- 1Department of Anesthesiology, Jiangsu Province Hospital, The First Affiliated Hospital of Nanjing Medical University, Nanjing, China
- 2Department of Infectious Diseases, Nanjing First Hospital, Nanjing Medical University, Nanjing, China
- 3NHC Key Laboratory of Antibody Technique, Nanjing Medical University, Nanjing, China
- 4State Key Laboratory of Reproductive Medicine, Nanjing Medical University, Nanjing, China
- 5Analysis Center, Nanjing Medical University, Nanjing, China
- 6Nanjing University Medical School, The Affiliated Nanjing Drum Tower Hospital, Nanjing, China
- 7Department of Immunology, Nanjing Medical University, Nanjing, China
Acute lung injury (ALI) is the leading cause of morbidity and mortality in critically ill patients. Neutrophil extracellular traps (NETs) have been well documented in the ALI model of bacterial infection. In the present study, we demonstrated that poly I:C could induce pulmonary NETs. Upon poly I:C intratracheal inoculation, neutrophil infiltration in the bronchoalveolar lavage fluid (BALF) was significantly increased. Furthermore, the inflammatory cytokines IL-1β, IL-6, and TNF-α in the lung were also significantly elevated. Neutrophil depletion abolished NETs and decreased both neutrophil infiltration and IL-1β in the lung. As expected, DNase I, an inhibitor of MPO and NADPH, decreased pulmonary inflammation and NETs. Blocking of the poly I:C receptor TLR3 reduced lung inflammation and NETs. The MAPK kinase inhibitor p38 diminished the formation of NETs and restored the expression of the tight junction protein claudin-5 in the mouse lung when challenged with poly I:C. In summary, poly I:C induced the formation of pulmonary NETs and ALI, which may be associated with the activation of p38 MAPK and the decreased expression of claudin-5.
Introduction
Acute lung injury (ALI) and acute respiratory distress syndrome (ARDS) are the leading causes of morbidity and mortality in critically ill patients (Butt et al., 2016). Neutrophils, which are orchestrators and key players of the innate immune response, play diverse roles in ALI and ARDS (Abraham, 2003; Zhou et al., 2012). Inflammatory cytokines and chemokines from these accumulated activated neutrophils, evoke and aggravate lung inflammation. For example, in the lung-protective ventilatory strategy, neutrophil quantification and pro-inflammatory cytokine concentrations were markedly reduced (Goodman et al., 2003). In contrast, the uncontrolled and lethal cytokine storm, which recruited neutrophils and other immune cells, was responsible for ALI in the deadly H5N1 influenza virus infection (Tisoncik et al., 2012). Furthermore, the influenza virus triggered the induction of IL-8 and other inflammatory cytokines from neutrophils (Wang et al., 2008). Neutrophil extracellular traps (NETs) consist of nuclear chromatin, hyper citrullinated histones, granular antimicrobial proteins and cytoplasmic proteins. In an ALI model of bacterial infection (Narayana Moorthy et al., 2013), LPS challenge (Tadie et al., 2013; Liu et al., 2016), transfusion (Hishizawa et al., 2009; Looney et al., 2009), and ventilation (Yildiz et al., 2015), NETs have been well documented, suggesting pivotal roles for NETs in ALI.
The human respiratory syncytial virus (RSV) induces NETs in the lungs, contributing to airway obstruction (Cortjens et al., 2016). The influenza virus infection recruits large amounts of neutrophils, leading to the development of NETs in the lung and contributing to ALI (Narasaraju et al., 2011). NETs may also be linked to the human immunodeficiency virus (HIV), which is detected by Toll-like receptor 7 (TLR7) and TLR8 in neutrophils and induces the generation of NETs (Saitoh et al., 2012). The RSV, influenza virus and HIV are single-stranded viruses. In one study, poly I:C, a synthetic analog of a double stranded RNA (dsRNA) virus, stimulated NETs in the liver (Jenne et al., 2013). Though poly I:C induced lung inflammation and impaired lung function in mice (Stowell et al., 2009), whether poly I:C could induce NETs in the lungs as well as their role in ALI, remain largely elusive.
TLR3 is a potent poly (I:C) receptor expressed in the lung epithelial cells (Bals and Hiemstra, 2004). Furthermore, poly (I:C) induces the elevated expression of TLR3 in the small airway epithelial cells (Ritter et al., 2005). Mitogen-activated protein kinase (MAPK) p38 is required for poly (I:C)/TLR3-mediated cytokine production (Pisegna et al., 2004). The lung barrier is largely controlled by tight junction proteins known as claudins (Schlingmann et al., 2015). Claudin-5, which is expressed by both the pulmonary epithelial and endothelial cells, is closely associated with acute lung injury (Chen et al., 2014).
In this study, we provided a detailed in vivo exploration of NETs in poly I:C-induced ALI, which was involved in the TLR3/p38 MAPK signaling pathway and the aberrant expression of claudin-5. Therefore, we hypothesized that NETs may be potential targets in the therapy of dsRNA virus-associated ALI.
Materials and Methods
Experimental Animals
Female C57BL/6J mice that were 6–8 weeks of age and free of specific pathogens, were obtained from the College of Veterinary Medicine Yangzhou University (Yangzhou, China). All experimental animals used in this study were maintained under a protocol 1709011 and approved by the Institutional Animal Care and Use Committee of the Nanjing Medical University. All methods were performed in accordance with the relevant guidelines and regulations.
Acute Lung Injury Model and Treatment
The acute lung injury model was established as described previously (Aeffner et al., 2011). Briefly, the mice were anesthetized with an intraperitoneal (IP) injection of a mixture of ketamine (200 mg/kg) and xylazine (10 mg/kg). After exposing the trachea, a trimmed sterile 31-gauge needle was inserted into the tracheal lumen. Poly I:C (InvivoGen, CA, United States) diluted in endotoxin-free PBS (SCBT, sc-286634) was intratracheally (IT.) injected at a dose of 2.5 mg/kg in 50 μl PBS, followed by 100 μl air to ensure the full distribution of poly I:C into the lung. PBS was only instilled as an ALI model control.
To deplete neutrophils, anti-Ly6G (clone 1A8, Bio X Cell, NH, United States) or isotype control Ab at a dose of 200 μg in 200 μl PBS, was administered via the tail vein, 24 h before the establishment of the ALI model (Daley et al., 2008). To treat ALI mice, a DNase I (Roche, 11284932001), MPO inhibitor (4-ABH, sigma, A41909), NADPH inhibitor (Diphenyliodonium chloride, sigma, 43088), TLR3 inhibitor (Merck, 614310) or p38 inhibitor (SB203580, Selleck) was dissolved in DMSO and administered via an IP injection, 2 h before the inoculation of poly I:C. DNase I was given at 5 mg/kg, MPO inhibitor at 50 mg/kg, TLR3 inhibitor at 50 mg/kg, NADPH inhibitor at 10 mg/kg, and p38 inhibitor at 50 mg/kg. DMSO administration was set as the treatment control.
Analysis of BALF Samples
Four hours after the poly I:C or PBS challenge, the mice were anesthetized via an IP injection of a mixture of ketamine (200 mg/kg) and xylazine (10 mg/kg). A catheter was inserted into the trachea by way of an injected opening located in the cervical part, and the airways were washed three times with a total of 1.0 ml PBS. The pooled bronchoalveolar lavage fluid (BALF) was centrifuged at 1000 g for 5 min at 4°C, and the total cells were quantified with a hemocytometer, by two independent blinded investigators. Furthermore, the supernatant was collected to measure the quantity of protein using the Bradford Protein Assay Kit (Beyotime, P0006). The total cell counts and protein concentration in the BALF from the PBS control, isotype antibody control and DMSO control were greatly similar. Therefore, the data for the cell counts and protein concentration were pooled.
Flow Cytometry Analysis
Next, the cells in the BALF were stained with fluorescent antibodies to quantify neutrophils, macrophages and lymphocytes. Briefly, the cells in the BALF were blocked with anti-CD16/CD32 (BioLegend, 101302) to reduce non-specific binding at 4°C for 10 min. Anti-Gr-1 conjugated with APC (BioLegend, 108412), anti-F4/80 conjugated with FITC (eBioscience, 11-4801-81), and anti-CD4 conjugated with PE (eBioscience, 12-0042-85) were added to label neutrophils, macrophages and CD4+ lymphocytes at 37°C for 30 min in darkness. Finally, the staining tubes were centrifuged to remove the supernatant, and the cells were resuspended in 500 μl PBS and analyzed in the BD FACSCalibur. All FACS data were analyzed using the FlowJo V10.
Histopathology Imaging
After the BALF was collected, PBS was pumped into the right ventricle to clear the blood in the pulmonary vasculature. Then, the upper right lung lobe was removed and fixed in 10% neutral buffered formalin for 24 h; then, the specimens were dehydrated and embedded in paraffin. To carry out a histological examination, 5 μm sections of fixed embedded tissues were cut on a Leica model 2165 rotary microtome (Leica, Nussloch, Germany) and stained with hematoxylin and eosin. Histological analyses were performed by two independent pathologists blinded to the treatment groups.
