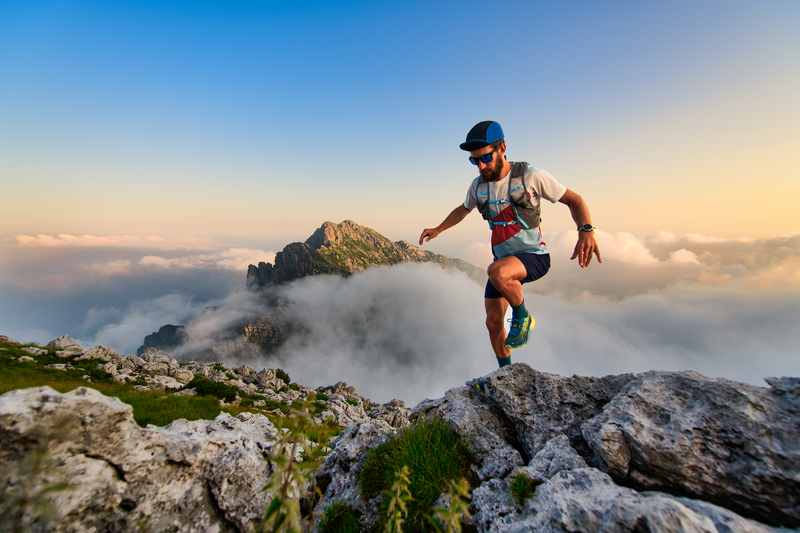
95% of researchers rate our articles as excellent or good
Learn more about the work of our research integrity team to safeguard the quality of each article we publish.
Find out more
REVIEW article
Front. Microbiol. , 30 October 2018
Sec. Microbial Immunology
Volume 9 - 2018 | https://doi.org/10.3389/fmicb.2018.02603
This article is part of the Research Topic Towards Host-Directed Drug Therapies for Infectious and Non-Communicable Diseases View all 11 articles
Impaired lung function is common in people with a history of tuberculosis. Host-directed therapy added to tuberculosis treatment may reduce lung damage and result in improved lung function. An understanding of the pathogenesis of pulmonary damage in TB is fundamental to successfully predicting which interventions could be beneficial. In this review, we describe the different features of TB immunopathology that lead to impaired lung function, namely cavities, bronchiectasis, and fibrosis. We discuss the immunological processes that cause lung damage, focusing on studies performed in humans, and using chest radiograph abnormalities as a marker for pulmonary damage. We highlight the roles of matrix metalloproteinases, neutrophils, eicosanoids and cytokines, like tumor necrosis factor-α and interleukin 1β, as well as the role of HIV co-infection. Finally, we focus on various existing drugs that affect one or more of the immunological mediators of lung damage and could therefore play a role as host-directed therapy.
In 2016, an estimated 10.4 million people developed tuberculosis (TB) worldwide. Although effective diagnosis and treatment saved about 53 million lives between 2000 and 2016, TB remains a major threat worldwide: 16% of TB cases die from the disease, corresponding to 1.7 million deaths in 2016 (World Health Organization, 2017). Among those who are cured successfully, residual pulmonary impairment is common. Various studies have looked at lung function in patients with a known history of TB; they found abnormal lung function in 34 – 94% of patients, varying in severity from mild to severe (Willcox and Ferguson, 1989; Plit et al., 1998; de Valliere and Barker, 2004; Chung et al., 2011; Vecino et al., 2011; Akkara et al., 2013; Baez-Saldana et al., 2013; Ralph et al., 2013a; de la Mora et al., 2015; Nihues Sde et al., 2015; Manji et al., 2016). It results in considerable medical costs (Jordan et al., 2010) and decreased quality of life (Ralph et al., 2013a; de la Mora et al., 2015).
Impaired lung function is associated with chest radiograph (CXR) abnormalities in most of the studies. It can easily be measured using spirometry, which measures air volumes and airflow rates of the lung. Forced vital capacity (FVC) is the maximal volume of air exhaled by a patient from the position of maximal inspiration, by means of a rapid, maximally forced expiration; forced expiratory volume in 1 s (FEV1) is the amount of air exhaled during the first second of the FVC maneuver. The nature and severity of pulmonary impairment can be categorized by combining these two measurements: obstruction is defined as a FEV1/FVC ratio < 70%, restriction is suggested by a low FVC (<80% of the predicted value). Obstruction, low FVC, and mixed defects have all been reported in patients with previous TB.
The aim of TB treatment is to kill the causative mycobacteria with anti-mycobacterial agents. Because of the lengthy duration of the treatment, the possibilities of drug toxicity, and increasing drug resistance, host-directed therapies (HDT), have gained attention (Hawn et al., 2013; Wallis and Hafner, 2015; Zumla et al., 2015). HDTs are agents that can augment host defense mechanisms, modulate excessive inflammation or both, by manipulating the hosts response to a pathogen rather than targeting the pathogen itself. This may lead to improved clinical treatment outcomes such as reduced morbidity, mortality, and end-organ damage, and long-term functional recovery. Supplementing anti-TB treatment with drugs that reduce pulmonary damage could result in improved pulmonary function. To predict which interventions could be beneficial, an understanding of the pathogenesis of pulmonary damage in TB is important. What are the immunological processes leading to lung damage in humans? Where and how in the process could we intervene to prevent or reduce lung damage? How much damage is already done at diagnosis and how much still occurs during treatment?
The established paradigm positions the caseating granuloma as the characteristic lesion of TB. However, this paradigm originates from animal studies in the late 20th century, when data on histology of human TB had become rare. Studies done before the 1950s describe two characteristic presentations in human pulmonary TB: the caseous granuloma and the tuberculous pneumonia. They divide lung pathology into primary and post-primary TB. Primary TB is the infection that occurs when people first encounter Mycobacterium tuberculosis (Mtb). Post-primary TB occurs later, as a result of reactivation of latent TB or reinfection, and causes the majority of clinical TB (Hunter, 2011). The two differ with regard to their location in the lung, the host immune response and their histopathology. Primary TB typically occurs mainly in the lower zones of the lung. It is usually self-limiting but leads to consolidative pneumonia or lymphadenitis in a small proportion of individuals. It is characterized by a greater bacillary load and reduced lipid accumulation in the alveoli and the interstitium compared to post-primary TB, as well as an acute inflammatory response; cavitation however, is rare. Post-primary TB is said to develop mainly in the apices of the lung. It is characterized by obstructive pneumonia, which is frequently asymptomatic in its early stages. Endobronchial spread from the small peripheral airways can lead to necrotic caseous pneumonia, associated with progressive tissue necrosis and cavity formation or fibrocaseous disease (Long et al., 1998; Hunter, 2016). TB typically heals with persisting cavities, scarring, and pleural adhesions, as observed in autopsies of persons with previous TB who died of other causes (Theegarten et al., 2006). However, abnormal findings need not be present and viable TB can be found in both macroscopically normal and abnormal appearing lung tissue (Kuhne and Willgeroth, 1988).
Chest radiographs are commonly used to visualize pulmonary damage. Radiologists distinguish primary and post-primary TB as the two typical patterns in active TB. Primary TB is characterized by lymphadenopathy and air space consolidation often in the middle or lower lobes, with or without an accompanying pleural effusion. Post-primary TB consists of consolidation and/or nodules, frequently in the upper lobes or apices of the lower lobes, with or without cavitation (Nachiappan et al., 2017). CXRs of people with previous TB show abnormalities in 14–100%, including fibrosis, bronchiectasis, and persisting cavities, the latter occurring more often in re-treatment patients or those with multi-drug resistant TB (Meghji et al., 2016). All these abnormalities are associated with impaired lung function.
Computed tomography (CT) scans are more sensitive than CXRs, especially for imaging of centrilobular small nodules or the so-called tree-in-bud sign; these classical features of early endobronchial spread of TB are often underestimated on a CXR (Skoura et al., 2015); [18F]-fluoro-2-deoxy-D-glucose positron emission tomography (FDG-PET) with CT combines anatomic imaging with imaging of metabolic activity of lesions. It has been used in TB to follow the evolution of lung lesions during treatment (Martinez et al., 2012; Malherbe et al., 2016) and, importantly, has shown that metabolically active lung lesions may be present before the onset of clinical disease (Esmail et al., 2016), and persist after treatment completion (Malherbe et al., 2016).
After Mtb enters the lung, the bacilli are taken up by alveolar macrophages, dendritic cells, and neutrophils, or occasionally epithelial cells; the latter possibly resulting in limited early bacterial growth. Infected cells start producing and secreting antimicrobial peptides, cytokines (like interleukin (IL)-1β, tumor necrosis factor (TNF)-α, IL-12, and IL-6) and chemokines. Other immune cells and permissive macrophages are attracted to the site of infection (O’Garra et al., 2013). Mtb itself, using multiple strategies, directs the recruitment of macrophages and triggers granuloma formation (Ndlovu and Marakalala, 2016). Secondary granulomas are formed by infected macrophages departing the primary granuloma or when a granuloma ruptures. While Mtb replicates freely in the macrophages, dendritic cells migrate to the local lymph nodes, to activate T cells. The arrival of Mtb specific T-cells in the lung usually does not happen until 14–21 days after initiation of the infection (Gallegos et al., 2008). Their production of TNF-α and interferon-γ (IFN-γ) stimulates killing activities by macrophages. Moreover, T-cells complete granuloma formation by forming the lymphocytic cuff surrounding it (O’Garra et al., 2013).
The balance between the eicosanoids prostaglandin E2 (PGE2) and lipoxin A4 (LXA4) affects the mode of death of infected macrophages. LXA4 promotes macrophage necrosis, resulting in cell lysis of the macrophage, thereby allowing Mtb to escape and spread to neighboring cells. PGE2 stimulates apoptosis, leaving the macrophage plasma membrane intact, containing the bacilli, and enhancing immunity (Chen et al., 2008). Leukotriene (LT) B4, through regulation of TNF-α production (Tobin et al., 2012) and possibly attraction of neutrophils (Lammermann et al., 2013), is also involved, with both high and low levels of LTB4 inducing macrophage necrosis (Tobin et al., 2012).
In only 10% of individuals, progressive primary disease occurs; in the remaining 90% the initial infection is contained and latent infection is established (O’Garra et al., 2013). Current thinking views active and latent TB on a spectrum of tuberculosis disease, rather than as two distinct disease states as historically classified. (Barry et al., 2009).
Most human granulomas are composed of a center of infected macrophages, with the ability to differentiate, for example into epithelioid cells, multi-nucleated giant cells, and foamy macrophages. An outer layer of lymphocytes surrounds these cells, and many other cells, including neutrophils, dendritic cells, natural killer (NK) cells and fibroblasts may form part of the granuloma. The granuloma contains the mycobacteria, preventing their spread, but at the same time serves as a site of replication and persistence for Mtb (Ndlovu and Marakalala, 2016). Different types of granuloma exist: cellular, suppurative, fibrotic, or caseous (Canetti, 1955). Caseous necrosis occurs when cells within the granuloma undergo necrosis (O’Garra et al., 2013); alternatively, it has been suggested that – in post-primary TB - granulomas form in response to existing areas of necrotic caseous pneumonia (Hunter, 2016). Caseous necrosis happens in conjunction with extracellular matrix (ECM) destruction. In the classical paradigm, tissue destruction occurs as a result of caseous necrosis (O’Garra et al., 2013). However, an alternative theory proposes that collagen destruction precedes caseation and, therefore, ECM destruction is the initial pathological event (Al Shammari et al., 2015).
Diverse types of granulomas can be present in one lung at the same time, ranging from small cellular granulomas to multiple caseous granulomas that coalesce and expel their contents to form large cavities; they behave independently of each other, and different immunologic profiles exist between (Ulrichs et al., 2005; Subbian et al., 2015) and within (Marakalala et al., 2016) granulomas. Granulomas can be stable, or either resolve or progress. Clinically, the behavior of a few or even a single poorly controlled granuloma can determine the outcome of the disease on a host level (Flynn, 2018).
The lung consists of both cellular and extracellular components. The ECM is comprised of the interstitial connective tissue matrix, which forms the parenchyma of the lung, surrounding cells and providing structural scaffolding, and the basement membrane, which separates the alveolar epithelium or endothelium from the surrounding stroma. Support of the alveoli by the ECM is needed for normal lung function; destruction or abnormal remodeling of the ECM occurs in many pulmonary diseases and leads to pulmonary impairment (Elkington and Friedland, 2006). The ECM of the lung is mainly made up of type I collagen and elastin. Type III and IV collagen are important components of the alveolar wall and basement membrane. Large fibers are connected by smaller fibrils. Dissemination of mycobacteria from the lung parenchyma into the airways as well as formation of cavities requires destruction of the ECM through cleavage of both small fibrils and large fibers. Collagens, however, are highly resistant to cleavage by proteolytic enzymes; only matrix metalloproteinases (MMPs) are capable of completely degrading the ECM (Elkington and Friedland, 2006). Consequently, MMPs play an important role in the development of cavities, bronchiectasis as well as fibrosis.
The development of cavities in TB has been studied extensively in rabbits, using Mycobacterium bovis. In these studies, cavities developed from liquefied caseating granulomas, that contained large numbers of actively growing bacteria. Bacteria release high amounts of tuberculin-like products causing a tissue-damaging delayed-type hypersensitivity reaction (Dannenberg, 2006). This T-cell mediated immune reaction is important; cavities developed mainly in pre-sensitized rabbits and desensitization or immune suppression could prevent cavity formation (Yamamura et al., 1968; Yamamura et al., 1974). Cavities are formed when expanding granulomas ruptures their caseous contents into a bronchus (Dannenberg, 2006).
