- 1Intercollege Graduate Degree Program in Plant Biology, Pennsylvania State University, University Park, PA, United States
- 2Department of Plant Pathology and Environmental Microbiology, Pennsylvania State University, University Park, PA, United States
- 3Genetics Department, Faculty of Agriculture, Tanta University, Tanta, Egypt
- 4State Key Laboratory of Mycology, Institute of Microbiology, Chinese Academy of Sciences, Beijing, China
- 5Plant Protection Department, Ilam University, Ilam, Iran
Certain Trichoderma strains protect plants from diverse pathogens using multiple mechanisms. We report a novel mechanism that may potentially play an important role in Trichoderma-based biocontrol. Trichoderma virens and T. viride significantly increased the amount/activity of secreted antifungal metabolites in response to volatile compounds (VCs) produced by 13 strains of Fusarium oxysporum, a soilborne fungus that infects diverse plants. This response suggests that both Trichoderma spp. recognize the presence of F. oxysporum by sensing pathogen VCs and prepare for attacking pathogens. However, T. asperellum did not respond to any, while T. harzianum responded to VCs from only a few strains. Gene expression analysis via qPCR showed up-regulation of several biocontrol-associated genes in T. virens in response to F. oxysporum VCs. Analysis of VCs from seven F. oxysporum strains tentatively identified a total of 28 compounds, including six that were produced by all of them. All four Trichoderma species produced VCs that inhibited F. oxysporum growth. Analysis of VCs produced by T. virens and T. harzianum revealed the production of compounds that had been reported to display antifungal activity. F. oxysporum also recognizes Trichoderma spp. by sensing their VCs and releases VCs that inhibit Trichoderma, suggesting that both types of VC-mediated interaction are common among fungi.
Introduction
The global need for quality food, feed, and fiber will continue to increase. Efforts to meet this need face multiple complex challenges, one of which is how to protect crop plants from diverse diseases and pests without heavily relying on synthetic pesticides. Due to the multi-faceted negative impact of synthetic pesticides, we cannot afford to keep expanding their use. Biocontrol agents (BCAs) have long been touted as an environment-friendly alternative to synthetic pesticides. However, the following hurdles hinder the full realization of their potential: (a) inconsistent efficacy under different production systems and environmental conditions; (b) limited knowledge about underlying reasons for biocontrol failures; and (c) poor understanding of how BCAs work in light of complex and varied biotic and abiotic factors (Mazzola and Freilich, 2016; Timmusk et al., 2017). Until we convincingly demonstrate that biocontrol can work reliably and effectively, biocontrol will continue to be perceived by risk-averse growers as a risky and high-cost practice.
Several Trichoderma species have been marketed as bio-fungicides against diverse crop pathogens or as bio-fertilizers (Woo et al., 2014). Trichoderma BCAs have been shown to control pathogens via multiple synergistic mechanisms, including mycoparasitism, antibiosis, competition for space and nutrients, and induction of plant systemic resistance (Howell, 2003; Harman et al., 2004; Harman, 2006; Shoresh et al., 2010; Sawant, 2014; Woo et al., 2014). Compared to these mechanisms, relatively little is known about how Trichoderma BCAs recognize pathogens. The prevailing model is that bioactive molecules diffused from pathogens activate the biocontrol machinery (Druzhinina et al., 2011). Considering that water may not be readily available to mediate this form of recognition in the soil, waterborne molecules may not be the only signals. In this study, we investigated if and how volatile compounds (VCs) from both the Trichoderma and pathogen sides affect their interaction. Since previous studies already showed strong inhibition of plant pathogenic fungi such as Fusarium oxysporum, Rhizoctonia solani, Sclerotium rolfsii, Sclerotinia sclerotiorum, and Alternaria brassicicola by Trichoderma VCs (Amin et al., 2010; Meena et al., 2017), we focused on investigating how pathogen VCs affect Trichoderma using F. oxysporum, a soilborne fungal species complex consisting of genetically and phenotypically diverse members (O'Donnell et al., 2009; Kang et al., 2014). Pathogenic F. oxysporum strains collectively infect many economically important plants, causing vascular wilt, root rot, or damping-off diseases (Nelson, 1981; Beckman, 1987; Gordon and Martyn, 1997; Sharma and Muehlbauer, 2007).
Due to their ability to move through the air, VCs perform many crucial functions in animals and plants. Many olfactory receptors (~350 in humans and ~1,000 in mice) are produced by animals (Buck, 2004) because their survival heavily depends on the ability to track foods, find mates, and recognize threats via volatile cues. Plant VCs function as homing cues to pollinators, seed dispersers, and parasitoids (Baldwin, 2010; Herrmann, 2010), mediate communication within and between plants (Baldwin, 2010), and protect plants from diverse predators (Baldwin, 2010; Huang et al., 2012; Arasimowicz-Jelonek and Floryszak-Wieczorek, 2014). Similarly, microbial VCs appear to participate in microbial interactions (Bailly and Weisskopf, 2012; Bennett et al., 2012; Bitas et al., 2013) and also affect plant growth, development, and stress resistance (Bailly and Weisskopf, 2012; Bitas et al., 2013; Li et al., 2016; Li and Kang, 2018). VCs produced by biocontrol agent Paenibacillus polymyxa and soilborne fungal pathogen Verticillium longisporum appeared to affect the production of antimicrobial VCs and other metabolites in the other side (Rybakova et al., 2017). VCs from Aspergillus flavus and Ralstonia solanacearum, soilborne fungal and bacterial pathogens, respectively, affected multiple traits of the other side (Spraker et al., 2014). Here, we report that besides antibiosis, some VCs from both Trichoderma and F. oxysporum likely function as signals in their interactions.
Materials and Methods
Fungal Cultures and Growth Conditions
The following Trichoderma strains were cultured from commercial biocontrol products: (a) T. virens G-41 from RootShield® Plus+ (BioWorks, Victor, NY, United States), (b) T. harzianum T-22 from RootShield® (BioWorks), (c) T. asperellum ICC 012 from BIO-TAM® 2.0 (Isagro, Morrisville, NC, United States), and (d) T. harzianum and T. viride from Custom GP (Custom Biologicals, Boca Raton, FL, United States). Genomic DNA from these strains was extracted as previously described (Cassago et al., 2002) to confirm identity by sequencing the ITS regions. Table 1 lists the F. oxysporum strains used. Fungal strains, preserved as spore suspension in 20% glycerol at −80°C, were activated by inoculating on 0.5x potato dextrose agar (PDA; Difco, Houston, TX). For all the experiments described below, a plug of culture (4 mm in diameter), cut from the actively growing margin of stock culture using a cork borer, was placed on 15 mL 0.75x PDA. We only used one incubator for each experiment to avoid potential variation caused by using multiple incubators. We randomly placed VC-treated and control plates in incubator shelves.
Evaluation of VC-Mediated Inhibition Between F. oxysporum and Trichoderma
Sandwiched Petri plates, a setup described in Dennis and Webster (1971), was employed to determine if and how Trichoderma VCs affect the growth of F. oxysporum. After inoculating F. oxysporum and Trichoderma on PDA plate, F. oxysporum plate was placed on top of Trichoderma plate, sealed with three layers of Parafilm, and incubated at 25°C (Figure 1A). Each plate of F. oxysporum also was sandwiched with an un-inoculated PDA plate (control treatment). Colony diameter of F. oxysporum was measured 5 days later. We evaluated the inhibitory effect of F. oxysporum VCs on Trichoderma in the same way except that 5-day-old (after the inoculation of culture plug) F. oxysporum culture was used to ensure enough F. oxysporum biomass. Colony diameter of Trichoderma was measured 36 h later. The duration of VC exposure between the two experiments was different because Trichoderma grew much faster than F. oxysporum. Each treatment included three biological replicates and was repeated three times.
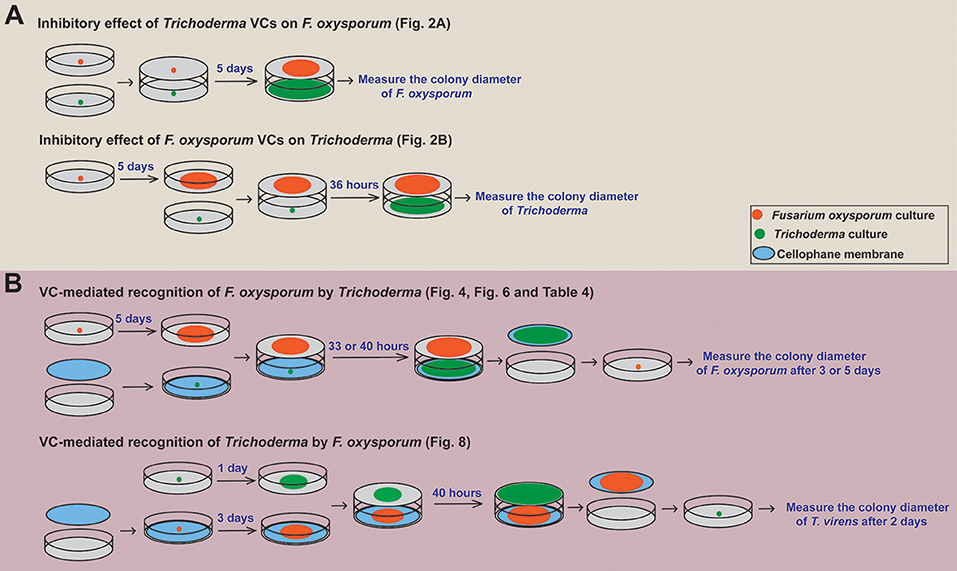
Figure 1. Overview of the experimental setups employed. A schematic representation of how we determined (A) the inhibitory effect of Trichoderma VCs on the growth of F. oxysporum and vice versa and (B) if Trichoderma and F. oxysporum sense VCs from the other side to prepare for subsequent attack is shown. The duration of VC exposure varied significantly among the experiments because Trichoderma grew much faster than F. oxysporum. Specific figures and table generated using each method are noted.
