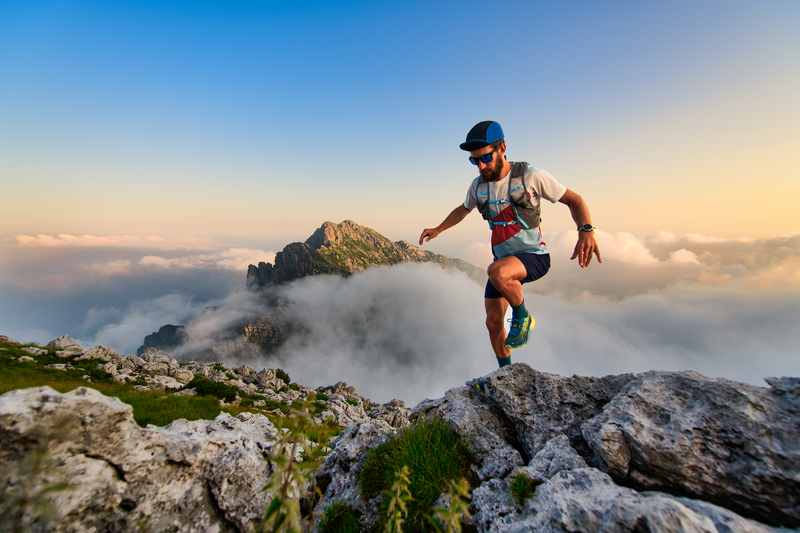
95% of researchers rate our articles as excellent or good
Learn more about the work of our research integrity team to safeguard the quality of each article we publish.
Find out more
ORIGINAL RESEARCH article
Front. Microbiol. , 21 December 2018
Sec. Terrestrial Microbiology
Volume 9 - 2018 | https://doi.org/10.3389/fmicb.2018.03197
This article is part of the Research Topic Landscape and Ecosystem Microbiology View all 10 articles
Application of swine manure to agricultural land allows recycling of plant nutrients, but excess nitrate, phosphorus and fecal bacteria impact surface and drainage water quality. While agronomic and water quality impacts are well studied, little is known about the impact of swine manure slurry on soil microbial communities. We applied swine manure to intact soil columns collected from plots maintained under chisel plow or no-till with corn and soybean rotation. Targeted 16S-rRNA gene sequencing was used to characterize and to identify shifts in bacterial communities in soil over 108 days after swine manure application. In addition, six simulated rainfalls were applied during this time. Drainage water from the columns and surface soil were sampled, and DNA was extracted and sequenced. Unique DNA sequences (OTU) associated with 12 orders of bacteria were responsible for the majority of OTUs stimulated by manure application. Proteobacteria were most prevalent, followed by Bacteroidetes, Firmicutes, Actinobacteria, and Spirochaetes. While the majority of the 12 orders decreased after day 59, relative abundances of genes associated with Rhizobiales and Actinomycetales in soil increased. Bacterial orders which were stimulated by manure application in soil had varied responses in drainage waters over the course of the experiment. We also identified a “manure-specific core” of five genera who comprised 13% of the manure community and were not significantly abundant in non-manured control soils. Of these five genera, Clostridium sensu stricto was the only genus which did not return to pre-manure relative abundance in soil by day 108. Our results show that enrichment responses after manure amendment could result from displacement of native soil bacteria by manure-borne bacteria during the application process or growth of native bacteria using manure-derived available nutrients.
Rising demands for pork products in developing countries has led to increased production in the United States (Narrod et al., 2011). In 2018, pork production in the United States is estimated to reach 27 billion pounds, increasing 2017 totals by 5% (Economic Research Service [ERS], and United States Department of Agriculture [USDA] et al., 2018). Increasing demands, coupled with production shifting toward confined animal feeding operations (CAFOs), has resulted in increased availability of manure as well as needs for its disposal (Choudhary et al., 1996). Because swine manure contains valuable nutrients, it is often applied to agricultural land as an alternative to inorganic fertilizer. Previous work has documented both benefits and risks of manure application for soil health. Among benefits, swine manure application has been observed to increase aggregate stability, bacterial diversity and soil microbial biomass and activity following application (Larkin et al., 2006; Hammesfahr et al., 2008, 2011; Zhang et al., 2012; Adesanya et al., 2016). The addition of organic nutrients through manure amendments also introduces foreign microbial communities, which could be of concern to humans, e.g., fecal-borne pathogens, to surrounding soils (Ziemer et al., 2010; Gordoncillo et al., 2013; Haack et al., 2015; Givens et al., 2016). With rainfall, manure-associated nutrients and microbial communities also are transported to downstream waters (Sharpley and Withers, 1994; Hoang et al., 2013; Luby et al., 2016).
Currently, little is understood about manure microbial communities and their response in soils after manure application. Past studies have identified significant differences between the microbial community structures of agricultural soils and manure microbiomes. Depending on location and management practices, major phyla in agricultural soils largely consist of varying combinations of Proteobacteria, Verrucomicrobia, Acidobacteria, Chloroflexi, and Actinobacteria (Trivedi et al., 2016). Conversely, Firmicutes and Bacteroidetes are the primary constituents in swine manure microbiomes (Looft et al., 2012; Lu et al., 2014; Kumari et al., 2015; Pajarillo et al., 2015). Because of the observed contrast between manure-associated and native soil microbial communities, there is a need to identify and track manure-amended microbial impacts in agricultural environments. Short-term increases in manure-associated species or operational taxonomic units (OTUs) closely related to Proteobacteria, Bacteroidetes, and Chloroflexi have been observed following manure amendments to soils (Ding et al., 2014; Riber et al., 2014; Leclercq et al., 2016; Liu et al., 2017). These previous inquiries provide information regarding manure’s impact on existing soil microbial communities, but lack the ability to track changes in drainage waters associated with agricultural soils.
The impacts of manure amendment to surrounding environments in United States’ Upper Midwestern agriculture, (Illinois, Iowa, Minnesota, and Wisconsin), is further amplified by the presence of artificial subsurface drainage, which can accelerate the movement of nutrients and microorganisms. Approximately one third of Iowa cropland contains subsurface drainage to artificially lower the water table, allowing access to fields and aerated soils for crop growth (Zucker and Brown, 1998). Previous studies have shown that tile drainage provides a pathway that can expedite the movement of nutrients, herbicides, pesticides, and bacteria into water (Buhler et al., 1993; Flury, 1996; Jaynes et al., 2001; Rieke et al., 2018a). However, less in known regarding the impact of manure’s microbial community on existing agricultural soil microbial communities and communities in associated subsurface drainage.
