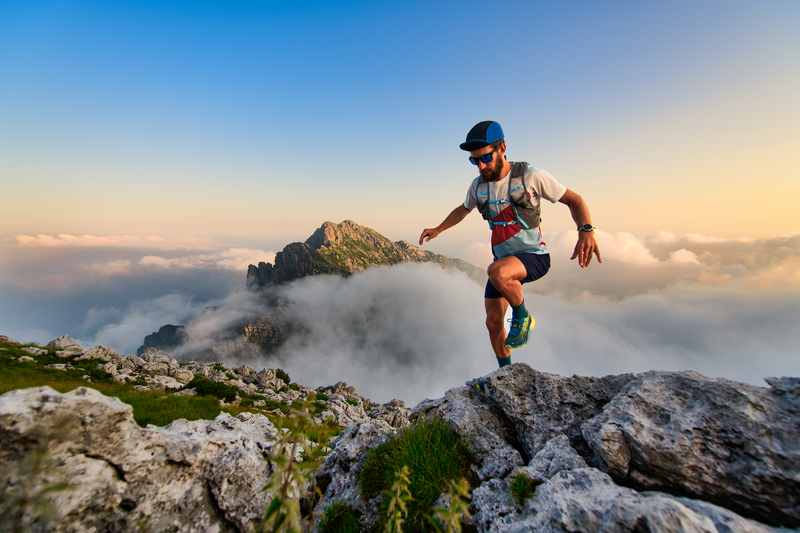
95% of researchers rate our articles as excellent or good
Learn more about the work of our research integrity team to safeguard the quality of each article we publish.
Find out more
ORIGINAL RESEARCH article
Front. Microbiol. , 03 December 2018
Sec. Antimicrobials, Resistance and Chemotherapy
Volume 9 - 2018 | https://doi.org/10.3389/fmicb.2018.02892
Fungal infections caused by Candida albicans and non-albicans Candida [NAC] species are becoming a growing threat in immunodeficient population, people with long-term antibiotic treatment and patients enduring kinds of catheter intervention. The resistance to one or more than one conventional antifungal agents contributes greatly to the widespread propagation of Candida infections. The severity of fungal infection requires the discovery of novel antimycotics and the extensive application of combination strategy. In this study, a group of Candida standard and clinical strains including C. albicans as well as several NAC species were employed to evaluate the antifungal potentials of palmatine (PAL) alone and in combination with fluconazole (FLC)/itraconazole (ITR) by microdilution method, checkerboard assay, gram staining, spot assay, and rhodamine 6G efflux test. Subsequently, the expressions of transporter-related genes, namely CDR1, CDR2, MDR1, and FLU1 for C. albicans, CDR1 and MDR1 for Candida tropicalis and Candida parapsilosis, ABC1 and ABC2 for Candida krusei, CDR1, CDR2, and SNQ2 for Candida glabrata were analyzed by qRT-PCR. The susceptibility test showed that PAL presented strong synergism with FLC and ITR with fractional inhibitory concentration index (FICI) in a range of 0.0049–0.75 for PAL+FLC and 0.0059–0.3125 for PAL+ITR in planktonic cells, 0.125–0.375 for PAL+FLC and 0.0938–0.3125 for PAL+ITR in biofilms. The susceptibility results were also confirmed by gram staining and spot assay. After combinations, a vast quantity of rhodamine 6G could not be pumped out as considerably intracellular red fluorescence was accumulated. Meanwhile, the expressions of efflux-associated genes were evaluated and presented varying degrees of inhibition. These results indicated that PAL was a decent antifungal synergist to promote the antifungal efficacy of azoles (such as FLC and ITR), and the underlying antifungal mechanism might be linked with the inhibition of efflux pumps and the elevation of intracellular drug content.
Candida-related infections are becoming a universal threat to the health of human who usually undergo immunosuppressive therapy or aggressive medical intervention. The most commonly found Candida species is C. albicans, an amicable resident in human intestinal tract and genital mucosal surface which can switch into an opportunistic pathogen when external environmental cues are altered (such as pH, temperature, immunocompetence, etc.) (Poulain, 2013; Sardi et al., 2013). With the expanding spread of antifungal agents and the frequent use of broad-spectrum immune-inhibitors, emerging non-albicans Candida (NAC) species including C. glabrata, C. parapsilosis, C. tropicalis, C. krusei and C. guilliermondii are causing increasingly high morbidity and mortality. Besides C. albicans accounting for most cases of invasive candidosis (ca. 35–55%) (Messer et al., 2006), the above-mentioned NAC are also capable to cause a series of disturbs ranging from mildly superficial mucosal discomforts to deadly disseminated bloodstream and deep-seated tissue infections. The candidosis incidence attributed to NAC species are reported to be varying in a range of 14–21% for C. glabrata, 15–23% C. parapsilosis, 20–45% for C. tropicalis, 2.7% for C. krusei, and 0.6–3.7% for C. guilliermondii depending on infection site and geography (Pfaller et al., 2006, 2014; Savini et al., 2011; Silva et al., 2012; Rodrigues et al., 2014).
The relatively high incidence rates of C. albicans and NAC species are supposed to be closely related with their recalcitrant resistance to conventional antifungal agents including, for example, azoles, polyenes, echinocandins and the unique phenotype biofilms (Silva et al., 2012; Zavrel and White, 2015). Fluconazole (FLC) and itraconazole (ITR) are two commonly prescribed antifungal drugs which exert decent inhibitions on most pathogenic Candida species by interrupting the biosynthesis of the fungal-specific membrane sterol ergosterol. However, C. glabrata, C. krusei, and C. guilliermondii have been revealed to be inherently resistant to azoles (Sanguinetti et al., 2015). Although less primary resistances to FLC are reported in C. albicans (1.4%), C. parapsilosis (3.6%) and C. tropicalis (4.1%), it should be noted that an ascending tendency of acquired resistance to azoles are emerging in the three Candida species which have been recorded to take up 48% of resistant isolates in a retrospective case-comparator study (Oxman et al., 2010; Pfaller et al., 2010b). Of increasing concern are the frequency of multidrug resistant isolates of C. albicans and NAC which display cross-resistance to azoles in especially high-risk patients receiving antifungal prophylaxis (Pfaller et al., 2010a).