VC-Mediated Recognition of F. oxysporum by Trichoderma
To determine whether Trichoderma recognizes F. oxysporum VCs and prepares for attack, we compared the activity of antifungal molecules secreted by Trichoderma in the presence and absence of F. oxysporum VCs. This experiment was performed as outlined in Figure 1B. After inoculating a plug of Trichoderma culture on sterilized cellophane membrane (Paper Mart, Orange, CA, United States) overlaid on PDA, each plate was sandwiched with a plate of 5-day-old F. oxysporum culture or a un-inoculated PDA plate (Control). After 33 h (for T. harzianum and T. asperellum) or 40 h (for T. virens and T. viride) of co-cultivation, cellophane membrane along with Trichoderma culture was removed. The harvested culture was immediately frozen in liquid nitrogen and stored at −80°C until RNA extraction. The antifungal activity of molecules secreted into the medium by Trichoderma after each treatment was measured by inoculating a plug of F. oxysporum culture on the medium. Colony diameter of F. oxysporum was measured after 3 days for T. harzianum and T. asperellum and 5 days for T. virens and T. viride due to stronger inhibition by the latter two. Relative colony diameter after each VC treatment (%) was calculated using (Dtreatment/Dcontrol) × 100, where Dcontrol and Dtreatment indicated the colony diameters of F. oxysporum in the control and VC treatments, respectively.
Whether F. oxysporum VCs affect the amount/activity of antifungal VCs released by Trichoderma was determined as follows. After co-cultivation as described above, each Trichoderma plate was sandwiched with a new plate inoculated with a plug of F. oxysporum culture. The colony diameter of F. oxysporum was measured 3 days later. Each treatment consisted of three biological replicates and was repeated three times.
VC-Mediated Recognition of Trichoderma by F. oxysporum
The ability of F. oxysporum to sense Trichoderma VCs was evaluated similarly (Figure 1B). Each plate of 3-day-old F. oxysporum culture on sterilized cellophane membrane was sandwiched with a 1-day-old Trichoderma culture or control (un-inoculated PDA) plate. After 40 h of co-cultivation, F. oxysporum culture was removed, and a plug of T. virens culture was inoculated. Colony diameter was measured 2 days later. Each treatment was performed in three biological replicates and repeated three times.
Determination of the Protein Permeability of Cellophane Membrane
First, we placed four drops of 5 μL bovine serum albumin (Amresco, Solon, OH) solution at the concentrations of 0.01, 0.1, and 1 mg/ml on cellophane membrane overlaid on PDA and incubated the plate overnight. Drops of the same solutions were applied to PDA without cellophane membrane. These plates were stained using 20 mL Coomassie blue solution (Bio-Rad Laboratories, Richmond, CA, United States) for 1 h. Second, PDA plate containing a plug of Trichoderma culture inoculated on cellophane membrane was incubated for 40 h. After removing the cellophane membrane, the plate was stained to detect the presence of secreted proteins. We also compared the activity of antifungal molecules permeated through the cellophane and dialysis (Spectra/Por® 3 with the molecular weight cut off of 3.5 kD; Spectrum Laboratories, Rancho Dominguez, CA, United States) membranes. A plug of Trichoderma culture was inoculated on each membrane on PDA. After 40 h of incubation, the membrane with Trichoderma culture was removed, and a plug of F. oxysporum culture was inoculated on the same medium to measure the degree of growth inhibition. This comparison was repeated twice with three biological replicates.
Analysis of VCs Produced by F. oxysporum and Trichoderma
A culture dish of 10 cm in diameter and 4 cm in height (SPL Life Sciences, South Korea) that contains 250 mL PDA was used to extract F. oxysporum VCs (Supplementary Figure 1). A hole (5 mm in diameter) on its lid, created using a flamed cork borer, was sealed using a piece of tape. A PDA block beneath the hole was removed before inoculating F. oxysporum. We cultured F. oxysporum for 14 days before extracting headspace VCs. Trichoderma was cultured on PDA slant in a 15 ml glass vial for 3 days. Extraction of headspace VCs (two biological replicates) was performed as previously described (Li et al., 2018). A solid phase micro-extraction (SPME) fiber with 50/30 μm divinylbenzene/carboxen/polydimethylsiloxane coating was conditioned at 230°C for 1 h before VC extraction to remove any VCs from the fiber. Headspace VCs released from PDA without fungal culture were analyzed to identify VCs derived from the medium. VCs in the headspace were adsorbed for 1 h. The SPME fiber was desorbed at 230°C for 10 min in the split/splitless injector port (set at the splitless mode) of QP 2010 Ultra GC-MS system (Shimadzu, Japan) equipped with an Rtx-Wax capillary column (60 m × 0.25 mm × 0.25 μm, RESTEK, Bellefonte, PA). The temperature program was 35°C for 5 min, ramped to 230°C at 5°C/min (F. oxysporum) or 2°C/min (Trichoderma spp., for better separation of compounds), and kept for 15 min at 230°C. We applied helium (carrier gas) at the constant flow rate of 1 mL/min. The injector, transfer line, and ion source temperatures were set at 230°C. The electron ionization source was set at 70 eV. Data acquisition started at 13 min, and the mass scan range was 35–500 m/z. An alkane standard mixture (1 μL; C7-C40 containing 100 mg/L of each alkane in n-hexane) was analyzed to calculate the retention indices of individual VCs detected. We tentatively identified individual VCs by comparing their mass spectral profiles and retention indices with those archived in the NIST 11 library using the version 2.7 of the AMDIS (automated mass spectral deconvolution and identification system) software. We used the following criteria for compound identification: a match factor of ≥90% between sample spectra and reference spectra and ≤ ± 2% deviation of experimentally determined retention index values from those in the literature. The identity of the following compounds, which were produced by all F. oxysporum strains analyzed, was confirmed using commercially available standards: 1-hexanol, 3-methyl-1-butanol, isopentyl acetate, and 2-phenylethyl alcohol (Sigma-Aldrich) and 4-ethylanisole (Alfa Aesar, Haverhill, MA, United States). We used 20 μL of each compound diluted using hexane for GC-MS analysis.
RNA Extraction and Quantitative Real-Time PCR (qPCR) Analysis
To determine whether F. oxysporum VCs affect the expression of biocontrol-associated genes in Trichoderma, we extracted total RNAs from Trichoderma cultures harvested after treating with F. oxysporum VCs. The experiment was performed once with three biological replicates. Each biological replicate consisted of two Trichoderma cultures independently treated with F. oxysporum VCs or PDA. After treating extracted RNAs with DNase I (Ambion, Foster City, CA), they were purified using Qiagen RNeasy kit (Qiagen, Valencia, CA). Concentration and quality of purified RNAs were assessed using the A260/A280 and A260/230 absorbance ratios and gel electrophoresis. RNAs (1 μg) were reverse transcribed to cDNAs in a 20 μL reaction (Applied Biosystems, Foster City, CA, United States). Sequences of the 13 genes used for gene expression analysis (Table 2) were mined from genome sequences of T. virens Gv29-8 (V2.0) and T. harzianum CBS 226.95 (V1.0) available at http://genome.jgi.doe.gov/. The primers used (Supplementary Table 1) were designed using Primer 3 (http://primer3.ut.ee/). Primer specificity was tested using genomic DNA as a template. Each PCR reaction with three technical replicates was performed using Power SYBR® Green kit (Applied Biosystems) and consisted of 12.5 μL PCR master mix, 1 μL each of forward and reverse primers (10 μM), 2 μL diluted cDNAs (1:5 dilution), and 8.5 μL UltraPure™ DNase/RNase-free distilled water. qPCR conditions were: (a) one cycle of 10 min at 95°C, (b) 40 cycles of 30 s at 95°C, 30 s at 60°C, and 45 s at 72°C, (c) one cycle of 1 min at 95°C and 30 s at 55°C, and (d) final ramp to 95°C to check primer dimerization and nonspecific amplification. We used sterile water as no DNA control and RNAs after DNase treatment to confirm the absence of genomic DNA. Genes encoding elongation factor 1-α and β-actin were used to normalize gene expression (Seidl et al., 2009; Liu et al., 2010). Relative expression levels of individual genes were calculated from the threshold cycle using the Pfaffl method for efficiency correction (Pfaffl, 2001). Amplification efficiency was calculated as previously described (Rasmussen, 2001) using serially diluted cDNA samples (1, 1/5, 1/25, 1/125, and 1/625), which showed values between 90% and 110%.
Statistical Analysis
One-way analysis of variation (ANOVA) was performed using Minitab 18 (Minitab Inc., State College, PA, United States). The significance of each treatment was determined using the F-value. When a significant F-test was observed, separation of the means was carried out using Fisher's test. Statistical significance was determined at P = 0.05.
Results
Sandwiched Culture Plates Were Used to Study How Trichoderma BCAs and F. oxysporum Interact Via VCs
To prevent physical contact and exposure to secreted molecules diffused through medium while studying how VCs from each side affect the other, we initially evaluated I plate. This plate contains two compartments separated by a central divider and has been used for studying how fungal VCs affect plants (Bitas et al., 2015; Li and Kang, 2018; Li et al., 2018). However, because Trichoderma jumped over the divider even after we removed a strip of medium along the divider in both compartments, we used a method reported by Dennis and Webster (1971). We did not detect any incidence of F. oxysporum spores dropping to the bottom plate during co-cultivation, which was checked multiple times by placing a PDA plate at the bottom.
We investigated the following questions via the experiments outlined in Figure 1: (a) do VCs released by Trichoderma inhibit F. oxysporum growth and vice versa?; (b) does Trichoderma recognize the presence of F. oxysporum by sensing volatile cues from F. oxysporum and vice versa?; and (c) does VC-mediated recognition cause molecular changes potentially associated with biocontrol? All Trichoderma strains analyzed were isolated from commercial biocontrol products to facilitate future studies on the role of VC-mediated interactions in biocontrol. Thirteen F. oxysporum strains (Table 1), corresponding to seven formae speciales, were used.