To specifically track manure-associated impacts on soils and drainage waters, we constructed soil columns to simulate the field environment. Precisely, soil columns were amended with manure and treated with simulated rainfall to assess the temporal variation in manure-associated and endemic soil microbial communities in the soil and drainage water. Using phylogenetic marker sequencing, we identified manure-derived operational taxonomic units (MDOs) and manure-stimulated operational taxonomic units (MSOs). We hypothesized manure addition (i.e., MDOs) to the soil may introduce and/or sustain manure associated microbial communities or promote growth of native soil microbes in soil and drainage waters (i.e., MSOs). The objectives of this study were to identify specific MDOs that are not abundant in soils and track the fate of MDOs and MSOs in soils and drainage waters.
Soil columns under four different management practices were collected on November 6, 2015 from plots at Iowa State’s Northeast Research and Demonstration Farm, near Nashua, IA, United States (43.0° N, 92.5° W). All plots where columns were sampled were maintained in corn-soybean rotations, with nitrogen application prior to the corn growing season. Nitrogen was applied either in the form of swine manure or urea ammonium nitrate (UAN) and plots were maintained under chisel plow or no-till managements, creating four different overall management practices: manured no-till, non-manured no-till, manured chisel plow, and non-manured chisel plow. Soils at the farm are Mollisols consisting of: moderately well to poorly drained Kenyon silty–clay loam (fine-loamy, mixed, super active, mesic Typic Hapludolls), Floyd loam (fine-loamy, mixed, super active, mesic aquic Pachic Hapludolls), and Readlyn loam (fine-loamy, mixed, superactive, mesic Aquic Hapludolls) over glacial till, with 1–3% slopes (Farthelrahman et al., 2011). Manure was last injected as 10–15 cm deep bands on November 6, 2014. Manure had not been applied to control (non-manured) plots since 1978, while application rates to plots receiving manure have varied since 1993. Chisel plow tillage and no-till management were chosen due to their prevalence in Iowa agriculture. Agricultural fields under no-till management form preferential flow paths, or macropores, which may enhance leaching of bacteria (Jamieson et al., 2002). Chisel plow tillage disrupts macropores and aerates soil, creating different transport pathways for constituents through soil profiles.
Thirty-six PVC pipes (15.24 cm inner diameter, 60.96 cm long) were gently pushed into the soil and pulled out using a Giddings soil probe to obtain minimally disturbed soil columns from the plots described previously (Supplementary Figure 1). Soil depths within the column ranged from 46 to 51 cm. Foam blocks were taped to the bottom of columns for transport back to Iowa State University. Column bottoms were fitted with 15.24 cm diameter PVC pipe end caps. Prior to attachment, a single hole was drilled in the middle of the end cap to insert tubing for drainage collection (Supplementary Figure 2). Caps were then filled with ASTM 20-30 Test Sand (Humboldt Mfg Co, Elgin, IL, United States) in order to create a sand–soil interface when attached. Fiberglass mesh, with 1.4 mm square sizing, was attached to the inside of caps over the drilled hole to prevent sand loss. Prior to construction (161 days following extraction) soil columns were periodically treated with 200 mL of deionized water to maintain soil moisture as described below.
The manure for this study was obtained from a commercial finishing swine facility, near the Iowa State Northeast Research Farm. The facility maintains 2.5 animal rotations per year, with manure pumped annually from a storage pit located below the animal housing. Manure samples were collected directly from the injector on November 3, 2015. Samples were stored on ice while being transported back to Iowa State University. Samples were stored at 4°C in 19 L buckets until applying on soil columns. The manure contained a moisture content of 92% and a pH of 7.5. Dry matter within the manure was comprised of 8% total nitrogen, 38% carbon, and 6% phosphorus.
We simulated manure injection zones in manure-treated columns by removing a 10.2 cm diameter, 15.2 cm deep section of soil from the center of each column on day 0 of the experiment (Supplementary Figure 2). The displaced soil was homogenized by hand and subsampled in 10–20 g aliquots. The tops of non-manured control columns were also sampled at the same time by scraping off 10–20 g off the top of the column, rather than removing a 15.2 cm deep section and frozen at -20°C. Manured columns were treated with 750 mL of liquid swine manure to approximate a 7.8 cm wide, 15.24 cm deep manure injection band based on volume. After manure application, excavated soil was replaced in manured columns to obtain pre-application soil depths. Integration of excavated soil was implemented to mimic the soil–manure interface observed in field, following knifing of soil and manure injection. Manure subsamples were frozen at this at time at -20°C for DNA extractions. Soil columns were maintained in a vertical position in a constructed rack at room temperature.
Simulated rainfall events were conducted at 10, 24, 35, 59, 80, and 108 days after manure application (DAM). Single column rainfall simulators were constructed by drilling six holes in 15.24 cm diameter PVC pipe end caps to hold 20 G × 1½ in. hypodermic needles (BD Franklin Lakes, NJ, United States). One hole was drilled in the center of the cap, with the remaining five holes drilled in a circular pattern with a 5.1 cm radius from the central hole. Simulators were placed on top of each column and filled with 1 L of deionized (DI) water. Leachate was collected in 1 L sterile Nalgene bottles located on racks below the columns and processed 48 h after the beginning of each event. In order to maintain soil moisture contents representative of field conditions columns received additional wettings in between rainfall events using the individual rainfall applicators. A 1-L pan of DI water, with a 15.2 cm diameter, was placed on the top of the column rack during the experiment. At 48 h prior to rainfall events the amount of water evaporated from the pan was recorded. DI water was added at a rate of amount evaporated plus an additional 100 mL to each column in order to conservatively approximate field capacity at the time of each rainfall event. After measurement of DI volume evaporated from the pan, the pan was emptied and filled with a fresh 1 L of DI water. Additionally, this process was repeated once a week throughout the experiment during weeks without simulated rain events. Three chisel plow and three no-till columns were sacrificed for sampling 48 h after rainfall events conducted 24, 59, and 108 DAM. The top 15 cm of each column were extracted separately and mixed by hand due to moist soil conditions and high concentrations of organic matter in soils amended with manure. The homogenized soils were subsampled into 10–20 g aliquots and frozen at -20°C. Composite no-till and chisel plow soil samples from plots with a history of manure application collected on days 0, 24, 108 were analyzed for total nitrogen, total carbon, pH, and texture.