Facing increasing severity of global resistance of Candida species recovered clinically, the lag of effective drugs with antifungal purpose alone and/or in combination with traditional antimycotics is worrying. Palmatine (PAL) is an isoquinoline alkaloid of medicinal plant drug that can be isolated from Rhizoma Coptidis and Mahonia aquifolium. PAL exhibits diverse biochemical and pharmacological functions on jaundice, dysentery, hypertension, inflammation, bacterial, virus and fungal infections with less host toxicity (Vollekova et al., 2003; Ishikawa et al., 2016; Wu et al., 2016). Xiao et al. (2015) reported that PAL in combination with berberine (BER) presented remarkably strong antifungal activities to treat Microsporum canis-induced dermatitis in rabbits. Besides, increasing evidence also favored the anti-Candida effects of PAL, inferring that PAL might deserve for further reversion study of resistance especially to azoles (such as FLC and ITR) and underlying mechanism, for example, related with efflux pumps.
This study aims to study the synergism of PAL with FLC and ITR against C. albicans SC5314 and several FLC-resistant clinical isolates as well as five NAC standard isolates including C. glabrata, C. parapsilosis, C. tropicalis, C. krusei, and C. guilliermondii by susceptibility test, spot assay and gram staining. The antifungal effects of PAL alone and in combination with FLC or ITR on rhodamine 6G efflux and the expressions of efflux pump genes are also examined.
C. albicans SC5314 was a gift of Prof. Yuanying Jiang, School of Pharmacy, Second Military Medical University (Shanghai, China). Six clinical C. albicans isolates including Z3044, Z2003, Z1402, Z1407, Z826, and Z1309 were donated by Huaiwei Lu, Clinical Laboratory, Anhui Provincial Hospital (Hefei, China). C. parapsilosis ATCC22019 and C. tropicalis GDM2.147 were purchased from Guangdong Culture Collection Center (Guangzhou, China). C. glabrata ATCC2340 and C. krusei ATCC1182 were obtained from Bianzhen Biotech. Co. (Nanjing, China). C. guilliermondii ATCC6260 were acquired from Shfeng Biotech. Co. (Shanghai, China). All of stock cultures of these strains were routinely maintained in Sabouraud’s agar and were propagated in Liquid Sabouraud Medium (Hope Biotech Co., Qingdao, China) at 37°C for 12–16 h till the strains reached the exponential growth phase. The revived Candida cells were pooled by centrifugation of 3000 g. After washing twice by sterile phosphate-buffered saline (PBS, Leagene, Beijing, China), the fungal cells were resuspended in RPMI-1640 medium (Invitrogen, Carlsbad, CA, United States) without pH adjustment and adjusted to a defined cell density using a hemocytometer prior for following tests.
The initial inoculum was adjusted to 2 × 103 CFU/mL. The minimum inhibitory concentrations (MICs) of Pal, FLC, and ITR were performed in a 96-well flat-bottomed microplate (Corning, Corning, NA, United States) by microdilution method. The concentrations of the tested drugs were serially twofold diluted in a range of 2–1024 μg/mL for Pal, 0.125–1024 μg/mL for FLC and 0.125–512 μg/mL for ITR, respectively. The fungal cells were incubated with the drugs used at 37°C for 48 h. The interaction of two drugs was carried out by checkerboard assay with initial inoculum of 2 × 106 CFU/mL at 37°C for 48 h according to Clinical and Laboratory Standards Institute (CLSI) M27-A3 (CLSI, 2008). The concentrations of the tested drugs were also serially twofold diluted in a range of 0.125–512 μg/mL for Pal, 0.015625–16 μg/mL for FLC and 0.03125–8 μg/mL for ITR, respectively. The MIC80 was determined as the drug concentration that inhibited 80% of planktonic fungal cells by comparing the optical density (OD) with that of drug-free control at 630 nm. The fractional inhibitory concentration index (FICI) was calculated as (MIC-PAL in combination/MIC-PAL alone) plus [MIC-FLC (ITR) in combination/MIC-FLC (ITR) alone], in which synergism was interpreted as FICI ≤ 0.5, indifference was defined as 0.5 < FICI < 4.0, and antagonism was FICI ≥ 4.0 (Odds, 2003).
The initial fungal cells were set at 2 × 106 CFU/mL. For sessile MIC (SMIC), the tested concentrations ranged from 1 to 1024 μg/mL for PAL, FLC, and ITR. In checkerboard assay, the final concentrations of the three drugs were modified in a range of 0.03125–512 μg/mL. The SMIC80 was defined as the drug concentration that inhibited 80% of fungal biofilm cells by comparing the metabolic activity with that of drug-free control at 490 nm using XTT method. The XTT procedures were the same as our previous work (Shao et al., 2015). The sessile fractional inhibitory concentration index (sFICI) was calculated as (SMIC-PAL in combination/SMIC-PAL alone) plus [SMIC-FLC (ITR) in combination/SMIC-FLC (ITR) alone], in which synergism was interpreted as sFICI ≤ 0.5, indifference was defined as 0.5 < sFICI < 4.0, and antagonism was sFICI ≥ 4.0 (Shao et al., 2014).
The procedures were described as a previous report with less modifications (Mukhopadhyay et al., 2002). Briefly, the revived fungal cells were resuspended and 10-fold diluted by RPMI-1640 medium to three final concentrations of 1 × 102–1 × 104 CFU/mL. Five microliters of the prepared serial dilutions of each Candida culture was spotted onto YPD plates in the absence (control) and presence of PAL, FLC, PAL+FLC at the concentrations showing synergism. Growth differences were recorded following incubation of the plates for 48 h at 37°C.
One hundred microliter strain medium (2 × 106 CFU/mL) was co-incubated with the same volumes of PAL and/or FLC at the final concentrations showing synergism in susceptibility tests in a 96-well flat-bottomed microplate at 37°C for 6 h. The supernatant was then discarded, resuspended with 100 μL PBS, stained with Gram solution (Leagene, Beijing, China), observed by oil lens (×1000) and photographed by OLYMPUS IX51 (Tokyo, Japan).
The experiment was performed according to the procedures described in one of our previous work with few modifications (Shao et al., 2016). Briefly, two milliliters of fungal culture was firstly adjusted to 2 × 106 CFU/mL with RPMI 1640, and then co-incubated with the same volume of PAL, FLC, and PAL+FLC at the concentrations showing synergism at 37°C for 24 h. The supernatant was discarded after 2000 g of centrifugation. The remnants were mixed with 2 mL sterile PBS for 2 h of incubation at 37°C in an orbital shaker (ca. 200 rpm). The final concentration of 10 μM rhodamine-6G (150 μL) was added for 2 h of incubation at 37°C without light. The supernatant was discarded after 3000 g of centrifugation. The pellets were washed three times by sterile PBS. The glucose required for rhodamine 6G efflux was dissolved in PBS to the final concentration of 2 mM. The suspension was centrifuged at 3000 g, and 100 μL of supernatant was visualized at the excitation wavelength of 525 nm and the emission wavelength of 550 nm by an inverted fluorescence microscope IX71 (Olympus, Tokyo, Japan).