Both Trichoderma and F. oxysporum Produced Antifungal VCs
Trichoderma BCAs secrete metabolites inhibitory to diverse pathogens (Reino et al., 2007; Vinale et al., 2014; Zeilinger et al., 2016), and some such metabolites are volatile (Amin et al., 2010; Meena et al., 2017). Here, we tested if VCs produced by four Trichoderma spp. inhibit F. oxysporum and vice versa (Figure 2). VCs produced by all Trichoderma spp. significantly inhibited the growth of three F. oxysporum strains (Figure 2A). T. viride VCs inhibited NRRL26379 (f. sp. radicis-lycopersici) most, whereas T. harzianum VCs inhibited NRRL38499 (f. sp. lycopersici) and NRRL54003 (f. sp. lycopersici) more than NRRL26379. To identify candidate VCs inhibitory to F. oxysporum, we analyzed VCs produced by 3-day-old T. harzianum and T. viride cultures. Most of the identified compounds, corresponding to alcohols, acids, esters, ketones, and sesquiterpenes, are species-specific (Figure 3 and Table 3). While T. harzianum emitted several alcohols, including 2-methyl-1-propanol, 3-methyl-1-butanol, 1-pentanol, 1-hexanol, 1-heptanol, 1-octanol, and 2-phenylethyl alcohol, T. virens produced only 3-methylcyclopentanol. Only compounds that were produced by both species are 1-octen-3-ol, 3-octanone, and acetic acid. It was evident that T. virens produces more sesquiterpenes in both the number and amount than T. harzianum.
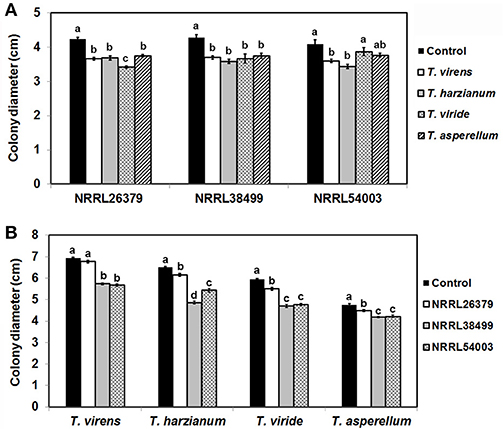
Figure 2. Inhibitory effect of volatile compounds (VCs) produced by Trichoderma and Fusarium oxysporum. After sandwiching culture plates of Trichoderma and F. oxysporum as described in Figure 1A, colony diameters of (A) three F. oxysporum strains after co-cultivation with four Trichoderma spp. and (B) four Trichoderma spp. after co-cultivation with three F. oxysporum strains were measured. Control treatments for (A,B) consisted of F. oxysporum and Trichoderma culture plates, respectively sandwiched with un-inoculated PDA plates. Values shown correspond to the mean ± standard deviation (SD) of data from three replicates. Different letters indicate significant differences based on Fisher's test at P = 0.05.
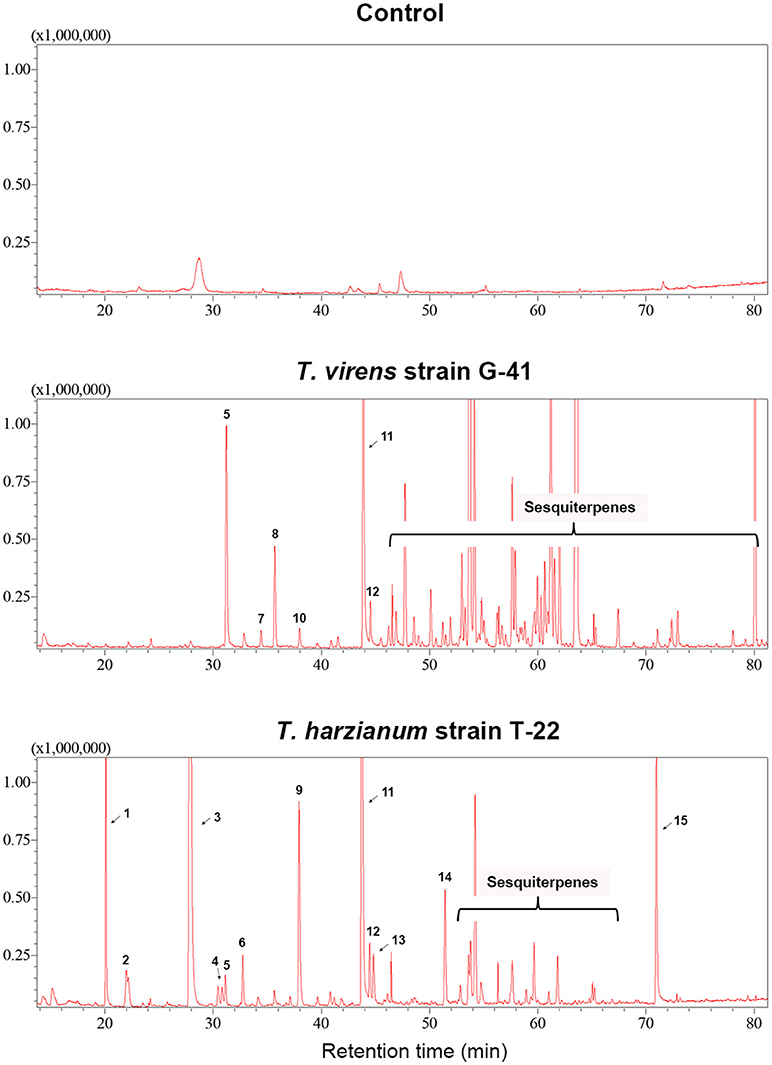
Figure 3. VCs produced by T. virens and T. harzianum. Headspace volatiles extracted from Control (PDA only) and cultures of T. virens strain G-41 and T. harzianum strain T-22 on PDA were separated using GC. The identity of compound corresponding to each numbered peak is shown in Table 3. X-axis, retention time (in minutes); Y-axis, intensity (counts per second).
We tested whether F. oxysporum also releases VCs inhibitory to Trichoderma (Figure 2B). Because F. oxysporum grows much more slowly than Trichoderma, we initiated F. oxysporum culture 5 days before co-cultivation to ensure that F. oxysporum strains produce sufficient amounts of VCs. VCs from all three F. oxysporum strains inhibited T. harzianum, T. viride, and T. asperellum with VCs from NRRL38499 and NRRL54003 causing stronger inhibition than VCs from NRRL26379. VCs from NRRL38499, and NRRL54003 but not NRRL26379 significantly inhibited T. virens.
F. oxysporum VCs Increased the Amount/Activity of Antifungal Molecules Secreted by T. virens
To test the hypothesis that Trichoderma recognizes F. oxysporum by sensing VCs from F. oxysporum and prepares for attack, we determined if F. oxysporum VCs induce the amount/activity of antifungal molecules secreted by T. virens. First, T. virens was cultured on sterilized cellophane membrane overlaid on PDA to prevent its hyphae from reaching medium but allow the passage of secreted molecules to the medium during co-cultivation with F. oxysporum. After removing the membrane along with T. virens culture, F. oxysporum strain NRRL54003 was inoculated on the same medium to measure its growth. Exposure to VCs from NRRL26379, NRRL38499, and NRRL54003 caused significantly higher growth inhibition than control treatments, but T. virens responded more strongly to VCs from NRRL26379 and NRRL54003 than VCs from NRRL38499 (Figure 4A). The growth of two other F. oxysporum strains was also measured to determine whether the increased antifungal activity caused by VCs from individual strains similarly affects them (Figures 4B,C). Their growth was also significantly inhibited, but the degree of inhibition looked different from that observed with NRRL54003 as a tester. The degree of inhibition after exposure to NRRL26379 VCs was significantly lower than that caused by VCs from NRRL38499 and NRRL54003 (Figure 4). We also evaluated whether F. oxysporum VCs increase the production of antifungal VCs by T. virens. Results from the VC and control treatments were indistinguishable(Supplementary Figure 2).
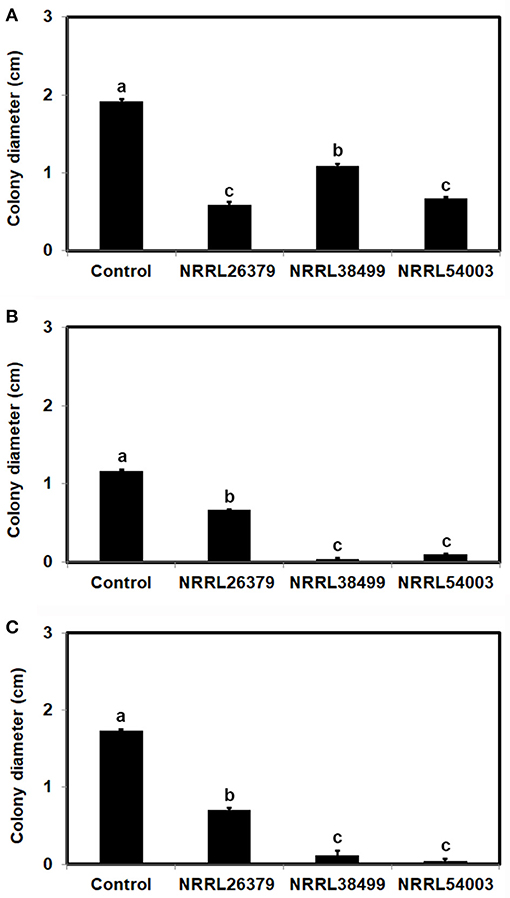
Figure 4. Increased amount/activity of antifungal molecules secreted by T. virens in response to F. oxysporum VCs. T. virens strain G-41 inoculated on cellophane membrane overlaid on PDA was co-cultivated with three F. oxysporum strains as well as un-inoculated PDA (Control) for 40 h. After removing the cellophane membrane with T. virens culture, a plug of (A) NRRL54003, (B) NRRL26379, or (C) NRRL38499 culture was inoculated. Colony diameters after 5 days of incubation are shown. Values shown correspond to the mean ± SD of data from three replicates. Different letters indicate significant differences based on Fisher's test at P = 0.05.