Column effluent was filtered through 0.22 μm sterile filters and frozen at -20°C until DNA extractions were performed using Mo Bio Power Water DNA kits. Three 50 mL aliquots were filtered from manure treated effluent from columns which were associated with the following column sacrifice. In total, 18 50 mL aliquots were collected from each rainfall event, creating an overall sum of 108 effluent samples. Soil DNA was extracted using PowerSoil 96 Well Soil DNA Isolation kits (Qiagen). Soil aliquots for each well were weighed and recorded. The target weight for each soil aliquot was 0.25 g. Two soil aliquots were used for DNA extraction from each of the 36 columns on day 0 of the experiment, prior to manure application. Additionally, two soil subsamples were collected for DNA extractions from manured treated soil columns which were destructed. In total, soil DNA extraction was performed on 180 soil samples. DNA samples above 10 μg mL-1 were diluted to 10 μg mL-1 to help negate potential effects of inhibitors and non-specific binding of primers. The V4 region of bacterial 16S rRNA gene using F515/R806 primers was amplified using methods previously described (Kozich et al., 2013). Sequencing of bacterial amplicons was performed on Illumina Miseq with Miseq Reagent Kit v2. Sequences were deposited in the NCBI SRA with the following accession number: PRJNA506065 (Supplementary Table 4).
Ribosomal Database Project (RDP) Paired-end Reads Assembler (Cole et al., 2014) was applied to assemble 150 base paired-end bacterial 16S rRNA gene sequences. Minimal and maximum assembled lengths were 250 bases (l–250) and 280 bases (-L 280). Assembled sequences with expected maximum error adjusted Q scores less than 25 over the whole sequence were removed. Chimeras de novo were removed with Usearch (8.1 64bit) (Edgar et al., 2011). RDP’s 16S rRNA gene training set sequences (No15) was used to eliminate chimeras of known reference genes. Resulting sequences were clustered at 97% sequence similarity by CD-HIT (4.6.1) (Fu et al., 2012), and abundances in each sample were estimated. CD-HIT was chosen due to its speed and demonstrated production of clusters which were highly similar to true numbers of OTUs derived from simulated complex data (Bonder et al., 2012; Chen et al., 2013). OTU sequences were assigned taxonomic information using RDP classifier (Wang et al., 2007) with a confidence cutoff at 50% (-c 0.5). OTUs witnessed less than five times were removed from the dataset. Additionally, manure, soil and drainage samples containing less than 6,000 sequences were removed, which resulted in 162 samples used in the experiment. Remaining samples were rarified to 6,076 sequences using the function rarify_even_depth in the R package phyloseq (McMurdie and Holmes, 2013).
Permutational multivariate analysis of variance using distance matrices was performed with the adonis function found in the R package vegan (Dixon, 2009). Differential expression analysis based on the negative binomial distribution (Gamma-Poisson) was completed using the function Deseq from the R package DESeq2 (Love et al., 2014) to identify OTUs whose abundances were significantly different based on selected treatments. Non-metric multidimensional scaling (NMDS) was performed using the ordinate command in Phyloseq (McMurdie and Holmes, 2013) with Bray–Curtis distances. Alpha diversity was calculated using the Shannon diversity index resulting from the estimate_diversity command in Phyloseq. Code for analysis is located at https://github.com/lizrieke/Nashua-Manure-Column-Study.
Our experiment resulted in a total of 3 manure, 117 soil, and 69 water samples from a total of 36 columns over 108 days sampling period. Analysis was performed to characterize physical and chemical changes following manure application (Table 1). Both total nitrogen and to carbon increased following manure application. Total carbon and nitrogen percentages remained elevated for the remaining portion of the environment. Conversely, soil pH increased following manure application, but returned to pre-manure application values by the end of the experiment. Soil texture remained largely unchanged over the course of the study.
Table 1. Manured soil total nitrogen, total carbon, pH, and texture of no-till and chisel plow 15 cm composite samples.
For each sample, the microbial community structure was determined from amplicon sequencing of the 16S rRNA gene. We observed significant differences (p < 0.05) in both soil and water microbial communities from control (no-manure amendments) and manure amended columns using the Adonis function in vegan (v. 2.5-3) (Oksanen et al., 2013). Overall, variation in soil and water community structures were driven by two main factors, sample matrix and the number of DAM. Permutational multivariate analysis results identified 40% of variation in effluent community structure in manured columns was derived from DAM, while DAM attributed to 56% of variation in manured soil community structures. This analysis was run separately by matrix due to the experiment’s unbalanced sampling design (four soil sampling time points and six water sampling time points). Permutational multivariate analysis was run on a subset of water and soil samples (24, 59, and 108 DAM) to ensure a balanced sampling design. Results indicated 22% of variation in soil and effluent microbial community structure was attributed to sample matrix. NMDS identified similar groupings of microbial communities based on matrix (manure, soil or drainage water) and DAM (Figure 1). Although tillage history was associated with significant differences in community structure, only 2% of variation was explained by this factor and therefore no-till and chisel plow treatment were combined for the remaining analysis.
Figure 1. Non-metric multidimensional scaling (NMDS) of the 16S-rRNA gene sequences from manure, manure applied to soil, and water draining from manure-treated soil. K = 2, stress value = 0.1180937 using Bray–Curtis distances. Day 0 soil samples were collected prior to manure application. Dashed ellipses represent 95% confidence intervals around soil and water group centroids by sampling day. Manure samples are colored gray.
The major phyla comprising manure and soil microbial communities prior to manure amendment were characterized. Overall, manure was comprised primarily of Firmicutes, contributing to 64% of total relative abundances of the total microbial community (Figure 2). The remaining 36% of OTUs were associated with Acidobacteria, Euryarchaeota, Proteobacteria, Actinobacteria, Verrucomicrobia, and Bacteroidetes. Unamended soil microbial communities (DAM 0) relative to manure communities were more diverse, comprised mainly of Acidobacteria, Actinobacteria, Proteobacteria, and Verrucomicrobia. Unamended soil microbial communities contained an average a Shannon diversity index of 6.1 ± 0.21, compared to an average of 4.9 ± 0.141 in manure. After manure amendment, we observed various shifts in the relative abundances of multiple phyla in soil. Acidobacteria decreased from 31% of total soil bacteria before manure application to 4% at 108 DAM. Verrucomicrobia also decreased from 12% before manure application to 5% 108 DAM (Supplementary Table 1). Acidobacteria relative abundances in non-manured control soil columns increased slightly from 26 to 29% 108 days into the experiment. Additionally, Verrucomicrobia relative abundances increased in non-manured control columns from 8 to 11% by the end of the experiment. OTUs classified within Proteobacteria were enriched after manure application, rising from 12 to 35% 108 DAM, while relative abundances in non-manured control soils decreased from 18 to 12%. Firmicutes also increased from <1% of total bacteria in soil prior to manure application to 10% 108 DAM. Bacteroidetes increased after manure application from 2% before manure application to 28% 59 DAM, but subsequently decreased to 14% by 108 DAM. Firmicutes and Bacteroidetes relative abundances remained below 3% in non-manured control soils over the course of the experiment.
