estado do Tocantins – SESAU) and LACEN/TO. Patient consent was not required, since the data presented in this study do not relate to any specific person or persons.
Phenotypic Detection of Antibiotic Resistance and Carbapenemase Productions
The identification of K. pneumoniae and the evaluation of their susceptibility profiles were performed using the VITEK 2 system (bioMérieux, Inc., Hazelwood, MO, United States) following the Clinical and Laboratory Standards Institute guidelines (Clinical and Laboratory Standards Institute [CLSI], 2017). All K. pneumoniae was tested for their resistance against the following 15 antibiotics: ampicillin/sulbactam (SAM), piperacillin/tazobactam (TZP), cefuroxime (CXM), cefoxitin (FOX), ceftazidime (CAZ), ceftriaxone (CRO), cefepime (FEP), ertapenem (ERP), imipenem (IMP), meropenem (MEM), amikacin (AMK), gentamicin (GEN), ciprofloxacin (CIP), tigecycline (TGC), and colistin (CST). Susceptibility to TGC was interpreted using breakpoints proposed by the European Committee on Antimicrobial Susceptibilities Testing (EUCAST)1.
Determination of the production of carbapenemase was carried out by modified Hodge test, synergy test, and the ethylenediaminetetraacetic acid (EDTA) test under the CLSI guidelines (Clinical and Laboratory Standards Institute [CLSI], 2017) and as described elsewhere (Miriagou et al., 2010; Nordmann et al., 2011; Okoche et al., 2015).
Multidrug-resistant (MDR) K. pneumoniae isolates were defined by non-susceptibility to at least one agent in three or more antibiotic categories (Magiorakos et al., 2012).
Genomic DNA Extraction
Genomic DNA was extracted from an overnight culture using the Wizard® Genomic DNA Purification Kit (Promega, Madison, WI, United States). The concentration of the DNA extract and purity was determined by measuring absorbance at wavelengths of 260 nm and 280 nm (NanoVue Plus; GE Healthcare Life Sciences, Marlborough, MA, United States). The integrity of genomic DNA was tested by way of electrophoresis.
Detection of Multidrug Resistance Genes
The detection of resistance genes was performed by polymerase chain reaction (PCR) and their identities confirmed by sequencing. Isolates were screened by PCR amplification using specific primers for the detection of ESBL-encoding genes (blaTEM; blaSHV; blaCTX-M; and blaOXA1,4,and30), carbapenemases genes (blaKPC, blaVIM, blaIMP, blaNDM, and blaOXA48), a tetracycline resistance gene (tetB), and a CST resistance gene (mcr-1). Moreover, efflux pump (AcrAB, mdtK, and ToIC), and porin-coding (OmpK35 and OmpK36) genes were also investigated. The specific primers (Exxtend, São Paulo, Brazil) and the length of expected PCR products are presented in Table 1. Amplicons were analyzed by gel electrophoresis in 1.5% agarose and visualized under ultraviolet (UV) light. The forward primers were used for DNA sequencing.
Serotypes and Virulence-Associated Genes Detection
Polymerase chain reaction was used to detect the presence of capsule serotypes (K1 and K2), and virulence-associated genes. These virulence-associated genes included those encoding for regulators of mucoid phenotype A (rmpA), type 1 and type 3 adhesins (fimH-1 and mrkD), enterobactin (entB), yersiniabactin (YbtS), and aerobactin siderophore system (iutA). Isolated DNA samples were screened using specific primers (Exxtend, São Paulo, Brazil) for the detection of virulence genes (Table 2). The forward primers were used for DNA sequencing.
Sequence Analysis of Resistance and Virulence Genes
The PCR products were extracted from agarose gels, using the Illustra GFX PCR DNA and Gel Band Purification Kit (GE Healthcare, Chicago, IL, United States), and some of them were randomly selected for DNA Sanger sequencing (Macrogen Inc., Korea). The nucleotide sequences of the corresponding genes of the isolates were submitted to the GenBank database with accession numbers MK106173 to MK106187. The sequences were edited with Ugene v1.18.0 (Okonechnikov et al., 2012). Each sequence was compared using BlastN tools2 with the K. pneumoniae genome as the reference. Access to genetic heritage was approved by the National System for the Management of Genetic Heritage (SisGen) (no. AFF27ED).
Enterobacterial Repetitive Intergenic Consensus Polymerase Chain Reaction
Enterobacterial repetitive intergenic consensus PCR (ERIC-PCR) analysis was performed to evaluate the genetic similarity among the bacterial isolates used in this study. ERIC-PCR reactions were executed as previously described by Versalovic et al. (1994), using the primers ERIC1R (5′-ATGTAAGCTCCTGGGGATTCAC-3′) and ERIC2 (5′-AAGTAAGTGACTGGGGTGAGCG-3′). All amplifications were carried out in a total volume of 50 μL, using the enzyme TaKaRa Ex Taq® DNA Polymerase (Takara Bio, Kusatsu, Japan), while standardizing the amount of 100 ng of DNA template for each isolate. The amplified products were separated by 1.5% agarose gel electrophoresis and stained with ethidium bromide using UV radiation for visualization of the bands. The band profile analysis was performed using the BioNumerics program version 5.1 (Applied Maths, Keistraat, Belgium) for construction of the similarity dendrogram by the unweighted pair group mean method, Dice’s similarity coefficient, and 1% band position tolerance. Only bands representing amplicons between 300 bp and 3,000 bp were considered for this analysis. The ERIC-PCR assays were performed in triplicate.
MLST
Ten isolates belonging to the main clusters of the dendrogram obtained by ERIC-PCR were selected for multilocus sequence typing (MLST). Information on the methodology used, including the primers and PCR reaction conditions, is available in the MLST database for K. pneumoniae3. The alleles and sequence types (STs) of each isolate studied by MLST were determined using the MLST database platform for K. pneumoniae.
The determination of the clonal and epidemiological relationships and the formation of clonal complexes (CCs), were completed by analyzing a genetic similarity diagram constructed with the aid of the eBURSTv3 program (eBURSTv3 has been developed and is hosted at The Department of Infectious Disease Epidemiology Imperial College London) (Feil et al., 2004).
Statistical Analysis
The statistical analysis was performed using Fisher’s exact test (p ≤ 0.05).
Results
Antibiotic Resistance Patterns
In the present study, a total of 25 K. pneumoniae strains were isolated from samples collected from ICUs patients and devices of a tertiary hospital located in the northern region of Brazil. Most K. pneumoniae isolates were obtained from a rectal swab (56%; n = 14), followed by tracheal aspirate (16%, n = 4), urine (4%, n = 1), cerebrospinal fluid (4%, n = 1), wound (4%, n = 1), sputum (4%, n = 1), abscess (4%, n = 1), surgical drain (4%, n = 1), and catheter tip (4%, n = 1). A statistical difference was found only between the rectal swab and tracheal aspirate for isolates with resistance to the antibiotic TGC (Supplementary Table S1). Patients ages ranged from 1 day to 75 years (median age: 39 years old), and no significant differences were found regarding age group or gender and anti-microbial resistance. K. pneumoniae strains tested were resistant to all β-lactams (SAM, TZP, CXM-S, CXM, FOX, CAZ, CRO, FEP, ETP, IPM, MEM). These isolates also showed different degrees of resistance to other antibiotics like GEN (80%, n = 20), CIP (64%, n = 16), TGC (52%, n = 13) CST (36%, n = 9), and AMK (4%, n = 1). Demographic characteristics of the patients and antibiotic resistance profiles of the K. pneumoniae isolates to the 16 antibiotics tested are shown in Table 3.
Detection of Genes Coding for Outer Membrane Porins and Multidrug-Resistant Efflux Pumps and Antimicrobial Susceptibility
The majority of isolates (84%, 21/25) were classified as MDR with high-level resistance to at least one agent in three or more antibiotic categories. Among the MDR K. pneumoniae, all (100%, 21/21) isolates contained both ArcAB and TolC efflux pumps genes; 86% (18/21) had AcrAB, mdtK, and ToIC genes, simultaneously; and only 14% (3/21) of isolates did not present with the mdtK multidrug efflux gene. PCR results showed that 33% (7/21) of isolates lacked both OmpK35 and OmpK36 porin genes, while 38% (8/21) of isolates lacked the OmpK36 gene.
Of the four isolates (Kp2, Kp67, Kp74, and Kp75) that did not show MDR profiles, three (Kp2, Kp74, and Kp75) had the AcrAB, mdtK and ToIC genes but not the OmpK35 and OmpK36 porin genes and one isolate (Kp67) carried both the AcrAB, and mdtK efflux pumps genes and the OmpK35 and OmpK36 porin genes. The antibiotic resistance profiles of the K. pneumoniae isolates are presented in Table 4. PCR amplification results for these genes are shown in Supplementary Figure S1.
