- 1Department of Chemistry and Biology, National Institute of Technology, Fukui College, Sabae, Japan
- 2Bioproduction Research Institute, National Institute of Advanced Industrial Science and Technology (AIST), Sapporo, Japan
- 3Graduate School of Agriculture, Hokkaido University, Sapporo, Japan
- 4Department of Bioscience and Technology, School of Biological Sciences and Engineering, Tokai University, Sapporo, Japan
- 5Division of Marine Life Science, Faculty of Fisheries Sciences, Hokkaido University, Hakodate, Japan
- 6Hakodate Junior College, Hakodate, Japan
- 7College of Industrial Technology, Nihon University, Narashino, Japan
In Mitchell’s chemiosmotic theory, a proton (H+) motive force across the membrane (Δp), generated by the respiratory chain, drives F1Fo-ATPase for ATP production in various organisms. The bulk-base chemiosmotic theory cannot account for ATP production in alkaliphilic bacteria. However, alkaliphiles thrive in environments with a H+ concentrations that are one-thousandth (ca. pH 10) the concentration required by neutralophiles. This situation is similar to the production of electricity by hydroelectric turbines under conditions of very limited water. Alkaliphiles manage their metabolism via various strategies involving the cell wall structure, solute transport systems and molecular mechanisms on the outer surface membrane. Our experimental results indicate that efficient ATP production in alkaliphilic Bacillus spp. is attributable to a high membrane electrical potential (ΔΨ) generated for an attractive force for H+ on the outer surface membrane. In addition, the enhanced F1Fo-ATPase driving force per H+ is derived from the high ΔΨ. However, it is difficult to explain the reasons for high ΔΨ formation based on the respiratory rate. The Donnan effect (which is observed when charged particles that are unable to pass through a semipermeable membrane create an uneven electrical charge) likely contributes to the formation of the high ΔΨ because the intracellular negative ion capacities of alkaliphiles are much higher than those of neutralophiles. There are several variations in the adaptation to alkaline environments by bacteria. However, it could be difficult to utilize high ΔΨ in the low aeration condition due to the low activity of respiration. To explain the efficient ATP production occurring in H+-less and air-limited environments in alkaliphilic bacteria, we propose a cytochrome c-associated “H+ capacitor mechanism” as an alkaline adaptation strategy. As an outer surface protein, cytochrome c-550 from Bacillus clarkii possesses an extra Asn-rich segment between the region anchored to the membrane and the main body of the cytochrome c. This structure may contribute to the formation of the proton-binding network to transfer H+ at the outer surface membrane in obligate alkaliphiles. The H+ capacitor mechanism is further enhanced under low-aeration conditions in both alkaliphilic Bacillus spp. and the Gram-negative alkaliphile Pseudomonas alcaliphila.
Introduction
Bacteria that thrive under extreme environmental conditions, such as low or high temperatures and high or low pH, are called extremophiles. Each extremophile possesses certain strategies for adaptation under different conditions. These complex strategies consist of environmental adaptation mechanisms, such as alterations in specific characteristics of enzymes and membranes. Although there is a common fundamental basis for extremophile metabolism, these organisms differ slightly in the characteristics of certain components necessary for environmental adaptation (e.g., modification of cell surface structures and protein characteristics and production of molecular chaperones).