RNA Preparation and Quantitative RT-PCR
After the upper lobe was removed for histopathology, the total RNA was purified from the residual right lung lobes using a TRIzol reagent (Invitrogen Life Technologies) and reverse transcribed using the Superscript II (Takara) to obtain the cDNA. Real-time PCR using the SYBR® Premix Ex TaqTM (Takara, Tli RNaseH Plus) and the PrimeScriptTM RT Master Mix (Takara, Perfect Real Time) was performed on the cDNA samples. The amplification conditions were 1 cycle at 50°C for 2 min, 1 cycle at 95°C for 30 s, followed by 40 cycles of thermal cycling at 95°C for 5 s and 60°C for 45 s.
Primer pair DNA sequences are shown as follows: (1) IL-1β, upper: 5′-AGCTCTCCACCTCAATGGA-3′; lower: 5′-TTGCTTGGGATCCACACTCT-3′; (2) IL-6, upper: 5′-GACTGATGCTGGTGACAACC-3′; lower: 5′-AGACAGGTCTGTTGGGAGTG-3′; (3) TNF-α, upper: 5′-GGTGAGGCAGCAAGAGATTG-3′, lower: 5′-GAGCAGCAGGTTTCAGGATG-3′. β-actin was used as an internal control using the following primer sequences: upper: 5′-ATGTTTGAGACCTTCAACAC-3′, lower: 5′-CACGTCACACTTCATGATGG-3′. Quantitative PCR assays were conducted in triplicate for each sample and performed using the 2-ΔΔCt method. Data were expressed as an n-fold difference relative to the expression of inner housekeeping gene β-actin. Blank controls lacking a template or without a reverse transcriptase, were also performed.
Fluorescence Microscopy
The left lung lobe was removed and immobilized with OCT (Sakura Finetek) at -80°C for at least 24 h. Frozen tissue blocks were cut on a cryostat microtome, and 5 μm sections were placed on coated glass slides. The tissue sections were fixed in cold acetone and rehydrated in PBS. After gently washing in PBS three times, the slides were blocked with 5% goat serum (Gibco, 16210-064) for 30 min at 37°C to reduce non-specific binding. After blocking, the sections were washed with PBS and stained with myeloperoxidase antibody (Abcam, ab90810) and anti-Histone H3 (citrulline R2 + R8 + R17) (Abcam, ab5103) at 4°C overnight in darkness. After staining, the sections were gently washed with PBS, followed by incubation with the secondary antibodies: Alexa Fluor 647-Anti-mouse IgG (Life Technology, A32728) or Alexa Fluor 555-Anti-rabbit IgG (Life Technology, A21428) at 37°C for 60 min in darkness. After washing in PBS to remove the unbound fluorescent antibody, the sections were incubated with Sytox Green (Thermo, s7020) diluted in PBS (1:2000) at 37°C for 15 min. The sections were rinsed again and mounted with glycerol. The frozen sections were recorded using confocal microscopy (Carl Zeiss LSM710). Positive fluorescence (MPO staining and citrullinated histone 3 staining) was calculated with the ImageJ software. The data were expressed as the percentage of the area in each field of view, covered by positive fluorescent staining.
Neutrophil extracellular traps in the BALF were quantified according to the manufacturers’ recommendations for the NETs Assay Kit (NO. 601010, Cayman) with modification. Briefly, the selective substrate was added to the BALF and quantified at 405 nm. The elastase activity in each BALF sample was calculated according to the NET standards. Instead, elastase activity could be indicative of NETs or neutrophil necrosis.
Western Blotting
The lower right lung lobes were removed and stored in liquid nitrogen for protein analysis. Briefly, the lung tissues were lysed on ice in a RIPA (P0013B, Beyotime) buffer containing 1 mM PMSF (Beyotime). After sonication, the protein concentration in the supernatants was measured using a bicinchoninic acid protein assay kit (Beyotime). Equal amounts of protein were loaded in 10% SDS–PAGE, and then the proteins were transferred to polyvinylidene fluoride membranes and blocked in 5% bovine serum albumin for 1 h at room temperature. After rinsing in PBST 5 times, the membranes were incubated with each primary antibody overnight at 4°C: the anti-claudin 5 (Abcam, ab15106), the phosphor-MAPK family Antibody sampler kit (cell-signaling technology, #9910) the MAPK family Antibody sampler kit (cell-signaling technology, #9926), and β-actin (cell-signaling technology, #4970). After washing with PBST, the membranes were finally incubated with the appropriate secondary antibody for 1 h at room temperature. Protein bands were detected using an ECL High-Signal reagent (Thermo). The band intensity was quantified using the ImageJ software.
Statistical Analysis
The experiments were repeated at least three times with consistent results. The significant difference between experimental groups and the control group was performed using the Student’s t-test with the SPSS software (SPSS, Chicago, IL, United States). P < 0.05 was considered statistically significant. All data are expressed as the mean ± SEM.
Results
Poly I:C Induced NETS in ALI
To determine whether poly I:C could induce NETs in the lung, we first inoculated poly I:C directly into the lung via the trachea. After poly I:C instillation, inflammation occurred in the lung, which was characterized by the infiltration of inflammatory cells and interstitial edema (Figure 1A). In accordance with the elevated lung inflammation, The BALF cells and proteins were significantly increased. Moreover, elastase activity was increased in the BALF for the mice inoculated with poly I:C, suggesting that poly I:C may induce NETs in the lung (Figure 1B). A flow cytometry analysis indicated that neutrophils, but not macrophages, were significantly increased in the BALF (Figure 1C). Additionally, the addition of poly I:C led to an increase in the inflammatory cytokines IL-1, IL-6, and TNF-α in the lung (Figure 1D). In the lung tissues from poly I:C treated mice, the intensities of MPO and citrullinated histone 3 (markers of neutrophils and NETs, respectively), were significantly increased (Figure 1E). The tight junction protein claudin-5, which may be responsible for ALI, was decreased in the lung tissues for poly I:C treated mice. Additionally, p38 MAPK was activated in poly I:C treated lung tissues, suggesting that the p38 MAPK pathway may be associated with poly I:C induced lung injury (Figure 1F). In summary, poly I:C induced NETs in ALI may be associated with elevated inflammatory cytokines, aberrant claudin-5 and the p38 MAPK pathway.
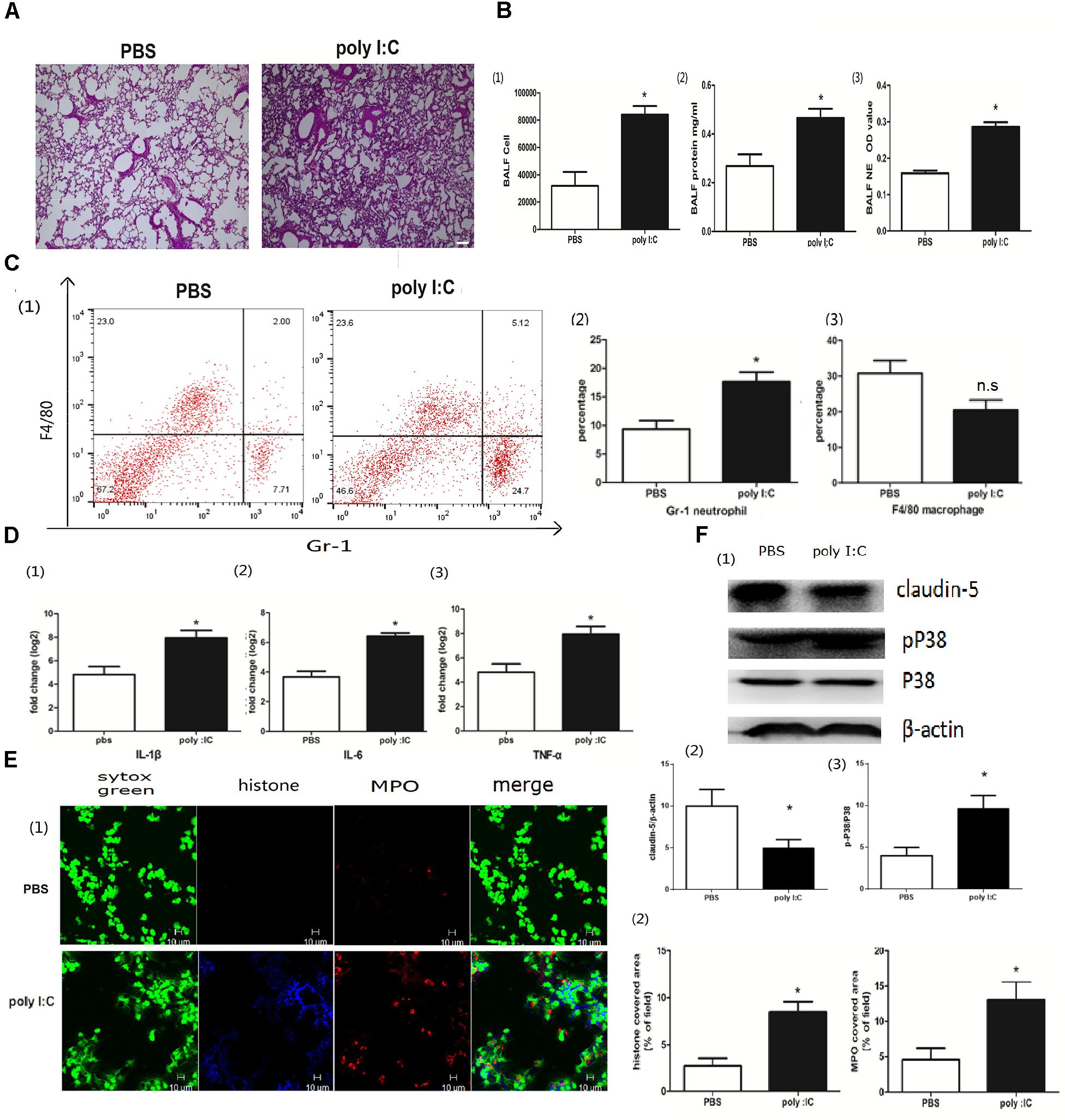
Figure 1. Poly I:C-induced inflammation and NETs in mice with ALI. (A) Infiltration of inflammatory cells and interstitial edema in the lung lobes from poly I:C-stimulated mice, bar = 100 μm; (B-1) cells were counted in the BALF, ∗p < 0.05; (B-2) protein concentration in the BALF, ∗p < 0.05; (B-3) neutrophil elastase (NE) activity was evaluated in the BALF, ∗p < 0.05; (C) flow cytometry analysis of the BALF cells, ∗p < 0.05; (D) inflammatory cytokines IL-1β, IL-6, and TNF-α in the lung were quantified using qRT-PCR, ∗p < 0.05; (E) NETs were observed and quantified in lung tissues from mice administered poly I:C. (F) Claudin-5 and p38 MAPK were measured in lung tissues using Western-blot.