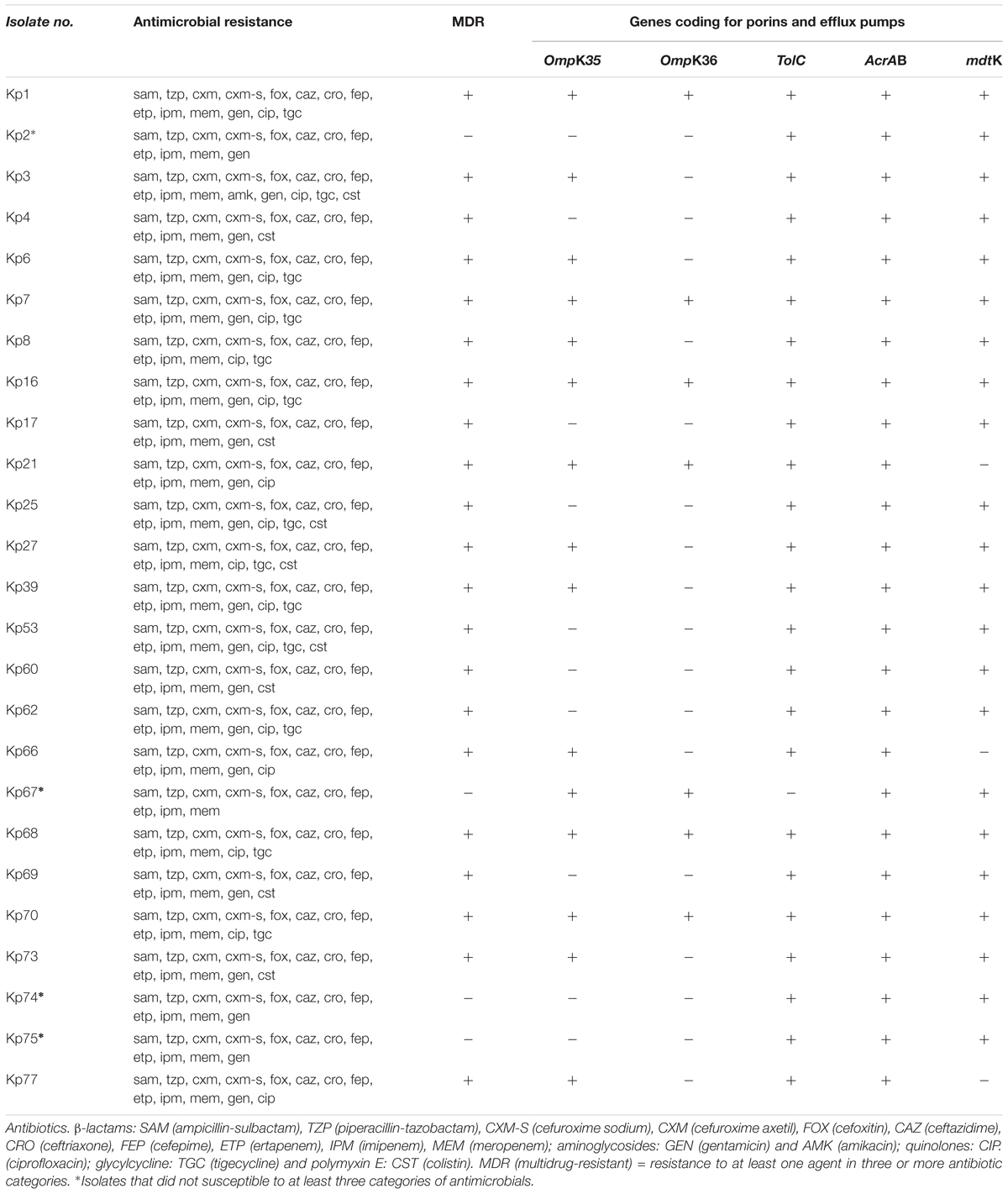
Table 4. Antimicrobial resistance of Klebsiella pneumoniae isolates and presence of genes coding for outer membrane porins and efflux pumps.
Antibiotic Resistance and Virulence-Associated Genes Detection
The distributions of the antibiotic resistance gene and virulence factors are shown in Table 5. All the 25 isolates were positive for the blaKPC gene. In addition, the K. pneumoniae isolates carried the blaTEM (100%, n = 25), blaSHV group (96%, n = 24), blaOXA-1 group (84%, n = 21), and blaCTX-M-1 group (72%, n = 18) ESBL-encoding genes. The blaIMP, blaOXA-48, blaNDM, blaVIM, mcr-1 and tet(B) genes were not detected. It was found that a high number of blaSHV in this study that may be associated with the presence of blaSHV -1, which it is reported to be universal in K. pneumoniae infection (Babini and Livermore, 2000). Additional PCR amplification results are shown in Supplementary Figures S2, S3.
Polymerase chain reaction analysis demonstrated that the fimH-1 and mrkD genes, encoding type 1 and type 3 fimbrial adhesins, were present in 88% (22/25) and 96% (24/25) of isolates, respectively. Additionally, the enterobactin (entB) gene was found in 100% (25/25), the yersiniabactin (ybtS) gene in 60% (15/25) and the aerobactin siderophore system (iutA) gene in 40% (10/25) of isolates. The regulators of the mucoid phenotype A (rmpA) gene were not detected. Only one isolate (4%), recovered from swab rectal, presented the capsular serotype K2, and the capsular K1 was not found (Table 5 and Supplementary Figure S1).
Enterobacterial Repetitive Intergenic Consensus Polymerase Chain Reaction
Genetic similarity among isolates was evaluated via ERIC-PCR, and the results indicated the vast majority of the isolates presented a rate of genetic similarity above 70%, separated into two main clusters (A and B) (Figure 1). Three isolates (Kp53, Kp60, and Kp62) showed 100% genetic similarity. Only four isolates (Kp4, Kp7, Kp17, and Kp67) were genetically more distant and did not cluster with the other isolates.
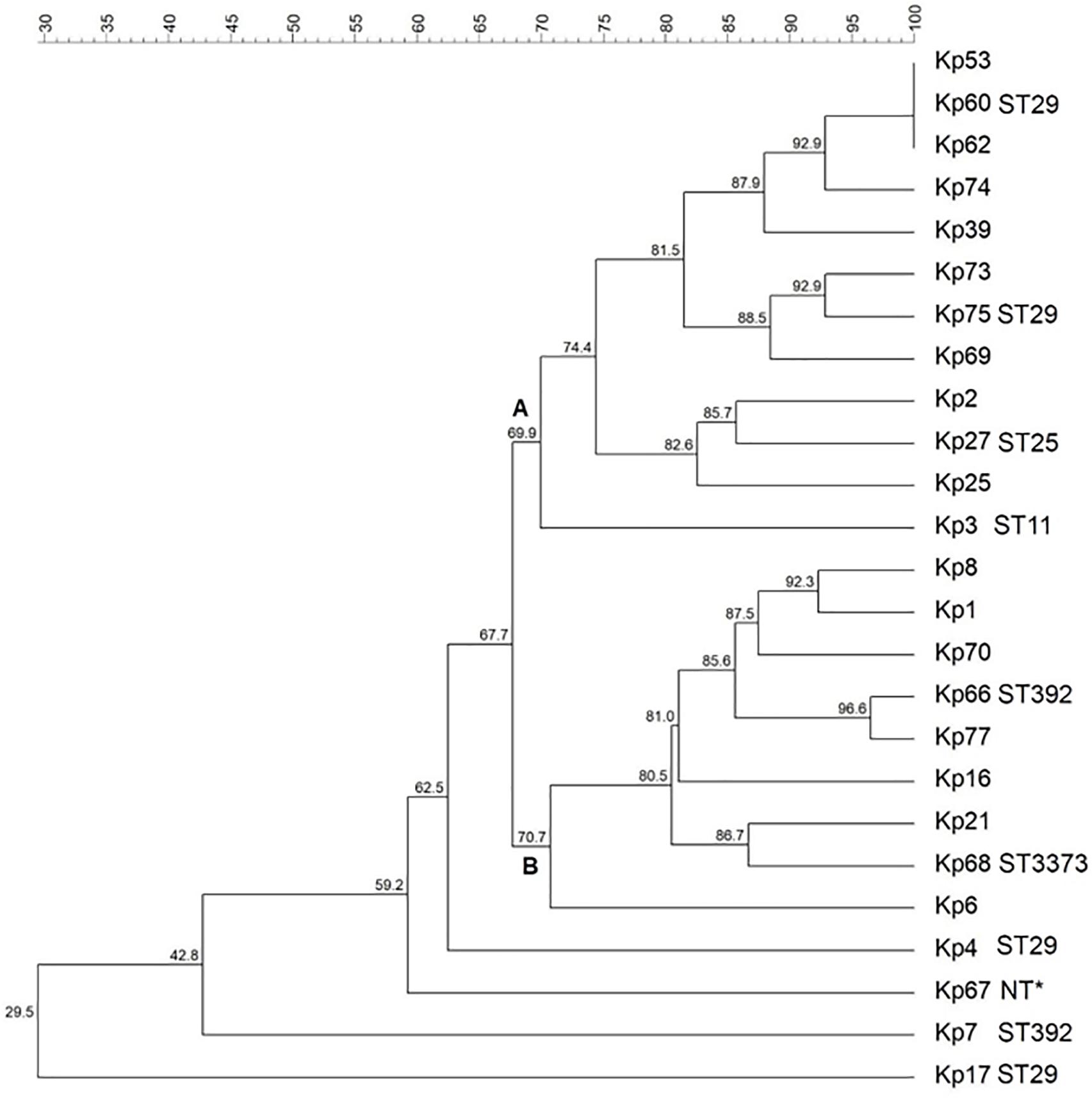
Figure 1. Dendrogram representing the genetic relationship among the 25 Klebsiella pneumoniae studied. Clusters were determined using the Unweighted Pair Group Mean (UPGMA) method and the Dice similarity coefficient. Similarity (%) among patterns is represented by the numbers beside the nodes. For each isolate typed by MLST, their respective sequence types (STs) are represented. ∗NT, not typed by MLST.
MLST
Multilocus sequence typing analysis demonstrated five different STs among 10 selected isolates (Figure 1). Four isolates (Kp4, Kp17, Kp60, and Kp65) belonged to ST29, which was the most predominant group. Furthermore, two isolates (Kp7 and Kp66) belonged to ST392, one isolate (Kp27) belonged to ST25, and another one (Kp3) belonged to ST11. The isolate Kp68 presented a novel ST by way of a new allele combination, which was named ST3373. It was not possible to analyze the isolate Kp67 by MLST because it did not show amplification for the tonB gene, even after several attempts and adjustments in the reaction.
The eBurst analysis showed that most of the STs (STs 11, 25, 29, and 3373) found were distributed in a more massive clonal complex called CC258 (also called CC258/11). Only the ST392 group, including isolates Kp7 and Kp66, was present into a smaller clonal complex, called CC147 (Figure 2).
