- 1Applied Microbial Ecology Laboratory, Departamento de Ciencias Químicas y Recursos Naturales, Universidad de La Frontera, Temuco, Chile
- 2Center of Plant, Soil Interaction and Natural Resources Biotechnology, Scientific and Technological Bioresource Nucleus (BIOREN), Universidad de La Frontera, Temuco, Chile
- 3Programa de Doctorado en Ciencias de Recursos Naturales, Universidad de La Frontera, Temuco, Chile
- 4Department of Soil, Water, and Climate, Department of Plant and Microbial Biology, BioTechnology Institute, University of Minnesota, Saint Paul, MN, United States
Acidic ash derived volcanic soils (Andisols) support 50% of cereal production in Chile. Nitrogen (N) is essential for cereal crops and commonly added as urea with consequent environmental concerns due to leaching. Despite the relevance of N to plant growth, few studies have focused on understanding the application, management and ecological role of N2-fixing bacterial populations as tool for improve the N nutrition of cereal crops in Chile. It is known that N2-fixing bacteria commonly inhabits diverse plant compartments (e.g., rhizosphere and root endosphere) where they can supply N for plant growth. Here, we used culture-independent and dependent approaches to characterize and compare the putative N2-fixing bacteria associated with the rhizosphere and root endosphere of wheat plants grown in an Andisol from southern Chile. Our results showed significantly greater bacterial loads in the rhizosphere than the root endosphere. Quantitative PCR results indicated that the copy number of the 16S rRNA gene ranged from 1012~1013 and 107~108 g−1 sample in rhizosphere and root endosphere, respectively. The nifH gene copy number ranged from 105~106 and 105 g−1 sample in rhizosphere and root endosphere, respectively. The total culturable bacteria number ranged from 109~1010 and 107~108 CFU g−1 sample in rhizosphere and 104~105 and 104 CFU g−1 sample in root endosphere using LB and NM-1 media, respectively. Indirect counts of putative N2-fixing bacteria were 103 and 102~103 CFU g−1 sample in rhizosphere and root endosphere using NFb medium, respectively. Sequencing of 16S rRNA genes from randomly selected putative N2-fixing bacteria revealed the presence of members of Proteobacteria (Bosea and Roseomonas), Actinobacteria (Georgenia, Mycobacterium, Microbacterium, Leifsonia, and Arthrobacter), Bacteroidetes (Chitinophaga) and Firmicutes (Bacillus and Psychrobacillus) taxa. Differences in 16S rRNA and putative nifH-containing bacterial communities between rhizosphere and root endosphere were shown by denaturing gradient gel electrophoresis (DGGE). This study shows a compartmentalization between rhizosphere and root endosphere for both the abundance and diversity of total (16S rRNA) and putative N2-fixing bacterial communities on wheat plants grown in Chilean Andisols. This information can be relevant for the design and application of agronomic strategies to enhance sustainable N-utilization in cereal crops in Chile.
Introduction
Agricultural production in southern Chile is established in acidic ash derived volcanic soils (Andisols), which support around 50% of cereal production in Chile (Laval and Garcia, 2018). In these soils, nitrogen (N) fertilization (as urea and other chemicals) is a common practice to improve agricultural production. The application of N is essential for crop yields and its availability is crucial during plant vegetative development and seed development (Ohyama et al., 2014), but also contributes to Andisol acidification and contamination of water bodies by N leaching (Nuñez et al., 2010).
Currently, it is widely accepted that the plant rhizomicrobiome contributes in a direct or indirect way to the growth and fitness of plants, providing phytohormones, solubilizing nutrients, fixing nitrogen (N2), establishing biocontrol of phytopathogens, and chelating metallic ions (De-la-Peña and Loyola-Vargas, 2014). Biological N2 fixation by bacteria is the most ecologically and agronomically relevant benefit obtained by plants from their interaction with bacteria, Atmospheric N2 is reduced to ammonia (NH3) by the bacterial nitrogenase enzyme complex making it accessible for plant uptake. Thus, the recruitment of N2-fixing bacteria under symbiotic or non-symbiotic relationships (e.g., nodulation of legume plants by Rhizobium spp. or interaction with free-living associative N2 fixers) helps the host plant to obtain N directly from atmosphere and fulfill its nutritional requirements (de Bruijn, 2015). Studies have also show that some genera of free-living bacteria (e.g., Azospirillum and Azotobacter, and others) can colonize diverse plant niches such as the rhizosphere (soil influenced by plant roots) and endosphere (inner tissues of plants), contributing to the N needs of non-leguminous plants (Bhattacharyya and Jha, 2012).
The inoculation or bioaugmentation of plants with N2-fixing bacteria is an attractive alternative to traditional N-fertilization practices and results in decreased fertilization costs and an environmentally friendly alternative to use of agrochemicals. In pastures grown in Chilean Andisols, studies have demonstrated that N2 fertilization induces changes in total rhizobacterial populations, including potential plant growth-promoting rhizobacteria and populations harboring the nifH gene (Martínez et al., 2011; Jorquera et al., 2014a). Symbiotic N2-fixing bacteria (e.g., Bradyrhizobium) have been isolated from nodules of yellow lupin (Lupinus luteus) grown in Chilean Andisols (Campos et al., 2014). Partial sequencing of 16S rRNA genes, the application of denaturing gradient gel electrophoresis (DGGE), and 454-Roche pyrosequencing revealed a great diversity of bacterial group present in pasture and cereal rhizospheres of plants grown in Chilean Andisols, including also some N2-fixing bacteria such as the bradyrhizobia (Jorquera et al., 2014b; Lagos et al., 2014).
However, despite the relevance of N nutrition in cereal production in Andisols in southern Chile, few studies have been done to explore the association of N2-fixing bacteria with cereals grown in Chilean acid volcanic soils. Several studies have demonstrated that the abundance, diversity, and activity of bacterial populations associated with plants may play a central role in its productivity (Turner et al., 2013; Berg et al., 2014). Therefore, information on N2-fixing bacterial populations in cereal crops can be relevant for bioprospecting of native bacterial strains as inoculants as well as the develop of management strategies to improve the N nutrition of plants and decreasing our dependency to chemical N fertilization.
In this study, we used culture-independent and dependent approaches to characterize and compare putative N2-fixing bacterial populations associated with the rhizosphere and root endosphere of wheat plants grown in an Andisol from southern Chile.
Materials and Methods
Sampling
Wheat plants and their adhered rhizosphere soil were placed into sterile flasks (in triplicates) and immediately transported on ice to the Applied Microbial Ecology Laboratory (EMAlab) of Universidad de La Frontera, Temuco, Chile. The samples were taken from four wheat cultivars (Triticum aestivum cv. Feña, Patras, Joker, and Rocky, labeled as F, P, J and R, respectively) grown in an Andisol located in the La Araucanía region (38°32′47.5″S, 72°27′43.6″W) of Chile under yearly rotation with rapeseed (Brassica napus) and oat (Avena sativa) since 2012. Prior to sampling, the soil was fertilized with 140 kg of urea ha−1 and treated with Bacara (Bayer Crop Science, Inc.), and a commercial mixture of the pre-emergence herbicides flufenacet, flurtamone, and diflufenican, to a final concentration of 1 L ha−1. Rhizosphere, roots and shoots were separated separately prior to further activities.
The chemical properties of rhizosphere samples was determined from composited samples as follows: the soil pH was measured in 1:2.5 soil:deionized water suspensions, extractable P (POlsen) was extracted using Na-bicarbonate (0.5 M) and analyzed using the molybdate-blue method (Murphy and Riley, 1962), exchangeable cations (K, Ca, Mg, and Na) were extracted with CH3COONH4 (1 M) at pH 7.0 and analyzed by flame atomic adsorption spectrophotometry (FAAS) (Warncke and Brown, 1998), and exchangeable aluminum was extracted with KCl (1 M) and analyzed by FAAS (Bertsch and Bloom, 1996).
Counts of Putative N2-Fixing Bacteria
Total and N2-fixing bacteria populations in rhizosphere and root endosphere samples were estimated by quantitative PCR (qPCR) using the 16S rRNA and nifH as target genes. Total DNA was extracted from rhizosphere samples (0.25 g) by using DNeasy PowerSoil Kits (Qiagen, Inc.), according to manufacturer instructions. For endosphere samples (0.15 g), root samples were initially vigorously washed with sterile distilled water (SDW) and rhizoplane surfaces were disinfected by three continuous washes in 80% ethanol for 5 min, followed by a 20 min immersion in 4% sodium hypochlorite and three rinses with SDW as described by Durán et al. (2014). The roots were macerated on sterile ceramic mortars and homogenized in 1.5 mL of sterile 0.85% saline solution. To verify sterility of rhizoplane samples, SDW from the third rinse was collected and processed as a sample for sterility control purposes. Root endosphere total DNA was extracted from 0.25 mL of surface sterile root homogenate using Quick-DNA™ Plant/Seed Miniprep Kit (Zymo Research Corp.), according to manufacturer instructions. The DNA concentrations were adjusted by dilution to 20 ng ul−1 and quality (260:280 ratio) was confirmed at ~1.8.