Histologic studies in humans show a different picture of cavity formation that challenges the paradigm described in rabbits (Hunter, 2016): cavities do not develop from liquefied caseating granulomas, but from a caseous pneumonia. Host lipids and mycobacterial antigens accumulate in the alveoli, but only small numbers of bacteria are present. Similar to the rabbit model, sudden necrosis related to a delayed-type hypersensitivity reaction against mycobacterial antigens occurs (Hunter, 2016). However, an alternative yet controversial theory, based on the small numbers of bacteria observed and several observations related to autoimmunity seen in patients with TB, proposes a role for autoimmunity: mycobacteria induce inappropriate host responses to self-antigens, causing autoimmune inflammation (Elkington et al., 2016). A considerable overlap in gene expression signatures between TB and autoimmune diseases, greater than seen with other infectious diseases, supports this theory (Clayton et al., 2017).
The lipid-rich necrotic material in granulomas does not have the enzymatic activity to degrade collagen and consequently, its build-up is only one component of cavity formation. Extracellular matrix breakdown takes place and involves MMPs. Indeed, increased concentrations of MMPs have been found in TB cavities in rabbits (Kubler et al., 2015) and in humans (Sakamoto et al., 2013; Ong et al., 2015). Neutrophils have also been found in cavities (Ong et al., 2015).
Bronchiectasis, an irreversible dilatation of the bronchi, is caused by an ongoing inflammatory process (like TB), which results in damage to the airway epithelium, leading to an inability to clear secretions, as well as destruction of the elastin in the airway walls (Milliron et al., 2015). Similar to cavity formation, MMPs have been implicated in the development of bronchiectasis, with increased levels being found in sputum, bronchoalveolar lavage fluid (BALF), and the lamina propria of patients with bronchiectasis (Sepper et al., 1995; Zheng et al., 2002; Guan et al., 2015). Neutrophils, together with macrophages and T-cells, are the dominant cell type in bronchiectatic inflammation (King, 2009). Alternatively, traction bronchiectasis can occur, secondary to scarring of the adjacent parenchyma or narrowing of more proximal bronchi (Milliron et al., 2015).
Fibrosis results from the excessive deposition of components of the ECM such as collagen and fibronectin in and around inflamed or damaged tissue by myofibroblasts. Its pathogenesis is complicated (Wynn and Ramalingam, 2012), with many innate and adaptive immune cells and cytokines playing a role. Transforming growth factor (TGF-β), produced by macrophages, lung epithelial cells, and fibroblasts, is one of the key players (Wynn and Ramalingam, 2012) and indeed, higher levels of TGF-β in serum and BALF correlate with an increase in fibrosis seen on high-resolution CT scan in patients with TB 6 months after the start of treatment (Ameglio et al., 2005). TNF-α, IL-β, and IL-17-induced neutrophil recruitment also seems to play a crucial role in the development of fibrosis (Wynn and Ramalingam, 2012). MMPs appear to be involved: some MMPs reduce fibrosis, but others – perhaps counterintuitively – promote it (Giannandrea and Parks, 2014). In a Taiwanese study, patients with an MMP-1 (-1607G) gene polymorphism, leading to excessive MMP-1 production, were more likely to have moderate to advanced fibrosis on CXR 1 year after completion of TB treatment (Wang et al., 2010).
Much of our recent knowledge of immunological processes in TB comes from animal models. Mice, rabbits, guinea pigs, and zebra-fish have all been used to study TB. However, none of these models completely replicate the immunopathology seen in human TB. More recently, non-human primates have also been used, exhibiting a spectrum of pathology closely resembling TB in humans (Flynn et al., 2015).
For this review, we included studies done in humans, where serum and BALF markers are commonly used to assess the immunological processes in the lung. Serum measurements reflect systemic responses and do not represent what happens in individual granulomas, as was shown by a difference in gene expression patterns between granuloma and blood (Subbian et al., 2015). BALF more closely reflects responses taking place in the lung, however, even BALF only reflects processes taking place in the airways and not necessarily those in the lung parenchyma. Histology is the only way to assess the immunological processes occurring within a granuloma; however, histological samples are more difficult to obtain and, therefore, most study findings in humans are built on assumptions using available body fluid. Studies that do include histological samples cannot present longitudinal data.
When conducting our review, we searched for studies that assessed inflammatory mediators, and associated them with radiological abnormalities as a marker for pulmonary damage (Figure 1).
FIGURE 1. Mediators of lung damage in TB and interplay with [lightning flash cartoon], virulent Mtb; COX, cyclooxygenase; IFN-I, type I interferon; IFN-γ, interferon gamma; IL, interleukin; LOX, lipoxygenase; LT, leukotriene; LTA4H, leukotriene A4 hydrolase; LX, lipoxin; mφ, macrophage; MMP, matrix metalloproteinase; Mtb, mycobacterium tuberculosis; neu, neutrophil recruitment; NO, nitric oxide; PGE2, prostaglandin E2; TNF, tumor necrosis factor. NO inhibits assembly of the NLRP3 inflammasome (Mishra et al., 2013).
There are 23 MMPs in humans. They can be secreted by a variety of cells, including macrophages/monocytes, neutrophils, and lung epithelial cells. Their generation is tightly regulated. They are not stored requiring gene transcription immediately before secretion; exceptions being MMP-8 and -9 stored in neutrophils. Once activated, they are regulated by endogenous inhibitors, called tissue inhibitors of metalloproteinases (TIMPs). Expression of MMPs is increased by prostaglandin and several cytokines (including IL-1β, IL-17 (Singh et al., 2018), TNF-α, and IFN-γ) (Elkington P.T. et al., 2011); hypoxic conditions, present in TB lesions, also increase expression and secretion of MMP-1 through the induction of hypoxia-inducible factor 1α (Belton et al., 2016). A recent study has demonstrated a role for platelets in MMP-1 upregulation in Mtb-infected monocytes, in addition to upregulation of IL-1β and IL-10 (Fox et al., 2018).
As described above, degradation of collagens and elastins by MMPs during active TB leads to the formation of cavities. Strong evidence of the role of MMPs in lung damage comes from studies in transgenic mice expressing human MMP-1. Wildtype mice do not express the ortholog of MMP-1 in lung and do not develop caseous necrosis or cavities in response to Mtb; in human MMP-1 transgenic mice, however, infection with TB leads to collagen destruction and caseous necrosis (Elkington P. et al., 2011; Al Shammari et al., 2015). MMPs also play a role in granuloma formation (Parasa et al., 2017).
Several MMPs are upregulated in blood, sputum, and BALF of patients with active TB, primarily MMP-1, -3, -7, -8, and -9 (Elkington P.T. et al., 2011). MMP-1 is the dominant collagenase in TB (Elkington P. et al., 2011); its secretion is driven by Mtb directly by activation of multiple intracellular signaling pathways and by intercellular networks (Ong et al., 2014). Corresponding TIMPs are not similarly upregulated by Mtb, leading to a matrix-degrading phenotype in TB (Price et al., 2001). In a zebrafish model, using M. marinum to study granuloma formation, mycobacterial-derived ESAT-6 induced MMP-9 secretion, enhancing monocyte recruitment to granulomas (Taylor et al., 2006; Volkman et al., 2010).
Increased levels of MMPs correlate with pulmonary damage: sputum levels of MMP-1, -2, and -8 were elevated in patients with cavities and correlated positively with the extent of infiltrates on CXR (Walker et al., 2012; Ong et al., 2015). Similarly, sputum levels of membrane type-1 MMP (a membrane-bound collagenase expressed on monocytes), plasma concentrations of procollagen III N-terminal propeptide (PIIINP, a degradation product of collagen type III), BALF levels of MMP-3, -7, and -8, and serum concentrations of MMP- 1, -8, and -9, correlated with more extensive CXR abnormalities in patients with TB from several different countries (Hrabec et al., 2002; Seddon et al., 2013; Singh et al., 2014a; Sathyamoorthy et al., 2015; Sigal et al., 2017). These findings suggest a central role for MMPs and extracellular matrix degradation in the development of lung damage in TB.
Neutrophils are abundant in the airways of humans with active TB (Eum et al., 2010). Their role in TB appears dichotomous: high numbers of neutrophils in the blood at the time of exposure are associated with lower likelihood of infection (Martineau et al., 2007). Conversely later in TB their numbers in blood were associated with worse patient outcomes (Barnes et al., 1988; Lowe et al., 2013). Various soluble mediators (amongst others Il-1β, IL-8, IL-17, PGE2, LTB4, and granulocyte colony-stimulating factor) promote neutrophil recruitment (Lowe et al., 2012); others, like IFN-γ and nitric oxide (NO), reduce neutrophil recruitment and survival, partly via inhibition of IL-17 (Nandi and Behar, 2011), IL-1β and 12-lipooxygenase (12-LOX) (Mishra et al., 2017).
At the time of presentation with active TB, neutrophils are associated with lung damage: a neutrophil-driven, IFN-inducible whole-blood transcript signature (Berry et al., 2010), higher blood (Abakay et al., 2015; Panteleev et al., 2017) and BALF (Nolan et al., 2013) neutrophil counts, and higher serum levels of S100 proteins (a protein produced by neutrophils, promoting their own recruitment) (Gopal et al., 2013; Berrocal-Almanza et al., 2016) in patients with active TB all relate with the extent of lung radiographic disease. Lung damage is thought to be contributed to by their indiscriminate killing mechanisms, which can result in significant bystander damage to surrounding host tissue. Moreover, neutrophils are the only cells that store MMPs (Ong et al., 2015), while they do not synthesize TIMPs, thus allowing for unrestrained effects of MMPs (Masure et al., 1991). Removing infected or dying neutrophils is necessary to protect the surrounding tissue. Removal of apoptotic neutrophils by macrophages promotes subsequent killing of Mtb, whereas removal of necrotic neutrophils allows for mycobacterial survival and proliferation inside the macrophages. Mtb drives neutrophil necrosis, a process that requires neutrophil-derived reactive oxygen species (ROS) (Dallenga et al., 2017b). Inhibition of ROS-production could restore growth control of Mtb by macrophages (Dallenga et al., 2017a).
The eicosanoids PGE2, LXA4, and LTB4 are all metabolites of arachidonic acid (AA). Cyclooxygenase (COX) converts AA into PGE2, while 5-lipooxygenase (5-LOX) generates LTA4, which is again converted into either LXA4 by 12-LOX, or LTB4 by leukotriene A4 hydrolase (LTA4H) (Dietzold et al., 2015). As mentioned previously, the balance between these eicosanoids influences the mechanism of macrophage death (Chen et al., 2008). Macrophage apoptosis leads to an early immune response with better control of the infection and minimal immunopathology, while macrophage necrosis leads to a delayed immune response, inadequate control of infection and greater immunopathology (Divangahi et al., 2013). Virulent strains of Mtb promote LXA4 production, thereby stimulating necrosis and mycobacterial spread (Chen et al., 2008). To our knowledge, no studies have correlated PGE2 or LXA4 with pulmonary function in human TB; one can speculate that tipping the eicosanoid-balance toward PGE2 may result in less lung damage. Findings in mice and latent TB in humans, however, show that levels of PGE2 were low early in the infection and increased later in and during active TB (Rangel Moreno et al., 2002; Shu et al., 2013; Mayer-Barber et al., 2014; Lee et al., 2015). This underlines the complex and poorly elucidated role of PGE2 in TB infection and may even suggest a changing role for PGE2 during the course of the disease. LTB4, which is generated by LTA4H, has been correlated with severity of TB on CXRs in one study (el-Ahmady et al., 1997).
Various studies have assessed the association between cytokines (including IFN–y and TNF–α, and several pro- and anti-inflammatory interleukins) and CXR abnormalities in TB (Dlugovitzky et al., 1997; Sodhi et al., 1997; Casarini et al., 1999; Tsao et al., 1999, 2000, 2002; van Crevel et al., 2000; Mazzarella et al., 2003; Ameglio et al., 2005; Wu et al., 2007; Berry et al., 2010; Su et al., 2010; Walker et al., 2012; Nolan et al., 2013; Chowdhury et al., 2014; Fan et al., 2015; Sigal et al., 2017). The different measuring methods used and the fact that several cytokines are not limited to a single effector function make comparison and interpretation challenging.
Only TNF-α and IL-1β in both blood and BALF seem to unambiguously correlate with CXR abnormalities. Higher levels of TNF-α and IL-1β correlate with the presence or size of cavities (Tsao et al., 2000; Ameglio et al., 2005; Chowdhury et al., 2014; Sigal et al., 2017) and with the extent of pulmonary involvement (Casarini et al., 1999; Walker et al., 2012). Moreover, lower levels of these cytokines were found in patients with an early radiological response to TB treatment (improved CXR after 2 months of treatment) compared to those with a later (at 6 months) response (Su et al., 2010). In animal models, the effect of TNF-α seems to be dose dependent, where both high and low doses lead to tissue destruction (Bekker et al., 2000; Tobin et al., 2012). LTA4H polymorphism, and subsequently eicosanoid patterns, play a role in its regulation (Tobin et al., 2012). Both TNF-α and IL-1β affect secretion of MMPs and MMPs in their turn can play a role in the release, activation or inactivation of TNF-α and IL-1β (Elkington and Friedland, 2006). IL-1β also associates with activation of fibroblasts (Borthwick, 2016) and the recruitment of neutrophils (Lowe et al., 2012; Mishra et al., 2017), which all associate with lung damage.