Neutrophil Depletion Reduced NETs and ALI
Extracellular traps can be released not only by neutrophils but also by macrophages (Boe et al., 2015). To better clarify the roles of neutrophils in the extracellular traps and ALI, we injected a specific Ly6G antibody (1A8) via the tail vein to deplete neutrophils (Sun et al., 2016; Zhang et al., 2016). Neutrophil depletion ameliorated lung inflammation, resulting in less infiltration of inflammatory cells and less interstitial edema (Figure 2A). The BALF cells and proteins, which are hallmarks of the severity of acute lung injury, were also significantly decreased in the mice with neutrophil depletion (Figure 2B). Elastase activity, which indicates the formation of NETs, was significantly reduced in the mice with neutrophil depletion (Figure 2B). The inflammatory cytokine IL-1β but not IL-6 or TNF-α was also significantly decreased in the mice with neutrophil depletion (Figures 2C,D). Moreover, 1A8 treatment almost abolished the extracellular traps, as indicated by the nearly undetectable levels of MPO and citrullinated histone 3 (Figure 2E). The role of 1A8 in neutrophil depletion is validated in Figure 2F. In summary, neutrophils rather than macrophages were the major producer of extracellular traps in poly I:C induced acute lung injury.
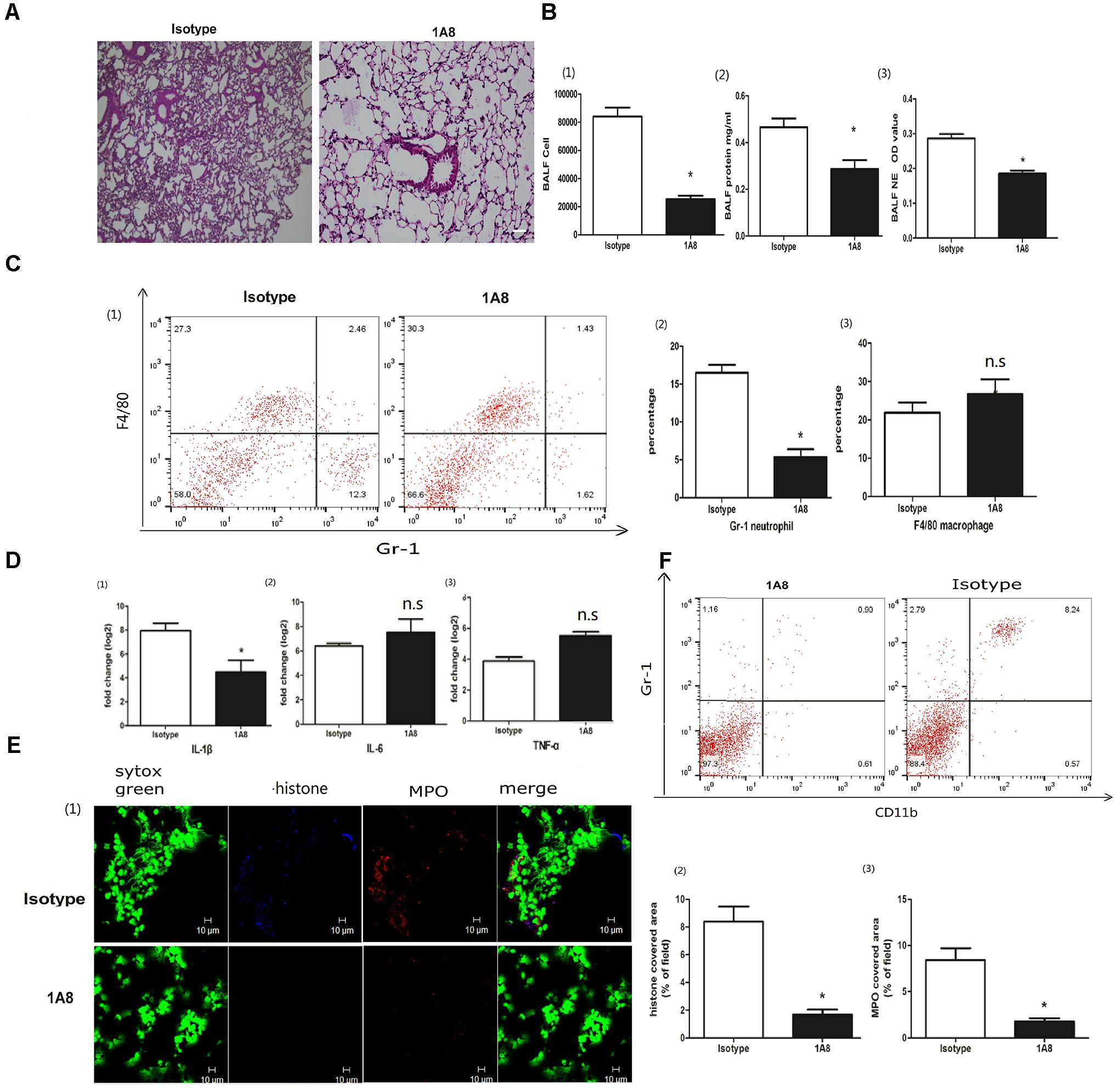
Figure 2. Neutrophil depletion reduced NETs and ALI. (A) Infiltration of inflammatory cells and interstitial edema in the lung lobe were reduced in the ALI model mice with neutrophil depletion, bar = 100 μm; (B-1) cells were counted in the BALF, ∗p < 0.05; (B-2) protein concentration in the BALF, ∗p < 0.05; (B-3) neutrophil elastase (NE) activity was evaluated in the BALF, ∗p < 0.05; (C) flow cytometry analysis of the BALF cells, ∗p < 0.05; (D) inflammatory cytokines IL-1β, IL-6, and TNF-α in the lung were quantified using qRT-PCR, ∗p < 0.05; (E) NETs were observed and quantified in lung tissues from mice administered poly I:C and 1A8 antibody. (F) Effects of 1A8 on neutrophil depletion were validated by flow cytometry.
DNase Protected the Lung From Poly I:C-Induced NETs
DNA is the scaffold of the NET (Zawrotniak and Rapala-Kozik, 2013). To better explore the roles of NETs in acute lung injury, we treated the mice with DNase I to degrade poly I:C-induced NETs. DNase administration reduced lung inflammation (Figure 3A). Infiltrated cells, proteins and NET formation in the BALF were also significantly reduced in the DNase I-treated mice (Figure 3B). Additionally, neutrophils in the BALF were significantly decreased in the DNase I-treated mice (Figure 3C). Interestingly, DNase I treatment played a non-significant role in the production of inflammatory cytokines in the lung tissue (Figure 3D). However, DNase I treatment significantly reduced the formation of NETs (Figure 3E), further implying that poly I:C-induced NETs may contribute to acute lung injury.