The quantitation of nifH genes was done by using the primer set nifH-g1-forB (5′-GGT TGT GAC CCG AAA GCT GA-3′) and nifH-g1-rev (5′-GCG TAC ATG GCC ATC ATC TC-3′) (Bürgmann et al., 2003). The following PCR conditions were used: 95°C for 11 s followed by cycles of 95°C for 15s, 60°C for 30 s, and a final extension step at 75°C for 8 s and at 72°C for 10 s (Bürgmann et al., 2003). The quantitation of 16S rRNA genes was done by using the mitochondria- and chloroplast excluding primer set 799f (5′-AAC MGG ATT AGA TAC CCK G-3′) and 1115r (5′-AGG GTT GCG CTC GTT G-3′) (Shade et al., 2013) with the following program: cycles of 94°C for 1 min, annealing at 53°C for 1 min and extension at 72°C during 1 min; with a final extension step at 72°C during 10 min. (Beckers et al., 2016). Both qPCR assays were done using PowerUp™ SYBR® Green Master Mix (Thermo Fisher Scientific Inc.). The quantitation of gene copies was estimated by using standard curves prepared with synthetic ~1,500 bp dsDNA ultramers (Integrated DNA Technologies, Inc.) of the nifH gene from Azospirillum brasilense Sp7 (NCBI accession no. X51500) and the 16S rRNA gene from Azospirillum picis (NCBI accession no. AM922283), respectively.
Counts of Culturable and Putative N2-Fixing Bacteria
Rhizosphere soil (2 g) was suspended in 50 mL of SDW. Previously macerated root samples (1 mL) from section Counts of Putative N2-Fixing Bacteria above were resuspended in 25 mL of SDW. Both sample types were homogenized by sonication for 30 s at 120 kHz. Suspensions were serially diluted in 0.85% saline used in plate counting methods described as follow. Counts of total culturable bacteria were done on Luria Bertani agar medium (LB) (10 g l−1 Triptone; 5 g l−1; Yeast Extract; 5 g l−1 NaCl; Sambrook and Russell, 2001), and on NM-1 oligotrophic medium (0.5 g l−1 D-glucose, 0.5 g l−1 polypeptone, 0.5 g l−1 Na- glutamate, 0.5 g l−1 yeast extract, 0.44 g l−1 KH2PO4, 0.1 g l−1 (NH4)2SO4, 0.1 gl−1 MgSO4 · 7H2O, 15 g l−1 agar, and 1 ml vitamin solution containing 1 g l−1 nicotinamide, 1 g l−1 thiamine hydrochloride, 0.05 g l−1 biotin, 0.5 g l−1 4-aminobenzoic acid, 0.01 g l−1 vitamin B12, 0.5 g l−1 D- pantothenic acid hemicalcium salt, 0.5 g l−1 pyridoxamine dihydrochloride, 0.5 g l−1 folic acid; (Nakamura et al., 1995)). Both culture media were amended with 10 μg ml−1 cycloheximide prevent fungal growth. Autoclaved agar was added before plating at final concentration of 1.5% for both media, as recommended by Tanaka et al. (2014), to prevent hydrogen peroxide formation, resulting in bacterial growth inhibition with the concomitant underestimation of total culturable bacteria counts (Supplementary Figure 1). Aliquots (50 μl) of appropriated dilutions of the rhizosphere and root endosphere suspensions were separately plated onto petri dishes containing LB and NM-1 media, and incubated for 4 days at 28°C. Colonies grown on agar plates were automatically counted by using CLIQS Colony Counter software (TotalLab Inc., UK).
In parallel, indirect counts of putative N2-fixing bacteria were carried-out by MPN analyses as recommend by Baldani et al. (2014), using semisolid (0.5% agar) NFb medium tubes [5 g L−1 malic acid, 0.5 g L−1 K2HPO4, 0.1 g L−1 NaCl, 0.02 g L−1 CaCl2×2H2O, 2 mL of micronutrient solution (0.4 g L−1 CuSO4×5H2O, 0.12 g L−1 ZnSO4, 1.4 g L−1 H3BO3, 1 g Na2MoO4×2H2O, 1.5 g and MnSO4×H2O), 2 mL 0.5% bromothymol blue in 0.2N KOH, 4 mL of 1.64% Fe[III] EDTA, and 1 mL of vitamin solution containing 100 mg L−1 biotin and 200 mg L−1 pyridoxamine dihydrochloride; (Hartmann and Baldani, 2006)]. Tubes containing 5 mL of NFb medium were inoculated with 100 μL of sample and incubated for 4 days at 37°C. The presence of white layer (or pellicle), an indicator of N2-fixing bacterial growth, was checked, and aseptically removed by using sterile cork borers. After MPN analyses, the isolation of putative N2-fixing bacteria was performed by resuspension of the pellicle on 1 mL 0.85% NaCl, and 100 μL reinoculated into fresh tubes of NFb medium. Tubes were incubated at 37°C for 4 days. After second incubation, the pellicle was newly aseptically removed, resuspended on 1 mL 0.85% NaCl, serially diluted and plated onto LB and Congo red malic-acid (CRMA; 0.5 g L−1 K2HPO4; 0.2 g L−1 MgSO4 × 7H2O; 0.1 g L−1 NaCl, 0.5 g−1 yeast extract, 0.015 g L−1 FeCl3×H2O; 5 g L−1 DL-malic acid, 4.8 g L−1 KOH, and 15 mL−1 of 1:400 Congo red; Rodriguez-Caceres, 1982) agar media. Colonies (75) were randomly selected from CRMA agar plates according to their morphology, purified by streaking on NFb agar plates, and stored in 1 mL sterile LB-Glycerol (7:3) at−80°C.
Characterization of Putative N2-Fixing Bacteria
Total DNA from selected rhizosphere and root endosphere isolates was extracted by using the Proteinase K-CTAB (cetyl-trimethylammonium bromide) method as described by Wilson (2001). To prevent analysis of clones, all isolates were firstly genotyping by using rep-PCR DNA fingerprinting and ERIC primers as described by Versalovic et al. (1991). The 16S rRNA genes were amplified from 20 and 18 genetically-different rhizosphere and root endosphere isolates, respectively, by using PCR and the universal bacterial primer set 27f (5′-AGA GTT TGA TCC TGG CTC AG-3′) and 1492r (5′-TAC GGY TAC CTT GTT ACG ACT T-3′) (Lane, 1991) and by using the PCR conditions suggested by Jorquera et al. (2012). In parallel, nifH genes was also amplified by using the primer set PolF (5′-TGC GAY CCS AAR GCB GAC TC-3′) and PolR (5′-ATS GCC ATC ATY TCR CCG GA-3′) and PCR condition suggested by Jorquera et al. (2014a).
Synthetic 16S rRNA and nifH genes from Azospirillum picis and Azospirillum brasilense Sp7 were used as positive controls, respectively.
In addition, a variety of universal nifH primer combinations were also tested, including PolFI and PolR; PolF and AQER; nifH-g1-forA and nifH-g1-rev; nifH-g1-forB and nifH-g1-rev; MehtaF and MehtaR (Supplementary Table 1). Despite the large number of primers used, however, sequence analyses indicated that the amplicons were unspecific and not related to nifH.
The PCR products were sequenced by Macrogen Inc. (Seoul, South Korea), trimmed, cleaned up, and compared with those deposited in GenBank database using BLASTn tool (https://blast.ncbi.nlm.nih.gov/Blast.cgi). To the presence of nifH, nucleotide sequences were translated into amino acids and compared with those present in GenBank by using BLASTx. Sequences (1 from rhizosphere and 7 from the root endosphere) showing positive alignment with the nifH enzyme were used to build a neighbor-joining tree using representative sequences of nifH reported in literature, including Azoarcus, Azospirillum, Azotobacter, Bosea, Bradyrhizobium, Bacillus, Burkholderia, Chitinophaga, Herbaspirillum, Mesorhizobium Microbacterium, Pontibacter, Rhizobium, and Roseomonas. The alignment of amino acids sequences was done by CLUSTAL W (Larkin et al., 2007) and neighbor-joining trees were built using Geneious version R11 (Bootstrap = 1000) (Kearse et al., 2012).