Autophagy is an intracellular self-digestion process: cytosolic material is engulfed by a double-membrane vesicle called the autophagosome, that delivers it to lysosomes for degradation and subsequently releases the degraded products back to the cytosol. Autophagy can be used by the host to eliminate intracellular pathogens and plays an important role in defense against Mtb (Gutierrez et al., 2004); both IFN-γ and TNF-α can induce autophagy (Songane et al., 2012). It can also downregulate IL-1β production mediated through the inflammasome (an intracellular multiprotein complex that triggers formation of proinflammatory cytokines), by removing large inflammasome complexes or damaged mitochondria - which, through production of ROS, trigger the inflammasome (Rathinam et al., 2012). Virulent Mtb can inhibit autophagy (Gupta et al., 2016), subsequently leading to increased IL-1β production (Songane et al., 2012). It was found that patients infected by Mtb strains with poor in vitro autophagy-inducing ability displayed more severe radiographic extent of disease (Li et al., 2016). Consequently, inducing autophagy could limit lung damage.
Globally, 13 percent of people with active TB who know their HIV status are co-infected with HIV-1 (World Health Organization, 2017). Although TB is also a risk factor for airflow obstruction in patients with HIV (Samperiz et al., 2014; Pefura-Yone et al., 2015; Gupte et al., 2017), in HIV positive patients with a low CD4 count (CD4 < 200/mm3) TB often presents with atypical CXR findings or even normal CXRs, while cavitation is 4-fold less common (Kwan and Ernst, 2011). These findings suggest that TB-related pulmonary damage might be reduced in HIV co-infected patients and the host immune response, necessary for protection against TB, is required for the development of cavities. Indeed, several of the factors previously discussed and implicated in pulmonary damage, are affected by HIV co-infection. For example, sputum levels of MMP-1, -2, -8, and -9 are reduced in HIV-TB co-infected patients, compared to patients without HIV (Walker et al., 2012, 2017) as is the activity and life span of neutrophils (Lowe et al., 2015). The effect of HIV co-infection on the levels of several of the other cytokines is variable across studies and thus it is difficult to interpret a clear trend (Zhang et al., 1994; Elliott et al., 1999; de Castro Cunha et al., 2005; Riou et al., 2012; Walker et al., 2012; Mihret et al., 2014; Kassa et al., 2016).
Paradoxical TB-associated immune reconstitution inflammatory syndrome (TB-IRIS) develops in approximately 18% (95% CI 16–21%) of patients on treatment for HIV-associated TB, usually within the first few weeks after starting ART (Namale et al., 2015). It results in new or recurrent TB signs and symptoms, commonly involving the lungs, such as cough, chest pain, and worsening radiographic pulmonary infiltrates. TB-IRIS is associated with increased levels of several cytokines, particularly IL-6, TNF-α and IFN-γ (Tadokera et al., 2011; Conesa-Botella et al., 2012; Lai et al., 2015; Ravimohan et al., 2015) and inflammasome activation (Lai et al., 2015). It results in increased neutrophil recruitment (Nakiwala et al., 2018), and up-regulation of MMP-1, -3, -7, -8, and -10 (Tadokera et al., 2014; Ravimohan et al., 2016; Walker et al., 2017). LT4AH also appears to play a role, as more severe TB-IRIS has been reported in patients with mutant (TT and CT) LTA4H genotypes (Narendran et al., 2016).
These findings suggest that TB-IRIS could result in pulmonary damage and impaired lung function. To date, only one study has explored the relationship between TB-IRIS and lung function in 14 patients with HIV-associated TB, 3 of whom developed TB-IRIS (Ravimohan et al., 2016). The study found that an increase in MMP-8 between baseline pre-ART and 4 weeks post-ART initiation strongly associated with impairment in lung function, but the small sample size limits definitive conclusions.
There are several uncertain areas around therapies to prevent or limit lung damage in TB. Changes in the lungs start to develop before clinical symptoms appear (Esmail et al., 2016; Zak et al., 2016; Scriba et al., 2017), and therefore, a large proportion of lung damage may already have occurred by the time the patient presents; several mediators of lung damage may have different roles at different stages of the disease; granulomas in various stages can be present at the same time in a single individual, and only a single or a few progressive granulomas can determine the outcome of the disease. Therefore, it remains uncertain what happens for example to the contained granulomas if we systemically treat the patient with potentially immunosuppressive therapy or what the right time is to intervene (Figure 2).
FIGURE 2. The potential effect of immune mediators on the development of lung damage at different stages of disease. G-CSF, granulocyte-colony stimulating factor; IL, interleukin; LTB4, leukotriene B4; LXA4, lipoxin A4; mφ, macrophage; neutro, neutrophils; NO, nitric oxide; PGE2, prostaglandin E2; TNF, tumor necrosis factor.
Sputum Mtb load is associated with systemic inflammation and, combined with pre-treatment C-reactive protein levels, inversely correlates with CXR improvement 60 days after start of treatment (Mesquita et al., 2016). Time between first TB symptoms and start of treatment (de Valliere and Barker, 2004; Baez-Saldana et al., 2013), duration of treatment (Chung et al., 2011), and smear positivity (Chung et al., 2011) are associated with impaired pulmonary function, suggesting that prompt diagnosis and treatment will limit lung damage. In addition to a direct anti-mycobacterial effect, in vitro studies suggest that some antimycobacterial agents may have immunomodulatory action. Pyrazinamide directly reduces levels of TNF-α, IL-6 and IL-1β (Manca et al., 2013), quinolones downregulate MMP-1, -3, and -9 (Singh et al., 2014a), and rifampicin downregulates MMP-3 production by bronchial epithelial cells (Singh et al., 2014a) and inhibits PGE2 production (Yuhas et al., 2007). P-aminosalicylic acid (PAS), which is an aspirin derivate, suppresses PGE2-dependent MMP-1 production (Rand et al., 2009). Both isoniazid (INH) and pyrazinamide (PZA) enhance autophagy (Kim et al., 2012).
In an adjunctive approach to TB therapy, treatment could be supplemented with host-directed therapies. Several readily available drugs affect cytokines, MMPs or eicosanoids and therefore potentially reduce pulmonary damage (Table 1).
Steroids have been used as adjunctive treatment in TB for several decades (Dooley et al., 1997; Critchley et al., 2013), mainly in TB meningitis, pericarditis, and TB-IRIS, even though corticosteroid use without concomitant TB treatment increases the risk of developing TB (Jick et al., 2006). Two recent reviews concluded that there is no high quality evidence that steroid treatment significantly affects mortality or sputum conversion rate in pulmonary TB (Critchley et al., 2014; Schutz et al., 2018). An earlier review – including mostly studies done in the 1960s and patients not on rifampicin-based TB treatment – did find a beneficial effect of steroids on radiographic resolution and regression of cavities (Smego and Ahmed, 2003). A meta-regression analysis of 12 studies found steroids do accelerate sputum TB culture conversion (Wallis, 2014) – which is inversely associated with development of airflow obstruction (Radovic et al., 2016); however, high doses (134 mg prednisone daily) for an extended period (2 months) are required to reach clinically relevant outcomes (Wallis, 2014). Moreover, the only two studies in this analysis in which patients were on rifampicin-based treatment show contradicting results.
Corticosteroids inhibit various cytokines in TB (IFN-γ, TNF-α, IL-1β) and TB-IRIS (IL-6, IL-10, IL-12p40, TNF-α, IFN-γ, and IP-10) (Mahuad et al., 2004; Mayanja-Kizza et al., 2005; Meintjes et al., 2012; Bongiovanni et al., 2015). In patients with tuberculous meningitis, the effect of corticosteroids was found to be LTA4H genotype modulated, with only patients with the mutant TT genotype, leading to a higher inflammatory response, benefitting from steroid treatment (Tobin et al., 2012). In patients with TB-IRIS, however, this difference in genotype on the effect of steroid treatment was not confirmed (Narendran et al., 2016). The effect of corticosteroid treatment and TB-IRIS on pulmonary function is being assessed in a substudy of the PredART trial (Meintjes et al., 2017).
Little evidence is available for other TNF-α blocking therapies. A trial of 16 patients with HIV-associated TB treated with etanercept (but no ART) showed a tendency to greater CXR improvement from baseline to 6 months compared to a placebo group, although this was not statistically significant (p = 0.2) (Wallis et al., 2004). Case reports describe successful treatment of paradoxical TB reactions or TB-IRIS – involving the pleura, lymph nodes or brain – with infliximab (Blackmore et al., 2008; Jorge et al., 2012; Hsu et al., 2016), or adalimumab (Wallis et al., 2009; Lee et al., 2012). Although only one case refers to pulmonary TB-IRIS [occurring after interruption of prior anti-TNF-α treatment (Wallis et al., 2009)], these case reports support the possible benefits of TNF-α blockers in the treatment of (complicated) TB. Restarting TNF-α blockers during or after TB treatment was safe and only led to one recurrence of TB in a cohort of 22 patients in Turkey followed for a median of 53 months (Ozguler et al., 2016).
Doxycycline is the only licensed MMP-inhibitor for use in humans. It suppresses MMP-1, -3, and -9 secretion by Mtb infected human macrophages and bronchial epithelial cells (Walker et al., 2012). Other agents also inhibit MMPs in vitro: prednisone – in patients with TB-IRIS – suppresses MMP-7 gene expression (Tadokera et al., 2014), vitamin D inhibits secretion of MMP-7 and -9 (Anand and Selvaraj, 2009; Coussens et al., 2009), and rapamycin (an mTOR-inhibitor and a known autophagy inducer that can also affect macrophage polarization (Mercalli et al., 2013)) inhibits MMP-1 and MMP-3 (Singh et al., 2014b). Use of the latter in TB is limited by the interaction with rifampicin. In mice, broad spectrum inhibition of MMPs enhances the efficacy of INH and RIF treatment (Xu et al., 2018). Conceptually, inhibition of MMPs may lead to less pulmonary damage, but so far, no clinical trials have directly assessed this. Currently, everolimus, a rapamycin derivate, is being tested as HDT in patients with moderate to far advanced pulmonary tuberculosis (together with vitamin D, auranofin [a gold complex with antimicrobial activity used in rheumatoid arthiritis], and CC-11050 [a phosphodiesterase 4 (PDE4) inhibitor]), using rifabutin-based anti-TB treatment (ClinicalTrials.gov NCT02968927); with change in FEV1 being one of the secondary outcomes. Both rapamycin, its derivates, and vitamin D could theoretically reduce lung damage through inhibition of MMPs, although the effect of vitamin D treatment on CXR abnormalities is variable (see below). PDE4 inhibitors, in combination with INH treatment, have been shown to reduce TB-associated lung damage in rabbits (Subbian et al., 2011) and pulmonary bacillary load in mice (Maiga et al., 2015). Doxycycline is being investigated for its potentially modulating effect on tissue destruction in pulmonary TB (ClinicalTrials.gov NCT02774993).
NSAIDs inhibit the enzyme cyclooxygenase (COX), thereby inhibiting PGE2 production and enhancing LXA4 production. An adjunctive role for NSAIDs in treatment of human TB has only been shown for acetylsalicylic acid in reducing PZA-induced arthralgia (Petty and Dalrymple, 1964; Horsfall et al., 1979) and possibly in TB meningitis (Misra et al., 2010; Schoeman et al., 2011; Mai et al., 2018). Negative effects have been described: a Taiwanese study found an association between NSAID use (both traditional NSAIDs and selective COX-2 inhibitors) and an increased risk of active TB (Wu et al., 2017). However, it is not clear whether this association is causative (i.e., decreased apoptosis at the very early stages of TB) or merely reflects an increased use of NSAIDs early during TB. In mice, inhibition of PGE2 by the NSAID ibuprofen was shown to affect lung pathology: inhibition early in the disease process leads to an increase in pulmonary inflammation and pathology (Rangel Moreno et al., 2002), whereas inhibition later during disease decreased lung pathology and neutrophil influx (Rangel Moreno et al., 2002; Vilaplana et al., 2013). Increasing PGE2 by early (day one post infection) administration of exogenous PGE2 (dinoproston – normally used for induction of labor) and/or the 5-lipo-oxygenase inhibitor zileuton (used in the treatment of asthma) to IL-1 deficient mice resulted in less necrotic lung pathology by TB (Mayer-Barber et al., 2014). No studies with dinoproston or zileuton have been performed in human TB to date. A pilot study is currently investigating the effect of ibuprofen added to multi-drug resistant TB treatment on radiological improvement of TB, amongst other endpoints (ClinicalTrials.gov NCT02781909).
In in vitro models, metformin, a widely used antidiabetic agent, has been shown to inhibit TNF production by monocytes (Arai et al., 2010), affect macrophage polarization (Nadella et al., 2017), and promote autophagy (Singhal et al., 2014). It affects Th1 responses, but data are conflicting: in mice infected with TB, metformin treatment promotes the expansion of Mtb-specific IFN-γ secreting T cells in the lungs (Singhal et al., 2014), whereas in human THP-1 cells (not infected with Mtb) metformin suppressed the production of Th1-related cytokines (Chen et al., 2018). Metformin use in patients with diabetes mellitus on treatment for TB was associated with decreased mortality compared to patients using other anti-diabetic drugs in two retrospective observational cohorts (Singhal et al., 2014; Degner et al., 2018). A retrospective cohort study of TB patients with diabetes mellitus showed that those using metformin at diagnosis and during TB treatment had fewer cavities and fewer CXR abnormalities compared to those using other anti-diabetic drugs (Singhal et al., 2014). Another retrospective study, however, showed increased cavitatory disease in patients using metformin (Degner et al., 2018).