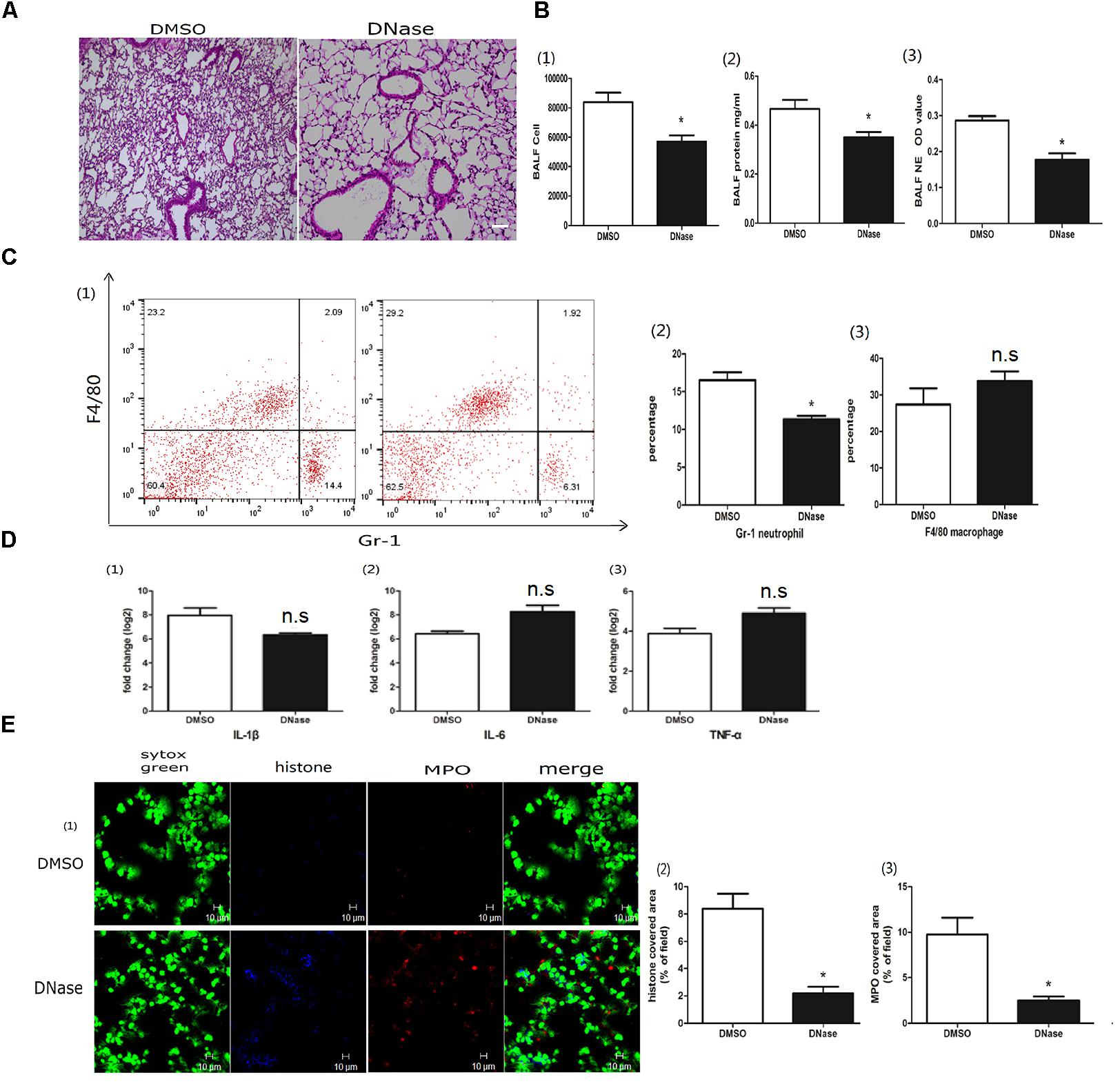
Figure 3. DNase protected the lung from poly I:C-induced NETs. (A) Infiltration of inflammatory cells and interstitial edema in the lung lobe were reduced in the ALI model mice upon DNase treatment, bar = 100 μm; (B-1) cells were counted in the BALF, ∗p < 0.05; (B-2) protein concentration in the BALF, ∗p < 0.05; (B-3) neutrophil elastase (NE) activity was evaluated in the BALF, ∗p < 0.05; (C) flow cytometry analysis of the BALF cells, ∗p < 0.05; (D) inflammatory cytokines IL-1β, IL-6, and TNF-α in the lung were quantified using qRT-PCR, ∗p < 0.05; (E) NETs were observed and quantified in lung tissues from mice administered poly I:C and DNase.
MPO Contributed to Poly I:C Induced NETs
MPO, the peroxidase enzyme in neutrophils, was required for NET formation in a Candida albicans infection (Metzler et al., 2011). To investigate whether MPO was involved in poly I:C-induced NETs in acute lung jury, we treated the mice with the MPO inhibitor 4-aminobenzoic hydrazide (4-ABH). Interestingly, 4-ABH suppressed the infiltration of inflammatory cells in the lung (Figure 4A). The BALF cells, proteins and NETs were all significantly reduced in mice upon 4-ABH administration (Figure 4B). Flow cytometry analysis revealed that MPO inhibition resulted in reduced neutrophil infiltration in the BALF (Figure 4C). IL-1β in the lung was significantly decreased in the 4-ABH treated mice (Figure 4D). Furthermore, 4-ABH almost eradicated the NETs (Figure 4E), suggesting the pivotal role of MPO in poly I:C-induced NETs and ALI.
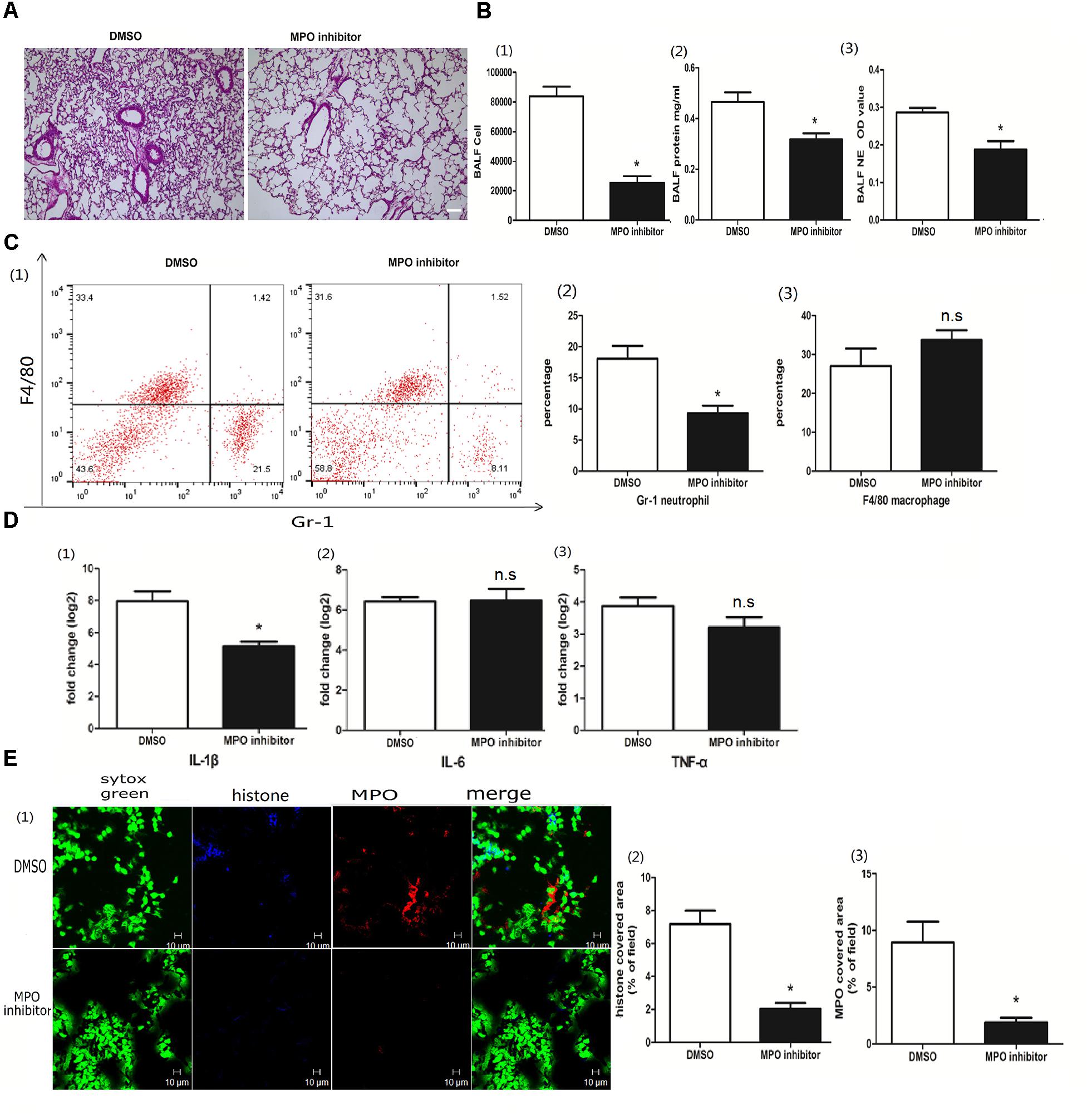
Figure 4. MPO contributed to poly I:C induced NETs. (A) Infiltration of inflammatory cells and interstitial edema in the lung lobe were decreased in the ALI mice treated with an MPO inhibitor, bar = 100 μm; (B-1) cells were counted in the BALF, ∗p < 0.05; (B-2) protein concentration in the BALF, ∗p < 0.05; (B-3) neutrophil elastase (NE) activity was evaluated in the BALF, ∗p < 0.05; (C) flow cytometry analysis of the BALF cells, ∗p < 0.05; (D) inflammatory cytokines IL-1β, IL-6, and TNF-α in the lung were quantified using qRT-PCR, ∗p < 0.05; (E) NETs were observed and quantified in lung tissues from mice administered poly I:C and an MPO inhibitor.