Figure 2. Relative abundance of sequences matching major phyla in manure, soil treated with manure and leachate water. Day 0 soil samples were collected prior to manure application from plots with a history of manure application. The other phyla grouping represent OTUs whose sequences matched known phyla, while the unclassified bacteria grouping represents relative abundances of OTUs which did not match known phyla.
Similar shifts as observed in soils were also observed in drainage water over the course of the experiment. Enrichment of Actinobacteria, Bacteroidetes, Firmicutes, Proteobacteria, and Verrucomicrobia were observed between samples taken 10 DAM and 24 DAM. While Actinobacteria, Proteobacteria, and Firmicutes relative abundances were greatest in soil at 108 DAM, their proportions in drainage began to decrease by 59 DAM. An exception to similar trends between soil and waters was increase of Verrucomicrobia proportions over time in waters which decreased in soils.
To further understand the impact of manure additions on soil microbial communities, we extended our comparison of differences of observed phyla (Figure 2 and Supplementary Tables 1, 2) to the distribution of species as defined by OTUs. Our approach to assessing the impact of manure additions consisted of two methods. Firstly, we identified the OTUs which were enriched due to manure amendments (MSOs), or significantly greater in abundance (p < 0.05) compared to pre-manured soils (manure last applied 525 days prior) using rarified sample reads in Deseq2 (v. 3.8) (Love et al., 2014). Next, among these MSOs, we identified the MDOs, or the OTUs which originally were associated with manure (greater than 13%) and detected at only low abundance in soils [less than 2.8 × 10-5%]. Consequently, in the context of this study, the MDOs are a subset of MSOs which have a high probability of originating from the manure amendment.
In total, we identified the enrichment of 250 MSOs (Supplementary Table 3). In manure, the MSOs comprised 64% of the microbial community and in soils, these MSOs were present at low relative abundances and comprised 3.8% of soil microbial communities. After manure amendment, these MSOs comprised 61% of the total microbial community (average) in soil 24 DAM and 68% in soil 59 DAM (Figure 3). By 108 DAM, the MSOs decreased in manure-amended soils to 37%, though relative abundances were still elevated when compared to their presence in pre-manure application samples (0 DAM).
Figure 3. Average relative abundances of MSOs in soil and water. OTUs were classified as manure stimulated if abundances were significantly greater (p < 0.05) in soil 24 DAM when compared to pre-manure application soil samples.
Overall trends in the MSO relative abundances in drainage varied slightly from those identified in corresponding soil samples. The MSOs averaged 58% of OTUs in artificial drainage 24 DAM (Figure 3). However, unlike in soils, average relative abundance of the MSOs decreased in drainage collected 59 DAM to 40%. Additionally, the set of MSOs sharply decreased in drainage to 11% on average of by 108 DAM application. Overall, in both soil and drainage, MSOs were observed to persist throughout this experiment.
The majority of the identified MSOs were most closely similar to sequences belonging to 12 different orders of bacteria (Figure 4). MSOs belonging to Proteobacteria were most prevalent, followed by Bacteroidetes, Firmicutes, Actinobacteria, and Spirochaetes. Relative abundances of sequences matching nine of the 12 orders began to decrease by day 108. Burkholderiales, Caulobacterales, Clostridiales, Flavobacteriales, Pseudomonadales, and Sphingomonadales continuously decreased in manure treated soil over the course of the experiment. However, Bacteroidales, Spirochaetales and Xanthomonadales reached their maximum relative abundances in manure treated soil 59 DAM before decreasing in samples taken 108 DAM. Relative abundances of the remaining three orders continued to increase in manured soil samples 109 DAM. Although the relative abundances of certain manure stimulated orders in soil remained above proportions identified in pre-manured soil samples by 108 DAM, relative abundances of all 12 orders in drainage decreased by 108 DAM or remained consistently negligible over the course of the experiment.
Figure 4. Relative abundances of bacterial OTUs in soil and water which significantly increased in soil after manure application grouped at the order level. Error bars represent standard deviations of mean soil and water relative abundances. Significant differences were determined using the function Deseq2. Day 0 soil samples were collected prior to manure application.
The 12 orders which represented the majority of MSOs in soil also displayed varied temporal responses in drainage waters (Figure 4). Similar trends in soil and drainage water relative abundances over time were recognized in Burkholderiales, Caulobacterales, Clostridiales, Flavobacteriales, and Sphingomonadales. Trends in relative abundances of Actinomycetales, Rhizobiales, Sphingobacteriales, and Xanthomonadales in drainage water differed from those identified in soil. Relative abundances of these four orders were initially greater in drainage than soil, but decreased by 108 DAM, while in soil their proportions continued to increase. Although, Bacteroidales, Pseudomonadales, and Spirochaetales relative abundances increased in soil following manure application, very few sequences matching these orders were identified in drainage. Variable physical and chemical properties within these 12 orders of bacteria were likely responsible for differential attenuation and transport patterns over the course of our experiment.
In order to identify and track bacterial populations directly introduced into soil and drainage through manure application, we next identified MDOs, which were considered a set of core bacteria specific to the swine manure and not abundant in soils. MDOs were specifically required to be identified at least 25 times in each manure sample and contained significantly greater abundance in manure compared to soil which had not received a manure application in over 30 years (p < 0.05). Application of these criteria resulted in five MDOs representative of: Clostridium sensu stricto, Methanobrevibacter, Phascolarctobacterium, Romboutsia, and Tissierella (Figure 5). Four of the MDOs fall within the phylum Firmicutes, while Methanobrevibacter is classified within the Euryarchaeota phylum. In manure samples, the five genera represent 13% of the total microbial community.
Figure 5. Relative abundances of manure-specific genera (MDO) in soil and drainage which significantly increased in soil after manure application. Error bars represent standard deviations of mean soil and water relative abundances. Day 0 soil samples were collected prior to manure application.
Relative abundance of the MDOs all began to decrease in soil samples 24 DAM, except Phascolarctobacterium, whose relative abundance peaked at 59 DAM. Additionally, all MDOs except for Clostridium sensu stricto returned close to pre-manure application relative abundances in soil at 108 DAM. Relative abundances of Clostridium sensu stricto, Methanobrevibacter, and Romboutsia steadily decreased in drainage samples over the course of the experiment. Conversely, Tissierella and Phascolarctobacterium were rarely identified in drainage samples. Although relative abundances of Clostridium sensu stricto were noticeably lower in drainage water samples when compared to soil at 108 DAM, sequences matching this genus were present in water at this time.