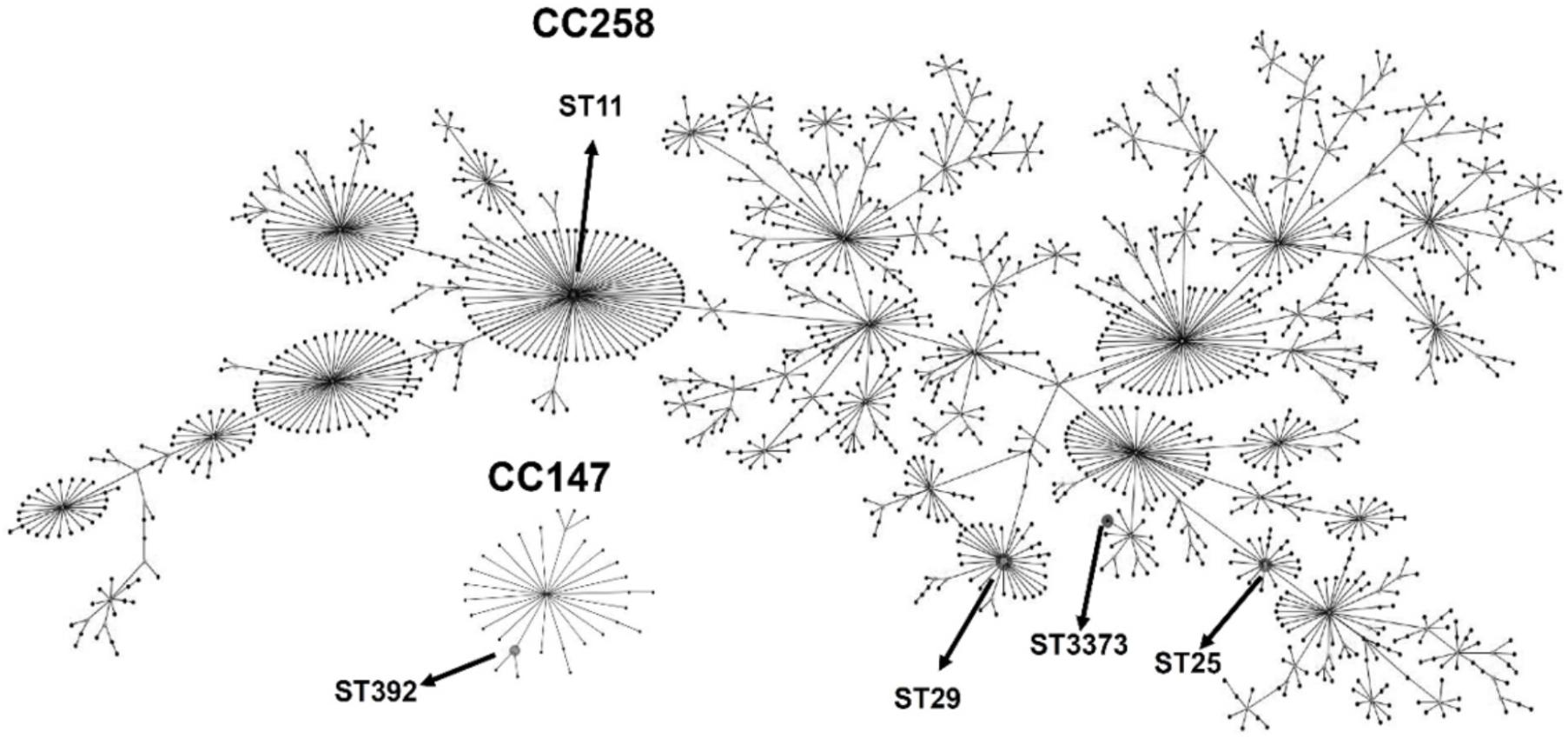
Figure 2. eBURST diagram generated with the MLST data, representing the five different sequence types (STs) obtained in this study (indicated by arrows), distributed in two clonal complexes: CC258, with the STs 11, 29, 25 and the novel ST3373 and CC147, with the ST392. The remaining STs were omitted from the diagram to facilitate visualization. Each dot represents an ST.
Discussion
Although K. pneumoniae is considered to be an important opportunistic pathogen and a frequent cause of hospital-acquired infections (Struve and Krogfelt, 2004), it is also found in non-clinical habitats, which include the mucosal surfaces of humans and animals, and environmental sources such as water, soil, sewage, and vegetation (Bagley, 1985; Podschun et al., 2001). Previous studies have shown that K. pneumoniae strains of environmental origin are similar to those strains of clinical origin in terms of biochemical patterns, virulence, and pathogenicity (Podschun et al., 2001; Struve and Krogfelt, 2004); however, clinical K. pneumoniae are significantly more resistant to antibiotics as compared with environmental K. pneumoniae (Matsen et al., 1974).
In our study, the vast majority (84%, 21/25) of K. pneumoniae isolates showed MDR patterns including a high resistance rate to the common antibiotics used either alone or in association with one another to treat K. pneumoniae infections, such as β-lactams (including carbapenems), aminoglycosides, quinolones, glycylcycline, and polymyxin E. Although the high prevalence of MDR K. pneumoniae patterns was similar to other results in previous studies (Pereira et al., 2013; Paneru, 2015; Wasfi et al., 2016), this is the first report of a high incidence of MDR K. pneumoniae in the state of Tocantins, Brazil. There are many possible contributing factors to the emergence, rise, and spread of antibiotic resistance, including the new acquisition of resistance genes; transfer of antibiotic resistance genes; healthcare exposure; use of indwelling medical devices; limited diagnostic facilities; lack of effective and reliable surveillance systems; immunosuppressed states; travel to areas with a high endemicity of MDR bacteria; lack of new antimicrobial therapeutics; and inappropriate and excessive antibiotic use in health care, food-producing animals, and agriculture (Fletcher, 2015; Vila, 2015; Ayukekbong et al., 2017; Martin and Bachman, 2018; Patolia et al., 2018). Therefore, many of these risk factors may have contributed to the high rates of antibiotic resistance found in our study.
The high rates of resistance to polymyxin E (i.e., CST) and glycylcycline (i.e., TGC) found in our study deserves particular attention because these antibiotic categories have typically been used as the drugs of last resort for the treatment of severe infections caused by Klebsiella pneumoniae carbapenemase (KPC)-producing organisms (Pereira et al., 2013). Previous studies have reported that high levels of CST are frequently administered in Brazilian ICUs, mainly after bacteria isolates have become resistant to almost all other available antibiotics (Furtado et al., 2007; Rossi, 2011). Therefore, the overuse and misuse of antibiotics can be associated with an increase of the occurrence of CST resistance found in the current study. The TGC resistance might be due to the presence of the AcrAB gene, which encodes the efflux pump AcrAB and is considered to be one of the main contributors to a reduced susceptibility to TGC in K. pneumoniae clinical isolates (Bialek-Davenet et al., 2015; Wang et al., 2015; Elgendy et al., 2018). In this study, we also found that several TGC-resistant bacteria were isolated from rectal swabs, showing an important association between pathogen-specific and local antibiotic resistance patterns.
K. pneumoniae produces two classics trimeric porins, OmpK35 and OmpK36, which allow the passage of small hydrophilic molecules such as iron, nutrients, and antibiotics through the outer cell membrane (Tsai et al., 2011). In our study, 28% of all K. pneumoniae isolates lacked the OmpK36 gene. Our findings are in agreement with those of other authors who reported that the absence of OmpK35 or OmpK36 can be responsible for resistance to carbapenems in K. pneumoniae that produced ESBL (Hernandez-Alles et al., 1999; Wang et al., 2009; Skurnik et al., 2010). The loss of both porins OmpK35 and OmpK36 produces an increase in carbapenem, CIP, and chloramphenicol resistance (Kaczmarek et al., 2006). However, some of our results are not in complete agreement with the literature, as the presence of OmpK35 and OmpK36 genes were correlated with both carbapenem and CIP resistance, in 28% of MDR K. pneumoniae isolates. In contrast, other studies have suggested that the presence of both porins (OmpK35 and OmpK36) in MDR isolates can be associated with the presence of point mutations, disruption in the protein coding sequence, or promoter region mutations (Doumith et al., 2009; Wasfi et al., 2016). Further investigations should be performed to evaluate the presence of the mutations in bacteria strains isolated in this study.
Efflux pump systems have been reported as essential mechanisms of resistance and cause of MDR in K. pneumoniae (Mahamoud et al., 2007; Meletis et al., 2012). In K. pneumoniae, the AcrAB and mdtK complexes are the best-characterized efflux pumps (Wasfi et al., 2016). Notably, in our research, the presence of AcrAB-TolC and mdtK genes were strongly associated with MDR K. pneumoniae patterns. These results are consistent with other previous studies, that demonstrated that the multidrug efflux pump system (AcrAB-TolC) in K. pneumoniae was responsible for resistance to quinolones, tetracyclines, TGC, and beta-lactams in various MDR isolates (Padilla et al., 2010; Yuhan et al., 2016).
In K. pneumoniae, the genes fimH and mrkD encode adhesins of type 1 and type 3 fimbriae, which mediate binding to the extracellular matrix; promote biofilm development (Hornick et al., 1992; Struve et al., 2008; Alcántar-Curiel et al., 2013; Fu et al., 2018); and may play a key role in colonization, invasion and pathogenicity (Shah et al., 2017). In the current study, the majority of the MRD K. pneumoniae isolates carried both fimH-1 and mrkD virulence genes. Although studies have reported that many clinical K. pneumoniae isolates normally express both type 1 and type 3 fimbrial adhesins (Sahly et al., 2008; Struve et al., 2009; Wasfi et al., 2016), one of the most important steps in the progression to K. pneumoniae infection is related to its ability to adhere to host surfaces and demonstrate persistent colonization. MrkD specifically mediates binding to the extracellular matrix, facilitating the adherence of K. pneumoniae to damaged tissue and coating indwelling devices (François et al., 1998; Paczosa and Mecsas, 2016), such as urinary catheters (Schroll et al., 2010; Stahlhut et al., 2012) and endotracheal tubes (François et al., 1998). Type 3 fimbriae were found to play an essential role in K. pneumoniae biofilm formation (Langstraat et al., 2001; Di Martino et al., 2003; Jagnow and Clegg, 2003; Schroll et al., 2010) and they can also mediate the binding of K. pneumoniae to endothelial cells and to epithelial cells of the respiratory and urinary tracts (Würker et al., 1990; Hornick et al., 1992; Tarkkanen et al., 1997). Type 1 fimbriae are expressed in 90% of both clinical and environmental K. pneumoniae isolates (Stahlhut et al., 2009); however, their precise role in the production of biofilms remains unclear (Paczosa and Mecsas, 2016). Type 1 fimbriae expressed by K. pneumoniae in particular cause urinary tract infections (Struve et al., 2008), and may play an important role in colonization of the intestine and in the delivery, entry, and persistence of K. pneumoniae in ventilator-associated pneumonia (Kollef, 2004; Struve et al., 2008; Kalanuria et al., 2014). Additionally, the presence of mrkD and fimH-1 has previously been associated with KPC-positive K. pneumoniae (De Cássia et al., 2014), which is in accordance with our findings. Although little is known regarding the potential virulence characteristics of KPC-producing K. pneumonia (Andrade et al., 2014; Liu Y. et al., 2014), studies have reported that ESBL-producing isolates of K. pneumoniae are able to produce more fimbrial adhesins, are more invasive, and are more resistant to the normal human serum bactericidal effect (Sahly et al., 2004). Therefore, the high frequency of fimH-1 (88%) and mrkD gene (96%) found in our results, illustrates the importance of evaluating these virulence factors.