NADPH Was Associated With the Poly I:C-Induced NETs
The role of NADPH in the NETs was subject to the stimulus, and, previously, either NADPH-dependent or -independent NETs were reported (Marcos et al., 2010; Parker et al., 2012). To better clarify whether poly I:C-induced NETs relied on NADPH, we used the NADPH oxidase inhibitor diphenyliodonium chloride (DPI) to block the enzyme. With DPI administration, we observed less infiltration of inflammatory cells in the lung (Figure 5A). Although the quantification of inflammatory cells in the BALF was comparable, protein concentration and NETs in the BALF were significantly decreased (Figure 5B). Again, DPI significantly reduced neutrophils infiltration and IL-1β in the lung (Figures 5C,D). More importantly, DPI reduced the formation of NETs (Figure 5E), suggesting that the role of poly I:C-induced NETs in ALI was NADPH dependent.
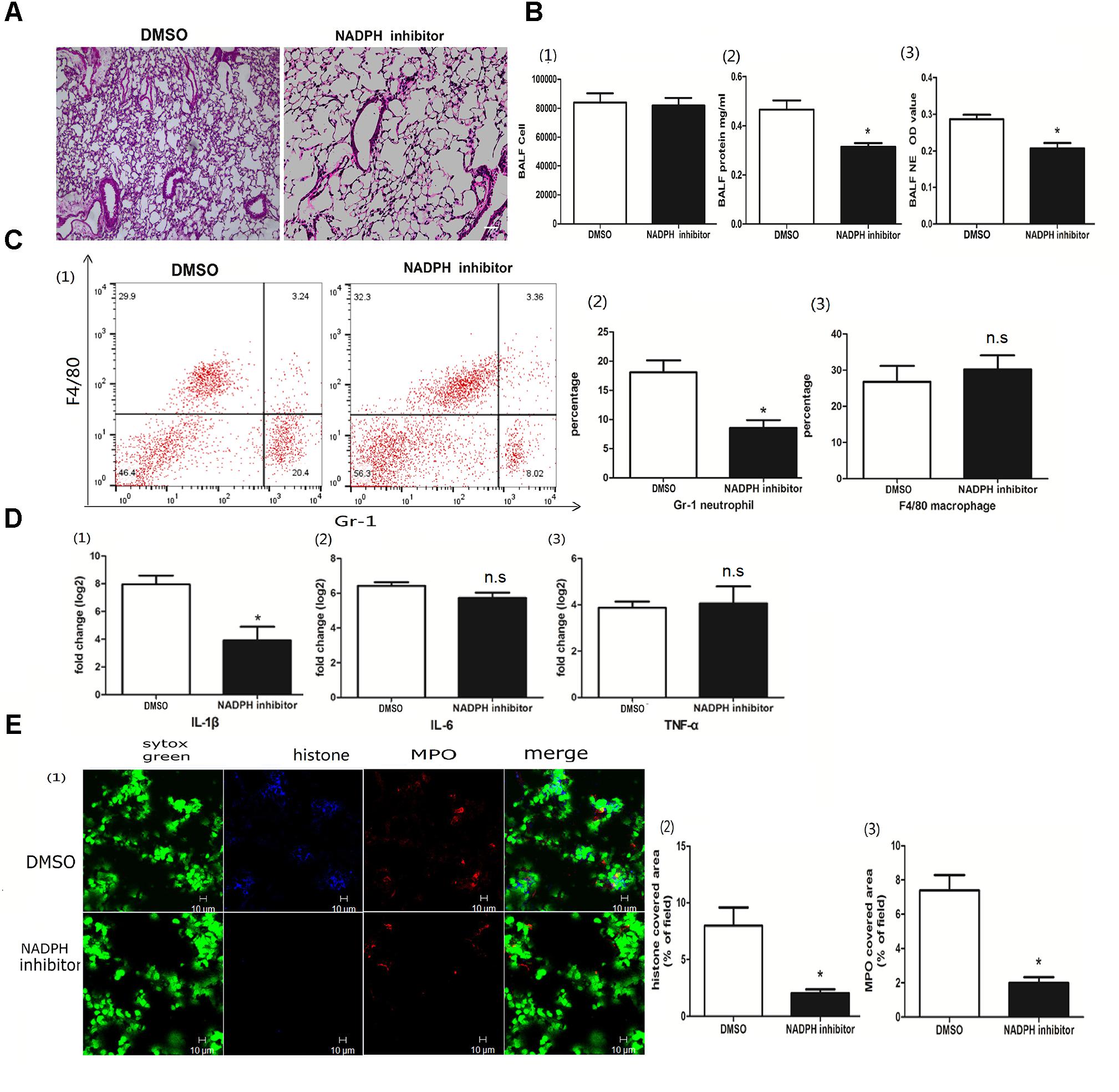
Figure 5. NADPH was associated with poly I:C-induced NETs. (A) Infiltration of inflammatory cells and interstitial edema in the lung lobe were inhibited in the ALI mice treated with an NADPH inhibitor, bar = 100 μm; (B-1) cells were counted in the BALF, ∗p < 0.05; (B-2) protein concentration in the BALF, ∗p < 0.05; (B-3) neutrophil elastase (NE) activity was were evaluated in the BALF, ∗p < 0.05; (C) flow cytometry analysis of the BALF cells, ∗p < 0.05; (D) inflammatory cytokines IL-1β, IL-6, and TNF-α in the lung were quantified using qRT-PCR, ∗p < 0.05; (E) NETs were observed and quantified in the lung tissues from mice administered poly I:C and an NADPH inhibitor.
The Role of Poly I:C-Induced NETs in ALI Was TLR3 Dependent
Toll-like receptor 3 (TLR3) is an intracellular poly I:C receptor (Prince et al., 2011). Unlike human neutrophils, mouse neutrophils express TLR3 (Applequist et al., 2002). To clarify whether the role of poly I:C-induced NETs in ALI was TLR3 dependent, we used a TLR3 inhibitor to suppress the recognition of TLR3 with poly I:C. The TLR3 inhibitor decreased not only lung inflammation (Figure 6A), but also cells and proteins in the BALF (Figure 6B), suggesting that poly I:C-induced ALI depends on TLR3. In line with the amelioration of ALI, NET formation in the BALF was almost eradicated upon TLR3 inhibitor administration (Figure 6B). Upon TLR3 blocking, the levels of neutrophil infiltration in the BALF (Figure 6C) and IL-1β in the lung (Figure 6D) were significantly decreased. Immunofluorescence microscopy showed that TLR3 inhibitors also significantly reduced NET formation in the lung (Figure 6E). Altogether, these data indicate that TLR3 is required for the role of poly I:C-induced NETs in ALI.
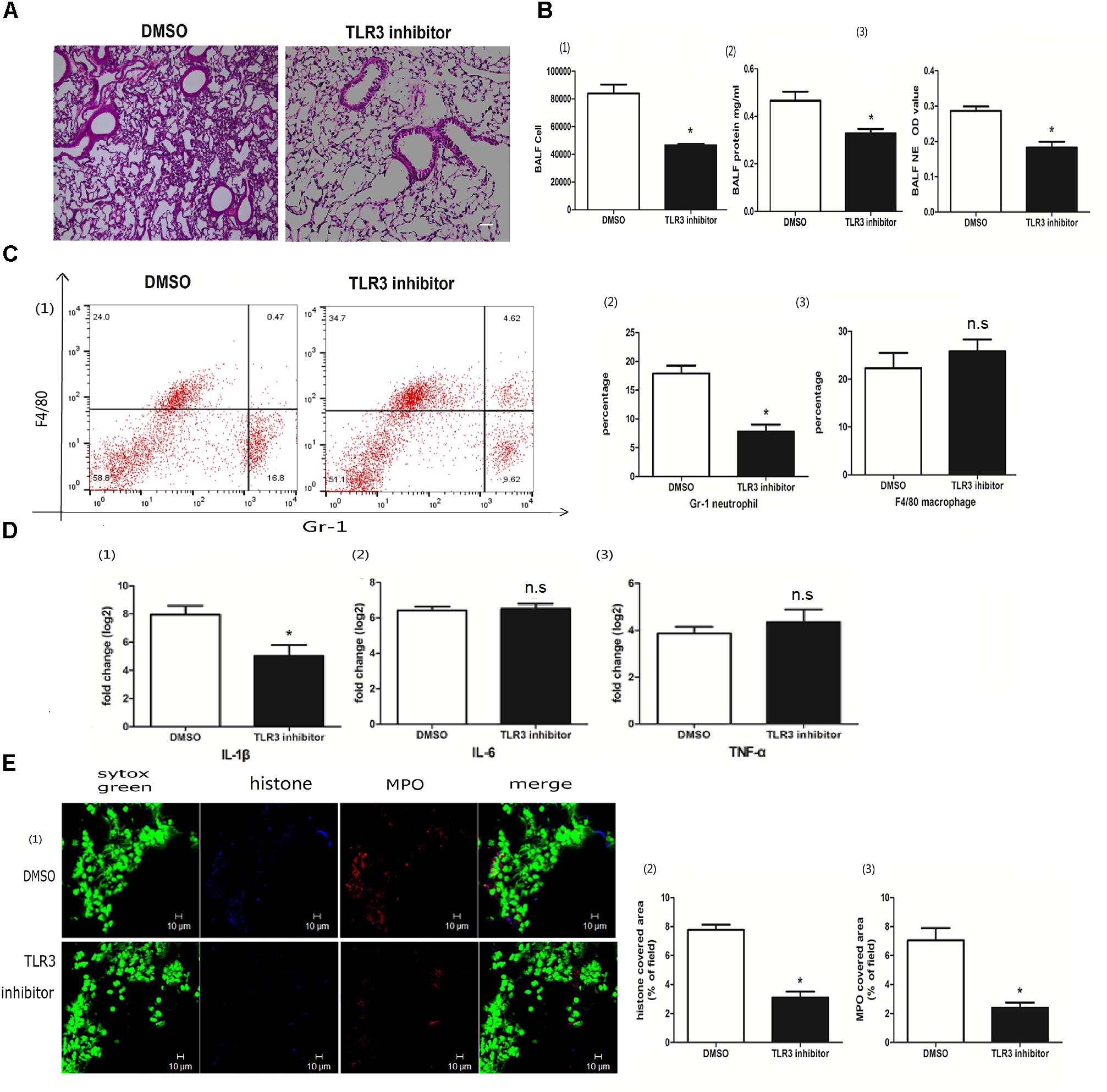
Figure 6. The role of poly I:C-induced NETs in ALI was TLR3 dependent. (A) Infiltration of inflammatory cells and interstitial edema in the lung lobe were reduced in the ALI mice treated with the TLR3 inhibitor, bar = 100 μm; (B-1) cells were counted in the BALF, ∗p < 0.05; (B-2) protein concentration in the BALF, ∗p < 0.05; (B-3) neutrophil elastase (NE) activity was evaluated in the BALF, ∗p < 0.05; (C) flow cytometry analysis of the BALF cells, ∗p < 0.05; (D) inflammatory cytokines IL-1β, IL-6, and TNF-α in the lung were quantified using qRT-PCR, ∗p < 0.05; (E) NETs were observed and quantified in lung tissues from mice administered poly I:C and a TLR3 inhibitor.