The use of soil columns in our experiment allowed us to directly assess the impacts of manure amendment on microbial communities in soil and drainage waters. Over the course of the 108-day experiment, we observed initial shifts (24 DAM) in microbial communities in soils and waters which subsequently decreased or returned to pre-manure application proportions (108 DAM). The enrichment of bacteria after manure amendments could be the direct result of manure amendment through the displacement of native soil bacteria by manure-borne bacteria or enrichment of native bacteria responding to manure available nutrients. Consequently, we defined two groups representative of manure associated communities based on their enrichment in soil and water samples (MSOs) and their likely origination from manure (MDOs).
Among MSOs, we observed short term increases in Burkholderiales, Clostridales, Pseudomonadales, and Xanthomonadales following manure incorporation, and these results are consistent with previous studies identifying these bacterial orders responding to manure amendment (Ding et al., 2014; Riber et al., 2014). Additionally, we observed that Actinomycetales, Rhizobiales, and Sphingobacteriales were also consistently enriched in manure-amended soils over the course of our experiment. Actinomycetales have also been previously identified as a dominant order associated with manure-based pyrogenic organic matter (Dai et al., 2017). Elevated levels of families within the order Actinomycetales have additionally been observed to remain 130 days following incorporation of dairy cow manure to soils, though in the same study, Rhizobiales associated families began to decrease prior to the end of the experiment (Udikovic-Kolic et al., 2014). These differences may be attributed to variations in interactions of native soil communities to the manure type and management histories of both the soils and the animals.
The MDOs identified in this study have previously been identified as abundant in swine gut or fecal microbiomes and include Clostridium sensu stricto, Methanobrevibacter, Tissierella, Phascolarctobacterium, and Romboutsia (Leser et al., 2002; Miller and Lin, 2002; Cotta et al., 2003; Ziemer, 2013; Tran et al., 2018). Sequences associated with Tissierella have previously been cited as one of few highly abundant OTUs in manure retrieved from a swine lagoon (Goh et al., 2009). Clostridium sensu stricto has previously been observed to also significantly increase in surface waters after fall manure application in an agriculturally dominated watershed (Rieke et al., 2018b). The Archaeal genus, Methanobrevibacter, known to colonize swine intestinal tracts, has also been identified in swine pens, swine waste storage pits, and anaerobic biodigesters (Peu et al., 2006; Ike et al., 2010; Da Silva et al., 2015). Overall, these results suggest that these MDOs are indicative of the presence and enrichment of swine manure microbial communities in environmental samples. While these genera have been identified in the swine gut microbiome and areas impacted by swine waste, the majority of the categorized strains within the five genera are not known to be pathogenic to humans. Additionally, it is notable that all five MDOs are obligate anaerobes. While soil is known to harbor obligate anaerobes, manure additions likely increase the number of anaerobic niche communities. However, additional test must be conducted before determining if sequences matching MDOs persist as live cells, spores, or relic DNA.
Our study identifies specific species that can be used to track swine manure impacts, and this approach has been used by others previously (Kildare et al., 2007; Okabe et al., 2007; Savichtcheva et al., 2007). In contrast to these previous studies, which often use a single species or oligotype to track manure impacts, we use five MDOs to assess the impacts of applying manure to soils. In our study, we observed the peak relative abundances of the five MDOs in soil to comprise between 0.1 and 0.5% of microbial populations. While these abundances are low fractions of the total microbial community, the MDOs were consistently detected in all manure-amended samples, further evidence for their usage in surveillance of manure-associated impacts. A key challenge to large-scale monitoring of environmental samples and manure impact is the cost and throughput of approaches to detect and quantify risks associated with manure applications. Notably, assuming 109 and 1011 organisms per gram manure (Cotta et al., 2003), the proportions of MDOs should be detectable through qPCR methods, suggesting that development of species-specific primers could also expand the detection of these genes from phylogenetic marker sequencing to direct target approaches.
In addition to identifying manure source tracking targets, this study expanded knowledge regarding how incorporation of organic amendments in agricultural soils affect existing microbial communities. Simulation of swine manure application in the form of bands brings native soil bacteria in direct contact with available organic matter, similar to crop residue integration. Organic carbon and nitrogen additions in the form of manure or plant residue added into agricultural soils have resulted in similar increases in Actinobacteria, Bacteroidetes, Proteobacteria, and Firmicutes (Pascault et al., 2013; Chávez-Romero et al., 2016; Leclercq et al., 2016). However, depending on the nutrient matrix and incorporation method, certain groups of native soil microbial community members are capable of outcompeting each other. Along with organic incorporation methodology, tillage regiments have also shown to impact microbial community structure (Figuerola et al., 2012; Sipilä et al., 2012).
Soil management history response (chisel plow tillage versus no-till) had little response to manure application in our study when compared to other independent variables. However, this result may not accurately portray field managements’ influence on microbial communities due to discrepancies between our experimental design and true field conditions. Removal of the top 15 cm of soil for manure application in both no-till and chisel plow likely disrupted differences in soil structure. The manure incorporation zone disrupted any aggregation formed under no-till conditions, creating similar drainage flow paths between the chisel plow and no-till soils. Identifying the magnitude of microbial response to soil management strategies is important due to bacteria’s potential to transfer through the soil profile and into surrounding drainage waters.
Our study was the first to incorporate simulated rainfall application on soils following manure application in order to better understand bacterial community transport through the soil profile. We identified increases in the relative abundance of members within Actinobacteria, Bacteroides, Firmicutes, and Proteobacteria phyla in both soil and artificial drainage. Inflated relative abundances of certain groups of bacteria following manure application, common both to soil and water microbial communities, suggest the stimulated populations are easily detached from soil–manure interaction zones during matrix flow events. Once transported to a stream environment, little is currently known regarding potential survival rates of these bacterial populations and is an opportunity for further study.
Overall, the observed decreases in the majority of gene relative abundances associated with MSOs and MDOs in soil and water over the course of the experiment demonstrate agricultural soil’s natural ability to adapt to changing physical and chemical soil characteristics following organic matter additions. Swine manure is commonly injected late-fall once soil temperatures drop below 10°C or early spring after soil thaws in the Midwestern United States. Our experimental design closely mimics spring manure application timing where manure is applied shortly before typical artificial drainage seasons. Results from our study expanded our knowledge of microbial constituents that are released from the soil profile into drainage waters. This study represents an important initial characterization of this system and is a model of more complex dynamics in the natural environment. It is important for subsequent studies to expand on this effort at larger environmental scales to better characterize broadly representative bacteria indicative of potential manure presence in agroecosystems.
ER, TM, MS, FY, and AH designed, performed, and analyzed the research and wrote the paper.
This project was partially supported by AFRI food safety grant no. 2016-68003-24604 from the USDA National Institute of Food and Agriculture. This project was also partially funded by the Center for Health Effects of Environmental Contamination.