Alkaliphiles are defined as microorganisms that exhibit better growth at pH ≥ 9 than at pH < 9. Since Vedder (1934) isolated the obligate alkaliphile Bacillus alcalophilus, many alkaliphilic Bacillus strains have been isolated from common environments such as garden soil and horse manure in the search for enzymatic resources, as alkaliphilic Bacillus strains produce heat-stable enzymes (Horikoshi and Akiba, 1982; Horikoshi and Grant, 1998; Horikoshi, 2006, 2011). Although various strains have been isolated, until Nielsen et al. (1995) proposed nine new Bacillus spp., it was not known whether alkaliphilic bacteria comprise multiple species due to the lack of a molecular identification method concomitant with a gene sequence database. Since the discovery made by Nielsen et al. (1995) many additional alkaliphilic bacteria have been isolated and identified as new species. Related reports indicate that diverse alkaliphiles are distributed in a variety of environments, which may indicate the presence of numerous small alkaline niches [e.g., the termite gut (Thongaram et al., 2003)] and/or large high-pH environments (e.g., alkaline lakes). Alkaline environments have been present throughout Earth’s history. Zavarzin (1993) hypothesized that modern soda lakes may represent a refuge for relict terrestrial communities from ancient continents of the Early Proterozoic Eon. In contrast, a leading hypothesis suggests that the origin of life can be track back to ocean-floor-based alkaline hydrothermal vents (Lane and Martin, 2012). Natural H+ gradients across the membranes of iron monosulfide bubbles could lead to the formation of protocells (Russell and Hall, 1997).
The phylogenetic diversity and wide distribution of alkaliphiles on Earth indicates that the evolution of alkaliphiles is not a specific phenomenon but a common event in natural environments. Therefore, many variations in alkaline adaptation mechanisms should be present in alkaliphiles. The range of reported alkaline adaptation mechanisms has not been explained to date. However, diverse adaptation mechanisms involving secondary cell wall variations were reported by Aono and Horikoshi (1983), Aono et al. (1993, 1995,1999). The formation of a negatively charged secondary cell wall results in the pH at the outer surface membrane being lower than the extracellular pH (i.e., medium pH) (Tsujii, 2002). The diversity of environmental adaptation mechanisms underlying the survival of numerous alkaliphiles of different taxa provides various possible explanations for the existence of life in alkaline environments. Alkaliphilic Bacillus spp. have an acidic secondary cell wall. B. halodurans C-125 produces an acidic secondary cell walls consisting of teichurono-peptide and teichuronic acid. In contrast, B. pseudofirmus OF4 cells produces the cell surface protein SlpA and polyglutamic acid (Gilmore et al., 2000). These structures attract H+ and repel OH-, and the structural functions of those components protects the intracellular metabolic pathways from severe extracellular environments. Although the acidic secondary cell wall is indispensable for alkaline adaptation in alkaliphilic Bacillus spp., the variations in the secondary cell wall structure, which are shared among alkaliphilic Bacillus spp., have not been elucidated to date.
Alkaline environments are not always favorable for alkaliphilic bacteria. Alkaliphilic Bacillus spp. have been reported to produce acid to reduce the pH when the ambient pH is too high for metabolism (Horikoshi, 2006). This acid production can often be observed even in media lacking sugars. In contrast, these bacteria create an alkaline environment when the ambient pH is too low for metabolism. These phenomena indicate that several alkaliphiles have the ability to increase the favorability of the ambient environment. Amphibacillus iburiensis is able to grow in broth medium adjusted to pH 11 prior to inoculation (Hirota et al., 2013). However, this bacterium exhibits distinct growth initiation at pH 9 after lowering the pH of the medium via acid production, as observed by monitoring the change in pH during incubation.
Alkaliphiles adapt to the environment by employing various combinations of mechanisms to adjust to alkaline conditions. Alkaliphilic Bacillus strains have been reported to adjust the intracellular pH to an appropriate level via the Na+/H+ antiporter, Na+ channels or stator force generator that drives Na+-dependent motility (Kitada et al., 1982; Ito et al., 2004a,b; Padan et al., 2005), which contribute to replace the H+-potential base transport system with the Na+-potential base transport system. Thus, the Na+/H+ antiporter and other Na+ or K+-related transport systems reduce the utilization of H+ by transport systems, which is very important for alkaliphiles that thrive at one-thousandth the concentration of H+ found under neutral conditions. In addition, the rotational torque of the flagella of alkaliphilic Bacillus strains is produced by the influx of Na+ derived from the Na+-base potential across the membrane (Ito et al., 2004a).