Sequences obtained in this study were deposited in GenBank under accession numbers MG835569 to MG835606 for 16S rRNA gene, and MH175481 - MH175487 as well as MH175490 for nifH gene sequences.
DGGE Fingerprinting of Total and N2-Fixing Bacterial Communities
Fingerprinting of total and N2-fixing bacterial communities in rhizosphere and root endosphere samples was done by using DGGE of 16S rRNA and nifH as target genes, respectively. For total bacterial communities, 16S rRNA genes were first amplified by using primer set 933f (5′-GCA CAA GCG GTG GAG CAT GTG G-3′) and 1492r. Then, ~600 bp bands were confirmed on electrophoresis, and used as template for a nested PCR with primer set 933f-gc and 1387r (5′-GCC CGG GAA CGT ATT CAC CG-3′). The GC-clamp (5′-CGC CCG CCG CGC GCG GCG GGC GGG GCG GGG GCA CGG GGG-3′) was attached to the 5′-end of primer 933f, and PCR reactions were carried as described by Jorquera et al. (2012). In parallel, the amplification of nifH was done by nested PCR using in the first PCR round the PolF (5′-TGC GAY CCS AAR GCB GAC TC-3′) and PolR (5′-ATS GCC ATC ATY TCR CCG GA-3′) primer set. After specific ~400 bp bands were confirmed on electrophoresis, a second PCR reaction was carried with the PolFI (5′-TGC GAI CCS AAI GCI GAC TC-3′) and AQER-GC30 (5′-GAC GAT GTA GAT YTC CTG GGG-3′) primer set. The GC-clamp (5′-CGC CCG CCG CGC CCC GCG CCC GGC CCG CCC GAC GAT GTA GAT YTC CTG-3′) was attached to the 5′-end of primer AQER and the PCR conditions were carried out according to described by Jorquera et al. (2014a).
The DGGE analysis was performed using a DCode system (Bio-Rad Laboratories, Inc.). PCR products (20 μL) were loaded onto 6% (w/v) polyacrylamide gel with a 50–75% denaturing gradient (7 M urea and 40% formamide) and electrophoresis was run for 16 h at 80 V. The gel was stained with SYBR Gold (Molecular Probes, Invitrogen Co.) for 30 min and photographed on a UV transilluminator. Image analysis and clustering of DGGE banding profiles were done under CLIQS 1D Pro software (TotalLab Ltd). Based on the matrix obtained from CLIQS 1D Pro analysis, the distances between the bacterial communities from rhizosphere and root endosphere 16S rRNA and nifH genes were calculated by similarity profile analysis (SIMPROF test) with Bray-Curtis similarity index with a 5% significance level and <0.15 stress values with Primer-E v6 (Primer-E Ltd.; http://www.primer-e.com/) (Clarke, 1993; Clarke et al., 2008). A graphical representation of this results was generated through non-metric multidimensional scaling (nMDS) plots, developed with the same software. The similarities of rhizosphere and root endosphere 16S rRNA and nifH communities were compared at 40 and 60%.
Statistical Analysis
The culture-dependent (dilutions, plating, and isolation) as well as -independent (DNA extractions, PCR, DGGE, and qPCR) procedures were performed in triplicates and analyzed by one-way ANOVA. Comparisons were carried out for each pair with Tukey HSD test using IBM SPSS Statistics 24 (IBM Corporation). Values are given as means ± standard deviation on means. Differences were considered to be significant when the P-value was ≤ 0.05.
Results
Rhizosphere Soil Properties
No large differences in soil chemical properties were observed between the rhizosphere soils of different wheat cultivars, showing typical characteristics of Chilean Andisols used in agriculture. The properties of rhizosphere soils (Table 1) were as follows: pH ranged from 6.29 to 6.39, organic matter ranged from 15 to 16%, and the ranges for the macronutrients N, P, and K were 52.7~63.1, 29~37, and 138~159 mg kg−1, respectively. The cation exchange capacity ranged from 16.08 to 18.01 cmol(+) kg−1, with an Al saturation between 0.06 and 0.25%.
Counts of Total- and N2-Fixing Bacteria
The qPCR analyses indicated that there were larges differences in total bacteria loads between rhizosphere and root endosphere samples (Figure 1). Significantly greater (P ≤ 0.05) counts of total bacteria were observed in the rhizosphere (1.8 × 1012~9.2 × 1013 copies of 16S rRNA genes g−1 sample) compared with the root endosphere (2.2 × 107~3.6 × 108 copies of 16S rRNA genes g−1 sample) samples. Similarly, significantly greater (P ≤ 0.05) counts of total N2-fixing bacteria were also observed in the rhizosphere (3.3 × 105~8.1 × 106 copies of nifH gene g−1 sample) compared with root endosphere (1.7~6.5 × 105 copies of nifH gene g−1 sample) samples. However, the differences between rhizosphere and root endosphere samples were lower when counts of the nifH gene are compared with 16S rRNA genes.
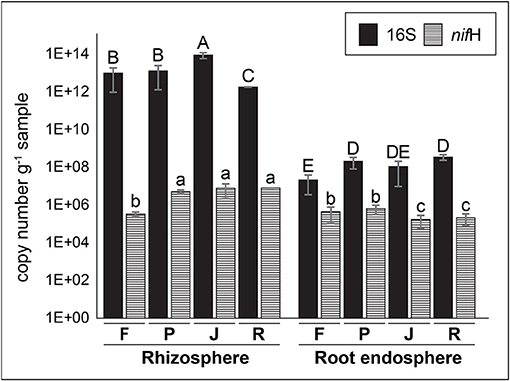
Figure 1. Counts (gene copy number per g of sample) of total and N2-fixing bacteria in rhizosphere and root endosphere samples of wheat plants by quantitative PCR (qPCR) using specific primer set for 16S rRNA and nifH genes. Samples labeled as F, P, J, and R correspond to Feña, Patras, Joker, and Rocky wheat cultivars, respectively. Error bars represent standard deviation and different lower letters denote statistical difference (P ≤ 0.05, Tukey HSD test) (n = 3).
Counts of Culturable and Putative N2-Fixing Bacteria
The use of culture media also showed larges differences in total culturable bacterial counts between rhizosphere and root endosphere samples (Figure 2A). Significantly greater (P ≤ 0.05) counts of total cultural bacteria were observed in the rhizosphere (1.1 × 109~1.9 × 1010 and 9.5 × 107~1.6 × 108 CFU g−1 sample with LB and NM1 media, respectively) compared with root endosphere samples (1.9 × 104~3.4 × 105 and 1.2~5.1 × 104 CFU g−1 sample) on the same media. Smaller distances (less than one order) (P ≤ 0.05) were observed between on putative N2-fixing culturable bacteria in CRMA medium for rhizosphere samples (2~8.2 × 103 CFU g−1) compared with those from the root endosphere (8.5 × 102~1.9 × 103 CFU g−1) (Figure 2B).
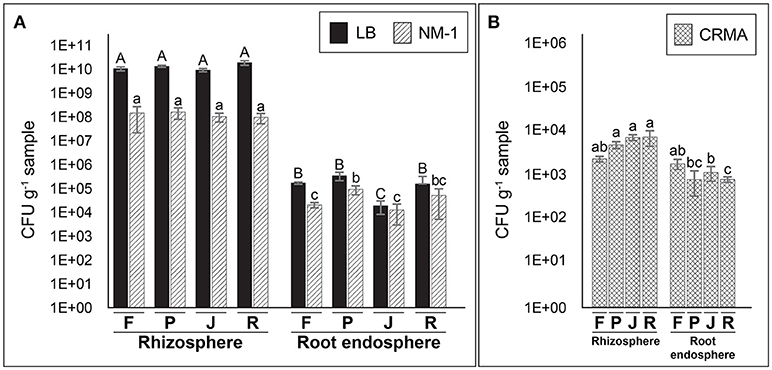
Figure 2. Counts (CFU per g of sample) of total culturable (A) in rhizosphere and root endosphere samples of wheat plants using general (LB and NM−1) and putative N2-fixing bacteria (B) with selective (Congo red malic–acid; CRMA) media. Samples labeled as F, P, J, and R correspond to Feña, Patras, Joker, and Rocky wheat cultivars, respectively. Error bars represent standard deviation and different lower letters denote statistical difference (P ≤ 0.05, Tukey HSD test) (n = 3).