The capsule is one of the most important virulence factors (Martin and Bachman, 2018) that protects K. pneumoniae from lethal serum factors and phagocytosis (Hsu et al., 2011). In K pneumoniae, capsular serotypes K1 and K2 have been considered as predominant virulent strains (Fung et al., 2002; Chuang et al., 2006). Studies using clinical samples have proposed that virulence factors such as K1, K2, K5, rmpA and the aerobactin gene, are absent in KPC-producing isolates (Siu et al., 2012). In agreement with these previous studies, our results showed that K1 and rmpA were not detected, K2 was present in only one isolate, K5 was not investigated, and all isolates were identified as KPC-producing K. pneumoniae. It is important to note that genes encoding rmpA, K1, or K2 were highly associated with the hypervirulent (hypermucoviscous) variant of K. pneumoniae (hvKP) (Fang et al., 2004; Yeh et al., 2007; Arena et al., 2017; Martin and Bachman, 2018), which causes serious community-acquired infection, and has emerged as a carbapenem-resistant hypervirulent K. pneumoniae (CR-HvKP) that can be found in clinical settings (Shon et al., 2013; Liu Y.M. et al., 2014; Zhang et al., 2015; Zhang Y. et al., 2016; Zhang R. et al., 2016). Therefore, this observation suggests that the K. pneumoniae in this study did not present molecular characteristics of the hypervirulent (hypermucoviscous) K. pneumoniae.
Siderophores are high-affinity, iron-chelating molecules that are critical for bacterial growth, replication, and virulence (Lawlor et al., 2007; Bachman et al., 2015; Holden and Bachman, 2015). The repertoire of siderophores differs among different strains (Behnsen and Raffatellu, 2016); thus, the role of each siderophore in virulence potential can vary (Paczosa and Mecsas, 2016; Lam et al., 2018). Siderophore-associated genes, such as entB, ybtS and iutA are widely disseminated among K. pneumonia strains (Compain et al., 2014). However, entB is only characterized for virulence when it occurs in association with iutA, ybtS, or kfu (Daehre et al., 2018). In agreement with previous studies, all K. pneumoniae carried the entB gene (Lavigne et al., 2013; Fu et al., 2018); however, the presence of the genes encoding entB in combination with iutA and ybtS was found in only 40%, while entB with ybtS were found in 60% of all the strains, respectively. Although K. pneumoniae secretes a specific combination of siderophores, which can affect tissue localization, systemic spreading, and host survival, the effect of these molecules on the host during infection is not clear (Holden et al., 2016).
Carbapenems are the antibiotic class of choice for the treatment of severe infections caused by Enterobacteriaceae-producing ESBLs (Jacoby and Munoz-Price, 2005). The primary determinant of carbapenem resistance in K. pneumoniae is KPC-type carbapenemases (Nordmann et al., 2011), which are encoded by the gene blaKPC and located mainly on a Tn3-based transposon, Tn4401 (Bina et al., 2015), demonstrating exceptional potential to spread throughout the world. In our findings, the presence of blaKPC in all K. pneumoniae isolates is in agreement with previous investigations, that suggest the wide dissemination of KPC-producing isolates in various regions of Brazil (Castanheira et al., 2012; Pereira et al., 2013; Biberg et al., 2015; Gonçalves et al., 2017). Besides, PCR analysis demonstrated that most bacteria (84%) coproduced the blaKPC and blaOXA-1 group resistance genes. In Brazil, several studies have reported the co-occurrence of blaKPC with the blaOXA-1 group in K. pneumoniae (Fehlberg et al., 2012; Flores et al., 2016). Furthermore, blaIMP, blaVIM, blaOXA48, and blaNDM are also genes that produce carbapenemases in K. pneumoniae (Lascols et al., 2012; Seibert et al., 2014); however, these genes were not found in our study.
Some reports have suggested that TEM (Temoniera), SHV (sulfhydryl variable), and CTX-M (cefotaxime-beta lactamases) are the primary genetic groups of ESBLs among clinically critical Gram-negative bacteria (Bradford, 2001; Paterson and Bonomo, 2005). Additional studies have indicated the presence of blaCTX-M, blaTEM, and blaSHV genes in K. pneumoniae (Monteiro et al., 2009; Peirano et al., 2009; Seki et al., 2011; Fehlberg et al., 2012), which is in accordance with our results. Globally, the CTX-M type has appeared as the most common type of ESBL, and its incidence is easily surpassing those of SHV and TEM ESBLs in most locales (Jorgensen et al., 2010; Bora et al., 2014). Although our PCR analysis revealed that blaTEM (100%) was the most frequent gene, followed by blaSHV (96%), the presence of the blaCTX-M (72%) group was also high, and can be related to the fluoroquinolone and aminoglycoside resistance (Pitout et al., 2005) found in this study. The co-production of blaKPC with blaTEM was detected in all isolates, while blaKPC, blaOXA, blaTEM, blaSHV, and blaCTX-M were observed in 72% and blaKPC, blaTEM, blaSHV, and blaCTX-M were found in 68% of the K. pneumoniae isolates, respectively. Our results suggest that the high antimicrobial resistance found in this study can also be associated with the presence of these β-lactams genes.
Our ERIC-PCR results indicated that, although bacteria were isolated from different patients, the circulating K. pneumoniae in this hospital have a high genetic relationship to each other. Ten isolates belonging to the main ERIC-PCR clusters were analyzed by MLST, and four of them (Kp4, Kp17, Kp60, and Kp65) belonged to ST29. ST29 has previously been reported in K. pneumoniae strains from various parts of the world, such as Europe, Asia, Oceania, and also in Brazil. Uz Zaman et al. (1994) found ST29 in MDR K. pneumoniae carrying the OXA-48 gene that showed variations in outer membrane protein 36, causing an outbreak in a tertiary care hospital in Saudi Arabia. However, the isolates from our study with ST29 were negative for OmpK36 and OXA-48 (Tables 4, 5). The ST25 has been described as being associated with virulent clones, especially belonging to the capsular serotypes K1 and K2 (McCulloh and Opal, 2018). In our study, the only isolate that presented the K2 antigen (Kp27) and various virulence genes also presented the ST25; thus, our findings corroborate with the prior research (Table 5). ST11, found in the isolate Kp3, has been described as widespread in Brazil and is considered an international high-risk clone (Gonçalves et al., 2017).
eBURST analysis showed that, except for ST392, all other STs belong to the large clonal complex CC258. Commonly, K. pneumoniae isolates grouped into CC258 are associated with the production of carbapenemases and harbor many virulence genes (Gonçalves et al., 2017), which corroborates with our results (Table 5). Moreover, the ST392, found in the Kp66 isolate, is part of CC147, which is a small internationally successful clonal complex and has been shown to be an important epidemic clone. Hasan et al. (2014) described a clonal expansion of CC147 by Verone integron-encoded metallo-beta-lactamase (VIM)-producing K. pneumoniae strains isolated from Greece. ST392 has been reported worldwide as an emergent clone associated with the spreading KPC-producing K. pneumoniae (Yang et al., 2013; Di Mento et al., 2018; Garza-Ramos et al., 2018). In Brazil, ST392 was previously reported in a KPC-2-producing K. pneumoniae harboring the mcr-1 gene.
Conclusion
Our results revealed a worrying situation concerning K. pneumoniae that is resistant to the drugs commonly used to treat infections and as well as those used as a last resort for life-threatening infections in patients admitted to the ICU. Additionally, our findings demonstrated the presence of high-risk international clones among isolates. Therefore, our data should be interpreted as an alert for need for prevention and control of the MDR K. pneumoniae in hospital settings. A careful and continued surveillance system that provides epidemiological and molecular information is important to limit the risk of infection and the spread of these strains.
Author Contributions
RF, BS, and GR performed the experiments. MB kindly provided the strains and aided with the phenotypic detection of antibiotic resistance. ES aided with the writing and edition of the manuscript. EC aided with the sequencing analysis and the sequence submission to the NCBI platform. MCP, AC, AP-S, and CF conceived the idea, wrote the manuscript and analyzed the data. MLST and ERIC-PCR were performed by RN-S.
Funding
This work was supported by Fundação de Amparo a Pesquisa do Estado de São Paulo (FAPESP grants 2016/10130-8 to AC, FAPESP grants 2018/26100-5 to MCP and 2013/22581-5 to AP-S), Conselho Nacional de Desenvolvimento Científico e Tecnológico (CNPq, grants 2013/485873 to MCP). This study was financed in part by the Coordenação de Aperfeiçoamento de Pessoal de Nível Superior – Brazil (CAPES) Finance code 001 as a fellowship to GR.
Conflict of Interest Statement
The authors declare that the research was conducted in the absence of any commercial or financial relationships that could be construed as a potential conflict of interest.
Acknowledgments
The authors thank the Laboratório Central de Saúde Pública do Tocantins, Palmas, TO, Brazil (LACEN-TO) who kindly provided the Klebsiella pneumoniae strains and Secretaria de Saúde do Estado do Tocantins (SESAU-TO) for facilitating the development of project.
Supplementary Material
The Supplementary Material for this article can be found online at: https://www.frontiersin.org/articles/10.3389/fmicb.2018.03198/full#supplementary-material
Footnotes
- ^http://www.eucast.org/clinical_breakpoints/
- ^https://blast.ncbi.nlm.nih.gov/
- ^http://www.pasteur.fr/recherche/genopole/PF8/mlst/Kpneumoniae.html
References
Alcántar-Curiel, M. D., Blackburn, D., Saldaña, Z., Gayosso-Vázquez, C., Iovine, N. M., De la Cruz, M. A., et al. (2013). Multi-functional analysis of Klebsiella pneumoniae fimbrial types in adherence and biofilm formation. Virulence 4, 129–138. doi: 10.4161/viru.22974
Amjad, A., Mirza, I. A., Abbasi, S., Farwa, U., Malik, N., and Zia, F. (2011). Modified Hodge test: a simple and effective test for detection of carbapenemase production. Iran J. Microbiol. 3, 189–193.