F. oxysporum VCs Induced the Amount of Antifungal Metabolites Secreted by Trichoderma
To determine whether the increased antifungal activity of T. virens secretome caused by F. oxysporum VCs was due to secreted metabolites, proteins or a combination of both, we evaluated the permeability of proteins through the cellophane membrane used in our work (Supplementary Figure 3). Although the two experiments shown in Supplementary Figure 3A could not directly answer this question, they suggested that proteins could not readily pass through the membrane. Besides, when we cultured T. virens on dialysis membrane (with the molecular weight cut off of 3.5 kD), the degree of inhibition was not significantly different from that based on cellophane membrane (Supplementary Figure 3B), suggesting that the increased antifungal activity after the exposure to F. oxysporum VCs (Figure 4) was mainly due to metabolites secreted into the medium.
T. virens and T. viride but not T. asperellum Responded to VCs From Diverse F. oxysporum Strains
We employed 10 additional strains corresponding to five formae speciales (Table 1) to determine T. virens' response. VCs produced by all of them significantly induced the amount of antifungal metabolites secreted by T. virens (Table 4). VCs produced by NRRL38487 (f.sp. conglutinans), NRRL37616 (f.sp. pisi) and NRRL26029 (f.sp. cubense) caused the highest degree of induction, completely blocking F. oxysporum growth. Similarly, VCs from all 13 strains significantly induced the amount of antifungal metabolites secreted by T. viride with VCs from five strains (9605, W6-1, NRRL38272, NRRL37611, and NRRL36118) causing complete growth inhibition (Table 4). However, none of them induced the amount of antifungal metabolites secreted by T. asperellum (Table 4).
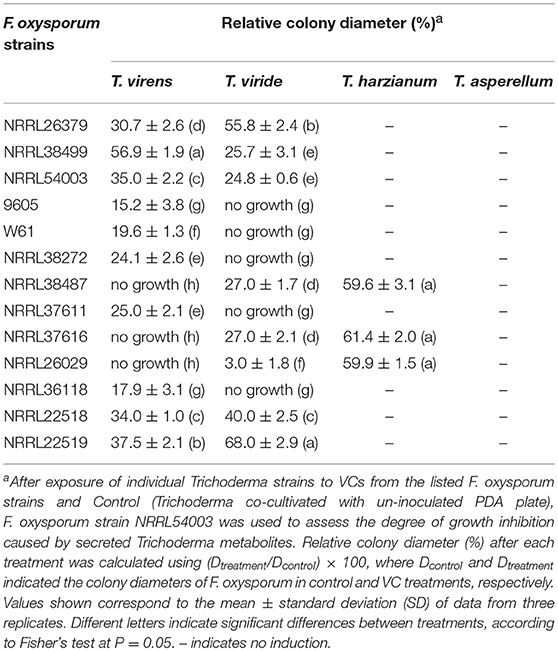
Table 4. Effect of VCs produced by diverse F. oxysporum strains on the secretion of antifungal metabolites by four Trichoderma spp.
T. harzianum Responded to VCs From Only Some F. oxysporum Strains
VCs from only NRRL38487, NRRL37616, and NRRL26029, which caused the highest induction in T. virens, significantly induced the amount of antifungal metabolites secreted by T. harzianum strain T-22 (Table 4). The degree of induction in T. harzianum was significantly lower than those observed in T. virens and T. viride. During this experiment, we observed that the amount of yellow pigment secreted by T. harzianum was also affected by F. oxysporum VCs (Figure 5). VCs from NRRL38272 and NRRL26029 appeared to suppress its production, while VCs from NRRL37616, NRRL22519, NRRL37611, and NRRL36118 increased its production. However, the amount secreted did not correlate with the degree of F. oxysporum inhibition. We determined if a different T. harzianum strain responds to VCs from some of the F. oxysporum strains that did not induce the secretion of antifungal metabolites by T. harzianum strain T-22. This T. harzianum strain responded to VCs from NRRL38499 and NRRL54003 but not NRRL26379 (Figure 6).
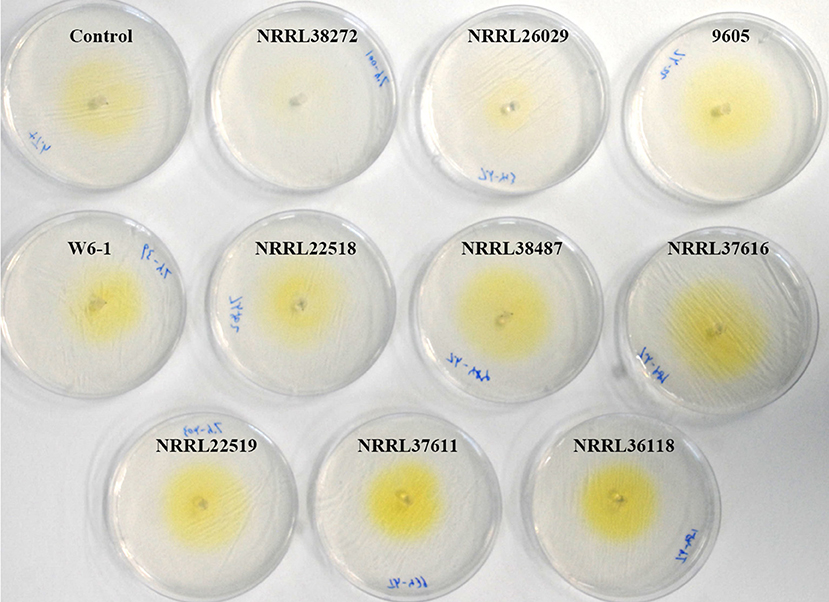
Figure 5. Effect of F. oxysporum VCs on the secretion of yellow pigment by T. harzianum. T. harzianum strain T-22 cultured on cellophane membrane overlaid on PDA was co-cultivated with 10 F. oxysporum strains and un-inoculated PDA (Control) for 33 h. After removing the cellophane membrane holding T-22 culture, plates were photographed (arranged based on the amount of pigment released).
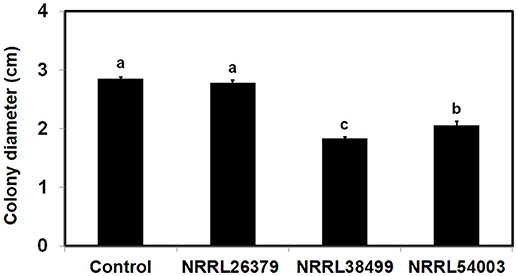
Figure 6. Effect of F. oxysporum VCs on the amount/activity of antifungal metabolites secreted by T. harzianum. T. harzianum strain isolated from Custom GP was exposed to VCs from three F. oxysporum strains and un-inoculated PDA (Control) for 33 h. After removing the cellophane membrane along with T. harzianum culture, one plug of NRRL54003 culture was inoculated on each plate. Colony diameters after 3 days of incubation are shown. Values shown correspond to the mean ± SD of data from three replicates. Different letters indicate significant differences based on Fisher's test at P = 0.05.
Expression of Some Biocontrol-Associated Genes in Trichoderma Was Affected by F. oxysporum VCs
To investigate if F. oxysporum VCs affect the expression of biocontrol-associated genes, we analyzed transcripts from 11 genes in T. virens and T. harzianum after co-cultivation with NRRL26379, NRRL38499, NRRL38487, and NRRL37616. VCs from NRRL38487 and NRRL37616 induced the secretion of antifungal metabolites much more strongly than VCs from NRRL38499 and NRRL26379 in both T. virens and T. harzianum (Table 4). Ten genes (Table 2) were chosen because their expression increased upon pathogen confrontation (Samolski et al., 2009; Seidl et al., 2009; Vieira et al., 2013; Yao et al., 2016). Four genes, including endo (endochitinase 42), qid (QID74), acs (acyl-CoA synthetase), and ans (anthranilate synthase component II), showed increased transcription even before physical contact (Seidl et al., 2009; Vieira et al., 2013), suggesting that airborne signals or small molecules diffused through medium might induce their expression. The terpene synthase (tps) gene was hypothesized to be involved in biocontrol (Kubicek et al., 2011). VCs from some strains up-regulated five T. virens genes (Figure 7A). Expression of chit (chitinase 33) was significantly higher after exposure to VCs from NRRL26379 and NRRL38499, but it was down-regulated by VCs from NRRL38487 and NRRL37616. Whereas VCs from NRRL38487 and NRRL37616 induced the expression of prb (subtilisin-like protease) and hsp (heat-shock protein), VCs from NRRL26379 and NRRL38499 slightly suppressed their expression. VCs from NRRL38499 and NRRL38487 significantly increased the level of qid transcripts. VCs from NRRL38499 significantly induced the expression of tps, where VCs from NRRL37616 suppressed its expression. VCs from NRRL37616 and NRRL38487 suppressed the expression of endo) and bgn (β-1, 3-endoglucanase), respectively. Expression of four genes (pra, acs, ans, and pks) did not appear significantly affected by any treatments. In T. harzianum (Figure 7B), only acs was induced by VCs from NRRL38499. Expression of prb was down-regulated by VCs from all four strains. VCs from NRRL38499, NRRL38487, and NRRL37616 appeared to suppress the expression of chit, endo, bgn, and tps. VCs from NRRL37616 suppressed the expression of qid and pks.