Vitamin D3 induces autophagy (Campbell and Spector, 2012) and inhibits the secretion of MMP-7, -9 (Anand and Selvaraj, 2009; Coussens et al., 2009), and several cytokines, for example IFN-y, TNF-α, IL-6, and IL-10 (Vidyarani et al., 2007; Harishankar et al., 2014) in vitro. However, its effect on radiological outcomes are ambiguous: three trials comparing vitamin D3 as adjunctive therapy demonstrated no effect on CXR score (Martineau et al., 2011; Ralph et al., 2013b; Mily et al., 2015) or pulmonary function (Ralph et al., 2013b), while one study found more CXR improvement in the vitamin-D3 treated group (Salahuddin et al., 2013).
Statins are widely used inhibitors of cholesterol biosynthesis. They induce autophagy in vitro (Parihar et al., 2014) with broad anti-inflammatory effects, although not directly demonstrated in TB (Hennessy et al., 2016). Their use has been associated with a reduced risk of developing active TB in some studies (Lai et al., 2016; Liao et al., 2017; Su et al., 2017), but not in all (Kang et al., 2014). No studies have been performed in humans assessing statins in relation to pulmonary damage in TB; in mice, statins have been found to reduce lung pathology (Parihar et al., 2014). A future study will look at the effect of pravastatin added to standard TB treatment on pulmonary function (NCT03456102).
Several studies looked at the effect of IFN-γ as adjunctive therapy for TB (Gao et al., 2011). The studies were small, and most were performed in patients with multi-drug resistant TB. Aerosolized IFN-γ in combination with TB treatment resulted in better CXR outcomes compared to TB treatment alone. This contradicts the finding in mice, where adding IFN-γ resulted in worse pulmonary outcomes (Sakai et al., 2016). The authors conclude that IFN-γ might be beneficial as adjunctive therapy in TB, but larger trials are needed to confirm this.
Mesenchymal stromal cells are tissue-resident non-hematopoietic adult progenitor cells. They are believed to facilitate organ homeostasis and tissue repair and can modulate immune responses; they have been used in treatment of graft-versus-host-disease and autoimmune diseases (Parida et al., 2015). In a phase 1 trial in patients with drug resistant TB, infusions of autologous mesenchymal stromal cells, 4 weeks after starting TB treatment, was safe and resulted in CXR improvement in 25/36 patients compared to 15/36 controls (Skrahin et al., 2016).
The immune mechanisms of parenchymal lung damage in human TB are complex and incompletely understood. The difference between pulmonary damage in animal models (mostly occurring as a result of primary TB) and humans (mostly occurring as a result of post-primary TB) further complicates study of this phenomenon. Processes taking place in the lung are heterogeneous, with granulomas with varying degrees of mycobacterial control existing next to each other and inflammatory cells and cytokines appearing to have different effects at different time points. MMPs seem to play an important role and consequently, inhibition of MMPs may lead to reduction in pulmonary damage, however, this remains to be proven in clinical trials. Neutrophils are another key mediator of pulmonary damage, whose recruitment could potentially be inhibited by NSAIDs. The role of other effectors is less clear and better insight into their effects over the course of TB infection and disease is needed to be able to guide potential intervention. Future studies of human TB and (host-directed) therapy should include radiographically assessed lung damage and pulmonary function as an outcome.
CS wrote the first draft of the manuscript. GM, BA, NW, RW, and LL critically revised the manuscript. All authors read and approved the submitted version.
RW was funded by The Wellcome Trust (104803 and 203135) and the Francis Crick Institute, which receives support from The Wellcome Trust (FC0010218); Research Councils United Kingdom (FC0010218); Cancer Research United Kingdom (FC0010218); National Institutes of Health (U01 A1 115940); The European & Developing Countries Clinical Trials Partnership (SRIA2015-1065). GM was supported by the Wellcome Trust (098316 and 203135/Z/16/Z), the South African Research Chairs Initiative of the Department of Science and Technology and National Research Foundation (NRF) of South Africa (Grant No. 64787), NRF incentive funding (UID: 85858) and the South African Medical Research Council through its TB and HIV Collaborating Centres Programme with funds received from the National Department of Health (RFA# SAMRC-RFA-CC: TB/HIV/AIDS-01-2014). The funders had no role in the writing of this review. The opinions, findings, and conclusion expressed in this manuscript reflect those of the authors alone.
The authors declare that the research was conducted in the absence of any commercial or financial relationships that could be construed as a potential conflict of interest.
Abakay, O., Abakay, A., Sen, H. S., and Tanrikulu, A. C. (2015). The relationship between inflammatory marker levels and pulmonary tuberculosis severity. Inflammation 38, 691–696. doi: 10.1007/s10753-014-9978-y
Akkara, S. A., Shah, A. D., Adalja, M., Akkara, A. G., Rathi, A., and Shah, D. N. (2013). Pulmonary tuberculosis: the day after. Int. J. Tuberc. Lung Dis. 17, 810–813. doi: 10.5588/ijtld.12.0317
Al Shammari, B., Shiomi, T., Tezera, L., Bielecka, M. K., Workman, V., Sathyamoorthy, T., et al. (2015). The extracellular matrix regulates granuloma necrosis in tuberculosis. J. Infect. Dis. 212, 463–473. doi: 10.1093/infdis/jiv076
Ameglio, F., Casarini, M., Capoluongo, E., Mattia, P., Puglisi, G., and Giosue, S. (2005). Post-treatment changes of six cytokines in active pulmonary tuberculosis: differences between patients with stable or increased fibrosis. Int. J. Tuberc. Lung Dis. 9, 98–104.
Anand, S. P., and Selvaraj, P. (2009). Effect of 1, 25 dihydroxyvitamin D(3) on matrix metalloproteinases MMP-7, MMP-9 and the inhibitor TIMP-1 in pulmonary tuberculosis. Clin. Immunol. 133, 126–131. doi: 10.1016/j.clim.2009.06.009
Arai, M., Uchiba, M., Komura, H., Mizuochi, Y., Harada, N., and Okajima, K. (2010). Metformin, an antidiabetic agent, suppresses the production of tumor necrosis factor and tissue factor by inhibiting early growth response factor-1 expression in human monocytes in vitro. J. Pharmacol. Exp. Ther. 334, 206–213. doi: 10.1124/jpet.109.164970
Baez-Saldana, R., Lopez-Arteaga, Y., Bizarron-Muro, A., Ferreira-Guerrero, E., Ferreyra-Reyes, L., Delgado-Sanchez, G., et al. (2013). A novel scoring system to measure radiographic abnormalities and related spirometric values in cured pulmonary tuberculosis. PLoS One 8:e78926. doi: 10.1371/journal.pone.0078926
Barnes, P. F., Leedom, J. M., Chan, L. S., Wong, S. F., Shah, J., Vachon, L. A., et al. (1988). Predictors of short-term prognosis in patients with pulmonary tuberculosis. J. Infect. Dis. 158, 366–371. doi: 10.1093/infdis/158.2.366
Barry, C. E. III, Boshoff, H. I., Dartois, V., Dick, T., Ehrt, S., Flynn, J., et al. (2009). The spectrum of latent tuberculosis: rethinking the biology and intervention strategies. Nat. Rev. Microbiol. 7, 845–855. doi: 10.1038/nrmicro2236
Bekker, L. G., Moreira, A. L., Bergtold, A., Freeman, S., Ryffel, B., and Kaplan, G. (2000). Immunopathologic effects of tumor necrosis factor alpha in murine mycobacterial infection are dose dependent. Infect. Immun. 68, 6954–6961. doi: 10.1128/IAI.68.12.6954-6961.2000
Belton, M., Brilha, S., Manavaki, R., Mauri, F., Nijran, K., Hong, Y. T., et al. (2016). Hypoxia and tissue destruction in pulmonary TB. Thorax 71, 1145–1153. doi: 10.1136/thoraxjnl-2015-207402
Berrocal-Almanza, L. C., Goyal, S., Hussain, A., Klassert, T. E., Driesch, D., Grozdanovic, Z., et al. (2016). S100A12 is up-regulated in pulmonary tuberculosis and predicts the extent of alveolar infiltration on chest radiography: an observational study. Sci. Rep. 6:31798. doi: 10.1038/srep31798
Berry, M. P., Graham, C. M., McNab, F. W., Xu, Z., Bloch, S. A., Oni, T., et al. (2010). An interferon-inducible neutrophil-driven blood transcriptional signature in human tuberculosis. Nature 466, 973–977. doi: 10.1038/nature09247
Blackmore, T. K., Manning, L., Taylor, W. J., and Wallis, R. S. (2008). Therapeutic use of infliximab in tuberculosis to control severe paradoxical reaction of the brain and lymph nodes. Clin. Infect. Dis. 47, e83–e85. doi: 10.1086/592695
Bongiovanni, B., Mata-Espinosa, D., D’Attilio, L., Leon-Contreras, J. C., Marquez-Velasco, R., Bottasso, O., et al. (2015). Effect of cortisol and/or DHEA on THP1-derived macrophages infected with Mycobacterium tuberculosis. Tuberculosis 95, 562–569. doi: 10.1016/j.tube.2015.05.011
Borthwick, L. A. (2016). The IL-1 cytokine family and its role in inflammation and fibrosis in the lung. Semin. Immunopathol. 38, 517–534. doi: 10.1007/s00281-016-0559-z
Campbell, G. R., and Spector, S. A. (2012). Vitamin D inhibits human immunodeficiency virus type 1 and Mycobacterium tuberculosis infection in macrophages through the induction of autophagy. PLoS Pathog. 8:e1002689. doi: 10.1371/journal.ppat.1002689
Canetti, G. (1955). The Tubercle Bacillus in the Pulmonary Lesion of Man. New York, NY: Springer Publishing.
Casarini, M., Ameglio, F., Alemanno, L., Zangrilli, P., Mattia, P., Paone, G., et al. (1999). Cytokine levels correlate with a radiologic score in active pulmonary tuberculosis. Am. J. Respir. Crit. Care Med. 159, 143–148. doi: 10.1164/ajrccm.159.1.9803066
Chen, M., Divangahi, M., Gan, H., Shin, D. S., Hong, S., Lee, D. M., et al. (2008). Lipid mediators in innate immunity against tuberculosis: opposing roles of PGE2 and LXA4 in the induction of macrophage death. J. Exp. Med. 205, 2791–2801. doi: 10.1084/jem.20080767
Chen, Y. C., Kuo, C. H., Tsai, Y. M., Lin, Y. C., Hsiao, H. P., Chen, B. H., et al. (2018). Suppressive effects of metformin on T-helper 1-related chemokines expression in the human monocytic leukemia cell line THP-1. Endocr. Res. doi: 10.1080/07435800.2018.1460605 [Epub ahead of print].
Chowdhury, I. H., Ahmed, A. M., Choudhuri, S., Sen, A., Hazra, A., Pal, N. K., et al. (2014). Alteration of serum inflammatory cytokines in active pulmonary tuberculosis following anti-tuberculosis drug therapy. Mol. Immunol. 62, 159–168. doi: 10.1016/j.molimm.2014.06.002
Chung, K. P., Chen, J. Y., Lee, C. H., Wu, H. D., Wang, J. Y., Lee, L. N., et al. (2011). Trends and predictors of changes in pulmonary function after treatment for pulmonary tuberculosis. Clinics 66, 549–556. doi: 10.1590/S1807-59322011000400005
Clayton, K., Polak, M. E., Woelk, C. H., and Elkington, P. (2017). Gene expression signatures in tuberculosis have greater overlap with autoimmune diseases than with infectious diseases. Am. J. Respir. Crit. Care Med. 196, 655–656. doi: 10.1164/rccm.201706-1248LE
Conesa-Botella, A., Meintjes, G., Coussens, A. K., van der Plas, H., Goliath, R., Schutz, C., et al. (2012). Corticosteroid therapy, vitamin D status, and inflammatory cytokine profile in the HIV-tuberculosis immune reconstitution inflammatory syndrome. Clin. Infect. Dis. 55, 1004–1011. doi: 10.1093/cid/cis577
Coussens, A., Timms, P. M., Boucher, B. J., Venton, T. R., Ashcroft, A. T., Skolimowska, K. H., et al. (2009). 1alpha,25-dihydroxyvitamin D3 inhibits matrix metalloproteinases induced by Mycobacterium tuberculosis infection. Immunology 127, 539–548. doi: 10.1111/j.1365-2567.2008.03024.x
Critchley, J. A., Orton, L. C., and Pearson, F. (2014). Adjunctive steroid therapy for managing pulmonary tuberculosis. Cochrane Database Syst. Rev. 11:CD011370. doi: 10.1002/14651858.CD011370
Critchley, J. A., Young, F., Orton, L., and Garner, P. (2013). Corticosteroids for prevention of mortality in people with tuberculosis: a systematic review and meta-analysis. Lancet Infect. Dis. 13, 223–237. doi: 10.1016/S1473-3099(12)70321-3
Dallenga, T., Linnemann, L., Paudyal, B., Repnik, U., Griffiths, G., and Schaible, U. E. (2017a). Targeting neutrophils for host-directed therapy to treat tuberculosis. Int. J. Med. Microbiol. doi: 10.1016/j.ijmm.2017.10.001 [Epub ahead of print].