The procedures of qRT-PCR analysis were described in one of our previous study with small modifications (Shao et al., 2016). Briefly, 1 × 106 CFU/mL of fungal culture was incubated for 24 h at 37°C. The fungal cells were then collected by 3000 g and total RNA samples were extracted according to the instructions of MagExtractor-RNA kit (Toyobo, Tokyo, Japan). Six microliters of extracted total RNA was incubated with 2 μL 4× DNA Master I (containing gDNA Remover) and 2 μL 5RT-Master Mix II, and reverse-transcribed into cDNA as recommended by ReverTra Ace qPCR RT Master Mix with gDNA Remover kit (Toyobo, Tokyo, Japan) with procedures as follows: 65°C for 5 min and 4°C for 1 min for initial RNA denaturation, followed by 37°C for 15 min, 50°C for 5 min, and 4°C for 1 min. The prepared cDNA was diluted 10-fold prior to the use for RT-PCR. Primers for C. albicans (Table 1) were designed by Primer Premier 5.0 and synthesized by Sangon Biotech (Shanghai, China), while those for C. tropicalis, C. parapsilosis, C. krusei, and C. glabrata were originated from the studies published previously (Sanguinetti et al., 2005; Ricardo et al., 2014; Souza et al., 2015; Shi et al., 2017). Twenty-five microliters of real time PCR mixture was freshly prepared containing 12.5 μL of 2× SYBR Green Realtime PCR, 1 μL of PCR Forward Primer, 1 μL of PCR Reverse Primer, 0.5 μL of cDNA, and 10 μL of ddH2O. The PCR process were performed on ABI7000 fluorescent quantitative PCR system (Applied Biosystem) with following cycles: 95°C for 60 s for pre-denaturation alone with 95°C for 15 s, 55°C for 15 s, 72°C for 45 s for a total of 40 cycles. All data were normalized to housekeeping gene ACT1 as the internal reference gene. The relative target-gene expression was calculated as a fold change of 2−ΔΔCt value, in which ΔCt = Cttargetgene - Ctinternal referencegenes as previously described (Livak and Schmittgen, 2001).
All experiments were performed in triplicate in three individual workdays. The results were recorded as mean ± standard deviation (n = 3) and calculated by SPSS 17.0 (SPSS Inc., Chicago, IL, United States). The data among groups were analyzed by one-way ANOVA with least significance difference (LSD) method, in which p < 0.05 was considered as statistically significant.
In planktonic cells, the susceptibility test showed that the MICs of PAL were in a range of 128–512 μg/mL against C. albicans SC5314 and six clinical isolates and 64 ≥ 1024 μg/mL against the five NAC isolates. The MICs of PAL ranged from 0.5 to 64 μg/mL in C. albicans isolates and 4 to 128 μg/mL in the five NAC isolates after in combination with FLC compared with the MICs alone (Table 2). Likewise, the MICs of PAL altered between 0.5–16 μg/mL in C. albicans isolates and 2–32 μg/mL in the five NAC isolates following simultaneous use of ITR compared with the MICs alone (Table 3). The FICIs were, respectively, of 0.0049–0.75 for PAL plus FLC and 0.0059–0.3125 for PAL plus ITR, reflecting the action mode of PAL+FLC/ITR could be defined as synergism in most isolates tested except C. albicans SC5314 and C. tropicalis GDM 2.147 in PAL plus FLC (Tables 2, 3).
TABLE 2. Interactions of PAL alone/in combination with FLC and PAL alone/in combination with ITR against planktonic cells of Candida spp.
TABLE 3. Interactions of PAL alone/in combination with FLC and PAL alone/in combination with ITR against biofilm cells of Candida spp.
A number of studies evaluated the MICs of PAL against a series of Candida species and found that they were of ≥500 μg/mL against C. albicans, C. glabrata, C. tropicalis, C. krusei, and C. guilliermondii, except C. parapsilosis which was inhibited at 15.6 μg/mL (Iwasa et al., 1998; Park et al., 1999; Vollekova et al., 2003). In this study, the MICs of PAL alone were lower than or approximate to 500 μg/mL against most Candida isolates (11/12) aside from C. guilliermondii ATCC6260 (MIC > 1024 μg/mL). This discrepancy might be due to the limited purity of PAL that was extracted from effective part of medicinal plants in those previous studies compared with ours. Intriguingly, the presence of PAL improved tremendously the susceptibility of Candida species, especially FLC-resistant C. albicans isolates, to FLC by even more than 1000-fold increase. Meantime, we also observed that the addition PAL promoted significantly the efficacy of ITR with an elevation of 2- to 64-fold compared with ITR used alone. These results showed the effectiveness of PAL in combination with FLC and ITR to inhibit planktonic cells of Candida strains.
The biofilm is a self-protected life-mode of Candida species different from the planktonic state with complex structure and powerful resistance to most antimycotics. The formation of biofilm therefore is supposed to be a crucial factor to render the incapability of conventional antifungal agents (Tobudic et al., 2012; Taff et al., 2013). Prior to this study, the anti-biofilm potential of PAL and its synergistic effect with azoles has not been documented to our knowledge. Herein, the SMIC80 were above 1024 μg/mL for PAL, FLC, and ITR being used individually in most tested Candida species, while decreased significantly by 4- to 128-fold for PAL and 4- to 32-fold for FLC, respectively, with FICI in a range of 0.125–0.375 by PAL plus FLC, and reduced remarkably by 8- to 512-fold for PAL and 8- to 256-fold for ITR, respectively, with FICI ranging from 0.0938 to 0.3125 by PAL plus ITR. The pairing of PAL plus FLC showed synergism for all of the tested strains, and so did that of PAL plus ITR except C. glabrata ATCC2340 (Table 3). These results demonstrated that the concomitant uses of PAL and FLC/ITR were applicable in the removal of Candida biofilms.