It is reasonable to consider that the entire solute transport system functions via the Na+ potential across the membrane. However, the ATP synthase-based energy production system is derived from the H+-base potential across the membrane (Dimroth and Cook, 2004), which may indicate localization of the H+-base potential across the membrane in the vicinity of the respiratory chain in the horizontal direction. In addition, if H+ is not attracted to the interface in the vicinity of the outer surface membrane, the H+ concentration will be quite low. The H+-base potential may also be present in the vicinity of the outer surface membrane in the perpendicular direction.
Alkaliphilic Bacillus spp. have been reported to exhibit higher membrane electrical potentials (ΔΨ) than neutralophilic Bacillus strains (Yumoto, 2003; Goto et al., 2005). However, the calculation of bulk-base parameters [ΔΨ and transmembrane pH gradient (ΔpH)] for the Δp driving F1Fo-ATPase does not account for ATP production because the high deficiency in ΔpH is not compensated by ΔΨ. We demonstrated the importance of a large ΔΨ in alkaliphiles by showing the contribution of ΔΨ to the retention of H+ in the vicinity of the outer surface of the membrane in the vertical direction and the contribution of efficient ATP production under conditions involving H+ scarcity (Yoshimune et al., 2010). Furthermore, ΔΨ ensures efficient ATP production to enhance the F1Fo-ATPase driving force per H+. In addition, structural and physicochemical mechanisms to retain H+ at the outer surface of the membrane are indispensable. However, it could be difficult to utilize high ΔΨ in the low aeration condition due to the low activity of respiratory system. In this context, we highlight “a high-potential H+ capacitor mechanism” based on the existence of membrane-bound or periplasmic cytochrome c in alkaliphiles.
Background on the General Bioenergetics and Growth of Alkaliphilic Bacillus spp.
According to Peter Mitchell’s chemiosmotic theory (Mitchell, 1961), the Δp across the membrane that drives F1Fo-ATPase to produce ATP consists of ΔpH (intracellular pH minus extracellular pH) and the difference in electrical potential across the membrane, i.e., ΔΨ (intracellulary electronegative and extracellularly electropositive across the membrane). The Δp can be calculated by the following formula.
R = gas constant (8.315 J mol-1); T = absolute temperature (298 K = 25°C); F = Faraday constant (96.485 kj [v⋅mol]-1).
The H+ gradient is also utilized for other energy-requiring processes such as transmembrane solute transport and signaling. In general, the parameters Δp, ΔΨ, and ΔpH apply to the bulk-base. Although it is difficult to estimate the values of ΔΨ and ΔpH based on the vicinity of the membrane surface, localization of these parameters in the vicinity of the membrane surface in both the horizontal and vertical directions should be considered, especially in the case of the outer surface membrane of alkaliphilic Bacillus spp. (Gennis, 2016; Sjöholm et al., 2017). These characteristics are common to biological energy production mechanisms. Alkaliphilic bacteria must possess distinctive characteristics at the outer surfaces of the membrane to effectively utilize existing stores of H+ at the outer surface membranes.
Although bulk-base calculations cannot account for ATP production by alkaliphilic Bacillus spp. Due to the great hindrance conferred by negative ΔpH values, the growth of these bacteria is vigorous under alkaline conditions (Guffanti and Hicks, 1991). The growth features of facultative alkaliphilic B. pseudofirmus OF4 were estimated at a steady state under various pH-controlled culture conditions. Strain OF4 exhibits specific growth rates of 0.77 and 1.10 h-1 in media maintained at pH values of 7.5 and 10.6, respectively. We estimated the growth characteristics of the obligate alkaliphilic B. clarkii K24-1U in batch culture. Strain K24-1U exhibited more rapid growth (μmax = 0.33 h-1) than the neutralophilic B. subtilis IAM 1026 (μmax = 0.26 h-1) (Hijikata, 2004). These superior growth characteristics are attributable to vigorous ATP production. Thus, in alkaliphilic Bacillus spp., these growth characteristics are not accounted for by bulk-based bioenergetic parameters.