Dallenga, T., Repnik, U., Corleis, B., Eich, J., Reimer, R., Griffiths, G. W., et al. (2017b). M. tuberculosis-induced necrosis of infected neutrophils promotes bacterial growth following phagocytosis by macrophages. Cell Host Microbe 22, 519–530.e3. doi: 10.1016/j.chom.2017.09.003
Dannenberg, A. M. Jr. (2006). Pathogenesis of Human Pulmonary Tuberculosis: Insights from the Rabbit Model. Washington, DC: ASM Press. doi: 10.1128/9781555815684
de Castro Cunha, R. M., Kallas, E. G., Rodrigues, D. S., Nascimento Burattini, M., and Salomao, R. (2005). Interferon-gamma and tumour necrosis factor-alpha production by CD4+ T and CD8+ T lymphocytes in AIDS patients with tuberculosis. Clin. Exp. Immunol. 140, 491–497. doi: 10.1111/j.1365-2249.2005.02796.x
de la Mora, I. L., Martinez-Oceguera, D., and Laniado-Laborin, R. (2015). Chronic airway obstruction after successful treatment of tuberculosis and its impact on quality of life. Int. J. Tuberc. Lung Dis. 19, 808–810. doi: 10.5588/ijtld.14.0983
de Valliere, S., and Barker, R. D. (2004). Residual lung damage after completion of treatment for multidrug-resistant tuberculosis. Int. J. Tuberc. Lung Dis. 8, 767–771.
Degner, N. R., Wang, J. Y., Golub, J. E., and Karakousis, P. C. (2018). Metformin use reverses the increased mortality associated with diabetes mellitus during tuberculosis treatment. Clin. Infect. Dis. 66, 198–205. doi: 10.1093/cid/cix819
Dietzold, J., Gopalakrishnan, A., and Salgame, P. (2015). Duality of lipid mediators in host response against Mycobacterium tuberculosis: good cop, bad cop. F1000Prime Rep. 7:29. doi: 10.12703/P7-29
Divangahi, M., Behar, S. M., and Remold, H. (2013). Dying to live: how the death modality of the infected macrophage affects immunity to tuberculosis. Adv. Exp. Med. Biol. 783, 103–120. doi: 10.1007/978-1-4614-6111-1_6
Dlugovitzky, D., Torres-Morales, A., Rateni, L., Farroni, M. A., Largacha, C., Molteni, O., et al. (1997). Circulating profile of Th1 and Th2 cytokines in tuberculosis patients with different degrees of pulmonary involvement. FEMS Immunol. Med. Microbiol. 18, 203–207. doi: 10.1111/j.1574-695X.1997.tb01046.x
Dooley, D. P., Carpenter, J. L., and Rademacher, S. (1997). Adjunctive corticosteroid therapy for tuberculosis: a critical reappraisal of the literature. Clin. Infect. Dis. 25, 872–887. doi: 10.1086/515543
el-Ahmady, O., Mansour, M., Zoeir, H., and Mansour, O. (1997). Elevated concentrations of interleukins and leukotriene in response to Mycobacterium tuberculosis infection. Ann. Clin. Biochem. 34(Pt 2), 160–164. doi: 10.1177/000456329703400205
Elkington, P., Shiomi, T., Breen, R., Nuttall, R. K., Ugarte-Gil, C. A., Walker, N. F., et al. (2011). MMP-1 drives immunopathology in human tuberculosis and transgenic mice. J. Clin. Invest. 121, 1827–1833. doi: 10.1172/JCI45666
Elkington, P. T., Ugarte-Gil, C. A., and Friedland, J. S. (2011). Matrix metalloproteinases in tuberculosis. Eur. Respir. J. 38, 456–464. doi: 10.1183/09031936.00015411
Elkington, P., Tebruegge, M., and Mansour, S. (2016). Tuberculosis: an infection-initiated autoimmune disease? Trends Immunol. 37, 815–818. doi: 10.1016/j.it.2016.09.007
Elkington, P. T., and Friedland, J. S. (2006). Matrix metalloproteinases in destructive pulmonary pathology. Thorax 61, 259–266. doi: 10.1136/thx.2005.051979
Elliott, A. M., Hurst, T. J., Balyeku, M. N., Quigley, M. A., Kaleebu, P., French, N., et al. (1999). The immune response to Mycobacterium tuberculosis in HIV-infected and uninfected adults in Uganda: application of a whole blood cytokine assay in an epidemiological study. Int. J. Tuberc. Lung Dis. 3, 239–247.
Esmail, H., Lai, R. P., Lesosky, M., Wilkinson, K. A., Graham, C. M., Coussens, A. K., et al. (2016). Characterization of progressive HIV-associated tuberculosis using 2-deoxy-2-[18F]fluoro-D-glucose positron emission and computed tomography. Nat. Med. 22, 1090–1093. doi: 10.1038/nm.4161
Eum, S. Y., Kong, J. H., Hong, M. S., Lee, Y. J., Kim, J. H., Hwang, S. H., et al. (2010). Neutrophils are the predominant infected phagocytic cells in the airways of patients with active pulmonary TB. Chest 137, 122–128. doi: 10.1378/chest.09-0903
Fan, L., Xiao, H., Mai, G., Su, B., Ernst, J., and Hu, Z. (2015). Impaired M. tuberculosis antigen-specific IFN-gamma response without IL-17 enhancement in patients with severe cavitary pulmonary tuberculosis. PLoS One 10:e0127087. doi: 10.1371/journal.pone.0127087
Flynn, J. (2018). Pathogenesis of Tuberculosis and Vaccine Prevention, CROI; Abstract Number 13. Pittsburgh, PA: University of Pittsburgh.
Flynn, J. L., Gideon, H. P., Mattila, J. T., and Lin, P. L. (2015). Immunology studies in non-human primate models of tuberculosis. Immunol. Rev. 264, 60–73. doi: 10.1111/imr.12258
Fox, K. A., Kirwan, D. E., Whittington, A. M., Krishnan, N., Robertson, B. D., Gilman, R. H., et al. (2018). Platelets regulate pulmonary inflammation and tissue destruction in tuberculosis. Am. J. Respir. Crit. Care Med. 198, 245–255. doi: 10.1164/rccm.201710-2102OC
Gallegos, A. M., Pamer, E. G., and Glickman, M. S. (2008). Delayed protection by ESAT-6-specific effector CD4+ T cells after airborne M. tuberculosis infection. J. Exp. Med. 205, 2359–2368. doi: 10.1084/jem.20080353
Gao, X. F., Yang, Z. W., and Li, J. (2011). Adjunctive therapy with interferon-gamma for the treatment of pulmonary tuberculosis: a systematic review. Int. J. Infect. Dis. 15, e594–e600. doi: 10.1016/j.ijid.2011.05.002
Giannandrea, M., and Parks, W. C. (2014). Diverse functions of matrix metalloproteinases during fibrosis. Dis. Model. Mech. 7, 193–203. doi: 10.1242/dmm.012062
Gopal, R., Monin, L., Torres, D., Slight, S., Mehra, S., McKenna, K. C., et al. (2013). S100A8/A9 proteins mediate neutrophilic inflammation and lung pathology during tuberculosis. Am. J. Respir. Crit. Care Med. 188, 1137–1146. doi: 10.1164/rccm.201304-0803OC
Guan, W. J., Gao, Y. H., Xu, G., Lin, Z. Y., Tang, Y., Gu, Y. Y., et al. (2015). Sputum matrix metalloproteinase-8 and -9 and tissue inhibitor of metalloproteinase-1 in bronchiectasis: clinical correlates and prognostic implications. Respirology 20, 1073–1081. doi: 10.1111/resp.12582
Gupta, A., Misra, A., and Deretic, V. (2016). Targeted pulmonary delivery of inducers of host macrophage autophagy as a potential host-directed chemotherapy of tuberculosis. Adv. Drug Deliv. Rev. 102, 10–20. doi: 10.1016/j.addr.2016.01.016
Gupte, A. N., Wong, M. L., Msandiwa, R., Barnes, G. L., Golub, J., Chaisson, R. E., et al. (2017). Factors associated with pulmonary impairment in HIV-infected South African adults. PLoS One 12:e0184530. doi: 10.1371/journal.pone.0184530
Gutierrez, M. G., Master, S. S., Singh, S. B., Taylor, G. A., Colombo, M. I., and Deretic, V. (2004). Autophagy is a defense mechanism inhibiting BCG and Mycobacterium tuberculosis survival in infected macrophages. Cell 119, 753–766. doi: 10.1016/j.cell.2004.11.038
Harishankar, M., Afsal, K., Banurekha, V. V., Meenakshi, N., and Selvaraj, P. (2014). 1,25-Dihydroxy vitamin D3 downregulates pro-inflammatory cytokine response in pulmonary tuberculosis. Int. Immunopharmacol. 23, 148–152. doi: 10.1016/j.intimp.2014.08.021
Hawn, T. R., Matheson, A. I., Maley, S. N., and Vandal, O. (2013). Host-directed therapeutics for tuberculosis: can we harness the host? Microbiol. Mol. Biol. Rev. 77, 608–627. doi: 10.1128/MMBR.00032-13
Hennessy, E., Adams, C., Reen, F. J., and O’Gara, F. (2016). Is there potential for repurposing statins as novel antimicrobials? Antimicrob. Agents Chemother. 60, 5111–5121. doi: 10.1128/AAC.00192-16
Horsfall, P. A., Plummer, J., Allan, W. G., Girling, D. J., Nunn, A. J., and Fox, W. (1979). Double blind controlled comparison of aspirin, allopurinol and placebo in the management of arthralgia during pyrazinamide administration. Tubercle 60, 13–24. doi: 10.1016/0041-3879(79)90051-5
Hrabec, E., Strek, M., Zieba, M., Kwiatkowska, S., and Hrabec, Z. (2002). Circulation level of matrix metalloproteinase-9 is correlated with disease severity in tuberculosis patients. Int. J. Tuberc. Lung Dis. 6, 713–719.
Hsu, D. C., Faldetta, K. F., Pei, L., Sheikh, V., Utay, N. S., Roby, G., et al. (2016). A paradoxical treatment for a paradoxical condition: infliximab use in three cases of mycobacterial IRIS. Clin. Infect. Dis. 62, 258–261. doi: 10.1093/cid/civ841
Hunter, R. L. (2011). Pathology of post primary tuberculosis of the lung: an illustrated critical review. Tuberculosis 91, 497–509. doi: 10.1016/j.tube.2011.03.007
Hunter, R. L. (2016). Tuberculosis as a three-act play: a new paradigm for the pathogenesis of pulmonary tuberculosis. Tuberculosis 97, 8–17. doi: 10.1016/j.tube.2015.11.010
Jick, S. S., Lieberman, E. S., Rahman, M. U., and Choi, H. K. (2006). Glucocorticoid use, other associated factors, and the risk of tuberculosis. Arthritis Rheum. 55, 19–26. doi: 10.1002/art.21705
Jordan, T. S., Spencer, E. M., and Davies, P. (2010). Tuberculosis, bronchiectasis and chronic airflow obstruction. Respirology 15, 623–628. doi: 10.1111/j.1440-1843.2010.01749.x
Jorge, J. H., Graciela, C., Pablo, A. P., and Luis, S. H. (2012). A life-threatening central nervous system-tuberculosis inflammatory reaction nonresponsive to corticosteroids and successfully controlled by infliximab in a young patient with a variant of juvenile idiopathic arthritis. J. Clin. Rheumatol. 18, 189–191. doi: 10.1097/RHU.0b013e318258b725
Kang, Y. A., Choi, N. K., Seong, J. M., Heo, E. Y., Koo, B. K., Hwang, S. S., et al. (2014). The effects of statin use on the development of tuberculosis among patients with diabetes mellitus. Int. J. Tuberc. Lung Dis. 18, 717–724. doi: 10.5588/ijtld.13.0854
Kassa, D., de Jager, W., Gebremichael, G., Alemayehu, Y., Ran, L., Fransen, J., et al. (2016). The effect of HIV coinfection, HAART and TB treatment on cytokine/chemokine responses to Mycobacterium tuberculosis (Mtb) antigens in active TB patients and latently Mtb infected individuals. Tuberculosis 96, 131–140. doi: 10.1016/j.tube.2015.05.015
Kim, J. J., Lee, H. M., Shin, D. M., Kim, W., Yuk, J. M., Jin, H. S., et al. (2012). Host cell autophagy activated by antibiotics is required for their effective antimycobacterial drug action. Cell Host Microbe 11, 457–468. doi: 10.1016/j.chom.2012.03.008
King, P. T. (2009). The pathophysiology of bronchiectasis. Int. J. Chron. Obstruct. Pulmon. Dis. 4, 411–419. doi: 10.2147/COPD.S6133
Kubler, A., Luna, B., Larsson, C., Ammerman, N. C., Andrade, B. B., Orandle, M., et al. (2015). Mycobacterium tuberculosis dysregulates MMP/TIMP balance to drive rapid cavitation and unrestrained bacterial proliferation. J. Pathol. 235, 431–444. doi: 10.1002/path.4432
Kuhne, W., and Willgeroth, C. (1988). [The significance of tuberculosis today and its pathomorphism, demonstrated in postmortem material]. Zentralbl. Allg. Pathol. 134, 665–669.