p38 Regulated the Poly I:C-Induced NETs and ALI
Poly I:C bound with TLR3 evokes a cascade reaction, including the MAPK signaling pathway activation (Kawai and Akira, 2010). Moreover, the MAPK pathway was also shown to be required for NETs (Hakkim et al., 2011). To further explore whether p38 MAPK kinase contributes to poly I:C-induced pulmonary NETs, mice were treated with the p38 inhibitor SB203580. As shown in Figure 7A, the p38 inhibitor SB203580 decreased the production of NETs in the BALF. Furthermore, the p38 inhibitor reduced neutrophil infiltration in the BALF (Figure 7B) and suppressed NETs in the lung (Figure 7C). Interestingly, the p38 inhibitor partially recovered the expression of claudin-5 (Figure 7D), highlighting that p38 and claudin-5 might be potential targets in poly I:C-induced NETs and ALI.
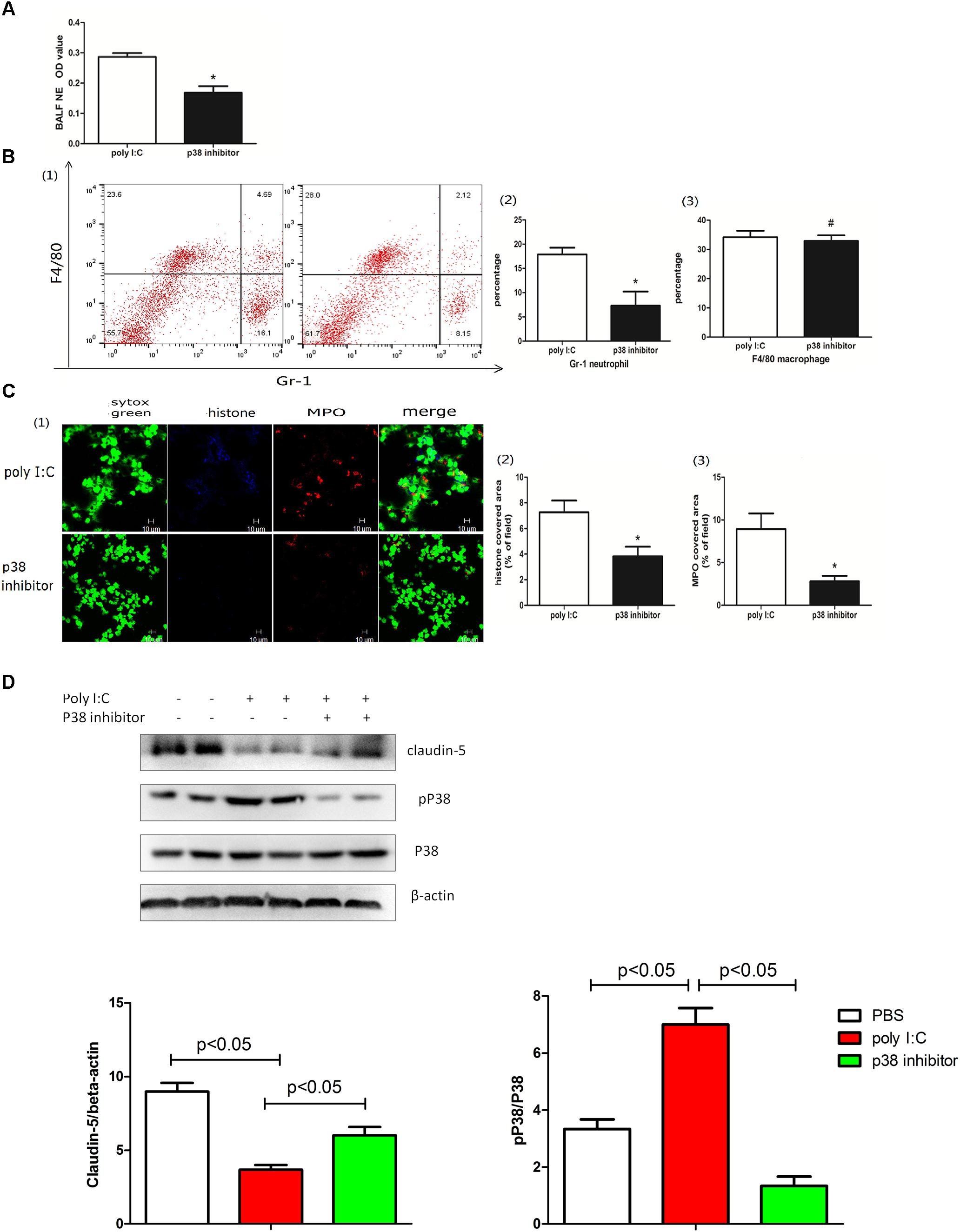
Figure 7. p38 regulated poly I:C-induced NETs and ALI. (A) neutrophil elastase (NE) activity was evaluated in the BALF, ∗p < 0.05; (B) flow cytometry analysis of the BALF cells, ∗p < 0.05 and #p > 0.05; (C) NETs were observed and quantified in lung tissues from mice administered poly I:C and a p38 inhibitor. (D) Claudin-5 and p38 were measured in lung tissues via Western-blot; ∗p < 0.05.
Discussion
As a critical illness syndrome, ALI is characterized by acute hypoxemic respiratory failure with the presence of bilateral diffuse pulmonary infiltrates (Wheeler and Bernard, 2007). Viral infections rank as top etiology of ALI in intensive care units (Erickson et al., 2007). More importantly, influenza virus (H1N1 (Narasaraju et al., 2011), H5N1 (Sun et al., 2012), H9N2 (Li et al., 2016)) and severe acute respiratory syndrome (SARS) virus (Kuba et al., 2005) might even induce deadly ALI and ARDS. It is not clear if viral replication or viral components (nucleic acids or proteins) are responsible for ALI and ARDS. In this study, we observed that poly I:C, a double-stranded RNA virus mimic, induced the infiltration of inflammatory cells in the lung, leading to ALI. Furthermore, extracellular diffuse DNA that was overlaid with MPO and citrullinated histone 3 (Wang et al., 2009) was recorded, suggesting a pulmonary NET formation. It is important to note that citrullinated histone 3 was not always perfectly overlaid with MPO. Citrullinated histone 3 has also been observed in the TNF-α associated inflammation (Sharma et al., 2012). MPO was most abundant in the neutrophils but was also present in monocytes and some populations of macrophages (McMillen et al., 2005). Collectively, we postulated that double-stranded viruses may evoke NETs via nucleic acids.
The Ly6G antibody 1A8 was more specific than the RB6-8C5, which recognized Ly6G on neutrophils and Ly6C on monocytes and macrophages; therefore, the latter antibody depleted neutrophils only (Daley et al., 2008). In this study, neutrophil depletion with 1A8 abolished NETs, confirming that the extracellular trap was released from neutrophils. In addition, DNA was the scaffold of NETs. DNase I, which degrades DNA, was indicated to be effective at eradicating NETs in vivo (Jenne et al., 2013). Different from Shuai Liu, who instilled DNase I into the trachea (Liu et al., 2016), we injected DNase I via the tail vein to break pulmonary NETs. Due to the anatomy of the lung, tracheal instillation with excessive liquid and air might cause pulmonary edema and emphysema, thus aggravating (rather than alleviating) acute lung injury. DNase I–coated nanoparticles effectively disrupted pulmonary NETs induced by cancer cells (Park et al., 2016), and they might be powerful tools for the treatment of poly I:C- or virus-induced ALI. In the ALI model, lung MPO was significantly correlated with intrapulmonary neutrophil diapedesis (Goldblum et al., 1985). It was shown that MPO is required for NET formation (Metzler et al., 2011). An MPO inhibitor suppressed NET formation and alleviated lung injury, suggesting that the MPO enzyme may be required for the development of poly I:C-induced NETs and ALI. The role of NADPH oxidase in NET formation is controversial. Furthermore, an NADPH inhibitor decreased both poly I:C-induced NET formation and ALI. Total leukocyte infiltration into the BALF, however, was not decreased upon NADPH inhibition. It was clear from the flow cytometry analysis that neutrophils were significantly reduced, and macrophages were slightly increased, which may explain the comparable diapedesis in the BALF for mice treated with or without the NADPH inhibitor.