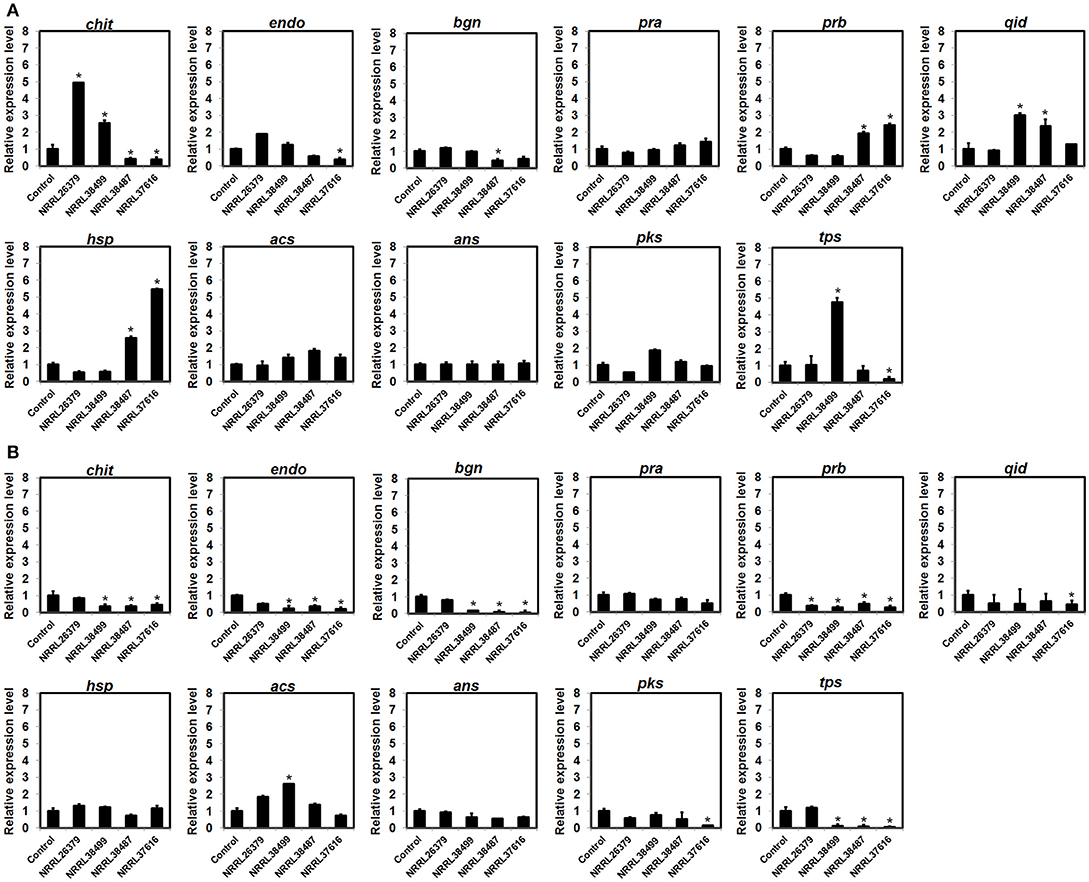
Figure 7. Expression patterns of biocontrol-associated genes after exposure to F. oxysporum VCs. Transcripts from 11 genes in (A) T. virens strain G-41 and (B) T. harzianum strain T-22 were quantified after co-cultivation with four F. oxysporum strains (NRRL26379, NRRL38499, NRRL38487, and NRRL37616) for 40 h and 33 h, respectively. Transcripts from the elongation factor 1-α and β-actin genes were used as internal references to normalize the level of transcripts for individual genes. Control corresponded to Trichoderma co-cultivated with un-inoculated PDA. Values shown correspond to the relative expression ± SD of data from three replicates. Gene identity based on the abbreviation scheme shown in Table 2 is indicated. * indicates up-regulation (relative expression level >2) or down-regulation (relative expression level < 0.5).
Seven F. oxysporum Strains Commonly Produced Six VCs
Increased secretion of antifungal metabolites by T. virens and T. viride in response to VCs from diverse F. oxysporum strains (Table 4) suggested that commonly produced compound(s) might be responsible. To identify such compounds, we analyzed VCs produced by seven strains corresponding to five formae speciales (Table 5). Initially, they were cultured in 15 mL glass vials to extract VCs. However, many compounds were unidentifiable because the amount of VCs captured was too small. We increased the concentration of headspace VCs by expanding the culture area while reducing the headspace volume (Supplementary Figure 1). Collectively, 28 VCs were identified (Table 5). Six compounds, including 3-methyl-1-butanol, 1-hexanol, 2-phenylethyl alcohol, isopentyl acetate, 4-ethylanisole, and α-cedrene, were produced by all strains. Two compounds, including β-caryophyllene and D-germacrene, were emitted by only one strain (NRRL38487 and NRRL54003, respectively). Two or more strains produced the remaining compounds.
F. oxysporum also Responded to trichoderma VCs by Increasing the Secretion of Its Antifungal Metabolites
To investigate whether the ability to respond to VCs from other fungi is unique to some Trichoderma spp., we evaluated the response of F. oxysporum strains NRRL38499 and NRRL54003 to VCs produced by four Trichoderma spp. (Figure 8). After exposing individual F. oxysporum strains cultured on sterilized cellophane membrane overlaid on PDA to Trichoderma VCs, we used T. virens to measure how strongly metabolites secreted by F. oxysporum inhibit its growth. VCs from all four Trichoderma spp. increased the amount of antifungal metabolites secreted by F. oxysporum. VCs from T. viride induced most in NRRL38499 (Figure 8A). However, for NRRL54003, VCs from T. virens, T. harzianum, and T. viride induced similarly, and the degree of induction by VCs from T. asperellum was significantly lower than the others (Figure 8B).
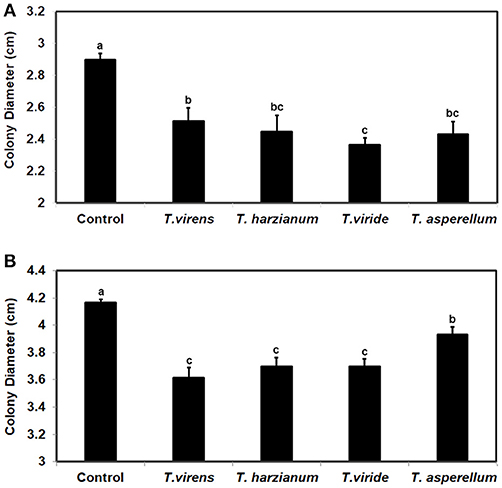
Figure 8. Increased secretion of antifungal metabolites by F. oxysporum in response to Trichoderma VCs. F. oxysporum strains (A) NRRL38499 and (B) NRRL54003 cultured on cellophane membrane overlaid on PDA were co-cultivated with four Trichoderma spp. and un-inoculated PDA (Control) for 40 h. After removing the cellophane membrane with F. oxysporum culture, one culture plug of T. virens strain G-41 was inoculated on each plate. Colony diameters after 2 days of incubation are shown. Values shown correspond to the mean ± SD of data from three replicates. Different letters indicate significant differences based on Fisher's test at P = 0.05.
Discussion
Microbes have evolved multiple mechanisms to interact with other organisms. The primary mechanism is the secretion of a wide variety of molecules (Braga et al., 2016; Vincent et al., 2016). Compared to rapid advances in research on how secreted proteins contribute to pathogenesis and symbiosis, research on secreted metabolites, especially those that are volatile, lags considerably behind in part due to an intrinsic bias caused by experimental design. Because studies on microbial interaction with other organisms typically rely on applying microbial cells, physical contact or waterborne molecules are often assumed to cause most responses. Using the assays described in Figure 1, we confirmed the role of VCs as a chemical weapon in Trichoderma-F. oxysporum interactions and also found that both fungi seem to recognize some VCs as an alarm for looming battle. We discuss how both types of VCs potentially contribute to biocontrol and fungal ecology below.
Fungal VCs as a Chemical Weapon
Analysis of VCs produced by T. virens and T. harzianum (Table 3) revealed a few candidate compounds. Both species produced 3-octanone and 1-octen-3-ol, which are fungistatic and fungicidal (Wheatley et al., 1997; Okull et al., 2003). Two compounds produced by T. harzianum, 2-phenylethyl alcohol and isopentyl acetate, also exhibited antimicrobial activities (Ando et al., 2015; Medina-Romero et al., 2017). However, T. virens did not produce them. Compared to T. harzianum, T. virens produced more volatile sesquiterpenes (Figure 2). Lee et al. (2016) also reported that T. virens produced more sesquiterpenes than T. harzianum. It remains to be determined if any of these sesquiterpenes inhibit F. oxysporum, but some volatile sesquiterpenes have been shown to function as antimicrobials or plant growth regulators (Stoppacher et al., 2010; Yamagiwa et al., 2011; Ditengou et al., 2015; Kottb et al., 2015). Previous studies showed the production of 6-pentyl-2H-pyran-2-one, a secondary metabolite inhibitory to several plant pathogenic fungi, by T. virens, T. viride, T. harzianum, T. atroviride, and T. koningii (Scarselletti and Faull, 1994; Worasatit et al., 1994). However, we did not detect this compound in VCs produced by T. harzianum and T. virens, perhaps because its production may be strain specific or affected by the medium used, pH, light, water content, the age of culture, or combinations of these environmental factors (Lee et al., 2015).
The ability to use VCs as a weapon is not unique to Trichoderma as shown in Figure 1. Some antifungal compounds produced by Trichoderma spp., such as 2-phenylethyl alcohol and isopentyl acetate, were also produced by seven F. oxysporum strains (Table 5). Although none of the F. oxysporum strains appeared to produce 3-octanone and 1-octen-3-ol, we detected their production by NRRL38499 and NRRL26379 in our earlier study (Bitas et al., 2015), again suggesting the significance of environmental factors in determining which VCs are produced. Employment of VCs as a chemical weapon from both sides of interaction is not too surprising because diverse bacteria and fungi have been shown to produce antimicrobial VCs (Bitas et al., 2013; Bennett and Inamdar, 2015; Tyc et al., 2017). For example, VCs produced by the endophytic fungus Muscodor crispans strongly inhibited Pythium ultimum, Phytophthora cinnamomi, Sclerotinia sclerotiorum, and Xanthomonas axonopodis (Mitchell et al., 2010). A critical question is whether Trichoderma VCs affect the structure and activity of neighboring microbial communities in ways that impact the outcome of biocontrol. For example, suppression of beneficial plant-associated microbes may weaken the plant's ability to manage other stresses, causing reduced plant fitness. A related question is whether pathogens like F. oxysporum utilize antimicrobial VCs to manipulate plant-associated microbial communities to ensure its rhizosphere competency and pathogenicity. Identification of antimicrobial VCs and the genes involved in their synthesis will help answer these questions by allowing genetic manipulations of the synthesis of specific candidate VCs.