Characterization of Putative N2-Fixing Bacteria
Thirty-eight of 77 strains examined with ERIC-PCR analysis (49.3%) were recognized as non-redundant putative N2-fixing bacterial isolates, whose taxonomic assignments are shown in Table 2. Based on partial sequencing of 16S rRNA genes, most of isolates from the rhizosphere samples had an affiliation with the genus Bacillus (15 of 20 isolates), followed by members of genera Microbacterium (3 isolates), Chitinophaga (1) and Arthrobacter (1) genera. In contrast, only 4 of 18 isolates from the root endosphere samples were characterized as member of the genus Bacillus based on sequencing of 16S rRNA genes. Other isolates were characterized as being members of the genera Roseomonas (3), Mycobacterium (3), Georgenia (2 isolates), Bosea (2), Microbacterium (1), Psychrobacillus (1), Chitinophaga (1) and Leifsonia (1).
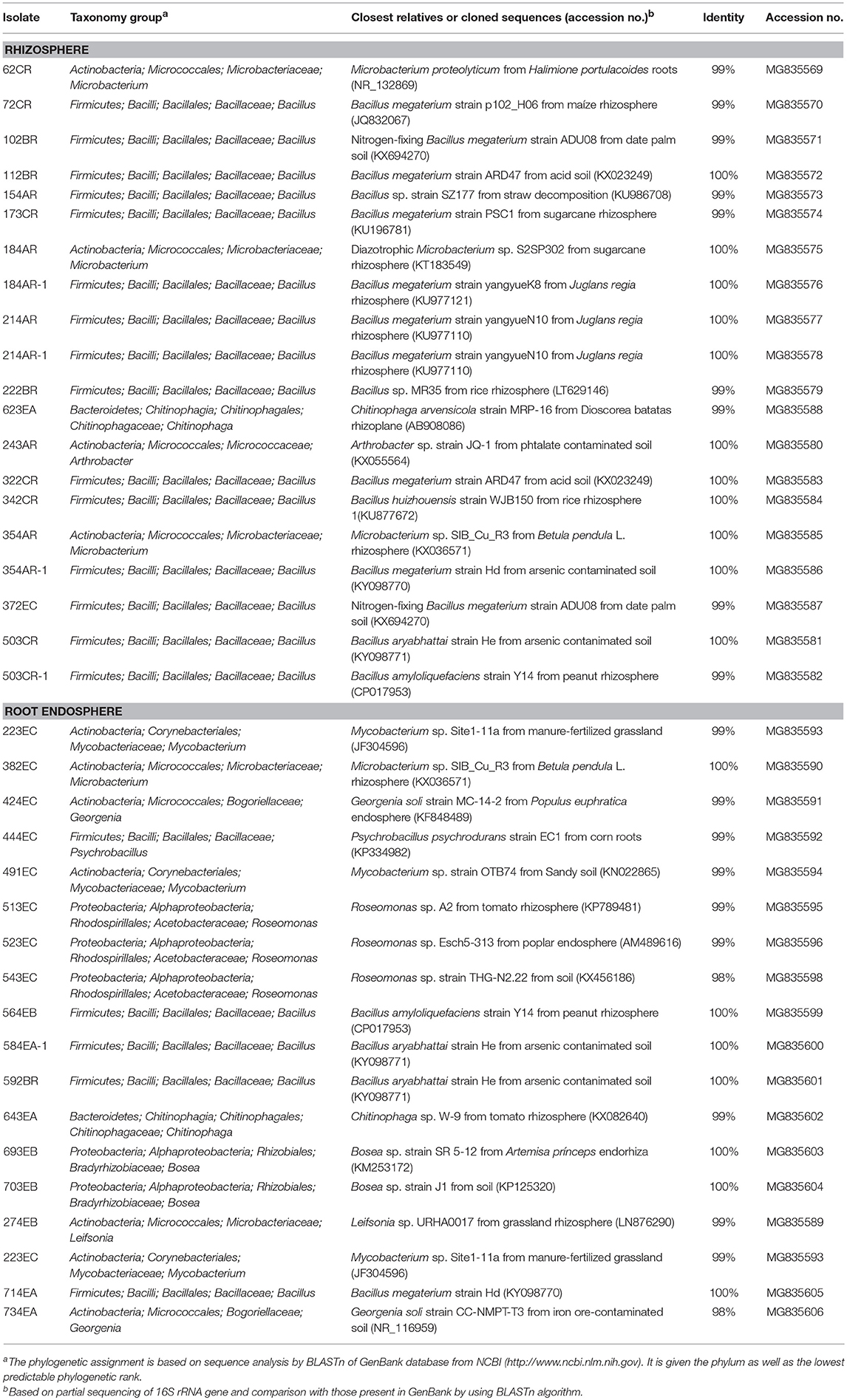
Table 2. Taxonomic identity of putative N2-fixing bacteria isolates obtained by sequencing of 16S rRNA genes.
BLASTx analyses (Table 3) predicted eight amino acid sequences (1 from the rhizosphere and 7 from the root endosphere) that coded the nitrogenase-characteristic P-loop NTPase conserved superfamily domain. The neighbor-joining tree analysis also revealed that our partial predicted nitrogenase-like enzymes showed higher dissimilarities compared with those representatives of nitrogenase enzymes taken from GenBank (Figure 3), except for sequences from Chitinophaga sp. 643EA and Roseomonas sp. 523EC.
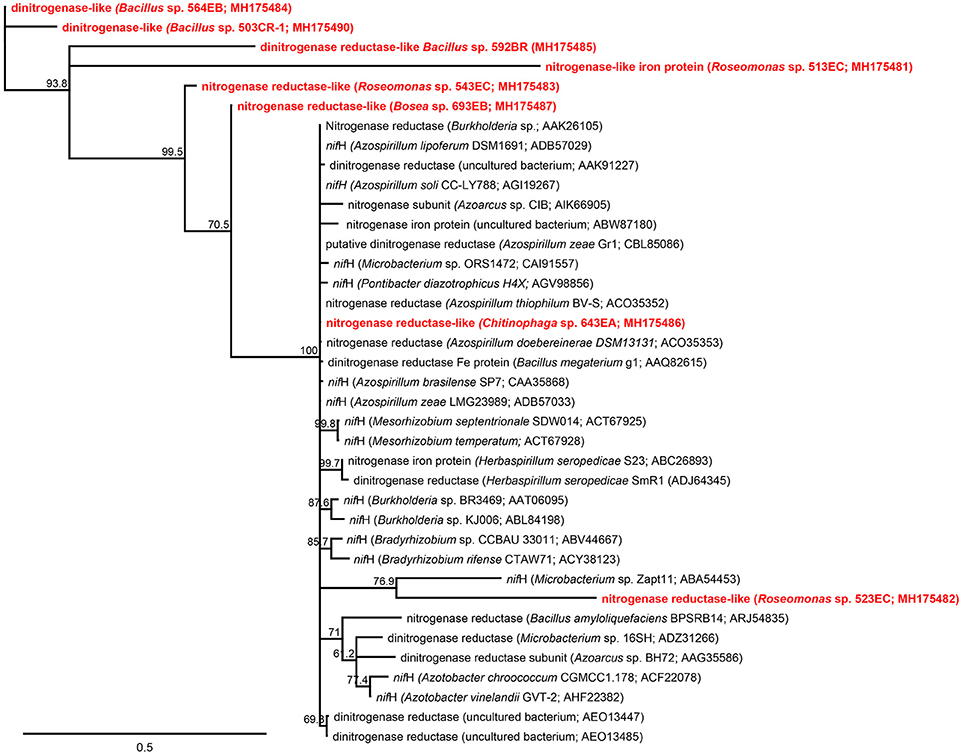
Figure 3. Neighbor-joining tree showing the phylogenetic affiliation between predicted amino acid sequences from nifH gene obtained from rhizosphere and root endosphere isolates in this study (red) and representative nifH-coded enzyme amino acid sequences from known representative plant-soil bacteria deposited in NCBI GenBank database (black). Scale represents substitution sites (Bootstrap = 1,000). In parenthesis is shown the accession number of representative sequences in GenBank or the taxonomic affiliation based on 16S rRNA gene sequencing of isolates.
Fingerprinting of Total and N2-Fixing Bacterial Communities
Fingerprint analysis of bacterial communities by DGGE revealed significant differences (P ≤ 0.05) between the total bacterial communities found in rhizosphere and root endosphere samples from the wheat cultivars examined (Figure 4A). Two clusters were clearly observed at the 40% similarity level. However, a specific bacterial community for each of the wheat cultivars was not observed, even at higher similarity percentages (60%). Similarly, significant differences (P ≤ 0.05) between rhizosphere and root endosphere samples of wheat cultivars with respect to nifH-harboring bacterial populations. However, a specific nifH-harboring bacterial community for each cultivar was not observed at higher similarity (60%), except for the root endosphere samples from wheat cv “Feña” (Figure 4B).