Kwan, C. K., and Ernst, J. D. (2011). HIV and tuberculosis: a deadly human syndemic. Clin. Microbiol. Rev. 24, 351–376. doi: 10.1128/CMR.00042-10
Lai, C. C., Lee, M. T., Lee, S. H., Hsu, W. T., Chang, S. S., Chen, S. C., et al. (2016). Statin treatment is associated with a decreased risk of active tuberculosis: an analysis of a nationally representative cohort. Thorax 71, 646–651. doi: 10.1136/thoraxjnl-2015-207052
Lai, R. P., Meintjes, G., Wilkinson, K. A., Graham, C. M., Marais, S., Van der Plas, H., et al. (2015). HIV-tuberculosis-associated immune reconstitution inflammatory syndrome is characterized by Toll-like receptor and inflammasome signalling. Nat. Commun. 6:8451. doi: 10.1038/ncomms9451
Lammermann, T., Afonso, P. V., Angermann, B. R., Wang, J. M., Kastenmuller, W., Parent, C. A., et al. (2013). Neutrophil swarms require LTB4 and integrins at sites of cell death in vivo. Nature 498, 371–375. doi: 10.1038/nature12175
Lee, H. S., Lee, Y., Lee, S. O., Choi, S. H., Kim, Y. S., Woo, J. H., et al. (2012). Adalimumab treatment may replace or enhance the activity of steroids in steroid-refractory tuberculous meningitis. J. Infect. Chemother. 18, 555–557. doi: 10.1007/s10156-011-0334-y
Lee, J. Y., Jung, Y. W., Jeong, I., Joh, J. S., Sim, S. Y., Choi, B., et al. (2015). Immune parameters differentiating active from latent tuberculosis infection in humans. Tuberculosis 95, 758–763. doi: 10.1016/j.tube.2015.08.003
Li, F., Gao, B., Xu, W., Chen, L., and Xiong, S. (2016). The defect in autophagy induction by clinical isolates of Mycobacterium tuberculosis is correlated with poor tuberculosis outcomes. PLoS One 11:e0147810. doi: 10.1371/journal.pone.0147810
Liao, K. F., Lin, C. L., and Lai, S. W. (2017). Population-based case-control study assessing the association between statins use and pulmonary tuberculosis in Taiwan. Front. Pharmacol. 8:597. doi: 10.3389/fphar.2017.00597
Long, R., Maycher, B., Dhar, A., Manfreda, J., Hershfield, E., and Anthonisen, N. (1998). Pulmonary tuberculosis treated with directly observed therapy: serial changes in lung structure and function. Chest 113, 933–943. doi: 10.1378/chest.113.4.933
Lowe, D. M., Bandara, A. K., Packe, G. E., Barker, R. D., Wilkinson, R. J., Griffiths, C. J., et al. (2013). Neutrophilia independently predicts death in tuberculosis. Eur. Respir. J. 42, 1752–1757. doi: 10.1183/09031936.00140913
Lowe, D. M., Bangani, N., Goliath, R., Kampmann, B., Wilkinson, K. A., Wilkinson, R. J., et al. (2015). Effect of antiretroviral therapy on HIV-mediated impairment of the neutrophil antimycobacterial response. Ann. Am. Thorac. Soc. 12, 1627–1637. doi: 10.1513/AnnalsATS.201507-463OC
Lowe, D. M., Redford, P. S., Wilkinson, R. J., O’Garra, A., and Martineau, A. R. (2012). Neutrophils in tuberculosis: friend or foe? Trends Immunol. 33, 14–25. doi: 10.1016/j.it.2011.10.003
Mahuad, C., Bay, M. L., Farroni, M. A., Bozza, V., Del Rey, A., Besedovsky, H., et al. (2004). Cortisol and dehydroepiandrosterone affect the response of peripheral blood mononuclear cells to mycobacterial antigens during tuberculosis. Scand. J. Immunol. 60, 639–646. doi: 10.1111/j.0300-9475.2004.01514.x
Mai, N. T., Dobbs, N., Phu, N. H., Colas, R. A., Thao, L. T., Thuong, N. T., et al. (2018). A randomised double blind placebo controlled phase 2 trial of adjunctive aspirin for tuberculous meningitis in HIV-uninfected adults. eLife 7:e33478. doi: 10.7554/eLife.33478
Maiga, M. C., Ahidjo, B. A., Maiga, M., and Bishai, W. R. (2015). Roflumilast, a type 4 phosphodiesterase inhibitor, shows promising adjunctive, host-directed therapeutic activity in a mouse model of tuberculosis. Antimicrob. Agents Chemother. 59, 7888–7890. doi: 10.1128/AAC.02145-15
Malherbe, S. T., Shenai, S., Ronacher, K., Loxton, A. G., Dolganov, G., Kriel, M., et al. (2016). Persisting positron emission tomography lesion activity and Mycobacterium tuberculosis mRNA after tuberculosis cure. Nat. Med. 22, 1094–1100. doi: 10.1038/nm.4177
Manca, C., Koo, M. S., Peixoto, B., Fallows, D., Kaplan, G., and Subbian, S. (2013). Host targeted activity of pyrazinamide in Mycobacterium tuberculosis infection. PLoS One 8:e74082. doi: 10.1371/journal.pone.0074082
Manji, M., Shayo, G., Mamuya, S., Mpembeni, R., Jusabani, A., and Mugusi, F. (2016). Lung functions among patients with pulmonary tuberculosis in Dar es Salaam - a cross-sectional study. BMC Pulm. Med. 16:58. doi: 10.1186/s12890-016-0213-5
Marakalala, M. J., Raju, R. M., Sharma, K., Zhang, Y. J., Eugenin, E. A., Prideaux, B., et al. (2016). Inflammatory signaling in human tuberculosis granulomas is spatially organized. Nat. Med. 22, 531–538. doi: 10.1038/nm.4073
Martineau, A. R., Newton, S. M., Wilkinson, K. A., Kampmann, B., Hall, B. M., Nawroly, N., et al. (2007). Neutrophil-mediated innate immune resistance to mycobacteria. J. Clin. Invest. 117, 1988–1994. doi: 10.1172/JCI31097
Martineau, A. R., Timms, P. M., Bothamley, G. H., Hanifa, Y., Islam, K., Claxton, A. P., et al. (2011). High-dose vitamin D(3) during intensive-phase antimicrobial treatment of pulmonary tuberculosis: a double-blind randomised controlled trial. Lancet 377, 242–250. doi: 10.1016/S0140-6736(10)61889-2
Martinez, V., Castilla-Lievre, M. A., Guillet-Caruba, C., Grenier, G., Fior, R., Desarnaud, S., et al. (2012). (18)F-FDG PET/CT in tuberculosis: an early non-invasive marker of therapeutic response. Int. J. Tuberc. Lung Dis. 16, 1180–1185. doi: 10.5588/ijtld.12.0010
Masure, S., Proost, P., Van Damme, J., and Opdenakker, G. (1991). Purification and identification of 91-kDa neutrophil gelatinase. Release by the activating peptide interleukin-8. Eur. J. Biochem. 198, 391–398. doi: 10.1111/j.1432-1033.1991.tb16027.x
Mayanja-Kizza, H., Jones-Lopez, E., Okwera, A., Wallis, R. S., Ellner, J. J., Mugerwa, R. D., et al. (2005). Immunoadjuvant prednisolone therapy for HIV-associated tuberculosis: a phase 2 clinical trial in Uganda. J. Infect. Dis. 191, 856–865. doi: 10.1086/427995
Mayer-Barber, K. D., Andrade, B. B., Oland, S. D., Amaral, E. P., Barber, D. L., Gonzales, J., et al. (2014). Host-directed therapy of tuberculosis based on interleukin-1 and type I interferon crosstalk. Nature 511, 99–103. doi: 10.1038/nature13489
Mazzarella, G., Bianco, A., Perna, F., D’Auria, D., Grella, E., Moscariello, E., et al. (2003). T lymphocyte phenotypic profile in lung segments affected by cavitary and non-cavitary tuberculosis. Clin. Exp. Immunol. 132, 283–288. doi: 10.1046/j.1365-2249.2003.02121.x
Meghji, J., Simpson, H., Squire, S. B., and Mortimer, K. (2016). A systematic review of the prevalence and pattern of imaging defined post-TB lung disease. PLoS One 11:e0161176. doi: 10.1371/journal.pone.0161176
Meintjes, G., Skolimowska, K. H., Wilkinson, K. A., Matthews, K., Tadokera, R., Conesa-Botella, A., et al. (2012). Corticosteroid-modulated immune activation in the tuberculosis immune reconstitution inflammatory syndrome. Am. J. Respir. Crit. Care Med. 186, 369–377. doi: 10.1164/rccm.201201-0094OC
Meintjes, G., Stek, C., Blumenthal, L., Thienemann, F., Schutz, C., Buyze, J., et al. (2017). Randomized Controlled Trial of Prednisone for the Prevention of TB-IRIS, CROI; Abstract Number 81LB. Cape Town: University of Cape Town.
Mercalli, A., Calavita, I., Dugnani, E., Citro, A., Cantarelli, E., Nano, R., et al. (2013). Rapamycin unbalances the polarization of human macrophages to M1. Immunology 140, 179–190. doi: 10.1111/imm.12126
Mesquita, E. D., Gil-Santana, L., Ramalho, D., Tonomura, E., Silva, E. C., Oliveira, M. M., et al. (2016). Associations between systemic inflammation, mycobacterial loads in sputum and radiological improvement after treatment initiation in pulmonary TB patients from Brazil: a prospective cohort study. BMC Infect. Dis. 16:368. doi: 10.1186/s12879-016-1736-3
Mihret, A., Abebe, M., Bekele, Y., Aseffa, A., Walzl, G., and Howe, R. (2014). Impact of HIV co-infection on plasma level of cytokines and chemokines of pulmonary tuberculosis patients. BMC Infect. Dis. 14:125. doi: 10.1186/1471-2334-14-125
Milliron, B., Henry, T. S., Veeraraghavan, S., and Little, B. P. (2015). Bronchiectasis: mechanisms and imaging clues of associated common and uncommon diseases. Radiographics 35, 1011–1030. doi: 10.1148/rg.2015140214
Mily, A., Rekha, R. S., Kamal, S. M., Arifuzzaman, A. S., Rahim, Z., Khan, L., et al. (2015). Significant effects of oral phenylbutyrate and vitamin D3 adjunctive therapy in pulmonary tuberculosis: a randomized controlled trial. PLoS One 10:e0138340. doi: 10.1371/journal.pone.0138340
Mishra, B. B., Lovewell, R. R., Olive, A. J., Zhang, G., Wang, W., Eugenin, E., et al. (2017). Nitric oxide prevents a pathogen-permissive granulocytic inflammation during tuberculosis. Nat. Microbiol. 2:17072. doi: 10.1038/nmicrobiol.2017.72
Mishra, B. B., Rathinam, V. A., Martens, G. W., Martinot, A. J., Kornfeld, H., Fitzgerald, K. A., et al. (2013). Nitric oxide controls the immunopathology of tuberculosis by inhibiting NLRP3 inflammasome-dependent processing of IL-1beta. Nat. Immunol. 14, 52–60. doi: 10.1038/ni.2474
Misra, U. K., Kalita, J., and Nair, P. P. (2010). Role of aspirin in tuberculous meningitis: a randomized open label placebo controlled trial. J. Neurol. Sci. 293, 12–17. doi: 10.1016/j.jns.2010.03.025
Nachiappan, A. C., Rahbar, K., Shi, X., Guy, E. S., Mortani Barbosa, E. J. Jr., Shroff, G. S., et al. (2017). Pulmonary tuberculosis: role of radiology in diagnosis and management. Radiographics 37, 52–72. doi: 10.1148/rg.2017160032
Nadella, V., Mohanty, A., Sharma, L., Yellaboina, S., Mollenkopf, H. J., Mazumdar, V. B., et al. (2017). Inhibitors of apoptosis protein antagonists (Smac mimetic compounds) control polarization of macrophages during microbial challenge and sterile inflammatory responses. Front. Immunol. 8:1792. doi: 10.3389/fimmu.2017.01792
Nakiwala, J. K., Walker, N. F., Diedrich, C. R., Worodria, W., Meintjes, G., Wilkinson, R. J., et al. (2018). Neutrophil activation and enhanced release of granule products in HIV-TB immune reconstitution inflammatory syndrome. J. Acquir. Immune Defic. Syndr. 77, 221–229. doi: 10.1097/QAI.0000000000001582
Namale, P. E., Abdullahi, L. H., Fine, S., Kamkuemah, M., Wilkinson, R. J., and Meintjes, G. (2015). Paradoxical TB-IRIS in HIV-infected adults: a systematic review and meta-analysis. Future Microbiol. 10, 1077–1099. doi: 10.2217/fmb.15.9
Nandi, B., and Behar, S. M. (2011). Regulation of neutrophils by interferon-gamma limits lung inflammation during tuberculosis infection. J. Exp. Med. 208, 2251–2262. doi: 10.1084/jem.20110919
Narendran, G., Kavitha, D., Karunaianantham, R., Gil-Santana, L., Almeida-Junior, J. L., Reddy, S. D., et al. (2016). Role of LTA4H polymorphism in tuberculosis-associated immune reconstitution inflammatory syndrome occurrence and clinical severity in patients infected with HIV. PLoS One 11:e0163298. doi: 10.1371/journal.pone.0163298
Ndlovu, H., and Marakalala, M. J. (2016). Granulomas and inflammation: host-directed therapies for tuberculosis. Front. Immunol. 7:434. doi: 10.3389/fimmu.2016.00434