In the following gram staining test and spot assay, the strong synergisms of PAL plus FLC/ITR were also confirmed. Without drug treatment, as shown, quantities of blastoconidia were observed in the tested strains including C. glabrata ATCC2340, C. parapsilosis ATCC22019, C. guilliermondii ATCC6260, C. krusei ATCC1182, and C. tropicalis GDM 2.147. Apart from C. krusei ATCC1182 with very less pseudohyphae, there were absence of hyphal or pseudohyphal forms in the other three isolates. These observations were consistent with the descriptions in several previous literatures, in which it is believed that C. glabrata had entirely no presence of pseudohyphae and hyphae, while the other three strains occasionally displayed pseudohyphae in a strain-dependent manner but were deemed to be unable to generate hyphae (Samaranayake and Samaranayake, 1994; Savini et al., 2011; Silva et al., 2012; Rodrigues et al., 2014). In comparison, C. albicans exhibited abundant hypha with less yeast cells in drug-free control. Following the treatments of PAL and FLC/ITR, the hyphal and blast conidia cells were remarkably reduced (Figures 1A,B). With 10-fold of series dilution, the combination of PAL with FLC/ITR also demonstrated their strong synergistic effects in the spot assay (Figures 2A,B).
FIGURE 1. Gram staining of Candida species incubated with (A) PAL, FLC, PAL+FLC, and (B) PAL, ITR, PAL+ITR at the concentrations of their MICs showing synergism. The initial inoculum was newly prepared at 2 × 106 CFU/mL. The drug-free strain-containing medium was set as the control. Oil lens: ×1000.
FIGURE 2. Spot assay of Candida species spreading on YPD agar containing (A) PAL, FLC, PAL+FLC, and (B) PAL, ITR, PAL+ITR at the concentrations of their MICs showing synergism. The initial inoculum was 10-fold diluted in a range of 1 × 102–1 × 104 CFU/mL. The drug-free strain-containing medium was set as the control.
The rhodamine 6G is a commonly used fluorescent dye with the same transporters as azoles in yeast (Maesaki et al., 1999). This assay measured the efflux activities in the selected Candida species being incubated with PAL and/or FLC/ITR at the concentrations showing synergism in terms of intracellular fluorescence (red color). As shown, drug-free and single drug induced no or less accumulation of intracellular rhodamine 6G, whereas concomitant use of PAL and FLC resulted in vast appearance of red fluorescence inferring there was a close association of the antifungal effects of PAL and/or FLC/ITR with the normal function of efflux pumps in the Candida species tested (Figures 3A,B).
FIGURE 3. Rhodamine 6G efflux in Candida species in incubation with (A) PAL, ITR, PAL+ITR, and (B) PAL, FLC, PAL+FLC at the concentrations of their MICs showing synergism. The initial inoculum was newly prepared at 2 × 106 CFU/mL. The drug-free strain-containing medium was set as the control. The excitation wavelength and the emission wavelength were of 525 and 550 nm, respectively. The micrographs were recorded in bright field and dark field with a magnification of 200.
It is known that two main classes of efflux pumps are considered to mediate the resistance of Candida species, i.e., ABC superfamilies and MFS pumps. The former is driven by ATP hydrolysis, and the latter employs the proton-motive force across the membrane (Cannon et al., 2009). Among the Candida species, both transporters are the most extensively studied in C. albicans. Current evidence indicated that CaCDR1, CaCDR2, CaMDR1, and CaFLU1 had specific substrates of conventional antifungal drugs. Overexpressions of CaCDR1 and CaCDR2 are correlative to high-frequency resistance in clinical isolates. CaCDR1 single mutant was hypersensitive to azoles, and CDR1CDR2 double mutant became more susceptible to azoles than CaCDR1 single mutant (Sanglard et al., 1996, 1997). However, accumulating reports demonstrated that CaCDR1 may play a more important role than CaCDR2 in azole resistance (Andes et al., 2006a,b; Holmes et al., 2008). Considering significance of CDR1 and CDR2 versus MDR1 and FLU1 in the resistance of C. albicans to azoles, it should be noted that CaCdr1p and CaCdr2p possess more azole substrates than CaMdr1p which is relatively specific for FLC (Cannon et al., 2009). Of interest, two independent pathways might be possibly present to control the levels of CDR1, CDR2, and MDR1 in azole-resistant C. albicans as the expressions of CDR1 and CDR2 were upregulated in some FLC-resistant strains, while the expression of MDR1 was upregulated in other strains (Cannon et al., 2009). The expression of FLU1 seemed to be not required in azole resistance of C. albicans as FLU1 mutant affected FLC susceptibility negligibly. Basically, the association of azole resistance with the levels of ABC superfamilies is comparatively stronger than MFS pumps (Cannon et al., 2009). Our PCR results revealed comparable decreases of CDR1 and CDR2 and considerable reductions of the four genes after treatments of PAL and PAL plus FLC (Figure 4A). We evaluated the expressions of CDR1 and CDR2 belonging to ABC superfamilies, MDR1 and FLU1 members of MFS pumps in a clinical isolate C. albicans Z3044 exposed to PAL and/or FLC and the standard reference strain C. albicans SC5314 treated with PAL and/or ITR. The results showed that the use of PAL alone with FLC inhibited the expressions of the four genes significantly (p < 0.05, Figure 4A), while the use of PAL alone with ITR only restrained the expressions of CDR2 and MDR1 (p < 0.01, p < 0.05, Figure 4B). It is speculated that the contributions of CDR1, CDR2, MDR1, and FLU1 to azole resistance are dependent on many factors including at least incubation conditions and strain types in C. albicans.
FIGURE 4. Expressions of efflux-associated genes in Candida species in incubation with (A) PAL, FLC, PAL+FLC, and (B) PAL, ITR, PAL+ITR at the concentrations of their MICs showing synergism. The initial inoculum was newly prepared at 1 × 106 CFU/mL. The drug-free strain-containing medium was set as the control. ∗p < 0.05; ∗∗p < 0.01; ∗∗∗p < 0.001; compared with the control.
We further evaluated the combined effects of PAL and/or FLC/ITR on the expressions of several efflux-associated genes in C. parapsilosis ATCC22019, C. glabrata ATCC2340, C. krusei ATCC1182, and C. tropicalis GDM2.147. Our results revealed that (i) PAL plus FLC/ITR caused a great impact on CDR1 and MDR1 (p < 0.05, p < 0.01) in C. parapsilosis ATCC22019; (ii) PAL plus FLC only affected the expressions of CDR2 (p < 0.01), while PAL plus ITR decreased the expressions of CDR1 and CDR2 dramatically (p < 0.01, p < 0.01) in C. glabrata ATCC2340; (iii) the expressions of ABC1 and ABC2 were lowered (p < 0.01, p < 0.05) after the use of PAL+ITR, while only ABC2 were suppressed (p < 0.05) in the case of PAL+FLC in C. krusei ATCC1182; (iv) the combination of PAL and ITR only influenced MDR1 (p < 0.05) in C. tropicalis GDM2.147 (Figures 4A,B).