Andrade, B. G. N., de Veiga Ramos, N., Marin, M. F. A., Fonseca, E. L., and Vicente, A. C. P. (2014). The genome of a clinical Klebsiella variicola strain reveals virulence-associated traits and a pl9-like plasmid. FEMS Microbiol. Lett. 360, 13–16. doi: 10.1111/1574-6968.12583
Arena, F., Henrici, De Angelis, L., D’Andrea, M. M., Cannatelli, A., Fossati, L., et al. (2017). Infections caused by carbapenem-resistant Klebsiella pneumoniae with hypermucoviscous phenotype: a case report and literature review. Virulence 8, 1900–1908. doi: 10.1080/21505594.2017.1286439
Ayukekbong, J. A., Ntemgwa, M., and Atabe, A. N. (2017). The threat of antimicrobial resistance in developing countries: causes and control strategies. Antimicrob. Resist. Infect. Control 6:47. doi: 10.1186/s13756-017-0208-x
Babini, G. S., and Livermore, D. M. (2000). Are SHV beta-lactamases universal in Klebsiella pneumoniae? Antimicrob. Agents Chemother. 44:2230. doi: 10.1128/AAC.44.8.2230-2230.2000
Bachman, M. A., Breen, P., Deornellas, V., Mu, Q., Zhao, L., Wu, W., et al. (2015). Genome-wide identification of Klebsiella pneumoniae fitness genes during lung infection. mBio 6:e00775. doi: 10.1128/mBio.00775-15
Bagley, S. T. (1985). Habitat association of Klebsiella species. Infect. Control 6, 52–58. doi: 10.1017/S0195941700062603
Behnsen, J., and Raffatellu, M. (2016). Siderophores: more than stealing iron. mBio 7:e01906-16. doi: 10.1128/mBio.01906-16
Bialek-Davenet, S., Lavigne, J. P., Guyot, K., Mayer, N., Tournebize, R., Brisse, S., et al. (2015). KPC-2-producing Klebsiella pneumoniae in a hospital in the Midwest region of Brazil. Braz. J. Microbiol. 46, 501–504. doi: 10.1590/S1517-838246246220140174
Biberg, C. A., Rodrigues, A. C., do Carmo, S. F., Chaves, C. E., Gales, A. C., and Chang, M. R. (2015). KPC-2-producing Klebsiella pneumoniae in a hospital in the Midwest region of Brazil. Braz. J. Microbiol. 46, 501–504. doi: 10.1590/S1517-838246246220140174
Bina, M., Pournajaf, A., Mirkalantari, S., Talebi, M., and Irajian, G. (2015). Detection of the Klebsiella pneumoniae carbapenemase (KPC) in K. pneumoniae Isolated from the Clinical Samples by the Phenotypic and Genotypic Methods. Iran. J. Pathol. 10, 199–205.
Bora, A., Hazarika, N. K., Shukla, S. K., Prasad, K. N., Sarma, J. B., and Ahmed, G. (2014). Prevalence of blaTEM, blaSHV and blaCTX-M genes in clinical isolates of Escherichia coli and Klebsiella pneumoniae from Northeast India. Indian J. Pathol. Microbiol. 57, 249–254. doi: 10.4103/0377-4929.134698
Bradford, P. A. (2001). Extended-spectrum beta-lactamases in the 21st century: characterization, epidemiology, and detection of this important resistance threat. Clin. Microbiol. 14, 933–951. doi: 10.1128/CMR.14.4.933-951.2001
Call, D. R., Bakko, M. K., Krug, M. J., and Roberts, M. C. (2003). Identifying antimicrobial resistance genes with DNA microarrays. Antimicrob. Agents Chemother. 47, 3290–3295. doi: 10.1128/AAC.47.10.3290-3295.2003
Castanheira, M., Costello, A. J., Deshpande, L. M., and Jones, R. N. (2012). Expansion of clonal complex 258 KPC-2-producing Klebsiella pneumoniae in Latin American hospitals: report of the SENTRY antimicrobial surveillance program. Antimicrob. Agents Chemother. 56, 1668–1669. doi: 10.1016/j.bjid.2016.04.003
Chuang, Y. P., Fang, C. T., Lai, S. Y., Chang, S. C., and Wang, J. T. (2006). Genetic determinants of capsular serotype K1 of Klebsiella pneumoniae causing primary pyogenic liver abscess. J. Infect. Dis. 193, 645–654.
Clinical and Laboratory Standards Institute [CLSI] (2017). Performance Standards for Antimicrobial Susceptibility Testing; Supplement M100, 27th Edn. Wayne, PA: Clinical and Laboratory Standards Institute.
Compain, F., Babosan, A., Brisse, S., Genel, N., Audo, J., Ailloud, F., et al. (2014). Multiplex PCR for detection of seven virulence factors and K1/K2 capsular serotypes of Klebsiella pneumoniae. J. Clin. Microbiol. 52, 4377–4380. doi: 10.1128/JCM.02316-14
Da Silva, G. J., and Mendonça, N. (2012). Association between antimicrobial resistance and virulence in Escherichia coli. Virulence 3, 18–28. doi: 10.4161/viru.3.1.18382
Daehre, K., Projahn, M., Friese, A., Semmler, T., Guenther, S., and Roesler, U. H. (2018). ESBL-Producing Klebsiella pneumoniae in the broiler production chain and the first description of ST3128. Front. Microbiol. 3:2302. doi: 10.3389/fmicb.2018.02302
Dallenne, C., Da Costa, A., Decre, D., Favier, C., and Arlet, G. (2010). Development of a set of multiplex PCR assays for the detection of genes encoding important b-lactamases in Enterobacteriaceae. J. Antimicrob. Chemother. 65, 490–495. doi: 10.1093/jac/dkp498
Davies, J., and Davies, D. (2010). Origins and evolution of antibiotic resistance. Microbiol. Mol. Biol. Rev. 74, 417–433. doi: 10.1128/MMBR.00016-10
De Cássia, A. M. R., de Barros, E. M., Loureiro, N. G., de Melo, H. R., Maciel, M. A., and Souza, A. C. L. (2014). Presence of fimH, mrkD, and irp2 virulence genes in KPC-2-producing Klebsiella pneumoniae isolates in Recife-PE, Brazil. Curr. Microbiol. 69, 824–831. doi: 10.1007/s00284-014-0662-0
Derakhshan, S., Peerayeh, S. N., and Bakhshi, B. (2016). Association between presence of virulence genes and antibiotic resistance in clinical Klebsiella Pneumoniae isolates. Lab. Med. 47, 306–311. doi: 10.1093/labmed/lmw030
Di Martino, P., Cafferini, N., Joly, B., and Darfeuille-Michaud, A. (2003). Klebsiella pneumoniae type 3 pili facilitate adherence and biofilm formation on abiotic surfaces. Res. Microbiol. 154, 9–16. doi: 10.1016/S0923-2508(02)00004-9
Di Mento, G., Cuscino, N., Carcione, C., Cardinale, F., Conaldi, P. G., and Douradinha, B. (2018). Emergence of a Klebsiella pneumoniae ST392 clone harbouring KPC-3 in an Italian transplantation hospital. J. Hosp. Infect. 98, 313–314. doi: 10.1016/j.jhin.2017.11.019
Doumith, M., Ellington, M. J., Livermore, D. M., and Woodford, N. (2009). Molecular mechanisms disrupting porin expression in ertapenem-resistant Klebsiella and Enterobacter spp. clinical isolates from the UK. J Antimicrob Chemother. 63, 659–667. doi: 10.1093/jac/dkp029
Doyle, D., Peirano, G., Lascols, C., Lloyd, T., Church, D. L., and Pitout, J. D. (2012). Laboratory detection of Enterobacteriaceae that produce carbapenemases. J. Clin. Microbiol. 50, 3877–3880. doi: 10.1128/JCM.02117-12
Dsouza, R., Pinto, N. A., Hwang, I., Cho, Y., Yong, D., Choi, J., et al. (2017). Panel strain of Klebsiella pneumoniae for beta-lactam antibiotic evaluation: their phenotypic and genotypic characterization. PeerJ 5:e2896. doi: 10.7717/peerj.2896
El Fertas-Aissani, R., Messai, Y., Alouache, S., and Bakour, R. (2013). Virulence profiles and antibiotic susceptibility patterns of Klebsiella pneumoniae strains isolated from different clinical specimens. Pathol. Biol. 61, 209–216. doi: 10.1016/j.patbio.2012.10.004
Elgendy, S. G., Abdel Hameed, M. R., and El-Mokhtar, M. A. (2018). Tigecycline resistance among Klebsiella pneumoniae isolated from febrile neutropenic patients. J. Med. Microbiol. 67, 972–975. doi: 10.1099/jmm.0.000770
Fair, R. J., and Tor, Y. (2014). Antibiotics and bacterial resistance in the 21st Century. Perspect. Med. Chem. 6, 25–64. doi: 10.4137/PMC.S14459
Fang, C. T., Lai, S. Y., Yi, W. C., Hsueh, P. R., Liu, K. L., and Chang, S. C. (2007). Klebsiella pneumoniae genotype K1: an emerging pathogen that causes septic ocular or central nervous systemcomplications from pyogenic liver abscess. Clin. Infect. Dis. 45, 284–293. doi: 10.1086/519262
Fang, C. T., Yi-Ping, C., Chia-Tung, S., Chang, S. C., and Wang, J. T. (2004). A Novel Virulence Gene in Klebsiella pneumoniae strains causing primary liver abscess and septic metastatic complications. J. Exp. Med. 199, 697–705. doi: 10.1084/jem.20030857
Fehlberg, L. C., Carvalho, A. M., Campana, E. H., Gontijo-Filho, P. P., and Gales, A. C. (2012). Emergence of Klebsiella pneumoniae-producing KPC-2 carbapenemase in Paraíba. Northeastern Brazil. Braz. J. Infect. Dis. 16, 577–580. doi: 10.1016/j.bjid.2012.07.001
Feil, E. J., Li, B. C., Aanensen, D. M., Hanage, W. P., and Spratt, B. G. (2004). eBURST: inferring patterns of evolutionary descent among clusters of related bacterial genotypes from multilocus sequence typing data. J. Bacteriol. 186, 1518–1530. doi: 10.1128/JB.186.5.1518-1530.2004
Fletcher, S. (2015). Understanding the contribution of environmental factors in the spread of antimicrobial resistance. Environ. Health Prev. Med. 20, 243–252. doi: 10.1007/s12199-015-0468-0
Flores, C., Maria, C. P. A., Kayo, B., Chaia, M. C., Angela, B., Ana Paula, S. S., et al. (2016). Detection of antimicrobial resistance genes in beta-lactamase- and carbapenemase-producing Klebsiella pneumoniae by patient surveillance cultures at an intensive care unit in Rio de Janeiro, Brazil. J. Bras. Patol. Med. Lab. 52, 1678–4774. doi: 10.5935/1676-2444.20160049
François, P., Vaudaux, P., and Lew, P. D. (1998). Role of plasma and extracellular matrix proteins in the physiopathology of foreign body infections. Ann. Vasc. Surg. 12, 34–40. doi: 10.1007/s100169900112
Fu, L., Huang, M., Zhang, X., Yang, X., Liu, Y., Zhang, L., et al. (2018). Frequency of virulence factors in high biofilm formation blaKPC-2 producing Klebsiella pneumoniae strains from hospitals. Microb. Pathog. 116, 168–172. doi: 10.1016/j.micpath.2018.01.030
Fung, C. P., Chang, F. Y., Lee, S. C., Hu, B. S., Kuo, B. I., Liu, C. Y., et al. (2002). A global emerging disease of Klebsiella pneumoniae liver abscess: is serotype K1 an important factor for complicated endophthalmitis? Gut 50, 420–424. doi: 10.1136/gut.50.3.420
Furtado, G. H. C., d’Azevedo, P. A., Santos, A. F., Gales, A. C., Pignatari, A. C., and Medeiros, E. A. (2007). Intravenous polymyxin B for the treatment of nosocomial pneumonia caused by multidrug-resistant Pseudomonas aeruginosa. Int. J. Antimicrob. Agents 30, 315–319. doi: 10.1016/j.ijantimicag.2007.05.017
Garza-Ramos, U., Barrios-Camacho, H., Moreno-Domínguez, S., Toribio-Jiménez, J., Jardón-Pineda, D., Cuevas-Peña, J., et al. (2018). A Phenotypic and molecular characterization of Klebsiella spp. isolates causing community-acquired infections. New Microbes New Infect. 23, 17–27.