Nihues Sde, S., Mancuzo, E. V., Sulmonetti, N., Sacchi, F. P., Viana Vde, S., Netto, E. M., et al. (2015). Chronic symptoms and pulmonary dysfunction in post-tuberculosis Brazilian patients. Braz. J. Infect. Dis. 19, 492–497. doi: 10.1016/j.bjid.2015.06.005
Nolan, A., Condos, R., Huie, M. L., Dawson, R., Dheda, K., Bateman, E., et al. (2013). Elevated IP-10 and IL-6 from bronchoalveolar lavage cells are biomarkers of non-cavitary tuberculosis. Int. J. Tuberc. Lung Dis. 17, 922–927. doi: 10.5588/ijtld.12.0610
O’Garra, A., Redford, P. S., McNab, F. W., Bloom, C. I., Wilkinson, R. J., and Berry, M. P. (2013). The immune response in tuberculosis. Annu. Rev. Immunol. 31, 475–527. doi: 10.1146/annurev-immunol-032712-095939
Ong, C. W., Elkington, P. T., Brilha, S., Ugarte-Gil, C., Tome-Esteban, M. T., Tezera, L. B., et al. (2015). Neutrophil-derived MMP-8 drives AMPK-dependent matrix destruction in human pulmonary tuberculosis. PLoS Pathog. 11:e1004917. doi: 10.1371/journal.ppat.1004917
Ong, C. W., Elkington, P. T., and Friedland, J. S. (2014). Tuberculosis, pulmonary cavitation, and matrix metalloproteinases. Am. J. Respir. Crit. Care Med. 190, 9–18. doi: 10.1164/rccm.201311-2106PP
Ozguler, Y., Hatemi, G., Ugurlu, S., Seyahi, E., Melikoglu, M., Borekci, S., et al. (2016). Re-initiation of biologics after the development of tuberculosis under anti-TNF therapy. Rheumatol. Int. 36, 1719–1725. doi: 10.1007/s00296-016-3575-3
Panteleev, A. V., Nikitina, I. Y., Burmistrova, I. A., Kosmiadi, G. A., Radaeva, T. V., Amansahedov, R. B., et al. (2017). Severe tuberculosis in humans correlates best with neutrophil abundance and lymphocyte deficiency and does not correlate with antigen-specific CD4 T-cell response. Front. Immunol. 8:963. doi: 10.3389/fimmu.2017.00963
Parasa, V. R., Muvva, J. R., Rose, J. F., Braian, C., Brighenti, S., and Lerm, M. (2017). Inhibition of tissue matrix metalloproteinases interferes with Mycobacterium tuberculosis-induced granuloma formation and reduces bacterial load in a human lung tissue model. Front. Microbiol. 8:2370. doi: 10.3389/fmicb.2017.02370
Parida, S. K., Madansein, R., Singh, N., Padayatchi, N., Master, I., Naidu, K., et al. (2015). Cellular therapy in tuberculosis. Int. J. Infect. Dis. 32, 32–38. doi: 10.1016/j.ijid.2015.01.016
Parihar, S. P., Guler, R., Khutlang, R., Lang, D. M., Hurdayal, R., Mhlanga, M. M., et al. (2014). Statin therapy reduces the Mycobacterium tuberculosis burden in human macrophages and in mice by enhancing autophagy and phagosome maturation. J. Infect. Dis. 209, 754–763. doi: 10.1093/infdis/jit550
Pefura-Yone, E. W., Fodjeu, G., Kengne, A. P., Roche, N., and Kuaban, C. (2015). Prevalence and determinants of chronic obstructive pulmonary disease in HIV infected patients in an African country with low level of tobacco smoking. Respir. Med. 109, 247–254. doi: 10.1016/j.rmed.2014.12.003
Petty, T. L., and Dalrymple, G. V. (1964). Inhibition of pyrazinamide hyperuricemia by small doses of acetylsalicylic acid. Ann. Intern. Med. 60, 898–900. doi: 10.7326/0003-4819-60-5-898
Plit, M. L., Anderson, R., Van Rensburg, C. E., Page-Shipp, L., Blott, J. A., Fresen, J. L., et al. (1998). Influence of antimicrobial chemotherapy on spirometric parameters and pro-inflammatory indices in severe pulmonary tuberculosis. Eur. Respir. J. 12, 351–356. doi: 10.1183/09031936.98.12020351
Price, N. M., Farrar, J., Tran, T. T., Nguyen, T. H., Tran, T. H., and Friedland, J. S. (2001). Identification of a matrix-degrading phenotype in human tuberculosis in vitro and in vivo. J. Immunol. 166, 4223–4230. doi: 10.4049/jimmunol.166.6.4223
Radovic, M., Ristic, L., Ciric, Z., Dinic-Radovic, V., Stankovic, I., Pejcic, T., et al. (2016). Changes in respiratory function impairment following the treatment of severe pulmonary tuberculosis - limitations for the underlying COPD detection. Int. J. Chron. Obstruct. Pulmon. Dis. 11, 1307–1316. doi: 10.2147/COPD.S106875
Ralph, A. P., Kenangalem, E., Waramori, G., Pontororing, G. J., Sandjaja, Tjitra, E., et al. (2013a). High morbidity during treatment and residual pulmonary disability in pulmonary tuberculosis: under-recognised phenomena. PLoS One 8:e80302. doi: 10.1371/journal.pone.0080302
Ralph, A. P., Waramori, G., Pontororing, G. J., Kenangalem, E., Wiguna, A., Tjitra, E., et al. (2013b). L-arginine and vitamin D adjunctive therapies in pulmonary tuberculosis: a randomised, double-blind, placebo-controlled trial. PLoS One 8:e70032. doi: 10.1371/journal.pone.0070032
Rand, L., Green, J. A., Saraiva, L., Friedland, J. S., and Elkington, P. T. (2009). Matrix metalloproteinase-1 is regulated in tuberculosis by a p38 MAPK-dependent, p-aminosalicylic acid-sensitive signaling cascade. J. Immunol. 182, 5865–5872. doi: 10.4049/jimmunol.0801935
Rangel Moreno, J., Estrada Garcia, I., De La Luz Garcia Hernandez, M., Aguilar Leon, D., Marquez, R., and Hernandez Pando, R. (2002). The role of prostaglandin E2 in the immunopathogenesis of experimental pulmonary tuberculosis. Immunology 106, 257–266. doi: 10.1046/j.1365-2567.2002.01403.x
Rathinam, V. A., Vanaja, S. K., and Fitzgerald, K. A. (2012). Regulation of inflammasome signaling. Nat. Immunol. 13, 333–342. doi: 10.1038/ni.2237
Ravimohan, S., Tamuhla, N., Kung, S. J., Nfanyana, K., Steenhoff, A. P., Gross, R., et al. (2016). Matrix metalloproteinases in tuberculosis-immune reconstitution inflammatory syndrome and impaired lung function among advanced HIV/TB co-infected patients initiating antiretroviral therapy. EBioMedicine 3, 100–107. doi: 10.1016/j.ebiom.2015.11.040
Ravimohan, S., Tamuhla, N., Steenhoff, A. P., Letlhogile, R., Nfanyana, K., Bellamy, S. L., et al. (2015). Immunological profiling of tuberculosis-associated immune reconstitution inflammatory syndrome and non-immune reconstitution inflammatory syndrome death in HIV-infected adults with pulmonary tuberculosis starting antiretroviral therapy: a prospective observational cohort study. Lancet Infect. Dis. 15, 429–438. doi: 10.1016/S1473-3099(15)70008-3
Riou, C., Perez Peixoto, B., Roberts, L., Ronacher, K., Walzl, G., Manca, C., et al. (2012). Effect of standard tuberculosis treatment on plasma cytokine levels in patients with active pulmonary tuberculosis. PLoS One 7:e36886. doi: 10.1371/journal.pone.0036886
Sakai, S., Kauffman, K. D., Sallin, M. A., Sharpe, A. H., Young, H. A., Ganusov, V. V., et al. (2016). CD4 T cell-derived IFN-gamma plays a minimal role in control of pulmonary Mycobacterium tuberculosis infection and must be actively repressed by PD-1 to prevent lethal disease. PLoS Pathog. 12:e1005667. doi: 10.1371/journal.ppat.1005667
Sakamoto, K., Kim, M. J., Rhoades, E. R., Allavena, R. E., Ehrt, S., Wainwright, H. C., et al. (2013). Mycobacterial trehalose dimycolate reprograms macrophage global gene expression and activates matrix metalloproteinases. Infect. Immun. 81, 764–776. doi: 10.1128/IAI.00906-12
Salahuddin, N., Ali, F., Hasan, Z., Rao, N., Aqeel, M., and Mahmood, F. (2013). Vitamin D accelerates clinical recovery from tuberculosis: results of the SUCCINCT Study [Supplementary Cholecalciferol in recovery from tuberculosis]. A randomized, placebo-controlled, clinical trial of vitamin D supplementation in patients with pulmonary tuberculosis’. BMC Infect. Dis. 13:22. doi: 10.1186/1471-2334-13-22
Samperiz, G., Guerrero, D., Lopez, M., Valera, J. L., Iglesias, A., Rios, A., et al. (2014). Prevalence of and risk factors for pulmonary abnormalities in HIV-infected patients treated with antiretroviral therapy. HIV Med. 15, 321–329. doi: 10.1111/hiv.12117
Sathyamoorthy, T., Tezera, L. B., Walker, N. F., Brilha, S., Saraiva, L., Mauri, F. A., et al. (2015). Membrane type 1 matrix metalloproteinase regulates monocyte migration and collagen destruction in tuberculosis. J. Immunol. 195, 882–891. doi: 10.4049/jimmunol.1403110
Schoeman, J. F., Janse van Rensburg, A., Laubscher, J. A., and Springer, P. (2011). The role of aspirin in childhood tuberculous meningitis. J. Child Neurol. 26, 956–962. doi: 10.1177/0883073811398132
Schutz, C., Davis, A. G., Sossen, B., Lai, R. P., Ntsekhe, M., Harley, Y. X., et al. (2018). Corticosteroids as an adjunct to tuberculosis therapy. Expert. Rev. Respir. Med. 12, 881–891. doi: 10.1080/17476348.2018.1515628
Scriba, T. J., Penn-Nicholson, A., Shankar, S., Hraha, T., Thompson, E. G., Sterling, D., et al. (2017). Sequential inflammatory processes define human progression from M. tuberculosis infection to tuberculosis disease. PLoS Pathog. 13:e1006687. doi: 10.1371/journal.ppat.1006687
Seddon, J., Kasprowicz, V., Walker, N. F., Yuen, H. M., Sunpath, H., Tezera, L., et al. (2013). Procollagen III N-terminal propeptide and desmosine are released by matrix destruction in pulmonary tuberculosis. J. Infect. Dis. 208, 1571–1579. doi: 10.1093/infdis/jit343
Sepper, R., Konttinen, Y. T., Ding, Y., Takagi, M., and Sorsa, T. (1995). Human neutrophil collagenase (MMP-8), identified in bronchiectasis BAL fluid, correlates with severity of disease. Chest 107, 1641–1647. doi: 10.1378/chest.107.6.1641
Shu, C. C., Wu, M. F., Hsu, C. L., Huang, C. T., Wang, J. Y., Hsieh, S. L., et al. (2013). Apoptosis-associated biomarkers in tuberculosis: promising for diagnosis and prognosis prediction. BMC Infect. Dis. 13:45. doi: 10.1186/1471-2334-13-45
Sigal, G. B., Segal, M. R., Mathew, A., Jarlsberg, L., Wang, M., Barbero, S., et al. (2017). Biomarkers of tuberculosis severity and treatment effect: a directed screen of 70 host markers in a randomized clinical trial. EBioMedicine 25, 112–121. doi: 10.1016/j.ebiom.2017.10.018
Singh, S., Kubler, A., Singh, U. K., Singh, A., Gardiner, H., Prasad, R., et al. (2014a). Antimycobacterial drugs modulate immunopathogenic matrix metalloproteinases in a cellular model of pulmonary tuberculosis. Antimicrob. Agents Chemother. 58, 4657–4665. doi: 10.1128/AAC.02141-13
Singh, S., Saraiva, L., Elkington, P. T., and Friedland, J. S. (2014b). Regulation of matrix metalloproteinase-1, -3, and -9 in Mycobacterium tuberculosis-dependent respiratory networks by the rapamycin-sensitive PI3K/p70(S6K) cascade. FASEB J. 28, 85–93. doi: 10.1096/fj.13-235507
Singh, S., Maniakis-Grivas, G., Singh, U. K., Asher, R. M., Mauri, F., Elkington, P. T., et al. (2018). Interleukin-17 regulates matrix metalloproteinase activity in human pulmonary tuberculosis. J. Pathol. 244, 311–322. doi: 10.1002/path.5013
Singhal, A., Jie, L., Kumar, P., Hong, G. S., Leow, M. K., Paleja, B., et al. (2014). Metformin as adjunct antituberculosis therapy. Sci. Transl. Med. 6:263ra159. doi: 10.1126/scitranslmed.3009885
Skoura, E., Zumla, A., and Bomanji, J. (2015). Imaging in tuberculosis. Int. J. Infect. Dis. 32, 87–93. doi: 10.1016/j.ijid.2014.12.007
Skrahin, A., Jenkins, H. E., Hurevich, H., Solodovnikova, V., Isaikina, Y., Klimuk, D., et al. (2016). Effectiveness of a novel cellular therapy to treat multidrug-resistant tuberculosis. J. Clin. Tuberc. Other Mycobact. Dis. 4, 21–27. doi: 10.1016/j.jctube.2016.05.003
Smego, R. A., and Ahmed, N. (2003). A systematic review of the adjunctive use of systemic corticosteroids for pulmonary tuberculosis. Int. J. Tuberc. Lung Dis. 7, 208–213.