The authors declare that the research was conducted in the absence of any commercial or financial relationships that could be construed as a potential conflict of interest.
The authors would like to thank Jared Flater, Rene Carson, Ellen Meis, and Leigh Ann Long for help with soil column collection and sample processing. The authors would also like to thank Drs. Heather Allen and Shannon Hinsa for providing advice on experimental design and data analysis. The use of trade, firm, or corporation names in this publication is for the information and convenience of the reader. Such use does not constitute an official endorsement or approval by the United States Department of Agriculture or the Agricultural Research Service of any product or service to the exclusion of others that may be suitable. USDA is an equal opportunity provider and employer.
The Supplementary Material for this article can be found online at: https://www.frontiersin.org/articles/10.3389/fmicb.2018.03197/full#supplementary-material
Adesanya, T., Akinremi, O., and Zvomuya, F. (2016). Physical properties of an Orthic Black Chernozem after 5 years of liquid and solid pig manure application to annual and perennial crops. Can. J. Soil Sci. 96, 145–153. doi: 10.1139/cjss-2015-0086
Bonder, M. J., Abeln, S., Zaura, E., and Brandt, B. W. (2012). Comparing clustering and pre-processing in taxonomy analysis. Bioinformatics 28, 2891–2897. doi: 10.1093/bioinformatics/bts552
Buhler, D. D., Randall, G. W., Koskinen, W. C., and Wyse, D. L. (1993). Water-Quality - atrazine and alachlor losses from subsurface tile drainage of a clay loam soil. J. Environ. Qual. 22, 583–588. doi: 10.2134/jeq1993.00472425002200030024x
Chávez-Romero, Y., Navarro-Noya, Y. E., Reynoso-Martínez, S. C., Sarria-Guzmán, Y., Govaerts, B., Verhulst, N., et al. (2016). 16S metagenomics reveals changes in the soil bacterial community driven by soil organic C, N-fertilizer and tillage-crop residue management. Soil Tillage Res. 159, 1–8. doi: 10.1016/J.STILL.2016.01.007
Chen, W., Zhang, C. K., Cheng, Y., Zhang, S., Zhao, H., and Casiraghi, M. (2013). A comparison of methods for clustering 16S rRNA sequences into OTUs. PLoS One 8:e70837. doi: 10.1371/journal.pone.0070837
Choudhary, M., Bailey, L. D., and Grant, C. A. (1996). Review of the use of swine manure in crop production: effects on yield and composition and on soil and water quality. Waste Manag. Res. 14, 581–595. doi: 10.1177/0734242X9601400606
Cole, J. R., Wang, Q., Fish, J. A., Chai, B., McGarrell, D. M., Sun, Y., et al. (2014). Ribosomal Database Project: data and tools for high throughput rRNA analysis. Nucleic Acids Res. 42, D633–D642. doi: 10.1093/nar/gkt1244
Cotta, M. A., Whitehead, T. R., and Zeltwanger, R. L. (2003). Isolation, characterization and comparison of bacteria from swine faeces and manure storage pits. Environ. Microbiol. 5, 737–745. doi: 10.1046/j.1467-2920.2003.00467.x
Da Silva, M. L. B., Cantão, M. E., Mezzari, M. P., Ma, J., and Nossa, C. W. (2015). Assessment of bacterial and archaeal community structure in swine wastewater treatment processes. Microb. Ecol. 70, 77–87. doi: 10.1007/s00248-014-0537-8
Dai, Z., Barberán, A., Li, Y., Brookes, P. C., and Xu, J. (2017). Bacterial community composition associated with pyrogenic organic matter (biochar) varies with pyrolysis temperature and colonization environment. mSphere 2:e00085-17. doi: 10.1128/mSphere.00085-17
Ding, G.-C., Radl, V., Schloter-Hai, B., Jechalke, S., Heuer, H., Smalla, K., et al. (2014). Dynamics of soil bacterial communities in response to repeated application of manure containing sulfadiazine. PLoS One 9:e92958. doi: 10.1371/journal.pone.0092958
Dixon, P. (2009). VEGAN, a package of R functions for community ecology. J. Veg. Sci. 14, 927–930. doi: 10.1111/j.1654-1103.2003.tb02228.x
Economic Research Service [ERS], and United States Department of Agriculture [USDA] (2018). Livestock, Dairy, and Poultry Outlook. Washington, DC: United States Department of Agriculture.
Edgar, R. C., Haas, B. J., Clemente, J. C., Quince, C., and Knight, R. (2011). UCHIME improves sensitivity and speed of chimera detection. Bioinformatics 27, 2194–2200. doi: 10.1093/bioinformatics/btr381
Farthelrahman, E., Ascough, J., Hoag, D., Malone, R., Heilman, P., Wiles, L., et al. (2011). Economic and stochastic efficiency comparison of experimental tillage systems in corn and soybean under risk. Exp. Agric. 47, 111–136. doi: 10.1017/S0014479710000979
Figuerola, E. L. M., Guerrero, L. D., Rosa, S. M., Simonetti, L., Duval, M. E., Galantini, J. A., et al. (2012). Bacterial indicator of agricultural management for soil under no-till crop production. PLoS One 7:e51075. doi: 10.1371/journal.pone.0051075
Flury, M. (1996). Experimental evidence of transport of pesticides through field soils—a review. J. Environ. Qual. 25, 25–45. doi: 10.2134/jeq1996.00472425002500010005x
Fu, L., Niu, B., Zhu, Z., Wu, S., and Li, W. (2012). CD-HIT: accelerated for clustering the next-generation sequencing data. Bioinformatics 28, 3150–3152. doi: 10.1093/bioinformatics/bts565
Givens, C. E., Kolpin, D. W., Borchardt, M. A., Duris, J. W., Moorman, T. B., and Spencer, S. K. (2016). Detection of hepatitis E virus and other livestock-related pathogens in Iowa streams. Sci. Total Environ. 566–567, 1042–1051. doi: 10.1016/j.scitotenv.2016.05.123
Goh, S. H. M., Mabbett, A. N., Welch, J. P., Hall, S. J., and McEwan, A. G. (2009). Molecular ecology of a facultative swine waste lagoon. Lett. Appl. Microbiol. 48, 486–492. doi: 10.1111/j.1472-765X.2009.02560.x
Gordoncillo, M. J. N., Donabedian, S., Bartlett, P. C., Perri, M., Zervos, M., Kirkwood, R., et al. (2013). Isolation and molecular characterization of vancomycin-resistant Enterococcus faecium from swine in Michigan, USA. Zoonoses Public Health 60, 319–326. doi: 10.1111/zph.12008
Haack, S. K., Duris, J. W., Kolpin, D. W., Fogarty, L. R., Johnson, H. E., Gibson, K. E., et al. (2015). Genes indicative of zoonotic and swine pathogens are persistent in stream water and sediment following a swine manure spill. Appl. Environ. Microbiol. 81, 3430–3441. doi: 10.1128/AEM.04195-14
Hammesfahr, U., Bierl, R., and Thiele-Bruhn, S. (2011). Combined effects of the antibiotic sulfadiazine and liquid manure on the soil microbial-community structure and functions. J. Plant Nutr. Soil Sci. 174, 614–623. doi: 10.1002/jpln.201000322
Hammesfahr, U., Heuer, H., Manzke, B., Smalla, K., and Ren Thiele-Bruhn, S. (2008). Impact of the antibiotic sulfadiazine and pig manure on the microbial community structure in agricultural soils. Soil Biol. Biochem. 40, 1583–1591. doi: 10.1016/j.soilbio.2008.01.010
Hoang, T. T. T., Soupir, M. L., Liu, P., and Bhandari, A. (2013). Occurrence of tylosin-resistant Enterococci in swine manure and tile drainage systems under no-till management. Water Air Soil Pollut. 224:1754. doi: 10.1007/s11270-013-1754-3
Ike, M., Inoue, D., Miyano, T., Liu, T. T., Sei, K., Soda, S., et al. (2010). Microbial population dynamics during startup of a full-scale anaerobic digester treating industrial food waste in Kyoto eco-energy project. Bioresour. Technol. 101, 3952–3957. doi: 10.1016/J.BIORTECH.2010.01.028
Jamieson, R., Gordon, R., Sharples, K., Stratton, G., and Madani, A. (2002). Movement and persistence of fecal bacteria in agricultural soils and subsurface drainage water: a review. Can. Biosyst. Eng. 44, 1–9.