TLR3, an intracellular receptor for viral RNA, is expressed in diverse cells. Therefore, we hypothesized that mouse neutrophils may directly recognize poly I:C via TLR3. Alternatively, epithelial cells expressing TLR3 rather than neutrophils might be a direct essential responder in TLR3 blocking (Murray et al., 2008). p38 MAPK is one of the downstream molecules of the TLR3 signaling pathway (Berube et al., 2009). In poly I:C-induced ALI, p38 MAPK was activated. Moreover, a p38 inhibitor reduced the formation of NETs and increased the expression of the tight junction protein claudin-5. In LPS-induced ALI, the roles of p38 and its inhibitor were controversial (Arcaroli et al., 2001; Liu et al., 2008). In swine influenza virus-induced ALI, p38 inhibition ameliorated the infiltration of inflammatory cells into the lung (Wei et al., 2014), which was in line with our observations. Of note, NETs may also be beneficial in pathogen killing (Brinkmann and Zychlinsky, 2007) and the resolution of inflammation by degrading inflammatory cytokines (Schauer et al., 2014). Therefore, the roles of NETs in poly I:C-induced ALI may warrant further investigation.
In conclusion, the present study provided direct evidence that poly I:C induced the formation of NETs in ALI. Neutrophils depletion or DNase reduced NETs, thereby alleviating the acute lung injury. Moreover, MPO and NADPH may be involved with poly I:C induced NETs in the lung. TLR3 and p38, the downstream signal molecules of TLR3, are at least partially responsible for poly I:C-induced NETs and ALI.
Availability of Data and Materials
Please contact authors for data requests.
Ethics Statement
This study was approved by the Institutional Animal Care and Use Committee of the Nanjing Medical University.
Author Contributions
YC and MZ designed the experiments. TG and YY performed the animal experiments. FH and XC performed the confocal microscopy experiments. JZ and YL verified the pathological results. YX and HW edited and revised the manuscript. TG and MZ drafted the paper.
Funding
This work was supported by the National Natural Science Foundation of China grant 81671563 (to MZ), the Natural Science Foundation of Jiangsu Province BK20151556 (to MZ), and the Nanjing Medical University key project 2014NJMUZD010 (to MZ).
Conflict of Interest Statement
The authors declare that the research was conducted in the absence of any commercial or financial relationships that could be construed as a potential conflict of interest.
Abbreviations
4-ABH, 4-aminobenzoic hydrazide; ALI, acute lung injury; ARDS, acute respiratory distress syndrome; BALF, bronchoalveolar lavage fluid; DPI, diphenyliodonium chloride; IT, intratracheally; MPO, myeloperoxidase; NETs, neutrophil extracellular traps; SARS, severe acute respiratory syndrome.
References
Aeffner, F., Traylor, Z. P., Yu, E. N., and Davis, I. C. (2011). Double-stranded RNA induces similar pulmonary dysfunction to respiratory syncytial virus in BALB/c mice. Am. J. Physiol. Lung. Cell Mol. Physiol. 301, L99–L109. doi: 10.1152/ajplung.00398.2010
Applequist, S. E., Wallin, R. P., and Ljunggren, H. G. (2002). Variable expression of Toll-like receptor in murine innate and adaptive immune cell lines. Int. Immunol. 14, 1065–1074.
Arcaroli, J., Yum, H. K., Kupfner, J., Park, J. S., Yang, K. Y., and Abraham, E. (2001). Role of p38 MAP kinase in the development of acute lung injury. Clin. Immunol. 101, 211–219.
Bals, R., and Hiemstra, P. S. (2004). Innate immunity in the lung: how epithelial cells fight against respiratory pathogens. Eur. Respir. J. 23, 327–333.
Berube, J., Bourdon, C., Yao, Y., and Rousseau, S. (2009). Distinct intracellular signaling pathways control the synthesis of IL-8 and RANTES in TLR1/TLR2, TLR3 or NOD1 activated human airway epithelial cells. Cell. Signal. 21, 448–456. doi: 10.1016/j.cellsig.2008.12.001
Boe, D. M., Curtis, B. J., Chen, M. M., Ippolito, J. A., and Kovacs, E. J. (2015). Extracellular traps and macrophages: new roles for the versatile phagocyte. J. Leukoc. Biol. 97, 1023–1035. doi: 10.1189/jlb.4RI1014-521R
Brinkmann, V., and Zychlinsky, A. (2007). Beneficial suicide: why neutrophils die to make NETs. Nat. Rev. Microbiol. 5, 577–582.
Butt, Y., Kurdowska, A., and Allen, T. C. (2016). Acute lung injury: a clinical and molecular review. Arch. Pathol. Lab. Med. 140, 345–350. doi: 10.5858/arpa.2015-0519-RA
Chen, W., Sharma, R., Rizzo, A. N., Siegler, J. H., Garcia, J. G. N., and Jacobson, J. R. (2014). Role of claudin-5 in the attenuation of murine acute lung injury by simvastatin. Am. J. Respir. Cell Mol. Biol. 50, 328–336. doi: 10.1165/rcmb.2013-0058OC
Cortjens, B., de Boer, O. J., de Jong, R., Antonis, A. F., Sabogal Pineros, Y. S., Lutter, R., et al. (2016). Neutrophil extracellular traps cause airway obstruction during respiratory syncytial virus disease. J. Pathol. 238, 401–411. doi: 10.1002/path.4660
Daley, J. M., Thomay, A. A., Connolly, M. D., Reichner, J. S., and Albina, J. E. (2008). Use of Ly6G-specific monoclonal antibody to deplete neutrophils in mice. J. Leukoc. Biol. 83, 64–70.
Erickson, S., Schibler, A., Numa, A., Nuthall, G., Yung, M., Pascoe, E., et al. (2007). Acute lung injury in pediatric intensive care in Australia and New Zealand: a prospective, multicenter, observational study. Pediatr. Crit. Care Med. 8, 317–323.
Goldblum, S. E., Wu, K. M., and Jay, M. (1985). Lung myeloperoxidase as a measure of pulmonary leukostasis in rabbits. J. Appl. Physiol. 59, 1978–1985.
Goodman, R. B., Pugin, J., Lee, J. S., and Matthay, M. A. (2003). Cytokine-mediated inflammation in acute lung injury. Cytokine Growth Factor Rev. 14, 523–535.
Hakkim, A., Fuchs, T. A., Martinez, N. E., Hess, S., Prinz, H., Zychlinsky, A., et al. (2011). Activation of the Raf-MEK-ERK pathway is required for neutrophil extracellular trap formation. Nat. Chem. Biol. 7, 75–77. doi: 10.1038/nchembio.496
Hishizawa, M., Mitsuhashi, R., and Ohno, T. (2009). Transfusion-related acute lung injury (TRALI) induced by donor-derived anti-HLA antibodies in aplastic anemia: possible priming effect of granulocyte-colony stimulating factor (G-CSF) on the recipient neutrophils. Intern. Med. 48, 1979–1983.
Jenne, C. N., Wong, C. H., Zemp, F. J., McDonald, B., Rahman, M. M., Forsyth, P. A., et al. (2013). Neutrophils recruited to sites of infection protect from virus challenge by releasing neutrophil extracellular traps. Cell Host Microbe 13, 169–180. doi: 10.1016/j.chom.2013.01.005
Kawai, T., and Akira, S. (2010). The role of pattern-recognition receptors in innate immunity: update on Toll-like receptors. Nat. Immunol. 11, 373–384. doi: 10.1038/ni.1863
Kuba, K., Imai, Y., Rao, S., Gao, H., Guo, F., Guan, B., et al. (2005). A crucial role of angiotensin converting enzyme 2 (ACE2) in SARS coronavirus-induced lung injury. Nat. Med. 11, 875–879.
Li, Y., Xu, J., Shi, W., Chen, C., Shao, Y., Zhu, L., et al. (2016). Mesenchymal stromal cell treatment prevents H9N2 avian influenza virus-induced acute lung injury in mice. Stem Cell Res. Ther. 7:159.
Liu, S., Feng, G., Wang, G. L., and Liu, G. J. (2008). p38MAPK inhibition attenuates LPS-induced acute lung injury involvement of NF-kappaB pathway. Eur. J. Pharmacol. 584, 159–165. doi: 10.1016/j.ejphar.2008.02.009
Liu, S., Su, X., Pan, P., Zhang, L., Hu, Y., Tan, H., et al. (2016). Neutrophil extracellular traps are indirectly triggered by lipopolysaccharide and contribute to acute lung injury. Sci. Rep. 6:37252. doi: 10.1038/srep37252
Looney, M. R., Nguyen, J. X., Hu, Y., Van Ziffle, J. A., Lowell, C. A., and Matthay, M. A. (2009). Platelet depletion and aspirin treatment protect mice in a two-event model of transfusion-related acute lung injury. J. Clin. Invest. 119, 3450–3461. doi: 10.1172/JCI38432
Marcos, V., Zhou, Z., Yildirim, A. O., Bohla, A., Hector, A., Vitkov, L., et al. (2010). CXCR2 mediates NADPH oxidase-independent neutrophil extracellular trap formation in cystic fibrosis airway inflammation. Nat. Med. 16, 1018–1023. doi: 10.1038/nm.2209
McMillen, T. S., Heinecke, J. W., and LeBoeuf, R. C. (2005). Expression of human myeloperoxidase by macrophages promotes atherosclerosis in mice. Circulation 111, 2798–2804.