Sodhi, A., Gong, J., Silva, C., Qian, D., and Barnes, P. F. (1997). Clinical correlates of interferon gamma production in patients with tuberculosis. Clin. Infect. Dis. 25, 617–620. doi: 10.1086/513769
Songane, M., Kleinnijenhuis, J., Netea, M. G., and van Crevel, R. (2012). The role of autophagy in host defence against Mycobacterium tuberculosis infection. Tuberculosis 92, 388–396. doi: 10.1016/j.tube.2012.05.004
Su, V. Y., Su, W. J., Yen, Y. F., Pan, S. W., Chuang, P. H., Feng, J. Y., et al. (2017). Statin use is associated with a lower risk of TB. Chest 152, 598–606. doi: 10.1016/j.chest.2017.04.170
Su, W. L., Perng, W. C., Huang, C. H., Yang, C. Y., Wu, C. P., and Chen, J. H. (2010). Association of reduced tumor necrosis factor alpha, gamma interferon, and interleukin-1beta (IL-1beta) but increased IL-10 expression with improved chest radiography in patients with pulmonary tuberculosis. Clin. Vaccine Immunol. 17, 223–231. doi: 10.1128/CVI.00381-09
Subbian, S., Tsenova, L., Kim, M. J., Wainwright, H. C., Visser, A., Bandyopadhyay, N., et al. (2015). Lesion-specific immune response in granulomas of patients with pulmonary tuberculosis: a pilot study. PLoS One 10:e0132249. doi: 10.1371/journal.pone.0132249
Subbian, S., Tsenova, L., O’Brien, P., Yang, G., Koo, M. S., Peixoto, B., et al. (2011). Phosphodiesterase-4 inhibition combined with isoniazid treatment of rabbits with pulmonary tuberculosis reduces macrophage activation and lung pathology. Am. J. Pathol. 179, 289–301. doi: 10.1016/j.ajpath.2011.03.039
Tadokera, R., Meintjes, G., Skolimowska, K. H., Wilkinson, K. A., Matthews, K., Seldon, R., et al. (2011). Hypercytokinaemia accompanies HIV-tuberculosis immune reconstitution inflammatory syndrome. Eur. Respir. J. 37, 1248–1259. doi: 10.1183/09031936.00091010
Tadokera, R., Meintjes, G. A., Wilkinson, K. A., Skolimowska, K. H., Walker, N., Friedland, J. S., et al. (2014). Matrix metalloproteinases and tissue damage in HIV-tuberculosis immune reconstitution inflammatory syndrome. Eur. J. Immunol. 44, 127–136. doi: 10.1002/eji.201343593
Taylor, J. L., Hattle, J. M., Dreitz, S. A., Troudt, J. M., Izzo, L. S., Basaraba, R. J., et al. (2006). Role for matrix metalloproteinase 9 in granuloma formation during pulmonary Mycobacterium tuberculosis infection. Infect. Immun. 74, 6135–6144. doi: 10.1128/IAI.02048-05
Theegarten, D., Kahl, B., and Ebsen, M. (2006). [Frequency and morphology of tuberculosis in autopsies: increase of active forms]. Dtsch. Med. Wochenschr. 131, 1371–1376. doi: 10.1055/s-2006-946581
Tobin, D. M., Roca, F. J., Oh, S. F., McFarland, R., Vickery, T. W., Ray, J. P., et al. (2012). Host genotype-specific therapies can optimize the inflammatory response to mycobacterial infections. Cell 148, 434–446. doi: 10.1016/j.cell.2011.12.023
Tsao, T. C., Hong, J., Huang, C., Yang, P., Liao, S. K., and Chang, K. S. (1999). Increased TNF-alpha, IL-1 beta and IL-6 levels in the bronchoalveolar lavage fluid with the upregulation of their mRNA in macrophages lavaged from patients with active pulmonary tuberculosis. Tuber. Lung Dis. 79, 279–285. doi: 10.1054/tuld.1999.0215
Tsao, T. C., Hong, J., Li, L. F., Hsieh, M. J., Liao, S. K., and Chang, K. S. (2000). Imbalances between tumor necrosis factor-alpha and its soluble receptor forms, and interleukin-1beta and interleukin-1 receptor antagonist in BAL fluid of cavitary pulmonary tuberculosis. Chest 117, 103–109. doi: 10.1378/chest.117.1.103
Tsao, T. C., Huang, C. C., Chiou, W. K., Yang, P. Y., Hsieh, M. J., and Tsao, K. C. (2002). Levels of interferon-gamma and interleukin-2 receptor-alpha for bronchoalveolar lavage fluid and serum were correlated with clinical grade and treatment of pulmonary tuberculosis. Int. J. Tuberc. Lung Dis. 6, 720–727.
Ulrichs, T., Kosmiadi, G. A., Jorg, S., Pradl, L., Titukhina, M., Mishenko, V., et al. (2005). Differential organization of the local immune response in patients with active cavitary tuberculosis or with nonprogressive tuberculoma. J. Infect. Dis. 192, 89–97. doi: 10.1086/430621
van Crevel, R., Karyadi, E., Preyers, F., Leenders, M., Kullberg, B. J., Nelwan, R. H., et al. (2000). Increased production of interleukin 4 by CD4+ and CD8+ T cells from patients with tuberculosis is related to the presence of pulmonary cavities. J. Infect. Dis. 181, 1194–1197. doi: 10.1086/315325
Vecino, M., Pasipanodya, J. G., Slocum, P., Bae, S., Munguia, G., Miller, T., et al. (2011). Evidence for chronic lung impairment in patients treated for pulmonary tuberculosis. J. Infect. Public Health 4, 244–252. doi: 10.1016/j.jiph.2011.08.005
Vidyarani, M., Selvaraj, P., Jawahar, M. S., and Narayanan, P. R. (2007). 1, 25 Dihydroxyvitamin D3 modulated cytokine response in pulmonary tuberculosis. Cytokine 40, 128–134. doi: 10.1016/j.cyto.2007.08.005
Vilaplana, C., Marzo, E., Tapia, G., Diaz, J., Garcia, V., and Cardona, P. J. (2013). Ibuprofen therapy resulted in significantly decreased tissue bacillary loads and increased survival in a new murine experimental model of active tuberculosis. J. Infect. Dis. 208, 199–202. doi: 10.1093/infdis/jit152
Volkman, H. E., Pozos, T. C., Zheng, J., Davis, J. M., Rawls, J. F., and Ramakrishnan, L. (2010). Tuberculous granuloma induction via interaction of a bacterial secreted protein with host epithelium. Science 327, 466–469. doi: 10.1126/science.1179663
Walker, N. F., Clark, S. O., Oni, T., Andreu, N., Tezera, L., Singh, S., et al. (2012). Doxycycline and HIV infection suppress tuberculosis-induced matrix metalloproteinases. Am. J. Respir. Crit. Care Med. 185, 989–997. doi: 10.1164/rccm.201110-1769OC
Walker, N. F., Wilkinson, K. A., Meintjes, G., Tezera, L. B., Goliath, R., Peyper, J. M., et al. (2017). Matrix degradation in human immunodeficiency virus type 1-associated tuberculosis and tuberculosis immune reconstitution inflammatory syndrome: a prospective observational study. Clin. Infect. Dis. 65, 121–132. doi: 10.1093/cid/cix231
Wallis, R. S. (2014). Corticosteroid effects on sputum culture in pulmonary tuberculosis: a meta-regression analysis. Open Forum Infect. Dis. 1:ofu020. doi: 10.1093/ofid/ofu020
Wallis, R. S., and Hafner, R. (2015). Advancing host-directed therapy for tuberculosis. Nat. Rev. Immunol. 15, 255–263. doi: 10.1038/nri3813
Wallis, R. S., Kyambadde, P., Johnson, J. L., Horter, L., Kittle, R., Pohle, M., et al. (2004). A study of the safety, immunology, virology, and microbiology of adjunctive etanercept in HIV-1-associated tuberculosis. AIDS 18, 257–264. doi: 10.1097/00002030-200401230-00015
Wallis, R. S., van Vuuren, C., and Potgieter, S. (2009). Adalimumab treatment of life-threatening tuberculosis. Clin. Infect. Dis. 48, 1429–1432. doi: 10.1086/598504
Wang, C. H., Lin, H. C., Lin, S. M., Huang, C. D., Liu, C. Y., Huang, K. H., et al. (2010). MMP-1(-1607G) polymorphism as a risk factor for fibrosis after pulmonary tuberculosis in Taiwan. Int. J. Tuberc. Lung Dis. 14, 627–634.
Willcox, P. A., and Ferguson, A. D. (1989). Chronic obstructive airways disease following treated pulmonary tuberculosis. Respir. Med. 83, 195–198. doi: 10.1016/S0954-6111(89)80031-9
World Health Organization (2017). Global Tuberculosis Report 2017. Geneva: World Health Organization.
Wu, C. W., Wu, J. Y., Lee, M. G., Lai, C. C., Wu, I. L., Tsai, Y. W., et al. (2017). Risk of incident active tuberculosis disease in patients treated with non-steroidal anti-inflammatory drugs: a population-based study. BMC Pulm. Med. 17:82. doi: 10.1186/s12890-017-0425-3
Wu, H. P., Hua, C. C., and Chuang, D. Y. (2007). Decreased in vitro interferon-gamma production in patients with cavitary tuberculosis on chest radiography. Respir. Med. 101, 48–52. doi: 10.1016/j.rmed.2006.04.016
Wynn, T. A., and Ramalingam, T. R. (2012). Mechanisms of fibrosis: therapeutic translation for fibrotic disease. Nat. Med. 18, 1028–1040. doi: 10.1038/nm.2807
Xu, Y., Wang, L., Zimmerman, M. D., Chen, K. Y., Huang, L., Fu, D. J., et al. (2018). Matrix metalloproteinase inhibitors enhance the efficacy of frontline drugs against Mycobacterium tuberculosis. PLoS Pathog. 14:e1006974. doi: 10.1371/journal.ppat.1006974
Yamamura, Y., Ogawa, Y., Maeda, H., and Yamamura, Y. (1974). Prevention of tuberculous cavity formation by desensitization with tuberculin-active peptide. Am. Rev. Respir. Dis. 109, 594–601. doi: 10.1164/arrd.1974.109.6.594
Yamamura, Y., Ogawa, Y., Yamagata, H., and Yamamura, Y. (1968). Prevention of tuberculous cavity formation by immunosuppressive drugs. Am. Rev. Respir. Dis. 98, 720–723. doi: 10.1164/arrd.1968.98.4.720
Yuhas, Y., Azoulay-Alfaguter, I., Berent, E., and Ashkenazi, S. (2007). Rifampin inhibits prostaglandin E2 production and arachidonic acid release in human alveolar epithelial cells. Antimicrob. Agents Chemother. 51, 4225–4230. doi: 10.1128/AAC.00985-07
Zak, D. E., Penn-Nicholson, A., Scriba, T. J., Thompson, E., Suliman, S., Amon, L. M., et al. (2016). A blood RNA signature for tuberculosis disease risk: a prospective cohort study. Lancet 387, 2312–2322. doi: 10.1016/S0140-6736(15)01316-1
Zhang, M., Gong, J., Iyer, D. V., Jones, B. E., Modlin, R. L., and Barnes, P. F. (1994). T cell cytokine responses in persons with tuberculosis and human immunodeficiency virus infection. J. Clin. Invest. 94, 2435–2442. doi: 10.1172/JCI117611
Zheng, L., Lam, W. K., Tipoe, G. L., Shum, I. H., Yan, C., Leung, R., et al. (2002). Overexpression of matrix metalloproteinase-8 and -9 in bronchiectasis airways in vivo. Eur. Respir. J. 20, 170–176. doi: 10.1183/09031936.02.00282402
Keywords: tuberculosis, lung damage, host-directed therapy, cavity, pulmonary function, matrix metalloproteinase, neutrophils, immune mechanisms
Citation: Stek C, Allwood B, Walker NF, Wilkinson RJ, Lynen L and Meintjes G (2018) The Immune Mechanisms of Lung Parenchymal Damage in Tuberculosis and the Role of Host-Directed Therapy. Front. Microbiol. 9:2603. doi: 10.3389/fmicb.2018.02603
Received: 07 June 2018; Accepted: 11 October 2018;
Published: 30 October 2018.
Edited by:
Alessandro Marcello, International Centre for Genetic Engineering and Biotechnology, ItalyCopyright © 2018 Stek, Allwood, Walker, Wilkinson, Lynen and Meintjes. This is an open-access article distributed under the terms of the Creative Commons Attribution License (CC BY). The use, distribution or reproduction in other forums is permitted, provided the original author(s) and the copyright owner(s) are credited and that the original publication in this journal is cited, in accordance with accepted academic practice. No use, distribution or reproduction is permitted which does not comply with these terms.
*Correspondence: Cari Stek, Y2FyaV9zdGVrQGhvdG1haWwuY29t
Disclaimer: All claims expressed in this article are solely those of the authors and do not necessarily represent those of their affiliated organizations, or those of the publisher, the editors and the reviewers. Any product that may be evaluated in this article or claim that may be made by its manufacturer is not guaranteed or endorsed by the publisher.
Research integrity at Frontiers
Learn more about the work of our research integrity team to safeguard the quality of each article we publish.