Background on Proton Behavior at the Outer Surface of the Membrane
The zone formed in the vicinity of the outer surface of the membrane formed due to the presence of phosphate and carbonyl headgroups stabilizes excess hydrated H+ relative to the bulk solution. However, the pH of the bulk phase at the outer side membrane in alkaliphiles is expected to be at least 2 units higher than that in neutralophiles. In addition, the rate of H+ exchange between the deep interface zone in the vicinity of the outer surface membrane and the bulk phase in alkaliphiles may be greater than that in neutralophiles due to the large difference in pH between the deep interface zone and the bulk phase at the outer membrane (Mulkidjanian et al., 2006; Gennis, 2016). Therefore, it survival under proton-less conditions without specific alkaline adaptation mechanisms is difficult. Although many neutralophilic Bacillus spp. can grow at pH 9, most cannot grow well at pH 10, unlike alkaliphilic Bacillus spp.
The H+ transfer mechanism on the outer surface of the membrane is dependent on the distance between the H+-vent (e.g., a respiratory complex such as cytochrome c oxidase) and H+-sink (e.g., F1Fo-ATPase). Sjöholm et al. (2017) investigated the distance effect between the terminal oxidase, cytochrome bo3 and F1Fo-ATPase by reconstituting these proteins in a vesicle and measuring ATP synthesis activity. An even shorter distance between the H+-vent and H+-sink than that observed in neutralophiles was expected to be favorable in alkaliphiles. A high cytochrome content has been reported in alkaliphilic Bacillus spp. (Lewis et al., 1980; Guffanti et al., 1986; Yumoto et al., 1997; Goto et al., 2005). This feature will relate to H+ behavior at the outer surface membrane.
Proton Behavior at the Outer Surface Membrane in Alkaliphiles
It is considered that the H+ concentration at the outer surface membrane in alkaliphiles is lower than that in neutralophiles due to the low background H+ concentration in alkaliphiles. In addition, the rate constant of H+ exchange between the deep interface zone of the outer surface membrane and the adjacent bulk zone may be higher in alkaliphiles than in neutralophiles due to the larger pH gap between the deep interface zone of the outer surface membrane and the adjacent bulk zone in alkaliphiles than that in neutralophiles. In addition to the membrane-surface-based ΔpH (ΔpH values) (Xiong et al., 2010), membrane-surface-based ΔΨ values may also exist (Lyu and Lazár, 2017). In addition, proteins present on the outer surface membrane may play a role in the retention of H+ in this region. A comparative experiment to detect H+ in the bulk phase during respiration in the obligate alkaliphilic B. clarkii K24-1U and the neutralophilic B. subtilis IAM 1026 was performed by monitoring the change in pH (Yoshimune et al., 2010). Whole-cell suspensions of both Bacillus stains consumed oxygen immediately after the introduction of oxygen, and a lag period was observed for H+ extrusion by the respiratory chain into the bulk phase. The lag period for alkaliphilic B. clarkii K24-1U was significantly longer at pH 10 than at other pH values and significantly longer than those for B. subtilis at various pH values. This observation may indicate that H+ was transferred into the bulk phase after all the H+ retention sites were occupied by H+ translocated via respiratory complexes. The introduction of monensin, which exchanges extracellular H+ for intracellular Na+, similar to a Na+/H+ antiporter, resulted in a prolonged lag period for H+ extrusion to the bulk, indicating that the H+ present at H+ retention sites on the outer surface membrane translocated to the intracellular side via countertransport with the Na+ present in the intracellular space. In contrast, when a ΔΨ-disrupting agent such as valinomycin or ETH-157 was introduced, the lag phase disappeared. These experiments reveal the meaning of ΔΨ values in alkaliphilic Bacillus spp. are larger than those in neutralophilic Bacillus spp.