Is VC-Mediated Recognition of F. oxysporum by Trichoderma a Novel Mechanism Critical for Biocontrol?
Trichoderma constitutively secretes a battery of enzymes, including proteases and cell wall degrading enzymes, at low levels (Kredics et al., 2003; Druzhinina et al., 2011; Vos et al., 2015). It has been hypothesized that specific peptides and cell wall components, released by the action of these enzymes, presumably function as signals for inducing the production of more enzymes and other weapons needed for mycoparasitism and directing growth (Woo et al., 2006; Seidl et al., 2009; Omann and Zeilinger, 2010; Druzhinina et al., 2011; Mukherjee et al., 2012). Consistent with this hypothesis, expression of T. harzianum genes encoding endochitinase CHIT42 and proteases PRA1 and PRB1 was highly induced in the presence of chitin (Samolski et al., 2009). Increased secretion of antifungal metabolites by T. virens and T. viride in response to F. oxysporum VCs (Table 4) suggests that both Trichoderma spp. sense F. oxysporum VC(s) to initiate preparation for pending attack. However, considering that the degree of VC-mediated growth inhibition by Trichoderma did not significantly increase after exposure to F. oxysporum VCs (Supplementary Figure 2), the induced antifungal metabolite(s) may not be volatile. Because the cellophane membrane used did not appear permeable to secreted proteins (Supplementary Figure 3), it remains to be determined whether F. oxysporum VCs also induce the secretion of antifungal proteins. The gene expression pattern in response to F. oxysporum VCs (Figure 7) suggests that the production of some such proteins might be affected.
In contrast to T. virens and T. viride, VCs from all F. oxysporum strains failed to induce the amount of antifungal metabolites secreted by T. asperellum (Table 4), and two T. harzianum strains responded to VCs from only some strains (Table 4 and Figure 6). However, the amount of unknown yellow metabolite secreted by T. harzianum (Figure 5) in response to F. oxysporum VCs suggested that VCs from some strains that did not induce the amount of antifungal metabolites but still affected T. harzianum. For example, VCs of NRRL38272 suppressed the amount of yellow metabolite secreted, while VCs of NRRL36118, NRRL37611, and NRRL22519 increased its secretion. VCs of NRRL26029, a strain that induced the amount of antifungal metabolites secreted by T. harzianum (Table 4), suppressed its secretion. Although we did not identify this metabolite, it probably is one of the chromogenic secondary metabolites called anthraquinones. Several Trichoderma spp., including T. harzianum, produce anthraquinones such as pachybasin, emodin, and ω- hydroxypachybasin (Reino et al., 2007). Some anthraquinones displayed antimicrobial activities (Chukwujekwu et al., 2006; Wu et al., 2006; Liu et al., 2009). However, it is not clear if its production is part of the preparation for chemical warfare because the amount secreted did not correlate with the degree of antifungal activity of secreted metabolites in response to F. oxysporum VCs (Table 4).
Differential expression of several biocontrol-associated genes in response to F. oxysporum VCs (Figure 7) also supports the hypothesis that F. oxysporum VCs affect cellular processes other than the secretion of antifungal metabolites in Trichoderma. However, similar to the secreted yellow metabolite, gene expression pattern did not present a clear picture of how VCs of individual strains affected Trichoderma. Although VCs of NRRL38487 and NRRL37616 induced the amount/activity of antifungal metabolites secreted by T. virens and T. harzianum much more strongly than VCs of NRRL26379 and NRRL38499, VCs of the former two significantly induced the expression of only prb (subtilisin-like protease) and hsp (small heat-shock protein) in T. virens. As for chit (chitinase), VCs of the latter two significantly induced its expression. All three genes were reported to be up-regulated by contact with pathogens (Samolski et al., 2009; Seidl et al., 2009; Vieira et al., 2013). In T. harzianum, all three genes appeared to be suppressed or unaffected by F. oxysporum VCs. Expression of qid, a gene that encodes a cell wall protein presumably involved in protecting cell wall from degradation (Rosado et al., 2007; Vieira et al., 2013), was induced in T. virens by VCs of NRRL38499 (mild inducer of the amount of antifungal metabolites secreted) and NRRL38487 (strong inducer), but not by VCs of the other two. Only VCs from NRRL38499 significantly induced tps, which encodes a terpene synthase, in T. virens. Most of the T. harzianum genes were down-regulated or unaffected by F. oxysporum VCs (Figure 7).
We hypothesize that pathogen recognition by sensing pathogen-derived volatile cues helps biocontrol. Complex molecular responses of Trichoderma spp. to F. oxysporum VCs (Table 4 and Figures 4–7) indicated several experiments needed to test this hypothesis. Global gene expression analysis of more Trichoderma strains/species via RNAseq will help better understand how F. oxysporum VCs affect Trichoderma BCAs and if any resulting changes are related to biocontrol. Another important work is determining how other Trichoderma spp. respond to VCs from diverse pathogens. Considering the phylogenetic positions of the four species tested (Kubicek et al., 2003, 2011), this ability does not appear to follow their evolutionary relationships. Perhaps, the most critical question is which VC(s) function as signals and how Trichoderma recognizes and processes such signals (e.g., receptor-mediated or other ways?). Analysis of VCs produced by seven F. oxysporum strains (Table 5) revealed that although each strain produced a unique mix of VCs, all of them produced six compounds. One of the compounds, 2-phenylethyl alcohol, was reported to stimulate the developmental transition of Saccharomyces cerevisiae from unicellular to filamentous (Chen and Fink, 2006). Similarly, 3-octanone and 1-octen-3-ol also regulate fungal development and mediate inter-colony communication in Penicillium and Trichoderma (Chitarra et al., 2004; Nemcovic et al., 2008). Real-time analysis of VCs using GC-MS with improved sensitivity would provide more accurate information about the composition and amount of candidate VCs produced by F. oxysporum over time.
Additional Questions and Future Directions
Recognition of Trichoderma VCs by F. oxysporum and vice versa (Table 4 and Figure 8) suggests that this form of interaction may occur between other fungi. As noted in Introduction, such VC-mediated interactions seem to occur even between bacteria and fungi (Spraker et al., 2014; Rybakova et al., 2017), which underscores the need to survey many combinations of microbes to study how such manipulations influence the structure and activity of microbial communities.
The methods we employed (Figure 1) can support rapid screening of diverse combinations of microbes to study if they interact via VCs. However, there exist some limitations. Although cellophane membrane allowed us to quantify the amount of antifungal metabolites secreted and harvest mycelia easily for subsequent analysis, it is not suitable for evaluating fungal strains that secrete enzymes with high cellulolytic activity. We observed that hyphae of some strains reached medium by breaching the membrane. Analysis of secreted metabolites is needed to identify antifungal compound(s) and how VCs control their synthesis. However, their presence in solid medium makes this analysis challenging. As noted above, changes in the secretion of antifungal proteins in response to VCs could not be determined. Considering that VCs from both sides likely affect each other in multiple ways, an assay system that allows unidirectional airflow would be highly desirable to determine how VCs from one culture affect the other.
Author Contributions
NL, AA, and WW performed most of the experiments and wrote the manuscript. MI and KN performed some of the experiments. XL contributed to GC-MS analyses. SK coordinated the study, designed experiments, and revised the manuscript.
Funding
This work was supported in part by the USDA- Specialty Crop Multi-State Program (AM170200XXXXG006). Fellowships from the Storkan-Hanes-McCaslin Foundation and the Huck Institutes of the Life Sciences at Penn State supported NL. The Fulbright Scholar Program supported AA's visit to Penn State.
Conflict of Interest Statement
The authors declare that the research was conducted in the absence of any commercial or financial relationships that could be construed as a potential conflict of interest.
Acknowledgments
We would like to think Yongho Jeon for help with some experiments.
Supplementary Material
The Supplementary Material for this article can be found online at: https://www.frontiersin.org/articles/10.3389/fmicb.2018.02614/full#supplementary-material
References
Amin, F., Razdan, V. K., Mohiddin, F. A., Bhat, K. A., and Sheikh, P. A. (2010). Effect of volatile metabolites of Trichoderma species against seven fungal plant pathogens in-vitro. J. Phytol. 2, 34–37.
Ando, H., Kurata, A., and Kishimoto, N. (2015). Antimicrobial properties and mechanism of volatile isoamyl acetate, a main flavour component of Japanese sake (Ginjo-shu). J. Appl. Microbiol. 118, 873–880. doi: 10.1111/jam.12764
Arasimowicz-Jelonek, M., and Floryszak-Wieczorek, J. (2014). Nitric oxide: an effective weapon of the plant or the pathogen? Mol. Plant Pathol. 15, 406–416. doi: 10.1111/mpp.12095
Bailly, A., and Weisskopf, L. (2012). The modulating effect of bacterial volatiles on plant growth. Plant Signal. Behav. 7, 79–85. doi: 10.4161/psb.7.1.18418
Bennett, J., and Inamdar, A. (2015). Are some fungal volatile organic compounds (VOCs) mycotoxins? Toxins 7, 3785–3804. doi: 10.3390/toxins7093785
Bennett, J. W., Hung, R., Lee, S., and Padhi, S. (2012). “Fungal and bacterial volatile organic compounds: an overview and their role as ecological signaling agents,” in Fungal Associations eds K. Esser, P. A. Lemke, B. Hock, and J. W. Bennett (Berlin Heidelberg: Springer), 373–393.