Jaynes, D. B., Colvin, T. S., Karlen, D. L., Cambardella, C. A., and Meek, D. W. (2001). Nitrate loss in subsurface drainage as affected by nitrogen fertilizer rate. J. Environ. Qual. 30, 1305–1314. doi: 10.2134/jeq2001.3041305x
Kildare, B. J., Leutenegger, C. M., Mcswain, B. S., Bambic, D. G., Rajal, V. B., and Wuertz, S. (2007). 16S rRNA-based assays for quantitative detection of universal, human-, cow-, and dog-specific fecal Bacteroidales: a Bayesian approach. Water Res. 41, 3701–3715. doi: 10.1016/j.watres.2007.06.037
Kozich, J. J., Westcott, S. L., Baxter, N. T., Highlander, S. K., and Schloss, P. D. (2013). Development of a dual-index sequencing strategy and curation pipeline for analyzing amplicon sequence data on the MiSeq Illumina sequencing platform. Appl. Environ. Microbiol. 79, 5112–5120. doi: 10.1128/AEM.01043-13
Kumari, P., Choi, H. L., and Sudiarto, S. I. A. (2015). Assessment of bacterial community assembly patterns and processes in pig manure slurry. PLoS One 10:e0139437. doi: 10.1371/journal.pone.0139437
Larkin, R. P., Honeycutt, C. W., and Griffin, T. S. (2006). Effect of swine and dairy manure amendments on microbial communities in three soils as influenced by environmental conditions. Biol. Fertil. Soils 43, 51–61. doi: 10.1007/s00374-005-0060-7
Leclercq, S. O., Wang, C., Sui, Z., Wu, H., Zhu, B., Deng, Y., et al. (2016). A multiplayer game: species of Clostridium, Acinetobacter, and Pseudomonas are responsible for the persistence of antibiotic resistance genes in manure-treated soils. Environ. Microbiol. 18, 3494–3508. doi: 10.1111/1462-2920.13337
Leser, T. D., Amenuvor, J. Z., Jensen, T. K., Lindecrona, R. H., Boye, M., and Møller, K. (2002). Culture-independent analysis of gut bacteria: the pig gastrointestinal tract microbiota revisited. Appl. Environ. Microbiol. 68, 673–690. doi: 10.1128/AEM.68.2.673-690.2002
Liu, P., Jia, S., He, X., Zhang, X., and Ye, L. (2017). Different impacts of manure and chemical fertilizers on bacterial community structure and antibiotic resistance genes in arable soils. Chemosphere 188, 455–464. doi: 10.1016/j.chemosphere.2017.08.162
Looft, T., Johnson, T. A., Allen, H. K., Bayles, D. O., Alt, D. P., Stedtfeld, R. D., et al. (2012). In-feed antibiotic effects on the swine intestinal microbiome. Proc. Natl. Acad. Sci. U.S.A. 109, 1691–1696. doi: 10.1073/pnas.1120238109
Love, M. I., Huber, W., and Anders, S. (2014). Moderated estimation of fold change and dispersion for RNA-seq data with DESeq2. Genome Biol. 15:550. doi: 10.1186/s13059-014-0550-8
Lu, X.-M., Lu, P.-Z., and Zhang, H. (2014). Bacterial communities in manures of piglets and adult pigs bred with different feeds revealed by 16S rDNA 454 pyrosequencing. Appl. Microbiol. Biotechnol. 98, 2657–2665. doi: 10.1007/s00253-013-5211-4
Luby, E. M., Moorman, T. B., and Soupir, M. L. (2016). Fate and transport of tylosin-resistant bacteria and macrolide resistance genes in artificially drained agricultural fields receiving swine manure. Sci. Total Environ. 550, 1126–1133. doi: 10.1016/j.scitotenv.2016.01.132
McMurdie, P. J., and Holmes, S. (2013). Phyloseq: an R package for reproducible interactive analysis and graphics of microbiome census data. PLoS One 8:e61217. doi: 10.1371/journal.pone.0061217
Miller, T. L., and Lin, C. (2002). Description of Methanobrevibacter gottschalkii sp. nov., Methanobrevibacter thaueri sp. nov., Methanobrevibacter woesei sp. nov. and Methanobrevibacter wolinii sp. nov. Int. J. Syst. Evol. Microbiol. 52, 819–822.
Narrod, C., Tiongco, M., and Scott, R. (2011). Current and predicted trends in the production, consumption and trade of live animals and their products. Rev. Sci. Tech. 30, 1–18. doi: 10.20506/rst.30.1.2014
Okabe, S., Okayama, N., Savichtcheva, O., and Ito, T. (2007). Quantification of host-specific Bacteroides–Prevotella 16S rRNA genetic markers for assessment of fecal pollution in freshwater. Environ. Biotechnol. 74, 890–901. doi: 10.1007/s00253-006-0714-x
Oksanen, J., Blanchet, F. G., Friendly, M., Kindt, R., Legendre, P., Mcglinn, D., et al. (2013). Community Ecology Package. Available at: https://cran.ism.ac.jp/web/packages/vegan/vegan.pdf [accessed November 20,2018].