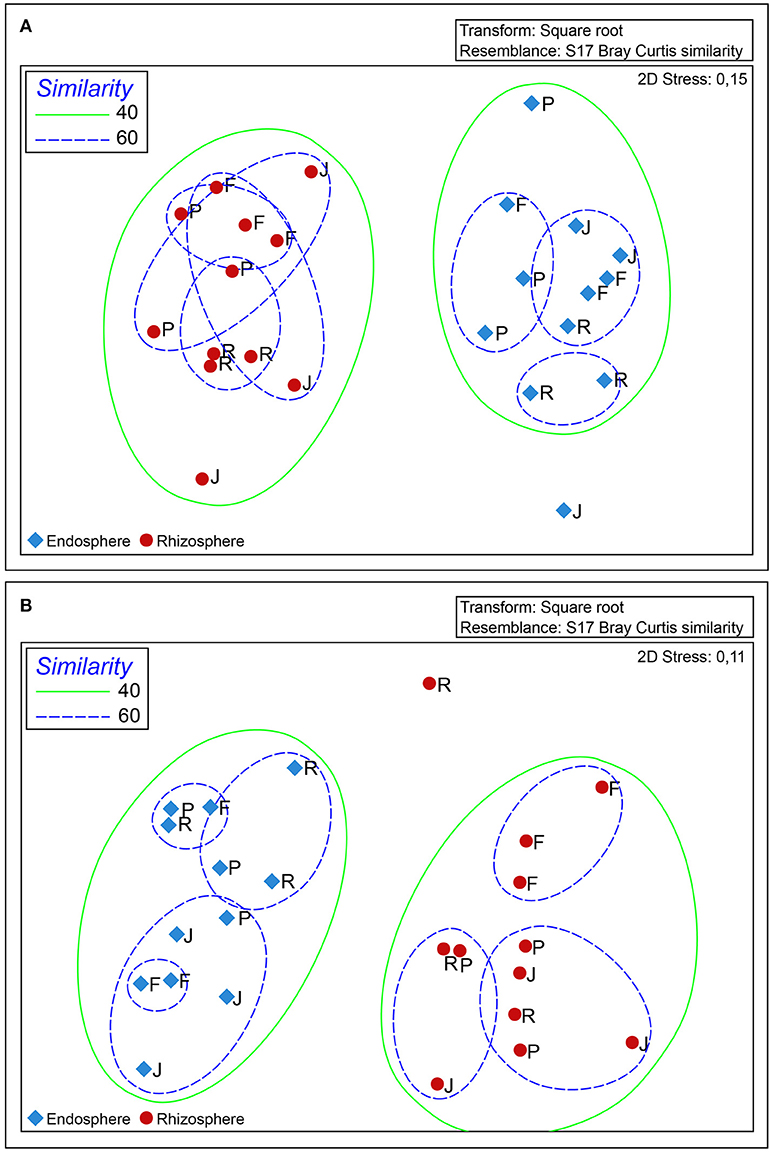
Figure 4. Non-metric multidimensional scaling (nMDS) analysis of DGGE fingerprinting of 16S rRNA (A) and nifH (B) analysis generated by Primer v6 software (http://www.primer-e.com) with the Bray-Curtis similarity index, 5% significance level, and < 0.1 stress values. Red and blue shapes represent rhizosphere and root endosphere, respectively. Samples labelled as F, P, J and R correspond to Feña, Patras, Joker and Rocky wheat cultivars, respectively.
Discussion
Nitrogen is an essential nutrient for plant growth and N2-fixing bacteria play an important role in plant nutrition. Studies focused to N2-fixing bacteria in Chilean agroecosystems are scarce, particularly with respect to cereal cropping systems. By using culture-dependent methods, N2-fixing bacteria have previously been isolated from alfalfa and lupin plants grown in Andisols from southern Chile (Langer et al., 2008; Campos et al., 2014). Culture-independent methods based on partial sequencing of 16S rRNA genes have also revealed the occurrence of Sinorhizobium strains on wheat (Jorquera et al., 2014b) and Azospirillum in ryegrass (Lagos et al., 2014) rhizospheres. In addition, and to our knowledge, no studies on endophytic N2-fixing bacteria in Chilean agroecosystems have not been done so far.
In this study, qPCR revealed the occurrence of nifH-harboring bacterial population in all samples of wheat cultivars analyzed, with a significantly higher abundance of total and N2-fixing bacteria in the rhizosphere, compared with root endosphere samples. Our results showed a large number of copies, 1012~1013, of 16S rRNA genes g−1 are present in rhizosphere soils, which is two orders of magnitude greater than those reported by Sanguin et al. (2009) in rhizosphere soils from wheat. Lower abundances (~108 gene copies g−1) were also reported in wheat rhizospheres by Reardon et al. (2014). The difference between our results and other wheat studies in literature could be attributed to the variability of 16s rRNA copy number of present in environmental bacteria, which could contain as much as 15 copies per cell (Kembel et al., 2012; Větrovský and Baldrian, 2013). Similarly, 16S rRNA copies in cotton plants has been reported around the values that we report here (1012 copies g−1 rhizosphere; Zhang et al., 2016).
In relation to the abundance of nifH genes, the values obtained in this study were close to those reported by Reardon et al. (2014). We found ~106 copies of nifH genes g−1 of rhizosphere soils in wheat plants. In contrast, Bouffaud et al. (2016) reported greater nifH gene abundances (~109 gene copies g−1) in rhizosphere soils of wheat plants.
Reported counts of 16S rRNA genes, by qPCR, in inner tissues or root endosphere of plants are scarce because of the potential for biased results due to the presence of ribosomes in chloroplasts and mitochondria (Shade et al., 2013). The use of the chloroplast- and mitochondria-excluding primer set 799f and 1115r (Shade et al., 2013) has been reported to produce reliable qPCR results on root endosphere samples (Beckers et al., 2016). Our results showed ~ 107-108 copies of 16S rRNA genes g−1 root, which are similar to those obtained by Ruppel et al. (2006) in the rice endosphere. Higher loads of endophytic bacteria (1010~1013 copies of 16S rRNA genes g−1 root) were reported in a rice-maize rotation (Breidenbach et al., 2017). In relation to nifH genes, studies have reported abundances of ~108 copies of nifH genes g−1 root in wheat and rapeseed plants (Bouffaud et al., 2016; Puri et al., 2016). Both studies reported three orders higher nifH gene numbers, compared to those obtained in this study (~105 genes copies g−1 root).
Similar to qPCR results, bacterial numbers obtained by the plate-counting studies done here suggested the occurrence of culturable N2-fixing bacteria in all samples of wheat cultivars analyzed, with a significant higher abundance in rhizosphere compared with root endosphere samples. Our results showed counts of total culturable bacteria of 109~1010 CFU g−1 and 107~108 CFU g−1 on LB and NM-1 media, respectively. In this context, Jorquera et al. (2014b) reported counts of 107 CFU g−1 rhizosphere on cereals (wheat and oats) and pastures (ryegrass) by using NM-1 medium, similar to the results described by Jia et al. (2015) in wheat rhizosphere on meat-peptone agar (108 CFU g−1 rhizosphere). In addition, the counts of culturable bacteria in root endosphere samples we examined (104-105 CFU g−1 root) were similar to those observed on wheat roots by Ruppel (1989) and Robinson et al. (2016) with counts of ~104 CFU g−1 root in wheat.
Our counts of putative N2-fixing bacteria in the rhizosphere (103 CFU g−1) of wheat were lower than those reported in the wheat (104 CFU g−1), chickpea and sugarcane (104-105 CFU g−1) rhizospheres examined by Pathania et al. (2014) and Ahmad et al. (2006). This may, in part, due to different culture conditions used in each study. However, our counts of endospheric N2-fixing bacteria (103 CFU g−1 root) are similar to those obtained by Ruppel (1989) (104 CFU g−1 root) in wheat and Patel and Archana (2017) in several Poaceae plant tissues (103-105 CFU g−1 root). Most studies on N2-fixing bacterial communities in the wheat root endosphere have examined colonization niches and physiological effect of different diazotrophic endophytes (Liu et al., 2017a,b), instead of determining how abundant are the N2-fixing root endosphere communities. That said, however, it is well known that culture medium type greatly affects the reported numbers of bacteria obtained via plate-counting. Media bias is always an issue in examining microbiota in environmental niches. In this context it has been described that the use of diluted of culture media improves CFU number determination and enhance isolation of N2-fixing bacteria (Janssen et al., 2002; Hashimoto et al., 2009). Despite this limitation, however, our analyses do allow relative comparisons of total and N2-fixing microbes in the plant compartments we examined.