Bitas, V., Kim, H. S., Bennett, J. W., and Kang, S. (2013). Sniffing on microbes: diverse roles of microbial volatile organic compounds in plant health. Mol. Plant. Microbe. Interact. 26, 835–843. doi: 10.1094/MPMI-10-12-0249-CR
Bitas, V., McCartney, N., Li, N., Demers, J., Kim, J. E., Kim, H. S., et al. (2015). Fusarium oxysporum volatiles enhance plant growth via affecting auxin transport and signaling. Front. Microbiol. 6:1248. doi: 10.3389/fmicb.2015.01248
Braga, R. M., Dourado, M. N., and Araújo, W. L. (2016). Microbial interactions: ecology in a molecular perspective. Braz. J. Microbiol. 47 (Suppl. 1), 86–98. doi: 10.1016/j.bjm.2016.10.005
Buck, L. B. (2004). Olfactory receptors and odor coding in mammals. Nutr. Rev. 62, S184–S188. doi: 10.1301/nr.2004.nov.S184
Cassago, A., Panepucci, R., Baião, A., and Henrique-Silva, F. (2002). Cellophane based mini-prep method for DNA extraction from the filamentous fungus Trichoderma reesei. BMC Microbiol. 2:14. doi: 10.1186/1471-2180-2-14
Chen, H., and Fink, G. R. (2006). Feedback control of morphogenesis in fungi by aromatic alcohols. Genes Dev. 20, 1150–1161. doi: 10.1101/gad.1411806
Chitarra, G. S., Abee, T., Rombouts, F. M., Posthumus, M. A., and Dijksterhuis, J. (2004). Germination of Penicillium paneum conidia is regulated by 1-octen-3-ol, a volatile self-inhibitor. Appl. Environ. Microbiol. 70, 2823–2829. doi: 10.1128/AEM.70.5.2823-2829.2004
Chukwujekwu, J. C., Coombes, P. H., Mulholland, D. A., and van Staden, J. (2006). Emodin, an antibacterial anthraquinone from the roots of Cassia occidentalis. S. Af. J. Bot. 72, 295–297. doi: 10.1016/j.sajb.2005.08.003
Dennis, C., and Webster, J. (1971). Antagonistic properties of species-groups of Trichoderma. Trans. Br. Mycol. Soc. 57, 41–48. doi: 10.1016/S0007-1536(71)80078-5
Ditengou, F. A., Müller, A., Rosenkranz, M., Felten, J., Lasok, H., van Doorn, M. M., et al. (2015). Volatile signalling by sesquiterpenes from ectomycorrhizal fungi reprogrammes root architecture. Nat. Commun. 6:6279. doi: 10.1038/ncomms7279
Druzhinina, I. S., Seidl-Seiboth, V., Herrera-Estrella, A., Horwitz, B., a, Kenerley, C. M., Monte, E., et al. (2011). Trichoderma: the genomics of opportunistic success. Nat. Rev. Microbiol. 9, 749–759. doi: 10.1038/nrmicro2637
Gordon, T. R., and Martyn, R. D. (1997). The evolutionary biology of Fusarium oxysporum. Annu. Rev. Phytopathol. 35, 111–128. doi: 10.1146/annurev.phyto.35.1.111
Harman, G. E. (2006). Overview of mechanisms and uses of Trichoderma spp. Phytopathology 96, 190–194. doi: 10.1094/PHYTO-96-0190
Harman, G. E., Howell, C. R., Viterbo, A., Chet, I., and Lorito, M. (2004). Trichoderma species—Opportunistic, avirulent plant symbionts. Nat. Rev. Microbiol. 2, 43–56. doi: 10.1038/nrmicro797
Herrmann, A. (2010). The Chemistry and Biology of Volatiles, ed A. Herrmann (Chichester: John Wiley & Sons Ltd).
Howell, C. R. (2003). Mechanisms employed by Trichoderma species in the biological control of plant diseases: the history and evolution of current concepts. Plant Dis. 87, 4–10. doi: 10.1094/PDIS.2003.87.1.4
Huang, M., Sanchez-Moreiras, A. M., Abel, C., Sohrabi, R., Lee, S., Gershenzon, J., et al. (2012). The major volatile organic compound emitted from Arabidopsis thaliana flowers, the sesquiterpene (E)-β-caryophyllene, is a defense against a bacterial pathogen. New Phytol. 193, 997–1008. doi: 10.1111/j.1469-8137.2011.04001.x
Kang, S., Demers, J., Jamenez-Gasco, M., Rep, M., Dean, R. A., Lichens-Park, A., et al. (2014). Genomics of Plant-Associated Fungi and Oomycetes: Dicot Pathogens, eds R. A. Dean, A. Lichens-Park, and C. Kole (Berlin, Heidelberg: Springer).
Kottb, M., Gigolashvili, T., Großkinsky, D. K., and Piechulla, B. (2015). Trichoderma volatiles effecting Arabidopsis: from inhibition to protection against phytopathogenic fungi. Front. Microbiol. 6, 1–14. doi: 10.3389/fmicb.2015.00995
Kredics, L., Antal, Z., Manczinger, L., Szekeres, A., Kevei, F., and Nagy, E. (2003). Influence of environmental parameters on Trichoderma strains with biocontrol potential. Food Technol. Biotechnol. 41, 37–42. Available online at: http://www.ftb.com.hr/images/pdfarticles/2003/January-March/41-37.pdf
Kubicek, C. P., Bissett, J., Druzhinina, I., Kullnig-Gradinger, C., and Szakacs, G. (2003). Genetic and metabolic diversity of Trichoderma: a case study on South-East Asian isolates. Fungal Genet. Biol. 38, 310–319. doi: 10.1016/S1087-1845(02)00583-2
Kubicek, C. P., Herrera-Estrella, A., Seidl-Seiboth, V., Martinez, D. A., Druzhinina, I. S., Thon, M., et al. (2011). Comparative genome sequence analysis underscores mycoparasitism as the ancestral life style of Trichoderma. Genome Biol. 12:R40. doi: 10.1186/gb-2011-12-4-r40
Lee, S., Hung, R., Yap, M., and Bennett, J. W. (2015). Age matters: the effects of volatile organic compounds emitted by Trichoderma atroviride on plant growth. Arch. Microbiol. 197, 723–727. doi: 10.1007/s00203-015-1104-5
Lee, S., Yap, M., Behringer, G., Hung, R., and Bennett, J. W. (2016). Volatile organic compounds emitted by Trichoderma species mediate plant growth. Fungal Biol. Biotechnol. 3:7. doi: 10.1186/s40694-016-0025-7
Li, N., Alfiky, A., Vaughan, M. M., and Kang, S. (2016). Stop and smell the fungi: fungal volatile metabolites are overlooked signals involved in fungal interaction with plants. Fungal Biol. Rev. 30, 134–144. doi: 10.1016/j.fbr.2016.06.004
Li, N., and Kang, S. (2018). Do volatile compounds produced by Fusarium oxysporum and Verticillium dahliae affect stress tolerance in plants? Mycology 9, 166–175. doi: 10.1080/21501203.2018.1448009
Li, N., Wang, W., Bitas, V., Subbarao, K., Liu, X., and Kang, S. (2018). Volatile compounds emitted by diverse Verticillium species enhance plant growth by manipulating auxin signaling. Mol. Plant-Microbe Interact. 31, 1021–1031. doi: 10.1094/MPMI-11-17-0263-R
Liu, S. Y., Lo, C. T., Shibu, M. A., Leu, Y. L., Jen, B. Y., and Peng, K. C. (2009). Study on the anthraquinones separated from the cultivation of Trichoderma harzianum strain Th-R16 and their biological activity. J. Agric. Food Chem. 57, 7288–7292. doi: 10.1021/jf901405c
Liu, Z., Yang, X., Sun, D., Song, J., Chen, G., Juba, O., et al. (2010). Expressed sequence tags-based identification of genes in a biocontrol strain Trichoderma asperellum. Mol. Biol. Rep. 37, 3673–3681. doi: 10.1007/s11033-010-0019-0
Mazzola, M., and Freilich, S. (2016). Prospects for biological soil-borne disease control: application of indigenous versus synthetic microbiomes. Phytopathology 107, 256–263. doi: 10.1094/PHYTO-09-16-0330-RVW
Medina-Romero, Y. M., Roque-Flores, G., and Macías-Rubalcava, M. L. (2017). Volatile organic compounds from endophytic fungi as innovative postharvest control of Fusarium oxysporum in cherry tomato fruits. Appl. Microbiol. Biotechnol. 101, 8209–8222. doi: 10.1007/s00253-017-8542-8
Meena, M., Swapnil, P., Zehra, A., Dubey, M. K., and Upadhyay, R. S. (2017). Antagonistic assessment of Trichoderma spp. by producing volatile and non-volatile compounds against different fungal pathogens. Arch. Phytopathol. Plant Prot. 50, 629–648. doi: 10.1080/03235408.2017.1357360
Mitchell, A. M., Strobel, G. A., Moore, E., Robison, R., and Sears, J. (2010). Volatile antimicrobials from Muscodor crispans, a novel endophytic fungus. Microbiology 156, 270–277. doi: 10.1099/mic.0.032540-0
Mukherjee, P. K., Horwitz, B. A., and Kenerley, C. M. (2012). Secondary metabolism in Trichoderma—A genomic perspective. Microbiology 158, 35–45. doi: 10.1099/mic.0.053629-0
Nelson, P. E. (1981). “Life cycle and epidemiology of Fusarium oxysporum,” in Fungal Wilt Diseases of Plants eds M. E. Mace, A. A. Bell, and C. H. Beckman (Amsterdam: Elsevier), 51–80.