Gonçalves, G. B., Furlan, J. P. R., Vespero, E. C., Pelisson, M., Stehling, E. G., and Silva, P. A. (2017). Spread of multidrug-resistant high-risk Klebsiella pneumoniae clones in a tertiary hospital from southern Brazil. Infect. Genet. Evol. 56, 1–7. doi: 10.1016/j.meegid.2017.10.011
Gorrie, C. L., Mirceta, M., Wick, R. R., Edwards, D. J., Thomson, N. R., and Strugnell, R. A. (2017). Gastrointestinal carriage is a major reservoir of Klebsiella pneumoniae infection in intensive care patients. Clin. Infect. Dis. 65, 208–215. doi: 10.1093/cid/cix270
Hasan, C. M., Turlej-Rogacka, A., Vatopoulos, A. C., Giakkoupi, P., Maâtallah, M., and Giske, C. G. (2014). Dissemination of blaVIM in Greece at the peak of the epidemic of 2005-2006: clonal expansion of Klebsiella pneumoniae clonal complex 147. Clin. Microbiol. Infect 20, 34–37. doi: 10.1111/1469-0691.12187
Hernandez-Alles, S., Albertí, S., Alvarez, D., Doménech-Sánchez, A., Martínez-Martínez, L., and Gil, J. (1999). Porin expression in clinical isolates of Klebsiella pneumoniae. Microbiology 145, 673–679. doi: 10.1099/13500872-145-3-673
Holden, V. I., and Bachman, M. A. (2015). Diverging roles of bacterial siderophores during infection. Metallomics 7, 986–995. doi: 10.1039/c4mt00333k
Holden, V. I., Breen, P., Houle, S., Dozois, C. M., and Bachman, M. A. (2016). Klebsiella pneumoniae siderophores induce inflammation, bacterial dissemination, and HIF-1α stabilization during pneumonia. mBio 7:e01397-16. doi: 10.1128/mBio.01397-16
Hornick, D. B., Allen, B. L., Horn, M. A., and Clegg, S. (1992). Adherence to respiratory epithelia by recombinant Escherichia coli expressing Klebsiella pneumoniae type 3 fimbrial gene products. Infect. Immun. 60, 1577–1588.
Hsu, C. R., Lin, T. L., Chen, Y. C., Chou, H. C., and Wang, J. T. (2011). The role of Klebsiella pneumoniae rmpA in capsular polysaccharide synthesis and virulence revisited. Microbiology 157, 3446–3457. doi: 10.1099/mic.0.050336-0
Jacoby, G. A., and Munoz-Price, L. S. (2005). The new β-lactamases. N. Engl. J. Med. 352, 380–391. doi: 10.1056/NEJMra041359
Jagnow, J., and Clegg, S. (2003). Klebsiella pneumoniae MrkD-mediated biofilm formation on extracellular matrix- and collagen-coated surfaces. Microbiology 149, 2397–2405. doi: 10.1099/mic.0.26434-0
Jorgensen, J. H., McElmeel, M. L., Fulcher, L. C., and Zimmer, B. L. (2010). Detection of CTX-M-type extended-spectrum beta-lactamase (ESBLs) by testing with MicroScan overnight and ESBL confirmation panels. J. Clin. Microbiol. 48, 120–123. doi: 10.1128/JCM.01507-09
Kaczmarek, F. M., Dib-Hajj, F., Shang, W., and Gootz, T. D. (2006). High-Level Carbapenem Resistance in a Klebsiella pneumoniae Clinical Isolate Is Due to the Combination of bla (ACT-1) β-Lactamase Production, Porin OmpK35/36 Insertional Inactivation, and Down-Regulation of the Phosphate Transport Porin PhoE. Antimicrob. Agents Chemother. 50, 3396–3406. doi: 10.1128/AAC.00285-06
Kalanuria, A. A., Zai, W., and Mirski, M. (2014). Ventilator-associated pneumonia in the ICU. Crit. Care 18:208. doi: 10.1186/cc13775
Karuniawati, A., Saharman, Y. R., and Lestari, D. C. (2013). Detection of carbapenemase encoding genes in Enterobacteriace, Pseudomonas aeruginosa, and Acinetobacter baumanii isolated from patients at Intensive Care Unit Cipto Mangunkusumo Hospital in 2011. . Acta Med. Indones. 45, 101–106.
Kollef, M. H. (2004). Prevention of hospital-associated pneumonia and ventilator-associated pneumonia. Crit. Care Med. 32, 1396–1405. doi: 10.1097/01.CCM.0000128569.09113
Lam, M. M. C., Wyres, K. L., Judd, L. M., Wick, R. R., Jenney, A., Brisse, S., et al. (2018). Tracking key virulence loci encoding aerobactin and salmochelin siderophore synthesis in Klebsiella pneumoniae. Genome Med. 10:77. doi: 10.1186/s13073-018-0587-5
Langstraat, J., Bohse, M., and Clegg, S. (2001). Type 3 fimbrial shaft (MrkA) of Klebsiella pneumoniae, but not the fimbrial adhesin (MrkD), facilitates biofilm formation. Infect. Immun. 69, 5805–5812. doi: 10.1128/IAI.69.9.5805-5812.2001
Lascols, C., Hackel, M., Hujer, A. M., Marshall, S. H., Bouchillon, S. K., Hoban, D. J., et al. (2012). Using nucleic acid microarrays to perform molecular epidemiology and detect novel β-lactamases: a snapshot of extended-spectrum b-lactamases trought the world. J. Clin. Microbiol. 50, 1632–1639. doi: 10.1128/JCM.06115-11
Lavigne, J. P., Cuzon, G., Combescure, C., Bourg, G., Sotto, A., and Nordmann, P. (2013). Virulence of Klebsiella pneumoniae isolates harboring bla KPC-2 carbapenemase gene in a Caenorhabditis elegans model. PLoS One 8:e67847. doi: 10.1371/journal.pone.0067847
Lawlor, M. S., O’Connor, C., and Miller, V. L. (2007). Yersiniabactin is a virulence factor for Klebsiella pneumoniae during pulmonary infection. Infect. Immun. 75, 1463–1472. doi: 10.1128/IAI.00372-06
Liu, Y., Li, X. Y., Wan, L. G., Jiang, W. Y., Yang, J. H., and Li, F. Q. (2014). Virulence and transferability of resistance determinants in a novel Klebsiella pneumoniae sequence type 1137 in China. Microb. Drug Resist. 20, 150–155. doi: 10.1089/mdr.2013.0107
Liu, Y. M., Li, B. B., Zhang, Y. Y., Zhang, W., Shen, H., Li, H., et al. (2014). Clinical and molecular characteristics of emerging hypervirulent Klebsiella pneumoniae bloodstream infections in mainland China. Antimicrob. Agents Chemother. 58, 5379–5385. doi: 10.1128/AAC.02523-14
Liu, Y. Y., Wang, Y., Walsh, T. R., Yi, L. X., Zhang, R., Spencer, J., et al. (2015). Emergence of plasmid mediated colistin resistance mechanism MCR-1 in animals and human beings in China: a microbiological and molecular biological study. Lancet Infect. 16, 161–168. doi: 10.1016/S1473-3099(15)00424-7
Magiorakos, A. P., Srinivasan, A., Carey, R. B., Carmeli, Y., Falagas, M. E., Giske, C. G., et al. (2012). Multidrug-resistant, extensively drug-resistant and pandrug-resistant bacteria: an international expert proposal for interim standard definitions for acquired resistance. Clin. Microbiol. Infect. 18, 268–281. doi: 10.1111/j.1469-0691.2011.03570.x
Mahamoud, A., Chevalier, J., Albert-Francho, S., Kern, W. N., and Pages, J. M. (2007). Antibiotic efflux pumps in gram negative bacteria: the inhibitor response strategy. J. Antimicrob. Chem. 59, 1223–1229. doi: 10.1093/jac/dkl493
Martin, R. M., and Bachman, M. A. (2018). Colonization, infection, and the accessory genome of Klebsiella pneumoniae. Front. Cell Infect. Microbiol. 22:4. doi: 10.3389/fcimb.2018.00004
Martins, A. F., Zavascki, A. P., Gaspareto, P. B., and Barth, A. L. (2007). Dissemination of Pseudomonas aeruginosa producing SPM-1-like and IMP-1-like metallo-beta-lactamases in hospitals from southern Brazil. Infection 35, 457–460. doi: 10.1007/s15010-007-6289-3
Matsen, J. M., Spindler, J. A., and Blosser, R. O. (1974). Characterization of Klebsiella isolates from natural receiving waters and comparison with human isolates. Appl. Microbiol. 28, 672–678.