Sequencing of 16S rRNA genes of rhizosphere isolates revealed the occurrence of members of genera Bacillus, Microbacterium, Chitinophaga, and Arthrobacter. Most of isolates were characterized as belonging to the genus Bacillus, which is a common inhabitant in the rhizosphere soil of plants grown in Andisols from southern Chile (Acuña and Jorquera, 2011; Martínez et al., 2011). In this context, diazotrophic Bacillus sp. strains have been shown to be associated with N2-fixation in wheats (Pathania et al., 2014), as well as sugarcane (Madhaiyan et al., 2011). It is noteworthy that most of isolates characterized as Bacillus (10 of 15) were phylogenetically close to Bacillus megaterium, a well-known N2-fixing and phosphate-solubilizing bacterium commonly studied as plant growth-promoting bacteria (Ding et al., 2005; Elkoca et al., 2007). The remaining five rhizosphere isolates we examined were characterized as Microbacterium, Chitinophaga, and Arthrobacter sp. strains, these microorganisms were previously proposed to be associated with N2-fixation in the rhizospheres of other plant species (Mirza and Rodrigues, 2012; Beneduzi et al., 2013; Moyes et al., 2016).
Our results also showed the occurrence of members of the genera Bacillus, Georgenia, Mycobacterium, Bosea, Microbacterium, Psychrobacillus, Roseomonas, Chitinophaga, and Leifsonia genera in the root endosphere of wheat. Bacteria belonging to the phylum Actinobacteria, such as members of the genera Georgenia, Mycobacterium, and Leifsonia, have been described as common inhabitants of the root endosphere of plants, as well as have many diazotrophs (Mårtensson et al., 2009; Han et al., 2011; Liaqat and Eltem, 2016). It is noteworthy, that the isolation of Proteobacteria belonging to the genera Bosea and Roseomonas have not been previously reported either soils or in planta in Chile. Isolates characterized as Bosea have been isolated from lupin root nodules by De Meyer and Willems (2012). In this context, Bosea spp. appear to be related to bacteria within the genus Rhizobium, well known for forming N2-fixation symbioses with legumes worldwide, as well as with plants grown in acidic soils in Chile (Langer et al., 2008).
In addition, despite that our analysis with BLASTx suggested the presence of the nitrogenase enzyme in genomes of our isolates; the neighbor-joining tree analysis did not show a high similarity when our predicted nitrogenase-like enzymes were compared with representative nitrogenases taken from GenBank. This result also might explain the low specificity of universal primer sets found in the literature and used in this study (Supplementary Table 1), which could not adequately cover the nitrogenases harbored by native bacteria living in Chilean Andisols. Accordingly, and as discussed by Gaby and Buckley (2012), while several universal primers have been designed and empirically tested for nitrogenase, some of them can generate false positive reactions; and therefore, primers must be used with caution and validated with genomic DNA from phylogenetically diverse N2-fixing strains from different environments.
Likewise, members of the genus Roseomonas have been recognized as a PGPB found in a wide variety of environments, including the in rhizospheres of rice (Ramaprasad et al., 2015) and Chinese cabbage (Kim and Ka, 2014), and in contaminated soils (Chen et al., 2014). In this study, we noted that there were large differences in microbiota present in rhizosphere and root endosphere samples. Similarly, Robinson et al. (2016) found differences in endophytic bacteria between roots and leaves, which were attributed to tissue type, phenological stage of plants, and soil nutrient availability. Bouffaud et al. (2016) also proposed that plants recruit its own N2-fixing endophytic microbiome. These differences in the composition and structure of bacterial communities between rhizosphere and root endosphere were also confirmed by DGGE. Our results suggest a compartmentalization between rhizosphere and root endosphere for both studied communities (16s rRNA and nifH). Such separation has been described as being common in plants (Mahaffee and Kloepper, 1997) and we propose that these differences might also be influenced by a combination of different factors, including soil composition (pH, organic matter, and nutrients), soil management (fertilization, rotation, and tillage) and plant (genotype, phonological stages, and defense mechanisms) and the presence of other microbial communities (fungi, nematode, and protozoa).
In Chilean Andisols, as wells as other agroecosystems, our knowledge on N2-fixing bacterial populations associated with plants is very limited. In this sense, based on the relevance of plant microbiome upon fitness and production of crops, an exhaustive study on the abundance, diversity and activity of N2-fixing bacterial populations could be essential to the develop of novel fertilizers and management agronomic strategies to improve the efficiency of N fertilization in the field with the consequent low cost for the farmers and environmental benefits.
Author Contributions
JR performed experiments and developed the manuscript. JA, MS, and MJ contributed to statistical and elaboration of the article.
Funding
This study was funded in part by grants from the Chilean government: FONDECYT Regular No. 1160302 and FONDECYT Initiation into Research No. 11160112, and by the University of Minnesota Agricultural Experiment Station (to MS). Joaquín Rilling acknowledges the support of CONICYT Doctoral Scholarship program, Grant No. 21150794.
Conflict of Interest Statement
The authors declare that the research was conducted in the absence of any commercial or financial relationships that could be construed as a potential conflict of interest.
Supplementary Material
The Supplementary Material for this article can be found online at: https://www.frontiersin.org/articles/10.3389/fmicb.2018.02710/full#supplementary-material
References
Acuña, J., and Jorquera, M. (2011). Indole acetic acid and phytase activity produced by rhizosphere bacilli as affected by pH and metals. J. Soil Sci. Plant Nutr. 11, 1–12. doi: 10.4067/S0718-95162011000300001
Ahmad, F., Ahmad, I., Aqil, F., Wani, A., and Sousche, Y. (2006). Plant growth promoting potential of free-living diazotrophs and other rhizobacteria isolated from Northern Indian soil. Biotechnol. J. 1, 1112–1123. doi: 10.1002/biot.200600132
Baldani, J., Reis, V., Videira, S., Boddey, L., and Baldani, V. (2014). The art of isolating nitrogen-fixing bacteria from non-leguminous plants using N-free semi-solid media: a practical guide for microbiologists. Plant Soil 384, 413–431. doi: 10.1007/s11104-014-2186-6
Beckers, B., Op De Beeck, M., Thijs, S., Truyens, S., Weyens, N., Boerjan, W., et al. (2016). Performance of 16s rDNA primer pairs in the study of rhizosphere and endosphere bacterial microbiomes in metabarcoding studies. Front. Microbiol. 7:650. doi: 10.3389/fmicb.2016.00650
Beneduzi, A., Moreira, F., Costa, P., Vargas, L., Lisboa, B., Favreto, R., et al. (2013). Diversity and plant growth promoting evaluation abilities of bacteria isolated from sugarcane cultivated in the South of Brazil. Appl. Soil Ecol. 63, 94–104. doi: 10.1016/j.apsoil.2012.08.010
Berg, G., Grube, M., Schloter, M., and Smalla, K. (2014). Unraveling the plant microbiome: looking back and future perspectives. Front. Microbiol. 5:148. doi: 10.3389/fmicb.2014.00148
Bertsch, P., and Bloom, P. (1996). “Aluminum,” in Methods of Soil Analysis, Part 3: Chemical Methods (Madison, WI: Soil Science Society of America, American Society of Agronomy Madison), 517–550.