[In NAC species, ABC superfamilies and MFS pumps are still the most studied efflux pumps in azole resistance, but different from those in C. albicans in types and contributions. The comprehensive knowledge of efflux pumps in NAC species is summarized in several literatures (Cannon et al., 2009; Whaley et al., 2017). In a study of elucidating FLC-resistance mechanism in C. parapsilosis, CDR1 and MDR1 were found to be overexpressed in 16 FLC-resistant isolates and 3 other resistant ones, respectively (Berkow et al., 2015). Several reports also observed the close relationship of CDR1 and MDR1 with FLC-resistance (Grossman et al., 2015; Souza et al., 2015). However, it was also supposed that overexpressions of CDR1 and MDR1 might not be the sole reason responsible for FLC-resistance in C. parapsilosis (Berkow et al., 2015). In C. glabrata, there are three ABC transporters identified to be linked directly to azole resistance, namely CDR1, CDR2 (PDH1) and SNQ2 (Sanglard et al., 1999, 2001; Torelli et al., 2008). Whereas MFS transporters might exert much less impact on azole resistance in C. glabrata (Costa et al., 2016). C. krusei has an inherent instinct of resistance to FLC. This innate resistance mechanism might be connected with ATP-binding cassette transporter Abc1p (Katiyar and Edlind, 2001; Lamping et al., 2009). In addition, resistance to ITR was attributed to reduced intracellular drug content and the overexpression of efflux pump Abc2p (Ricardo et al., 2014; He et al., 2015). As with C. tropicalis, the expressions of CDR1 and MDR1 were remarkably upregulated in azole-resistant strains (Barchiesi et al., 2000). We also found a critical role of CDR1 and MDR1 in berberine-treated C. tropicalis isolates (Shi et al., 2017). In our study, accumulated intracellular rhodamine 6G was an intense sign of the involvement of efflux pumps (Figures 3A,B). Subsequent qRT-PCR analyses confirmed the close relationship of most efflux-associated genes tested with the synergistic antifungal mechanisms of PAL plus FLC/ITR due to their varying degrees of inhibition (Figures 4A,B). Nevertheless, it should be noted that azole resistance is a multifactorial event with more than one gene implicated (such as ERG11), and more studies are necessarily performed to elucidate the involvements of these genes used in azole resistance and cross-resistance.
Growing evidence exhibit the huge antifungal potential of quantities of medicinal plant drugs which are easily accessible and less toxic to human with decent antifungal activities and reasonable prices (Li et al., 2013; da Silva et al., 2014; Singh et al., 2015; Sun et al., 2015; Shao et al., 2017). Aside from a few herbal drugs showing relatively low MIC alone (such as berberine and sodium houttuyfonate ≤64 μg/mL), the anti-Candida effect is relatively weak in a majority of plant drugs (usually MIC > 256 μg/mL). The case is also not changed for other non-conventional antifungal agents which are converted to antifungal purpose (Zhou et al., 2012; da Silva et al., 2013; Letscher-Bru et al., 2013; Shahzad et al., 2014). To strengthen the efficacy of individual drug for antifungal usage and traditional antimycotics in the treatment of Candida resistant strains, the combination strategy is a reliable and feasible approach to inspire the antifungal potentials of the two drugs used which can dramatically lower the MIC of each one. Further, the combination method is also a widely used and effective way in the removal of Candida biofilms. Lu et al. (2017) made a deep and all-sided review on this field. This study is another successful example of drug combination, by which the relative high MICs and SMICs of PAL and FLC alone were dramatically reduced.
We confirmed the antifungal effects of PAL alone and in combination with FLC/ITR on planktonic and biofilm cells of C. albicans as well as NAC species. The gram staining and spot assay results further demonstrated PAL was intensely synergistic with FLC/ITR against most of the tested strains. The rhodamine 6G and PCR results inferred that there might be a close association of the antifungal mechanism of PAL plus FLC/ITR with efflux pumps. This study indicated PAL might be a promising synergist against cross-resistance to FLC and ITR in Candida strains.
TW, WD, QL, and GS performed the experiments. DW reviewed the whole manuscript. JS and CW devised the experiments. JS wrote most of the main text. JS, WD, and QL also together arranged the Tables and Figures.
This work was supported by National Natural Science Foundation of China to JS (81603167) and CW (81573725 and 81774034), Outstanding Young Talents Key Project of Anhui Institution of Higher Education to JS (gxyqZD2018054), Anhui Public Welfare Technology Application Research Project to TW (1604f0804031), Key Project of Natural Science Foundation of Anhui University to TW (KJ2017A287), Discipline Construction Key Program of Anhui University of Chinese Medicine to CW (DC18100042), and Graduate Science and Technology Innovation Fund of Anhui University of Chinese Medicine to WD (2017YB08).
The authors declare that the research was conducted in the absence of any commercial or financial relationships that could be construed as a potential conflict of interest.
Andes, D., Forrest, A., Lepak, A., Nett, J., Marchillo, K., and Lincoln, L. (2006a). Impact of antimicrobial dosing regimen on evolution of drug resistance in vivo: fluconazole and Candida albicans. Antimicrob. Agents Chemother. 50, 2374–2383. doi: 10.1128/AAC.01053-05
Andes, D., Lepak, A., Nett, J., Lincoln, L., and Marchillo, K. (2006b). In vivo fluconazole pharmacodynamics and resistance development in a previously susceptible Candida albicans population examined by microbiologic and transcriptional profiling. Antimicrob. Agents Chemother. 50,2384–2394.
Barchiesi, F., Calabrese, D., Sanglard, D., Falconi Di Francesco, L., Caselli, F., Giannini, D., et al. (2000). Experimental induction of fluconazole resistance in Candida tropicalis ATCC 750. Antimicrob. Agents Chemother. 44, 1578–1584. doi: 10.1128/AAC.44.6.1578-1584.2000
Berkow, E. L., Manigaba, K., Parker, J. E., Barker, K. S., Kelly, S. L., and Rogers, P. D. (2015). Multidrug transporters and alterations in sterol biosynthesis contribute to azole antifungal resistance in Candida parapsilosis. Antimicrob. Agents Chemother. 59, 5942–5950. doi: 10.1128/AAC.01358-15
Cannon, R. D., Lamping, E., Holmes, A. R., Niimi, K., Baret, P. V., Keniya, M. V., et al. (2009). Efflux-mediated antifungal drug resistance. Clin. Microbiol. Rev. 22, 291–321. doi: 10.1128/CMR.00051-08
CLSI (2008). Reference Method for Broth Dilution Antifungal Susceptibility Testing of Yeasts-Third Edition: Approved Standard M27-A3. Wayne, PA: Clinical and Laboratory Standards Institute.