McCulloh, R. J., and Opal, S. M. (2018). “Sepsis management: important role of the pathogen,” in Handbook of Sepsis, Chap. 3, eds W. J. Wiersinga and C. Seymour (Chennai: Springer Nature Publishers), 174-193.
Meletis, G., Exindari, M., Vavatsi, N., Sofianou, D., and Diza, E. (2012). Mechanisms responsible for the emergence of carbapenem resistance in Pseudomonas aeruginosa. Hippokratia 16, 303–307.
Miriagou, V., Cornaglia, G., Edelstein, M., Galani, I., Giske, C. G., Gniadkowski, M., et al. (2010). Acquired carbapenemases in Gram-negative bacterial pathogens: detection and surveillance issues. Clin. Microbiol. Infect. 16, 112–122. doi: 10.1111/j.1469-0691.2009.03116.x
Monteiro, J., Santos, A., Asensi, M. D., Peirano, G., and Gales, A. C. (2009). First report KPC-2 producing Klebsiella pneumonia strains in Brazil. Antimicrob. Agents Chemother. 53, 333–334. doi: 10.1128/AAC.00736-08
Nordmann, P., Naas, T., and Poirel, L. (2011). Global spread of Carbapenemase-producing Enterobacteriaceae. Emerg. Infect. Dis. 17, 1791–1798. doi: 10.3201/eid1710.110655
Okoche, D., Asiimwe, B. B., Katabazi, F. A., Kato, L., and Najjuka, C. F. (2015). Prevalence and characterization of carbapenem-resistant Enterobacteriaceae isolated from mulago national referral hospital, Uganda. PLoS One 10:e0135745. doi: 10.1371/journal.pone.0135745
Okonechnikov, K., Golosova, O., Fursov, M., and Ugene team. (2012). Unipro UGENE: a unified bioinformatics toolkit. Bioinformatics 28, 1166–1167. doi: 10.1093/bioinformatics/bts091
Paczosa, M. K., and Mecsas, J. (2016). Klebsiella pneumoniae: going on the offense with a strong defense. Microbiol. Mol. Biol. Rev. 80, 629–661. doi: 10.1128/MMBR.00078-15
Padilla, E., Llobet, E., Doménech-Sánchez, A., Martínez-Martínez, L., Bengoechea, J. A., and Albertí, S. (2010). Klebsiella pneumoniae AcrAB efflux pump contributes to antimicrobial resistance and virulence. Antimicrob. Agents Chemother. 54, 177–183. doi: 10.1128/AAC.00715-09
Paneru, T. P. (2015). Surveillance of Klebsiella pneumoniae and antibiotic resistance a retrospective and comparative study through a period in Nepal. Danish J. Med. Biol. Sci. 29–36. doi: 10.6084/m9.figshare.1466724
Papp-Wallace, K. M., Endimiani, A., Taracila, M. A., and Bonomo, R. A. (2011). Carbapenems: past, present, and future. Antimicrob. Agents Chemother. 55, 4943–4960. doi: 10.1128/AAC.00296-11
Paterson, D. L., and Bonomo, R. A. (2005). Extended-spectrum beta-lactamases: a clinical update. Clin. Microbiol. Rev. 18, 657–686. doi: 10.1128/cmr.18.4.657-686.2005
Patolia, S., Abate, G., Patel, N., and Patolia, S. (2018). Frey S. Risk factors and outcomes for multidrug-resistant Gram-negative bacilli bacteremia. Ther. Adv. Infect. Dis. 5, 11–18. doi: 10.1177/2049936117727497
Peirano, G., Seki, M. L., Passos, V. L. V., Cristina, M. F. G., Guerra, R. L., and Asensi, M. D. (2009). Carbapenem-hydrolysing beta-lactamase KPC-2 in Klebsiella pneumoniae isolated in Rio de Janeiro, Brazil. J. Antimicrob. Chemother. 63, 265–268. doi: 10.1093/jac/dkn484
Pereira, P. S., Araujo, C. F. M., Seki, L. M., Zahner, V., Carvalho-Assef, A. P. D., and Asensi, M. D. (2013). Update of the molecular epidemiology of KPC-2-producing Klebsiella pneumonia in Brazil: spread of clonal complex 11 (ST11, ST437 and ST340). J Antimicrob Chemother. 68, 312–316. doi: 10.1093/jac/dks396
Pitout, J. D. D., Nordmann, P., Laupland, K. B., and Poirel, L. (2005). Emergence of Enterobacteriaceae producing extended-spectrum β-lactamases (ESBLs) in the community. J. Antimicrob. Chemother. 10, 1–8. doi: 10.1093/jac/dki166
Podschun, R., Pietsch, S., Höller, C., and Ullmann, U. (2001). Incidence of Klebsiella species in surface waters and their expression of virulence factors. Appl. Environ. Microbiol. 67, 3325–3327. doi: 10.1128/AEM.67.7.3325-3327.2001
Poirel, L., Dortet, L., Bernabeu, S., and Nordmann, P. (2011). Genetic features of blaNDM-1-Positive Enterobacteriaceae. Antimicrob. Agents Chemother. 55, 5403–5407. doi: 10.1128/AAC.00585-11
Rossi, F. (2011). The challenges of antimicrobial resistance in Brazil. Clin. Infect. Dis. 52, 1138–1143. doi: 10.1093/cid/cir120
Sahly, H., Aucken, H., Benedí, V. J., Forestier, C., Fussing, V., Hansen, D. S., et al. (2004). Increased serum resistance in Klebsiella pneumoniae strains producing extended-spectrum b-lactamases. Antimicrob. Agents Chemother. 48, 3477–3482. doi: 10.1123/AAC.48.9.3477-3482.2004
Sahly, H., Navon-Venezia, S., Roesler, L., Hay, A., Carmeli, Y., Podschun, R., et al. (2008). Extended-spectrum beta-lactamase production is associated with an increase in cell invasion and expression of fimbrial adhesins in Klebsiella pneumoniae. Antimicrob. Agents Chemother. 52, 3029–3034. doi: 10.1128/aac.00010-08
Samaha-Kfoury, J. N., and Araj, G. F. (2003). Recent developments in β lactamases and extended spectrum βlactamases. Br. Med. J. 327, 1209–1213. doi: 10.1136/bmj.327.7425.1209
Schembri, M. A., Blom, J., Krogfelt, K. A., and Klemm, P. (2005). Capsule and fimbria interaction in Klebsiella pneumoniae. Infect. Immun. 73, 4626–4633. doi: 10.1128/IAI.73.8.4626-4633.2005
Schroll, C., Barken, K. B., Krogfelt, K. A., and Struve, C. (2010). Role of type 1 and type 3 fimbriae in Klebsiella pneumoniae biofilm formation. BMC Microbiol. 10:179. doi: 10.1186/1471-2180-10-179
Seibert, G., Hörner, R., Meneghetti, B. H., Righi, R. A., Dal Forno, N. L., and Salla, A. (2014). Nosocomial infections by Klebsiella pneumoniae carbapenemase producing enterobacteria in a teaching hospital. Einstein 12, 282–286. doi: 10.1590/s1679-45082014ao3131
Seki, L. M., Pereira, P. S., de Souza, M. P., Conceição, M. S., Marques, E. A., and Porto, C. O. (2011). Molecular epidemiology of KPC-2- producing Klebsiella pneumoniae isolates in Brazil: the predominance of sequence type 437. Diagn. Microbiol. Infect. Dis. 70, 274–277. doi: 10.1016/j.diagmicrobio.2011.01.006
Shah, R. K., Ni, Z. H., Sun, X. Y., Wang, G. Q., and Li, F. (2017). The determination and correlation of various virulence genes, ESBL, serum bactericidal effect and biofilm formation of clinical isolated classical Klebsiella pneumoniae and Hypervirulent Klebsiella pneumoniae from respiratory tract infected patients. Pol. J. Microbiol. 66, 501–508. doi: 10.5604/01.3001.0010.7042
Shon, A. S., Bajwa, R. P., and Russo, T. A. (2013). Hypervirulent (hypermucoviscous) Klebsiella pneumoniae: a new and dangerous breed. Virulence 4, 107–118. doi: 10.4161/viru.22718
Siu, L. K., Fung, C., Chang, F., Lee, N., Yeh, K., Koh, T. H., et al. (2011). Molecular typing and virulence analysis of serotype K1 Klebsiella Pneumoniae strains isolated from liver abscess patients and stool samples from noninfectious subjects in Hong Kong, Singapore, and Taiwan. J. Clin. Microbiol. 49, 3761–3765. doi: 10.1128/JCM.00977-11
Siu, L. K., Lin, J. C., Gomez, E., Eng, R., and Chiang, T. (2012). Virulence and plasmid transferability of KPC Klebsiella pneumoniae at the Veterans Affairs Healthcare System of New Jersey. Microb. Drug Resist. 18, 380–384. doi: 10.1089/mdr.2011.0241
Skurnik, D., Lasocki, S., Bremont, S., Muller-Serieys, C., Kitzis, M. D., and Courvalin, P. (2010). Development of ertapenem resistance in a patient with mediastinitis caused by Klebsiella pneumoniae producing an extended-spectrum β-lactamase. J. Med. Microbiol. 59, 115–119. doi: 10.1099/jmm.0.012468-0
Stahlhut, S. G., Struve, C., Krogfelt, K. A., and Reisner, A. (2012). Biofilm formation of Klebsiella pneumoniae on urethral catheters requires either type 1 or type 3 fimbriae. FEMS Immunol. Med. Microbiol. 65, 350–359. doi: 10.1111/j.1574-695X.2012.00965.x
Stahlhut, S. G., Tchesnokova, V., Struve, C., Weissman, S. J., Chattopadhyay, S., Yakovenko, O., et al. (2009). Comparative structure-function analysis of mannose-specific FimH adhesins from Klebsiella pneumoniae and Escherichia coli. J. Bacteriol. 191, 6592–6601. doi: 10.1128/JB.00786-09
Struve, C., Bojer, M., and Krogfelt, K. A. (2008). Characterization of Klebsiella pneumoniae type 1 fimbriae by detection of phase variation during colonization and infection and impact on virulence. Infect. Immun. 76, 4055–4065. doi: 10.1128/IAI.00494-08
Struve, C., Bojer, M., and Krogfelt, K. A. (2009). Identification of a conserved chromosomal region encoding Klebsiella pneumoniae type 1 and type 3 fimbriae and assessment of the role of fimbriae in pathogenicity. Infect. Immun. 77, 5016–5024. doi: 10.1128/IAI.00585-09
Struve, C., and Krogfelt, K. A. (2004). Pathogenic potential of environmental Klebsiella pneumoniae isolates. Environ. Microbiol. 6, 584–589. doi: 10.1111/j.1462-2920.2004.00590.x
Tarkkanen, A. M., Virkola, R., Clegg, S., and Korhonen, T. K. (1997). Binding of the type 3 fimbriae of Klebsiella pneumoniae to human endothelial and urinary bladder cells. Infect. Immun. 65, 1546–1549.