Bhattacharyya, P., and Jha, D. (2012). Plant growth-promoting rhizobacteria (PGPR): emergence in agriculture. World J. Microbiol. Biotechnol. 28, 1327–1350. doi: 10.1007/s11274-011-0979-9
Bouffaud, M., Renoud, S., Moenne-Loccoz, Y., and Muller, D. (2016). Is plant evolutionary history impacting recruitment of diazotrophs and nifH expression in the rhizosphere? Sci. Rep. 6, 1–9. doi: 10.1038/srep21690
Breidenbach, B., Brenzinger, K., Brandt, F., Blaser, M., and Conrad, R. (2017). The effect of crop rotation between wetland rice and upland maize on the microbial communities associated with roots. Plant Soil 419, 435–445. doi: 10.1007/s11104-017-3351-5
Bürgmann, H., Widmer, F., Sigler, W. V., and Zeyer, J. (2003). mRNA extraction and reverse transcription-PCR protocol for detection of nifH gene expression by Azotobacter vinelandii in soil. Appl. Environ. Microbiol. 69, 1928–1935. doi: 10.1128/AEM.69.4.1928-1935.2003
Campos, D., Acevedo, F., Morales, E., Aravena, J., Amiard, V., Jorquera, M., et al. (2014). Microencapsulation by spray drying of nitrogen-fixing bacteria associated with lupin nodules. World J. Microbiol. Biotechnol. 30, 2371–2378. doi: 10.1007/s11274-014-1662-8
Chen, Q., Sun, L., Zhang, X., He, J., Kwon, S., Zhang, J., et al. (2014). Roseomonas rhizosphaerae sp. nov., a triazophos-degrading bacterium isolated from soil. Int. J. Syst. Evol. Microbiol. 64, 1127–1133. doi: 10.1099/ijs.0.057000-0
Clarke, K. (1993). Non-parametric multivariate analyses of changes in community structure. Austr. J. Ecol. 18, 117–143. doi: 10.1111/j.1442-9993.1993.tb00438.x
Clarke, K., Somerfield, P. J., and Gorley, R. N. (2008). Testing of null hypotheses in exploratory community analyses: similarity profiles and biota-environment linkage. J. Exp. Mar. Biol. Ecol. 366, 56–69. doi: 10.1016/j.jembe.2008.07.009
de Bruijn, F. (2015). Biological Nitrogen Fixation. Hoboken, NJ: Wiley Blackwell. doi: 10.1002/9781119053095
De Meyer, S. E., and Willems, A. (2012). Multilocus sequence analysis of Bosea species and description of Bosea lupini sp. nov., Bosea lathyri sp. nov. and Bosea robiniae sp. nov., isolated from legumes. Int. J. Syst. Evol. Microbiol. 62, 2505–2510. doi: 10.1099/ijs.0.035477-0
De-la-Peña, C., and Loyola-Vargas, V. M. (2014). Biotic interactions in the rhizosphere: a diverse cooperative enterprise for plant productivity. Plant Physiol. 166, 701–719. doi: 10.1104/pp.114.241810
Ding, Y., Wang, J., Liu, Y., and Chen, S. (2005). Isolation and identification of nitrogen-fixing bacilli from plant rhizospheres in Beijing region. J. Appl. Microbiol. 99, 1271–1281. doi: 10.1111/j.1365-2672.2005.02738.x
Durán, P., Acuña, J. J., Jorquera, M. A., Azcón, R., Paredes, C., Rengel, Z., et al. (2014). Endophytic bacteria from selenium-supplemented wheat plants could be useful for plant-growth promotion, biofortification and Gaeumannomyces graminis biocontrol in wheat production. Biol. Fertil. Soils 50, 983–990. doi: 10.1007/s00374-014-0920-0
Elkoca, E., Kantar, F., and Sahin, F. (2007). Influence of nitrogen fixing and phosphorus solubilizing bacteria on the nodulation, plant growth, and yield of chickpea. J. Plant Nutr. 31, 157–171. doi: 10.1080/01904160701742097
Gaby, J., and Buckley, D. (2012). A comprehensive evaluation of PCR primers to amplify the nifH gene of nitrogenase. PLoS ONE 7:e42149. doi: 10.1371/journal.pone.0042149
Han, J., Song, Y., Liu, Z., and Hu, Y. (2011). Culturable bacterial community analysis in the root domains of two varieties of tree peony (Paeonia ostii). FEMS Microbiol. Lett. 322, 15–24. doi: 10.1111/j.1574-6968.2011.02319.x
Hartmann, A., and Baldani, J. (2006). “The genus Azospirillum,” in The Prokaryotes, Vol. 5, ed M. Dworkin (New York, NY: Springer), 115–140. doi: 10.1007/0-387-30745-1_6
Hashimoto, T., Koga, M., and Masaoka, Y. (2009). Advantages of a diluted nutrient broth medium for isolating N2-producing denitrifying bacteria of α-Proteobacteria in surface and subsurface upland soils. Soil Sci. Plant Nutr. 55, 647–659. doi: 10.1111/j.1747-0765.2009.00404.x
Janssen, P., Yates, P., Grinton, B., Taylor, P., Sait, M., Janssen, P., et al. (2002). Improved culturability of soil bacteria and isolation in pure culture of novel members of the divisions Acidobacteria, Actinobacteria, Proteobacteria, and Verrucomicrobia. Appl. Environ. Microbiol. 68, 2391–2396. doi: 10.1128/AEM.68.5.2391-2396.2002
Jia, X., Zhao, Y., Wang, W., and He, Y. (2015). Elevated temperature altered photosynthetic products in wheat seedlings and organic compounds and biological activity in rhizosphere soil under cadmium stress. Sci. Rep. 5:14426. doi: 10.1038/srep14426
Jorquera, M., Inostroza, N., Lagos, L., Barra, P., Marileo, L., Rilling, J., et al. (2014a). Bacterial community structure and detection of putative plant growth-promoting rhizobacteria associated with plants grown in Chilean agro-ecosystems and undisturbed ecosystems. Biol. Fertil. Soils 50, 1141–1153. doi: 10.1007/s00374-014-0935-6
Jorquera, M., Martínez, O., Marileo, L., Acuña, J., Saggar, S., and Mora, M. (2014b). Effect of nitrogen and phosphorus fertilization on the composition of rhizobacterial communities of two Chilean Andisol pastures. World J. Microbiol. Biotechnol. 30, 99–107. doi: 10.1007/s11274-013-1427-9
Jorquera, M., Shaharoona, B., Nadeem, S., De, M., Mora, L., Crowley, D., et al. (2012). Plant growth-promoting rhizobacteria associated with ancient clones of creosote bush (Larrea tridentata). Microb. Ecol. 64, 1008–1017. doi: 10.1007/s00248-012-0071-5
Kearse, M., Moir, R., Wilson, A., Stones-Havas, S., Cheung, M., Sturrock, S., et al. (2012). Geneious Basic: an integrated and extendable desktop software platform for the organization and analysis of sequence data. Bioinformatics 28, 1647–1649. doi: 10.1093/bioinformatics/bts199
Kembel, S., Wu, M., Eisen, J., and Green, J. (2012). Incorporating 16S gene copy number information improves estimates of microbial diversity and abundance. PLoS Comput. Biol. 8:e1002743. doi: 10.1371/journal.pcbi.1002743
Kim, D., and Ka, J. (2014). Roseomonas soli sp. nov., isolated from an agricultural soil cultivated with Chinese cabbage (Brassica campestris). Int. J. Syst. Evol. Microbiol. 64, 1024–1029. doi: 10.1099/ijs.0.053827-0
Lagos, L., Navarrete, O., Maruyama, F., Crowley, D., Cid, F., Mora, M., et al. (2014). Bacterial community structures in rhizosphere microsites of ryegrass (Lolium perenne var. Nui) as revealed by pyrosequencing. Biol. Fertil. Soils 50, 1253–1266. doi: 10.1007/s00374-014-0939-2
Lane, D. (1991). “16S/23S sequencing,” in Nucleic Acid Techniques in Bacterial Systematics, eds E. Stackebrandt and M. Goodfellow (New York, NY: John Wiley and Sons), 115–175.
Langer, H., Nandasena, K. G., Howieson, J. G., Jorquera, M., and Borie, F. (2008). Genetic diversity of Sinorhizobium meliloti associated with alfalfa in Chilean volcanic soils and their symbiotic effectiveness under acidic conditions. World J. Microbiol. Biotechnol. 24, 301–308. doi: 10.1007/s11274-007-9471-y
Larkin, M., Blackshields, G., Brown, N., Chenna, R., McGettigan, P., McWilliam, H., et al. (2007). Clustal W and Clustal X version 2.0. Bioinformatics 23, 2947–2948. doi: 10.1093/bioinformatics/btm404
Laval, E., and Garcia, L. (2018). Cereales: producción, precios y comercio exterior de trigo, maíz y arroz. Oficina de Estudios y Políticas Agrarias (ODEPA), Ministerio de Agricultura, Gobierno de Chile, 4–24.