Nemcovic, M., Jakubíkov,á, L., Víden, I., and Farkas, V. (2008). Induction of conidiation by endogenous volatile compounds in Trichoderma spp. FEMS Microbiol. Lett. 284, 231–236. doi: 10.1111/j.1574-6968.2008.01202.x
O'Donnell, K., Gueidan, C., Sink, S., Johnston, P. R., Crous, P. W., Glenn, A., et al. (2009). A two-locus DNA sequence database for typing plant and human pathogens within the Fusarium oxysporum species complex. Fungal Genet. Biol. 46, 936–948. doi: 10.1016/j.fgb.2009.08.006
Okull, D. O., Beelman, R. B., and Gourama, H. (2003). Antifungal activity of 10-oxo-trans-8-decenoic acid and 1-octen-3-ol against Penicillium expansum in potato dextrose agar medium. J. Food Prot. 66, 1503–1505. doi: 10.4315/0362-028X-66.8.1503
Omann, M., and Zeilinger, S. (2010). How a mycoparasite employs G-protein signaling: using the example of Trichoderma. J. Signal Transduct. 2010, 1–8. doi: 10.1155/2010/123126
Pfaffl, M. W. (2001). A new mathematical model for relative quantification in real-time RT-PCR. Nucleic Acids Res. 29:e45. doi: 10.1093/nar/29.9.e45
Rasmussen, R. (2001). Rapid Cycle Real-Time PCR, eds S. Meuer, C. Wittwer, and K. I. Nakagawara (Berlin, Heidelberg: Springer).
Reino, J. L., Guerrero, R. F., Hernández-Galán, R., and Collado, I. G. (2007). Secondary metabolites from species of the biocontrol agent Trichoderma. Phytochem. Rev. 7, 89–123. doi: 10.1007/s11101-006-9032-2
Rosado, I. V., Rey, M., Codón, A. C., Govantes, J., Moreno-Mateos, M., and a, Benítez, T. (2007). QID74 cell wall protein of Trichoderma harzianum is involved in cell protection and adherence to hydrophobic surfaces. Fungal Genet. Biol. 44, 950–964. doi: 10.1016/j.fgb.2007.01.001
Rybakova, D., Rack-Wetzlinger, U., Cernava, T., Schaefer, A., Schmuck, M., and Berg, G. (2017). Aerial warfare: a volatile dialogue between the plant pathogen Verticillium longisporum and its antagonist Paenibacillus polymyxa. Front. Plant Sci. 8:1294. doi: 10.3389/fpls.2017.01294
Samolski, I., de Luis, A., Vizcaíno, J., Monte, E., and Suárez, M. B. (2009). Gene expression analysis of the biocontrol fungus Trichoderma harzianum in the presence of tomato plants, chitin, or glucose using a high-density oligonucleotide microarray. BMC Microbiol. 9:217. doi: 10.1186/1471-2180-9-217
Sawant, I. (2014). Trichoderma—foliar pathogen interactions. Open Mycol. J. 8 (Suppl. 1), 58–70. doi: 10.2174/1874437001408010058
Scarselletti, R., and Faull, J. L. (1994). In vitro activity of 6-pentyl-a-pyrone, a metabolite of Trichoderma harzianum, in the inhibition of Rhizoctonia solani and Fusarium oxysporum f. sp. lycopersici. Mycol. Res. 98, 1207–1209.
Seidl, V., Song, L., Lindquist, E., Gruber, S., Koptchinskiy, A., Zeilinger, S., et al. (2009). Transcriptomic response of the mycoparasitic fungus Trichoderma atroviride to the presence of a fungal prey. BMC Genomics 10:567. doi: 10.1186/1471-2164-10-567
Sharma, K. D., and Muehlbauer, F. J. (2007). Fusarium wilt of chickpea: physiological specialization, genetics of resistance and resistance gene tagging. Euphytica 157, 1–14. doi: 10.1007/s10681-007-9401-y
Shoresh, M., Harman, G. E., and Mastouri, F. (2010). Induced systemic resistance and plant responses to fungal biocontrol agents. Annu. Rev. Phytopathol. 48, 21–43. doi: 10.1146/annurev-phyto-073009-114450
Spraker, J. E., Jewell, K., Roze, L. V., Scherf, J., Ndagano, D., Beaudry, R., et al. (2014). A volatile relationship: profiling an inter-kingdom dialogue between two plant pathogens, Ralstonia solanacearum and Aspergillus flavus. J. Chem. Ecol. 40, 502–513. doi: 10.1007/s10886-014-0432-2
Stoppacher, N., Kluger, B., Zeilinger, S., Krska, R., and Schuhmacher, R. (2010). Identification and profiling of volatile metabolites of the biocontrol fungus Trichoderma atroviride by HS-SPME-GC-MS. J. Microbiol. Methods 81, 187–193. doi: 10.1016/j.mimet.2010.03.011
Timmusk, S., Behers, L., Muthoni, J., Muraya, A., and Aronsson, A.-C. (2017). Perspectives and challenges of microbial application for crop improvement. Front. Plant Sci. 8:49. doi: 10.3389/fpls.2017.00049
Tyc, O., Song, C., Dickschat, J. S., Vos, M., and Garbeva, P. (2017). The ecological role of volatile and soluble secondary metabolites produced by soil bacteria. Trends Microbiol. 25, 280–292. doi: 10.1016/j.tim.2016.12.002
Vieira, P. M., Coelho, A. S. G., Steindorff, A. S., de Siqueira, S. J. L., Silva, R. D. N., and Ulhoa, C. J. (2013). Identification of differentially expressed genes from Trichoderma harzianum during growth on cell wall of Fusarium solani as a tool for biotechnological application. BMC Genomics 14:177. doi: 10.1186/1471-2164-14-177
Vinale, F., Sivasithamparam, K., Ghisalberti, E. L., Woo, S. L., Nigro, M., Marra, R., et al. (2014). Trichoderma secondary metabolites active on plants and fungal pathogens. Open Mycol. J. 8, 127–139. doi: 10.2174/1874437001408010127
Vincent, D., Plummer, K. M., Solomon, P. S., Lebrun, M.-H., Job, D., and Rafiqi, M. (2016). Editorial: how can secretomics help unravel the secrets of plant-microbe interactions? Front. Plant Sci. 7:1777. doi: 10.3389/fpls.2016.01777
Vos, C. M. F., De Cremer, K., Cammue, B. P. A., and De Coninck, B. (2015). The toolbox of Trichoderma spp. in the biocontrol of Botrytis cinerea disease. Mol. Plant Pathol. 16, 400–412. doi: 10.1111/mpp.12189
Wheatley, R., Hackett, C., Bruce, A., and Kundzewicz, A. (1997). Effect of substrate composition on production of volatile organic compounds from Trichoderma spp. inhibitory to wood decay fungi. Int. Biodeterior. Biodegrad. 39, 199–205. doi: 10.1016/S0964-8305(97)00015-2
Woo, S. L., Ruocco, M., Vinale, F., Nigro, M., Marra, R., Lombardi, N., et al. (2014). Trichoderma-based products and their widespread use in agriculture. Open Mycol. J. 8, 71–126. doi: 10.2174/1874437001408010071
Woo, S. L., Scala, F., Ruocco, M., and Lorito, M. (2006). The molecular biology of the interactions between Trichoderma spp., phytopathogenic fungi, and plants. Phytopathology 96, 181–185. doi: 10.1094/PHYTO-96-0181
Worasatit, N., Sivasithamparam, K., Ghisalberti, E. L., and Rowland, C. (1994). Variation in pyrone production, lytic enzymes and control of rhizoctonia root rot of wheat among single-spore isolates of Trichoderma koningii. Mycol. Res. 98, 1357–1363. doi: 10.1016/S0953-7562(09)81063-0
Wu, Y. W., Ouyang, J., Xiao, X. H., Gao, W. Y., and Liu, Y. (2006). Antimicrobial properties and toxicity of anthraquinones by microcalorimetric bioassay. Chinese J. Chem. 24, 45–50. doi: 10.1002/cjoc.200690020
Yamagiwa, Y., Inagaki, Y., Ichinose, Y., Toyoda, K., Hyakumachi, M., and Shiraishi, T. (2011). Talaromyces wortmannii FS2 emits β-caryphyllene, which promotes plant growth and induces resistance. J. Gen. Plant Pathol. 77, 336–341. doi: 10.1007/s10327-011-0340-z
Yao, L., Tan, C., Song, J., Yang, Q., Yu, L., and Li, X. (2016). Isolation and expression of two polyketide synthase genes from Trichoderma harzianum 88 during mycoparasitism. Braz. J. Microbiol. 47, 468–479. doi: 10.1016/j.bjm.2016.01.004
Keywords: antifungal metabolites, biological control, fungal chemical ecology, Fusarium oxysporum, Trichoderma, volatile compounds
Citation: Li N, Alfiky A, Wang W, Islam M, Nourollahi K, Liu X and Kang S (2018) Volatile Compound-Mediated Recognition and Inhibition Between Trichoderma Biocontrol Agents and Fusarium oxysporum. Front. Microbiol. 9:2614. doi: 10.3389/fmicb.2018.02614
Received: 06 July 2018; Accepted: 12 October 2018;
Published: 31 October 2018.
Edited by:
Weiguo Fang, Zhejiang University, ChinaReviewed by:
Monika Schmoll, Austrian Institute of Technology (AIT), AustriaYoun-Sig Kwak, Gyeongsang National University, South Korea
Copyright © 2018 Li, Alfiky, Wang, Islam, Nourollahi, Liu and Kang. This is an open-access article distributed under the terms of the Creative Commons Attribution License (CC BY). The use, distribution or reproduction in other forums is permitted, provided the original author(s) and the copyright owner(s) are credited and that the original publication in this journal is cited, in accordance with accepted academic practice. No use, distribution or reproduction is permitted which does not comply with these terms.
*Correspondence: Seogchan Kang, c3hrNTVAcHN1LmVkdQ==
† These authors have contributed equally to this work