Metzler, K. D., Fuchs, T. A., Nauseef, W. M., Reumaux, D., Roesler, J., Schulze, I., et al. (2011). Myeloperoxidase is required for neutrophil extracellular trap formation: implications for innate immunity. Blood 117, 953–959. doi: 10.1182/blood-2010-06-290171
Murray, L. A., Knight, D. A., McAlonan, L., Argentieri, R., Joshi, A., Shaheen, F., et al. (2008). Deleterious role of TLR3 during hyperoxia-induced acute lung injury. Am. J. Respir. Crit. Care Med. 178, 1227–1237. doi: 10.1164/rccm.200807-1020OC
Narasaraju, T., Yang, E., Samy, R. P., Ng, H. H., Poh, W. P., Liew, A. A., et al. (2011). Excessive neutrophils and neutrophil extracellular traps contribute to acute lung injury of influenza pneumonitis. Am. J. Pathol. 179, 199–210. doi: 10.1016/j.ajpath.2011.03.013
Narayana Moorthy, A., Narasaraju, T., Rai, P., Perumalsamy, R., Tan, K. B., Wang, S., et al. (2013). In vivo and in vitro studies on the roles of neutrophil extracellular traps during secondary pneumococcal pneumonia after primary pulmonary influenza infection. Front. Immunol. 4:56. doi: 10.3389/fimmu.2013.00056
Park, J., Wysocki, R. W., Amoozgar, Z., Maiorino, L., Fein, M. R., Jorns, J., et al. (2016). Cancer cells induce metastasis-supporting neutrophil extracellular DNA traps. Sci. Transl. Med. 8:361ra138.
Parker, H., Dragunow, M., Hampton, M. B., Kettle, A. J., and Winterbourn, C. C. (2012). Requirements for NADPH oxidase and myeloperoxidase in neutrophil extracellular trap formation differ depending on the stimulus. J. Leukoc. Biol. 92, 841–849. doi: 10.1189/jlb.1211601
Pisegna, S., Pirozzi, G., Piccoli, M., Frati, L., Santoni, A., and Palmieri, G. (2004). p38 MAPK activation controls the TLR3-mediated up-regulation of cytotoxicity and cytokine production in human NK cells. Blood 104, 4157–4164.
Prince, L. R., Whyte, M. K., Sabroe, I., and Parker, L. C. (2011). The role of TLRs in neutrophil activation. Curr. Opin. Pharmacol. 11, 397–403. doi: 10.1016/j.coph.2011.06.007
Ritter, M., Mennerich, D., Weith, A., and Seither, P. (2005). Characterization of Toll-like receptors in primary lung epithelial cells: strong impact of the TLR3 ligand poly(I:C) on the regulation of Toll-like receptors, adaptor proteins and inflammatory response. J. Inflamm. 2:16.
Saitoh, T., Komano, J., Saitoh, Y., Misawa, T., Takahama, M., Kozaki, T., et al. (2012). Neutrophil extracellular traps mediate a host defense response to human immunodeficiency virus-1. Cell Host Microbe 12, 109–116. doi: 10.1016/j.chom.2012.05.015
Schauer, C., Janko, C., Munoz, L. E., Zhao, Y., Kienhofer, D., Frey, B., et al. (2014). Aggregated neutrophil extracellular traps limit inflammation by degrading cytokines and chemokines. Nat. Med. 20, 511–517. doi: 10.1038/nm.3547
Schlingmann, B., Molina, S. A., and Koval, M. (2015). Claudins: gatekeepers of lung epithelial function. Semin. Cell Dev. Biol. 42, 47–57. doi: 10.1016/j.semcdb.2015.04.009
Sharma, P., Azebi, S., England, P., Christensen, T., Moller-Larsen, A., Petersen, T., et al. (2012). Citrullination of histone H3 interferes with HP1-mediated transcriptional repression. PLoS Genet. 8:e1002934. doi: 10.1371/journal.pgen.1002934
Stowell, N. C., Seideman, J., Raymond, H. A., Smalley, K. A., Lamb, R. J., Egenolf, D. D., et al. (2009). Long-term activation of TLR3 by poly(I:C) induces inflammation and impairs lung function in mice. Respir. Res. 10:43. doi: 10.1186/1465-9921-10-43
Sun, D., Zhang, M., Liu, G., Wu, H., Li, C., Zhou, H., et al. (2016). Intravascular clearance of disseminating Cryptococcus neoformans in the brain can be improved by enhancing neutrophil recruitment in mice. Eur. J. Immunol. 46, 1704–1714. doi: 10.1002/eji.201546239
Sun, Y., Li, C., Shu, Y., Ju, X., Zou, Z., Wang, H., et al. (2012). Inhibition of autophagy ameliorates acute lung injury caused by avian influenza A H5N1 infection. Sci. Signal. 5:ra16. doi: 10.1126/scisignal.2001931
Tadie, J. M., Bae, H. B., Jiang, S., Park, D. W., Bell, C. P., Yang, H., et al. (2013). HMGB1 promotes neutrophil extracellular trap formation through interactions with Toll-like receptor 4. Am. J. Physiol. Lung. Cell Mol. Physiol. 304,L342–L349. doi: 10.1152/ajplung.00151.2012
Tisoncik, J. R., Korth, M. J., Simmons, C. P., Farrar, J., Martin, T. R., and Katze, M. G. (2012). Into the eye of the cytokine storm. Microbiol. Mol. Biol. Rev. 76, 16–32. doi: 10.1128/MMBR.05015-11
Wang, J. P., Bowen, G. N., Padden, C., Cerny, A., Finberg, R. W., Newburger, P. E., et al. (2008). Toll-like receptor-mediated activation of neutrophils by influenza A virus. Blood 112, 2028–2034. doi: 10.1182/blood-2008-01-132860
Wang, Y., Li, M., Stadler, S., Correll, S., Li, P., Wang, D., et al. (2009). Histone hypercitrullination mediates chromatin decondensation and neutrophil extracellular trap formation. J. Cell Biol. 184, 205–213. doi: 10.1083/jcb.200806072
Wei, D., Huang, Z. H., Zhang, R. H., Wang, C. L., Xu, M. J., Liu, B. J., et al. (2014). Roles of p38 MAPK in the regulation of the inflammatory response to swine influenza virus-induced acute lung injury in mice. Acta Virol. 58, 374–379.
Wheeler, A. P., and Bernard, G. R. (2007). Acute lung injury and the acute respiratory distress syndrome: a clinical review. Lancet 369, 1553–1564. doi: 10.1016/S0140-6736(07)60604-7
Yildiz, C., Palaniyar, N., Otulakowski, G., Khan, M. A., Post, M., Kuebler, W. M., et al. (2015). Mechanical ventilation induces neutrophil extracellular trap formation. Anesthesiology 122, 864–875. doi: 10.1097/ALN.0000000000000605
Zawrotniak, M., and Rapala-Kozik, M. (2013). Neutrophil extracellular traps (NETs) - formation and implications. Acta Biochim. Pol. 60, 277–284.
Zhang, M., Sun, D., Liu, G., Wu, H., Zhou, H., and Shi, M. (2016). Real-time in vivo imaging reveals the ability of neutrophils to remove Cryptococcus neoformans directly from the brain vasculature. J. Leukoc. Biol. 99, 467–473. doi: 10.1189/jlb.4AB0715-281R
Keywords: poly I:C, acute lung injury, neutrophil extracellular traps, p38, claudin-5
Citation: Gan T, Yang Y, Hu F, Chen X, Zhou J, Li Y, Xu Y, Wang H, Chen Y and Zhang M (2018) TLR3 Regulated Poly I:C-Induced Neutrophil Extracellular Traps and Acute Lung Injury Partly Through p38 MAP Kinase. Front. Microbiol. 9:3174. doi: 10.3389/fmicb.2018.03174
Received: 21 April 2018; Accepted: 07 December 2018;
Published: 21 December 2018.
Edited by:
Tamás Laskay, Universität zu Lübeck, GermanyReviewed by:
Leonardo H. Travassos, Instituto de Biofísica Carlos Chagas Filho (IBCCF), BrazilHridayesh Prakash, Amity University, Noida, India
Copyright © 2018 Gan, Yang, Hu, Chen, Zhou, Li, Xu, Wang, Chen and Zhang. This is an open-access article distributed under the terms of the Creative Commons Attribution License (CC BY). The use, distribution or reproduction in other forums is permitted, provided the original author(s) and the copyright owner(s) are credited and that the original publication in this journal is cited, in accordance with accepted academic practice. No use, distribution or reproduction is permitted which does not comply with these terms.
*Correspondence: Yu Chen, yuchen_njmu@163.com Mingshun Zhang, mingshunzhang@njmu.edu.cn
†These authors have contributed equally to this work