Pajarillo, E. A. B., Chae, J. P., Balolong, M. P., Kim, H. B., Seo, K.-S., and Kang, D.-K. (2015). Characterization of the fecal microbial communities of Duroc pigs using 16S rRNA gene pyrosequencing. Asian Australas. J. Anim. Sci. 28, 584–591. doi: 10.5713/ajas.14.0651
Pascault, N., Ranjard, L., Kaisermann, A., Bachar, D., Christen, R., Terrat, S., et al. (2013). Stimulation of different functional groups of bacteria by various plant residues as a driver of soil priming effect. Ecosystems 16, 810–822. doi: 10.1007/s10021-013-9650-7
Peu, P., Brugère, H., Pourcher, A.-M., Kérourédan, M., Godon, J.-J., Delgenès, J.-P., et al. (2006). Dynamics of a pig slurry microbial community during anaerobic storage and management. Appl. Environ. Microbiol. 72, 3578–3585. doi: 10.1128/AEM.72.5.3578-3585.2006
Riber, L., Poulsen, P. H. B., Al-Soud, W. A., Skov Hansen, L. B., Bergmark, L., Brejnrod, A., et al. (2014). Exploring the immediate and long-term impact on bacterial communities in soil amended with animal and urban organic waste fertilizers using pyrosequencing and screening for horizontal transfer of antibiotic resistance. FEMS Microbiol. Ecol. 90, 206–224. doi: 10.1111/1574-6941.12403
Rieke, E. L., Moorman, T. B., Douglass, E. L., and Soupir, M. L. (2018a). Seasonal variation of macrolide resistance gene abundances in the South Fork Iowa River Watershed. Sci. Total Environ. 610–611, 1173–1179. doi: 10.1016/j.scitotenv.2017.08.116
Rieke, E. L., Moorman, T. B., Soupir, M. L., Yang, F., and Howe, A. (2018b). Assessing pathogen presence in an intensively tile drained, agricultural watershed. J. Environ. Qual. 47, 1033–1042. doi: 10.2134/jeq2017.12.0500
Savichtcheva, O., Okayama, N., and Okabe, S. (2007). Relationships between Bacteroides 16S rRNA genetic markers and presence of bacterial enteric pathogens and conventional fecal indicators. Water Res. 41, 3615–3628. doi: 10.1016/j.watres.2007.03.028
Sharpley, A. N., and Withers, P. J. A. (1994). The environmentally-sound management of agricultural phosphorus. Fertil. Res. 39, 133–146. doi: 10.1007/BF00750912
Sipilä, T. P., Yrjälä, K., Alakukku, L., and Palojärvi, A. (2012). Cross-site soil microbial communities under tillage regimes: fungistasis and microbial biomarkers. Appl. Environ. Microbiol. 78, 8191–8201. doi: 10.1128/AEM.02005-12
Tran, H., Anderson, C. L., Bundy, J. W., Fernando, S. C., Miller, P. S., and Burkey, T. E. (2018). Effects of spray-dried porcine plasma on fecal microbiota in nursery pigs. J. Anim. Sci. 96, 1017–1031. doi: 10.1093/jas/skx034
Trivedi, P., Delgado-Baquerizo, M., Anderson, I. C., and Singh, B. K. (2016). Response of soil properties and microbial communities to agriculture: implications for primary productivity and soil health indicators. Front. Plant Sci. 7:990. doi: 10.3389/fpls.2016.00990
Udikovic-Kolic, N., Wichmann, F., Broderick, N. A., and Handelsman, J. (2014). Bloom of resident antibiotic-resistant bacteria in soil following manure fertilization. Proc. Natl. Acad. Sci. U.S.A. 111, 15202–15207. doi: 10.1073/pnas.1409836111
Wang, Q., Garrity, G. M., Tiedje, J. M., and Cole, J. R. (2007). Naive Bayesian classifier for rapid assignment of rRNA sequences into the new bacterial taxonomy. Appl. Environ. Microbiol. 73, 5261–5267. doi: 10.1128/AEM.00062-07
Zhang, Q.-C., Shamsi, I. H., Xu, D.-T., Wang, G.-H., Lin, X.-Y., Jilani, G., et al. (2012). Chemical fertilizer and organic manure inputs in soil exhibit a vice versa pattern of microbial community structure. Appl. Soil Ecol. 57, 1–8. doi: 10.1016/J.APSOIL.2012.02.012
Ziemer, C. J. (2013). Broad diversity and newly cultured bacterial isolates from enrichment of pig feces on complex polysaccharides. Microb. Ecol. 66, 448–461. doi: 10.1007/s00248-013-0185-4
Ziemer, C. J., Bonner, J. M., Cole, D., Vinjé, J., Constantini, V., Goyal, S., et al. (2010). Fate and transport of zoonotic, bacterial, viral, and parasitic pathogens during swine manure treatment, storage, and land application1. J. Anim. Sci. 88, E84–E94. doi: 10.2527/jas.2009-2331
Keywords: swine manure, soil, drainage water, bacterial communities, 16S rRNA
Citation: Rieke EL, Soupir ML, Moorman TB, Yang F and Howe AC (2018) Temporal Dynamics of Bacterial Communities in Soil and Leachate Water After Swine Manure Application. Front. Microbiol. 9:3197. doi: 10.3389/fmicb.2018.03197
Received: 17 July 2018; Accepted: 10 December 2018;
Published: 21 December 2018.
Edited by:
Andreas Ulrich, Leibniz-Zentrum für Agrarlandschaftsforschung (ZALF), GermanyReviewed by:
Kim Yrjälä, Zhejiang Agriculture and Forestry University, ChinaCopyright © 2018 Rieke, Soupir, Moorman, Yang and Howe. This is an open-access article distributed under the terms of the Creative Commons Attribution License (CC BY). The use, distribution or reproduction in other forums is permitted, provided the original author(s) and the copyright owner(s) are credited and that the original publication in this journal is cited, in accordance with accepted academic practice. No use, distribution or reproduction is permitted which does not comply with these terms.
*Correspondence: Elizabeth L. Rieke, RWxpemFiZXRoLmx1Ynkucmlla2VAZ21haWwuY29t
Disclaimer: All claims expressed in this article are solely those of the authors and do not necessarily represent those of their affiliated organizations, or those of the publisher, the editors and the reviewers. Any product that may be evaluated in this article or claim that may be made by its manufacturer is not guaranteed or endorsed by the publisher.
Research integrity at Frontiers
Learn more about the work of our research integrity team to safeguard the quality of each article we publish.