Tsai, Y. K., Fung, C. P., Lin, J. C., Chen, J. H., Chang, F. Y., Chen, T. L., et al. (2011). Klebsiella pneumoniae outer membrane porins OmpK35 and OmpK36 play roles in both antimicrobial resistance and virulence. Antimicrob. Agents Chemother. 55, 1485–1493. doi: 10.1128/AAC.01275-10
Ur Rahman, S., Ali, T., Ali, I., Khan, N. A., Han, B., and Gao, J. (2018). The growing genetic and functional diversity of extended spectrum beta-lactamases. Biomed. Res. Int. 26:9519718. doi: 10.1155/2018/9519718
Uz Zaman, T., Aldrees, M., Al Johani, S. M., Alrodayyan, M., and Aldughashem, F. A. (1994). Genomic fingerprinting of bacteria using repetitive sequence-based polymerase chain reaction. Methods Mol. Cell. Biol. 5, 25–40. doi: 10.1128/JCM.43.1.199-207.2005
Versalovic, J., Schneider, M., De Bruijn, F. J., and Lupski, J. R. (1994). Genomic fingerprinting of bacteria using repetitive sequence-based polymerase chain reaction Meth. Mol. Cell. Biol. 5, 25–40. doi: 10.1128/JCM.43.1.199-207.2005
Vila, A., Cassata, A., Pagella, H., Amadio, C., Yeh, K.-M., Chang, F.-Y., et al. (2011). Appearance of Klebsiella Pneumoniae liver abscess syndrome in argentina: case report and review of molecular mechanisms of pathogenesis. Open Microbiol. J. 5, 107–113. doi: 10.2174/1874285801105010107
Vila, J. (2015). Multidrug-resistant bacteria without borders: role of international trips in the spread of multidrug-resistant bacteria. J. Travel Med. 22, 289–291. doi: 10.1111/jtm.12231
Wang, X., Chen, H., Zhang, Y., Wang, Q., Zhao, C., Li, H., et al. (2015). Genetic characterization of clinical Klebsiella pneumoniae isolates with reduced susceptibility to tigecycline: role of the global regulator RamA and its local repressor RamR. Int. J. Antimicrob. Agents 45, 635–640. doi: 10.1016/j.ijantimicag.2014.12.022
Wang, X. D., Cai, J. C., Zhou, H. W., Zhang, R., and Chen, G.-X. (2009). Reduced susceptibility to carbapenems in Klebsiella pneumoniae clinical isolates associated with plasmid-mediated β-lactamase production and OmpK36 porin deficiency. J. Med. Microbiol. 58, 1196–1202. doi: 10.1099/jmm.0.008094-0
Wasfi, R., Elkhatib, W. F., and Ashour, H. M. (2016). Molecular typing and virulence analysis of multidrug resistant Klebsiella pneumoniae clinical isolates recovered from Egyptianhospitals. Sci. Rep. 6:38929. doi: 10.1038/srep38929
Wilke, M. S., Lovering, A. L., and Strynadka, N. C. J. (2005). Beta-lactam antibiotic resistance: a current structural perspective. Curr. Opin. Microbiol. 8, 525–533. doi: 10.1016/j.mib.2005.08.016
Wiuff, C., Madsen, M., Baggesen, D. L., and Aarestrup, F. M. (2000). Quinolone resistance among Salmonella enterica from cattle, broilers, and swine in Denmark. Microb. Drug Resist. 6, 11–17. doi: 10.1089/mdr.2000.6.11
World Health Organization [WHO] (2014). Antimicrobial Resistance: Global Report on Surveillance. Geneva: World Health Organization. Available at: http://www.who.int/drugresistance/documents/surveillancereport/en/
Würker, M., Beuth, J., Ko, H. L., Przondo-Mordarska, A., and Pulverer, G. (1990). Type of fimbriation determines adherence of Klebsiella bacteria to human epithelial cells. Zentralbl. Bakteriol. 274, 239–245. doi: 10.1016/S0934-8840(11)80106-4
Xiong, Z., Li, T., Xu, Y., and Li, J. (2007). Detection of CTX-M-14 extended-spectrum β-lactamase in Shigella sonnei isolates from China. J. Infect. 55:e125-8. doi: 10.1016/j.jinf.2007.07.017
Xiong, Z., Zhu, D., Wang, F., Zhang, Y., Okamoto, R., and Inoue, M. (2004). A Klebsiella pneumoniae producing three kinds of class A β-lactamases encoded by one single plasmid isolated from a patient in Huashan Hospital, Shanghai, China. Int. J. Antimicrob. Agents 23, 262–277. doi: 10.1016/j.ijantimicag.2003.07.011
Yan, B. C., Westfall, B. A., and Orlean, P. (2001). Ynl038wp (Gpi15p) is the Saccharomyces cerevisiae homologue of human Pig-Hp and participates in the first step in glycosylphosphatidylinositol assembly. Yeast 18, 1383–1389. doi: 10.1002/yea.783
Yang, J., Ye, L., Guo, L., Zhao, Q., Chen, R., Luo, Y., et al. (2013). A nosocomial outbreak of KPC-2-producing Klebsiella pneumoniae in a Chinese hospital: dissemination of ST11 and emergence of ST37, ST392 and ST395. Clin. Microbiol. Infect. 19, 509–515. doi: 10.1111/1469-0691
Yeh, K. M., Kurup, A., Siu, L. K., Koh, Y. L., Fung, C. P., Lin, J. C., et al. (2007). Capsular serotype K1 or K2, rather than magA and rmpA, is a major virulence determinant for Klebsiella pneumoniae liver abscess in Singapore and Taiwan. J. Clin. Microbiol. 45, 466–471. doi: 10.1128/JCM.01150-06
Yuhan, Y., Ziyun, Y., Yongbo, Z., Fuqiang, L., and Qinghua, Z. (2016). Over expression of AdeABC and AcrAB-TolC efflux systems confers tigecycline resistance in clinical isolates of Acinetobacter baumannii and Klebsiella pneumoniae. Rev. Soc. Bras. Med. Trop. 49, 165–171. doi: 10.1590/0037-8682-0411
Zhang, R., Lin, D., Chan, E. W., Gu, D., Chen, G. X., and Chen, S. (2016). Emergence of carbapenem-resistant serotype K1 hypervirulent Klebsiella pneumoniae (hvKP) strains in China. Antimicrob. Agents Chemother. 60, 709–711. doi: 10.1128/AAC.02173-15
Zhang, Y., Zhao, C., Wang, Q., Wang, X., Chen, H., Li, H., et al. (2016). High Prevalence of Hypervirulent Klebsiella pneumoniae Infection in China: geographic distribution, clinical characteristics, and antimicrobial resistance. Antimicrob. Agents Chemother. 60, 6115–6120. doi: 10.1128/AAC.01127-16
Keywords: Klebsiella pneumoniae, intensive care units, multi-drug resistance, β-lactams gene, virulence genes
Citation: Ferreira RL, da Silva BCM, Rezende GS, Nakamura-Silva R, Pitondo-Silva A, Campanini EB, Brito MCA, da Silva EML, Freire CCM, Cunha AF and Pranchevicius MC (2019) High Prevalence of Multidrug-Resistant Klebsiella pneumoniae Harboring Several Virulence and β-Lactamase Encoding Genes in a Brazilian Intensive Care Unit. Front. Microbiol. 9:3198. doi: 10.3389/fmicb.2018.03198
Received: 14 July 2018; Accepted: 10 December 2018;
Published: 22 January 2019.
Edited by:
Gilberto Igrejas, University of Trás-os-Montes and Alto Douro, PortugalReviewed by:
Jayapradha R., SASTRA University, IndiaJianmin Zhang, South China Agricultural University, China
Copyright © 2019 Ferreira, da Silva, Rezende, Nakamura-Silva, Pitondo-Silva, Campanini, Brito, da Silva, Freire, Cunha and Pranchevicius. This is an open-access article distributed under the terms of the Creative Commons Attribution License (CC BY). The use, distribution or reproduction in other forums is permitted, provided the original author(s) and the copyright owner(s) are credited and that the original publication in this journal is cited, in accordance with accepted academic practice. No use, distribution or reproduction is permitted which does not comply with these terms.
*Correspondence: Maria-Cristina da Silva Pranchevicius, bWNzcHJhbmNAZ21haWwuY29t
†These authors have contributed equally to this work