Costa, C., Ribeiro, J., Miranda, I. M., Silva-Dias, A., Cavalheiro, M., Costa-De-Oliveira, S., et al. (2016). Clotrimazole drug resistance in Candida glabrata clinical isolates correlates with increased expression of the drug: H(+) antiporters CgAqr1, CgTpo1_1, CgTpo3, and CgQdr2. Front. Microbiol. 7:526. doi: 10.3389/fmicb.2016.00526
da Silva, C. R., De Andrade Neto, J. B., De Sousa Campos, R., Figueiredo, N. S., Sampaio, L. S., Magalhaes, H. I., et al. (2014). Synergistic effect of the flavonoid catechin, quercetin, or epigallocatechin gallate with fluconazole induces apoptosis in Candida tropicalis resistant to fluconazole. Antimicrob. Agents Chemother. 58, 1468–1478. doi: 10.1128/AAC.00651-13
da Silva, C. R., De Andrade Neto, J. B., Sidrim, J. J., Angelo, M. R., Magalhaes, H. I., Cavalcanti, B. C., et al. (2013). Synergistic effects of amiodarone and fluconazole on Candida tropicalis resistant to fluconazole. Antimicrob. Agents Chemother. 57, 1691–1700. doi: 10.1128/AAC.00966-12
Grossman, N. T., Pham, C. D., Cleveland, A. A., and Lockhart, S. R. (2015). Molecular mechanisms of fluconazole resistance in Candida parapsilosis isolates from a U.S. Surveillance system. Antimicrob. Agents Chemother. 59, 1030–1037. doi: 10.1128/AAC.04613-14
He, X., Zhao, M., Chen, J., Wu, R., Zhang, J., Cui, R., et al. (2015). Overexpression of both ERG11 and ABC2 genes might be responsible for itraconazole resistance in clinical isolates of Candida krusei. PLoS One 10:e0136185. doi: 10.1371/journal.pone.0136185
Holmes, A. R., Lin, Y. H., Niimi, K., Lamping, E., Keniya, M., Niimi, M., et al. (2008). ABC transporter Cdr1p contributes more than Cdr2p does to fluconazole efflux in fluconazole-resistant Candida albicans clinical isolates. Antimicrob. Agents Chemother. 52, 3851–3862. doi: 10.1128/AAC.00463-08
Ishikawa, S., Tamaki, M., Ogawa, Y., Kaneki, K., Zhang, M., Sunagawa, M., et al. (2016). Inductive effect of palmatine on apoptosis in RAW 264.7 cells. Evid. Based Complement. Alternat. Med. 2016:7262054. doi: 10.1155/2016/7262054
Iwasa, K., Nariba, H., Lee, D.-U., and Kang, S.-I. (1998). Structure-activity relationships of protoberberines having antimicrobial activity. Planta Med. 64, 748–751. doi: 10.1055/s-2006-957572
Katiyar, S. K., and Edlind, T. D. (2001). Identification and expression of multidrug resistance-related ABC transporter genes in Candida krusei. Med. Mycol. 39, 109–116. doi: 10.1080/mmy.39.1.109.116
Lamping, E., Ranchod, A., Nakamura, K., Tyndall, J. D., Niimi, K., Holmes, A. R., et al. (2009). Abc1p is a multidrug efflux transporter that tips the balance in favor of innate azole resistance in Candida krusei. Antimicrob. Agents Chemother. 53, 354–369. doi: 10.1128/AAC.01095-08
Letscher-Bru, V., Obszynski, C., Samsoen, M., Sabou, M., Waller, J., and Candolfi, E. (2013). Antifungal activity of sodium bicarbonate against fungal agents causing superficial infections. Mycopathologia 175, 153–158. doi: 10.1007/s11046-012-9583-2
Li, D., Xu, Y., Zhang, D., Quan, H., Mylonakis, E., Hu, D., et al. (2013). Fluconazole assists berberine to kill fluconazole-resistant Candida albicans. Antimicrob. Agents Chemother. 57, 6016–6027. doi: 10.1128/AAC.00499-13
Livak, K. J., and Schmittgen, T. D. (2001). Analysis of relative gene expression data using real-time quantitative PCR and the 2-ΔΔCT method. Methods 25, 402–408. doi: 10.1006/meth.2001.1262
Lu, M., Li, T., Wan, J., Li, X., Yuan, L., and Sun, S. (2017). Antifungal effects of phytocompounds on Candida species alone and in combination with fluconazole. Int. J. Antimicrob. Agents 49, 125–136. doi: 10.1016/j.ijantimicag.2016.10.021
Maesaki, S., Marichal, P., Bossche, H. V., Sanglard, D., and Kohno, S. (1999). Rhodamine 6G efflux for the detection of CDR1-overexpressing azole-resistant Candida albicans strains. J. Antimicrob. Chemother. 44, 27–31. doi: 10.1093/jac/44.1.27
Messer, S. A., Jones, R. N., and Fritsche, T. R. (2006). International surveillance of Candida spp. and Aspergillus spp.: report from the SENTRY Antimicrobial Surveillance Program (2003). J. Clin. Microbiol. 44, 1782–1787. doi: 10.1128/JCM.44.5.1782-1787.2006
Mukhopadhyay, K., Kohli, A., and Prasad, R. (2002). Drug susceptibilities of yeast cells are affected by membrane lipid composition. Antimicrob. Agents Chemother. 46, 3695–3705. doi: 10.1128/AAC.46.12.3695-3705.2002
Odds, F. (2003). Synergy, antagonism, and what the chequerboard puts between them. J. Antimicrob. Chemother. 52:1. doi: 10.1093/jac/dkg301
Oxman, D. A., Chow, J. K., Frendl, G., Hadley, S., Hershkovitz, S., Ireland, P., et al. (2010). Candidaemia associated with decreased in vitro fluconazole susceptibility: is Candida speciation predictive of the susceptibility pattern? J. Antimicrob. Chemother. 65, 1460–1465. doi: 10.1093/jac/dkq136
Park, K. S., Kang, K. C., Kim, J. H., Adams, D. J., Johng, T. N., and Paik, Y. K. (1999). Differential inhibitory effects of protoberberines on sterol and chitin biosyntheses in Candida albicans. J. Antimicrob. Chemother. 43, 667–674. doi: 10.1093/jac/43.5.667