Liaqat, F., and Eltem, R. (2016). Identification and characterization of endophytic bacteria isolated from in vitro cultures of peach and pear rootstocks. 3 Biotech 6, 1–8. doi: 10.1007/s13205-016-0442-6
Liu, H., Wang, X., Qi, H., Wang, Q., Chen, Y., Li, Q., et al. (2017a). The infection and impact of Azorhizobium caulinodans ORS571 on wheat (Triticum aestivum L.). PLoS ONE 12:e0187947. doi: 10.1371/journal.pone.0187947
Liu, H., Zhang, L., Meng, A., Zhang, J., Xie, M., Qin, Y., et al. (2017b). Isolation and molecular identification of endophytic diazotrophs from seeds and stems of three cereal crops. PLoS ONE 12:e0187383. doi: 10.1371/journal.pone.0187383
Mårtensson, L., Díez, B., Wartiainen, I., Zheng, W., El-Shehawy, R., and Rasmussen, U. (2009). Diazotrophic diversity, nifH gene expression and nitrogenase activity in a rice paddy field in Fujian, China. Plant Soil 325, 207–218. doi: 10.1007/s11104-009-9970-8
Madhaiyan, M., Poonguzhali, S., Lee, J. S., Lee, K., and Hari, K. (2011). Bacillus rhizosphaerae sp. nov., an novel diazotrophic bacterium isolated from sugarcane rhizosphere soil. Antonie van Leeuwenhoek 100, 437–444. doi: 10.1007/s10482-011-9600-3
Mahaffee, W., and Kloepper, J. (1997). Bacterial communities of the rhizosphere and endorhiza associated with field-grown cucumber plants inoculated with a plant growth-promoting rhizobacterium or its genetically modified derivative. Can. J. Microbiol. 43, 344–353. doi: 10.1139/m97-048
Martínez, O., Jorquera, M., Crowley, D., and Luz Mora, M. (2011). Influence of nitrogen fertilisation on pasture culturable rhizobacteria occurrence and the role of environmental factors on their potential PGPR activities. Biol. Fertil. Soils 47, 875–885. doi: 10.1007/s00374-011-0593-x
Mirza, B., and Rodrigues, J. (2012). Development of a direct isolation procedure for free-living diazotrophs under controlled hypoxic conditions. Appl. Environ. Microbiol. 78, 5542–5549. doi: 10.1128/AEM.00714-12
Moyes, A., Kueppers, L., Pett-Ridge, J., Carper, D., Vandehey, N., O'Neil, J., et al. (2016). Evidence for foliar endophytic nitrogen fixation in a widely distributed subalpine conifer. New Phytologist 210, 657–668. doi: 10.1111/nph.13850
Murphy, J., and Riley, J. P. (1962). A modified single solution method for the determination of phosphate in natural waters. Anal. Chim. Acta 27, 31–36. doi: 10.1016/S0003-2670(00)88444-5
Nakamura, K., Hiraishi, A., Yoshimi, Y., Kawaharasaki, M., Masuda, K., and Kamagata, Y. (1995). Microlunatus phosphovorus gen. nov., sp. nov., a new gram-positive polyphosphate-accumulating bacterium isolated from activated sludge. Int. J. Syst. Bacteriol. 45, 17–22. doi: 10.1099/00207713-45-1-17
Nuñez, P., Demanet, R., Misselbrook, T., Alfaro, M., and Mora, M. (2010). Nitrogen Losses under Different Cattle Grazing Frequencies and Intensities in a Volcanic Soil of Southern Chile. Chilean J. Agri. Res. 70, 237–250. doi: 10.4067/S0718-58392010000200007
Ohyama, T., Momose, A., Ohtake, N., Sueyoshi, K., Sato, T., Nakanishi, Y., et al. (2014). “Nitrogen fixation in sugarcane,” in Advances in Biology and Ecology of Nitrogen Fixation, ed T. Ohyama (Tokyo: InTech), 50–70. doi: 10.5772/56993
Patel, J., and Archana, G. (2017). Diverse culturable diazotrophic endophytic bacteria from Poaceae plants show cross-colonization and plant growth promotion in wheat. Plant Soil 417, 99–116. doi: 10.1007/s11104-017-3244-7
Pathania, N., Gosal, S., Saroa, G., and Vikal, Y. (2014). Molecular characterization of diazotrophic bacteria isolated from rhizosphere of wheat cropping system from central plain region of Punjab. Afr. J. Microbiol. Res. 8, 862–871. doi: 10.5897/AJMR2013.5948
Puri, A., Padda, K., and Chanway, C. (2016). Evidence of nitrogen fixation and growth promotion in canola (Brassica napus L.) by an endophytic diazotroph Paenibacillus polymyxa P2b-2R. Biol. Fertil. Soils 52, 119–125. doi: 10.1007/s00374-015-1051-y
Ramaprasad, E., Sasikala, C., and Ramana, C. (2015). Roseomonas oryzae sp. nov., isolated from paddy rhizosphere soil. Int. J. Syst. Evol. Microbiol. 65, 3535–3540. doi: 10.1099/ijsem.0.000449
Reardon, C., Gollany, H., and Wuest, S. (2014). Diazotroph community structure and abundance in wheat-fallow and wheat-pea crop rotations. Soil Biol. Biochem. 69, 406–412. doi: 10.1016/j.soilbio.2013.10.038
Robinson, R., Fraaije, B., Clark, I., Jackson, R., Hirsch, P., and Mauchline, T. (2016). Endophytic bacterial community composition in wheat (Triticum aestivum) is determined by plant tissue type, developmental stage and soil nutrient availability. Plant Soil 405, 381–396. doi: 10.1007/s11104-015-2495-4
Rodriguez-Caceres, E. (1982). Improved medium for isolation of Azospirillum spp. Appl. Environ. Microbiol. 44, 990–991.
Ruppel, S. (1989). “Isolation and characterization of dinitrogen-fixing bacteria from the rhizosphere of Triticum aestivum and Ammophila arenaria,” in Developments in Soil Science, eds V. Vančura and F. Kunc (Prague: Elsevier), 253–262.
Ruppel, S., Rühlmann, J., and Merbach, W. (2006). Quantification and localization of bacteria in plant tissues using quantitative real-time PCR and online emission fingerprinting. Plant Soil 286, 21–35. doi: 10.1007/s11104-006-9023-5
Sambrook, J., and Russell, D. (2001). “Analysis of gene expression in cultured mammalian cells,” in Molecular Cloning: A Laboratory Manual, Vol. 3, eds J. Sambrook and D. Russel (New York, NY: Cold Spring Harbor Press), 9–10.
Sanguin, H., Sarniguet, A., Gazengel, K., Moënne-Loccoz, Y., and Grundmann, G. (2009). Rhizosphere bacterial communities associated with disease suppressiveness stages of take-all decline in wheat monoculture. New Phytol. 184, 694–707. doi: 10.1111/j.1469-8137.2009.03010.x
Shade, A., McManus, P., and Handelsman, J. (2013). Unexpected diversity during community succession in the apple. MBio 4, 1–12. doi: 10.1128/mBio.00602-12
Tanaka, T., Kawasaki, K., Daimon, S., Kitagawa, W., Yamamoto, K., Tamaki, H., et al. (2014). A hidden pitfall in the preparation of agar media undermines microorganism cultivability. Appl. Environ. Microbiol. 80, 7659–7666. doi: 10.1128/AEM.02741-14
Turner, T., James, E., and Poole, P. (2013). The plant microbiome. Adv. Bot. Res. 69, 279–309. doi: 10.1186/gb-2013-14-6-209
Versalovic, J., Koeuth, T., and Lupski, J. R. (1991). Distribution of repetitive DNA sequences in eubacteria and application to fingerprinting of bacterial genomes. Nucleic Acids Res. 19, 6823–6831. doi: 10.1093/nar/19.24.6823
Větrovský, T., and Baldrian, P. (2013). The variability of the 16S rRNA gene in bacterial genomes and its consequences for bacterial community analyses. PLoS ONE 8:e57923. doi: 10.1371/journal.pone.0057923
Warncke, D., and Brown, J. R. (1998). “Potassium and other basic cations,” in Recommended Chemical Soil Test Procedures for the North Central Region, NCR Publication No. 221, Missouri Agricultural Experiment Station SB 1001, ed J. R. Brown (Columbia: NCR Publications), 31–33.
Wilson, K. (2001). “Preparation of Genomic DNA from Bacteria,” in Current Protocols in Molecular Biology, Vol. 56, ed F. M. Ausubel (Hoboken, NJ: John Wiley & Sons, Inc.), 2.4.1–2.4.5. doi: 10.1002/0471142727.mb0204s56
Keywords: Andisol, root endosphere, N2-fixing bacteria, rhizosphere, wheat
Citation: Rilling JI, Acuña JJ, Sadowsky MJ and Jorquera MA (2018) Putative Nitrogen-Fixing Bacteria Associated With the Rhizosphere and Root Endosphere of Wheat Plants Grown in an Andisol From Southern Chile. Front. Microbiol. 9:2710. doi: 10.3389/fmicb.2018.02710
Received: 26 June 2018; Accepted: 23 October 2018;
Published: 20 November 2018.
Edited by:
Benjamin Gourion, Plant Interactions Laboratory Microorganisms (LIPM), FranceReviewed by:
Andrzej Tkacz, University of Oxford, United KingdomFeth-el-zahar Haichar, Microbial Ecology, France
Copyright © 2018 Rilling, Acuña, Sadowsky and Jorquera. This is an open-access article distributed under the terms of the Creative Commons Attribution License (CC BY). The use, distribution or reproduction in other forums is permitted, provided the original author(s) and the copyright owner(s) are credited and that the original publication in this journal is cited, in accordance with accepted academic practice. No use, distribution or reproduction is permitted which does not comply with these terms.
*Correspondence: Milko A. Jorquera, milko.jorquera@ufrontera.cl