Pfaller, M. A., Castanheira, M., Messer, S. A., Moet, G. J., and Jones, R. N. (2010a). Variation in Candida spp. Distribution and antifungal resistance rates among bloodstream infection isolates by patient age: report from the SENTRY Antimicrobial Surveillance Program (2008-2009). Diagn. Microbiol. Infect. Dis. 68, 278–283. doi: 10.1016/j.diagmicrobio.2010.06.015
Pfaller, M. A., Diekema, D. J., Gibbs, D. L., Newell, V. A., Ellis, D., Tullio, V., et al. (2010b). Results from the ARTEMIS DISK global antifungal surveillance study, 1997 to 2007: a 10.5-year analysis of susceptibilities of Candida Species to fluconazole and voriconazole as determined by CLSI standardized disk diffusion. J. Clin. Microbiol. 48, 1366–1377. doi: 10.1128/JCM.02117-09
Pfaller, M. A., Diekema, D. J., Mendez, M., Kibbler, C., Erzsebet, P., Chang, S. C., et al. (2006). Candida guilliermondii, an opportunistic fungal pathogen with decreased susceptibility to fluconazole: geographic and temporal trends from the ARTEMIS DISK antifungal surveillance program. J. Clin. Microbiol. 44, 3551–3556. doi: 10.1128/JCM.00865-06
Pfaller, M. A., Jones, R. N., and Castanheira, M. (2014). Regional data analysis of Candida non-albicans strains collected in United States medical sites over a 6-year period, 2006-2011. Mycoses 57, 602–611. doi: 10.1111/myc.12206
Poulain, D. (2013). Candida albicans, plasticity and pathogenesis. Crit. Rev. Microbiol. 41, 208–217. doi: 10.3109/1040841X.2013.813904
Ricardo, E., Miranda, I. M., Faria-Ramos, I., Silva, R. M., Rodrigues, A. G., and Pina-Vaz, C. (2014). In vivo and in vitro acquisition of resistance to voriconazole by Candida krusei. Antimicrob. Agents Chemother. 58, 4604–4611. doi: 10.1128/AAC.02603-14
Rodrigues, C. F., Silva, S., and Henriques, M. (2014). Candida glabrata: a review of its features and resistance. Eur. J. Clin. Microbiol. Infect. Dis. 33, 673–688. doi: 10.1007/s10096-013-2009-3
Samaranayake, Y. H., and Samaranayake, L. P. (1994). Candida krusei: biology, epidemiology, pathogenicity and clinical manifestations of an emerging pathogen. J. Med. Microbiol. 41, 295–310. doi: 10.1099/00222615-41-5-295
Sanglard, D., Ischer, F., and Bille, J. (2001). Role of ATP-binding-cassette transporter genes in high-frequency acquisition of resistance to azole antifungals in Candida glabrata. Antimicrob. Agents Chemother. 45, 1174–1183. doi: 10.1128/AAC.45.4.1174-1183.2001
Sanglard, D., Ischer, F., Calabrese, D., Majcherczyk, P. A., and Bille, J. (1999). The ATP binding cassette transporter gene CgCDR1 from Candida glabrata is involved in the resistance of clinical isolates to azole antifungal agents. Antimicrob. Agents Chemother. 43, 2753–2765. doi: 10.1128/AAC.43.11.2753
Sanglard, D., Ischer, F., Monod, M., and Bille, J. (1996). Susceptibilities of Candida albicans multidrug transporter mutants to various antifungal agents and other metabolic inhibitors. Antimicrob. Agents Chemother. 40, 2300–2305. doi: 10.1128/AAC.40.10.2300
Sanglard, D., Ischer, F., Monod, M., and Bille, J. (1997). Cloning of Candida albicans genes conferring resistance to azole antifungal agents: characterization of CDR2, a new multidrug ABC transporter gene. Microbiology 143, 405–416. doi: 10.1099/00221287-143-2-405
Sanguinetti, M., Posteraro, B., Fiori, B., Ranno, S., Torelli, R., and Fadda, G. (2005). Mechanisms of azole resistance in clinical isolates of Candida glabrata collected during a hospital survey of antifungal resistance. Antimicrob. Agents Chemother. 49, 668–679. doi: 10.1128/AAC.49.2.668-679.2005
Sanguinetti, M., Posteraro, B., and Lass-Florl, C. (2015). Antifungal drug resistance among Candida species: mechanisms and clinical impact. Mycoses 58, 2–13. doi: 10.1111/myc.12330
Sardi, J., Scorzoni, L., Bernardi, T., Fusco-Almeida, A., and Giannini, M. M. (2013). Candida species: current epidemiology, pathogenicity, biofilm formation, natural antifungal products and new therapeutic options. J. Med. Microbiol. 62, 10–24. doi: 10.1099/jmm.0.045054-0
Savini, V., Catavitello, C., Onofrillo, D., Masciarelli, G., Astolfi, D., Balbinot, A., et al. (2011). What do we know about Candida guilliermondii? A voyage throughout past and current literature about this emerging yeast. Mycoses 54, 434–441. doi: 10.1111/j.1439-0507.2010.01960.x
Shahzad, M., Sherry, L., Rajendran, R., Edwards, C. A., Combet, E., and Ramage, G. (2014). Utilising polyphenols for the clinical management of Candida albicans biofilms. Int. J. Antimicrob. Agents 44, 269–273. doi: 10.1016/j.ijantimicag.2014.05.017
Shao, J., Cui, Y., Zhang, M., Wang, T., Wu, D., and Wang, C. (2017). Synergistic in vitro activity of sodium houttuyfonate with fluconazole against clinical Candida albicans strains under planktonic growing conditions. Pharm. Biol. 55, 355–359. doi: 10.1080/13880209.2016.1237977
Shao, J., Lu, K. Q., Tian, G., Cui, Y. Y., Yan, Y. Y., Wang, T. M., et al. (2015). Lab-scale preparations of Candida albicans and dual Candida albicans-Candida glabrata biofilms on the surface of medical-grade polyvinyl chloride (PVC) perfusion tube using a modified gravity-supported free-flow biofilm incubator (GS-FFBI). J. Microbiol. Methods 109, 41–48. doi: 10.1016/j.mimet.2014.12.006
Shao, J., Wang, T. M., Yan, Y. Y., Shi, G. X., Cheng, H. J., Wu, D. Q., et al. (2014). Matrine reduces yeast-to-hypha transition and resistance of a fluconazole-resistant strain of Candida albicans. J. Appl. Microbiol. 117, 618–626. doi: 10.1111/jam.12555
Shao, J., Zhang, M., Wang, T., Li, Y., and Wang, C. (2016). The roles of CDR1, CDR2, and MDR1 in kaempferol-induced suppression with fluconazole-resistant Candida albicans. Pharm. Biol. 54, 984–992. doi: 10.3109/13880209.2015.1091483
Shi, G., Shao, J., Wang, T., Wu, D., and Wang, C. (2017). Mechanism of berberine-mediated fluconazole-susceptibility enhancement in clinical fluconazole-resistant Candida tropicalis isolates. Biomed. Pharmacother. 93, 709–712. doi: 10.1016/j.biopha.2017.06.106
Silva, S., Negri, M., Henriques, M., Oliveira, R., Williams, D. W., and Azeredo, J. (2012). Candida glabrata, Candida parapsilosis and Candida tropicalis: biology, epidemiology, pathogenicity and antifungal resistance. FEMS Microbiol. Rev. 36, 288–305. doi: 10.1111/j.1574-6976.2011.00278.x
Singh, B. N., Upreti, D. K., Singh, B. R., Pandey, G., Verma, S., Roy, S., et al. (2015). Quercetin sensitizes fluconazole-resistant Candida albicans to induce apoptotic cell death by modulating quorum sensing. Antimicrob. Agents Chemother. 59, 2153–2168. doi: 10.1128/AAC.03599-14
Souza, A. C., Fuchs, B. B., Pinhati, H. M., Siqueira, R. A., Hagen, F., Meis, J. F., et al. (2015). Candida parapsilosis resistance to fluconazole: molecular mechanisms and in vivo impact in infected galleria mellonella larvae. Antimicrob. Agents Chemother. 59, 6581–6587. doi: 10.1128/AAC.01177-15
Sun, L. M., Liao, K., Liang, S., Yu, P. H., and Wang, D. Y. (2015). Synergistic activity of magnolol with azoles and its possible antifungal mechanism against Candida albicans. J. Appl. Microbiol. 118, 826–838. doi: 10.1111/jam.12737
Taff, H. T., Mitchell, K. F., Edward, J. A., and Andes, D. R. (2013). Mechanisms of Candida biofilm drug resistance. Future Microbiol. 8, 1325–1337. doi: 10.2217/fmb.13.101
Tobudic, S., Kratzer, C., Lassnigg, A., and Presterl, E. (2012). Antifungal susceptibility of Candida albicans in biofilms. Mycoses 55, 199–204. doi: 10.1111/j.1439-0507.2011.02076.x
Torelli, R., Posteraro, B., Ferrari, S., La Sorda, M., Fadda, G., Sanglard, D., et al. (2008). The ATP-binding cassette transporter-encoding gene CgSNQ2 is contributing to the CgPDR1-dependent azole resistance of Candida glabrata. Mol. Microbiol. 68, 186–201. doi: 10.1111/j.1365-2958.2008.06143.x
Vollekova, A., Kost’alova, D., Kettmann, V., and Toth, J. (2003). Antifungal activity of Mahonia aquifolium extract and its major protoberberine alkaloids. Phytother. Res. 17, 834–837. doi: 10.1002/ptr.1256
Whaley, S. G., Berkow, E. L., Rybak, J. M., Nishimoto, A. T., Barker, K. S., and Rogers, P. D. (2017). Azole antifungal resistance in Candida albicans and emerging Non-albicans Candida species. Front. Microbiol. 7:2173. doi: 10.3389/fmicb.2016.02173
Wu, J., Xiao, Q., Zhang, N., Xue, C., Leung, A. W., Zhang, H., et al. (2016). Photodynamic action of palmatine hydrochloride on colon adenocarcinoma HT-29 cells. Photodiagnosis Photodyn. Ther. 15, 53–58. doi: 10.1016/j.pdpdt.2016.05.005
Xiao, C.-W., Ji, Q.-A., Wei, Q., Liu, Y., and Bao, G.-L. (2015). Antifungal activity of berberine hydrochloride and palmatine hydrochloride against Microsporum canis -induced dermatitis in rabbits and underlying mechanism. BMC Complement. Altern. Med 15:177. doi: 10.1186/s12906-015-0680-x
Zavrel, M., and White, T. C. (2015). Medically important fungi respond to azole drugs: an update. Future Microbiol. 10, 1355–1373. doi: 10.2217/FMB.15.47
Keywords: palmatine, fluconazole, itraconazole, Candida species, efflux, resistance
Citation: Wang T, Shao J, Da W, Li Q, Shi G, Wu D and Wang C (2018) Strong Synergism of Palmatine and Fluconazole/Itraconazole Against Planktonic and Biofilm Cells of Candida Species and Efflux-Associated Antifungal Mechanism. Front. Microbiol. 9:2892. doi: 10.3389/fmicb.2018.02892
Received: 10 July 2018; Accepted: 12 November 2018;
Published: 03 December 2018.
Edited by:
Santi M. Mandal, Indian Institute of Technology Kharagpur, IndiaReviewed by:
Ashok K. Chaturvedi, The University of Texas at San Antonio, United StatesCopyright © 2018 Wang, Shao, Da, Li, Shi, Wu and Wang. This is an open-access article distributed under the terms of the Creative Commons Attribution License (CC BY). The use, distribution or reproduction in other forums is permitted, provided the original author(s) and the copyright owner(s) are credited and that the original publication in this journal is cited, in accordance with accepted academic practice. No use, distribution or reproduction is permitted which does not comply with these terms.
*Correspondence: Jing Shao, dXN0Y25qbnVzanR1QDEyNi5jb20= Changzhong Wang, d2FuZ2NoYW5nemhvbmc1M0AxMjYuY29t
†These authors have contributed equally to this work
Disclaimer: All claims expressed in this article are solely those of the authors and do not necessarily represent those of their affiliated organizations, or those of the publisher, the editors and the reviewers. Any product that may be evaluated in this article or claim that may be made by its manufacturer is not guaranteed or endorsed by the publisher.
Research integrity at Frontiers
Learn more about the work of our research integrity team to safeguard the quality of each article we publish.