Efficiency of H+ Translocation From the Outer Surface Membrane to F1Fo-Atpase
The growth of alkaliphilic Bacillus spp. is much faster than that of neutralophilic Bacillus spp. as described above, which may be attributable to the higher ATP production rate in alkaliphilic Bacillus spp. than that in neutralophilic Bacillus spp. The ATP production rate was estimated using the obligate alkaliphile B. clarkii DSM 8720T and neutralophilic B. subtilis IAM 1026 (Hirabayashi et al., 2012). B. clarkii DSM 8720T produced 7.2 nmol ATP⋅mg protein-1⋅min-1 (endogenous substrate) at pH 10, which was comparable to the amount produced by B. pseudofirmus OF4 (6.6 ± 3.9 nmol ATP⋅mg protein-1⋅min-1 [starved cells re-energized with malate]) at pH 10.5 (Guffanti and Krulwich, 1992). In contrast, B. subtilis IAM 1026 produced 0.96 nmol ATP⋅mg protein-1⋅min-1 at pH 7. Thus, the ATP production rate in alkaliphilic Bacillus spp. was much higher (6.9-7.5 times) than that in neutralophilic B. subtilis.
If rapid ATP production by alkaliphilic Bacillus spp. was attributable to rapid H+ translocation across the membrane by respiratory complexes, then these bacteria would exhibit a rapid O2 consumption and/or high efficiency of H+ translocation across the membrane per molecule of O2 consumed. However, the oxygen consumption rate of B. clarkii DSM 8720T cells was 0.19 μmol O2⋅min-1⋅mg cell protein-1 at pH 10. Although these data were obtained with an endogenous substrate, the results were comparable to those obtained using malate as the substrate in other alkaliphilic Bacillus spp. (Lewis et al., 1980; Guffanti et al., 1986; Aono et al., 1996). The oxygen consumption rate of B. subtilis IAM 1026 was 0.50 μmol O2⋅min-1⋅mg cell protein-1 at pH 7. Thus, the oxygen consumption rate of whole B. subtilis IAM 1026 cells at pH 7 was 2.6 times higher than that of B. clarkii DSM 8720T at pH 10. Unlike the theoretical H+/O ratio calculated for Bacillus spp. (complex III, 4 plus complex IV, 2 = 6) (Sone et al., 1999), the H+/O ratio in B. clarkii DSM 8720T was 3.6 at pH 10. In contrast, the H+/O ratio in B. subtilis IAM 1026 was 5.3. The H+/O ratio in B. subtilis IAM 1026 was 1.5 times higher than that in B. clarkii DSM 8720T (Goto et al., 2016). Considering both the O2 consumption rate and the H+/O ratio in both strains, the H+ translocation rate in B. subtilis IAM 1026 (pH 7) was 3.9 times higher than that in B. clarkii DSM 8720T (pH 10). This finding indicated that the higher ATP production rate in neutralophilic B. subtilis IAM 1026 than that in alkaliphilic B. clarkii DSM 8720T cannot be accounted for by the rate of H+ translocation across the membrane via the respiratory chain. The large differences in ATP production rates between B. clarkii DSM 8720T and B. subtilis IAM 1026 are attributable to differences in the F1Fo-ATPase driving force per H+, which may arise from differences in ΔΨ. It is difficult to translocate H+ from the intracellular to the extracellular side of the membrane because ΔΨ hinders translocation in that direction by electrical attraction. However, once translocated to the outer surface of the membrane, H+ has high potential to drive F1Fo-ATPase. It has been reported that the ΔΨ in alkaliphilic Bacillus spp. is larger than that in neutralophilic Bacillus spp. (Hoffmann and Dimroth, 1991; Krulwich, 1995; Yumoto, 2002; Goto et al., 2005; Hirabayashi et al., 2012). The same phenomenon has been observed in the alkaliphilic B. clarkii DSM 8720T and neutralophilic B. subtilis IAM 1026. The ΔΨ values of B. clarkii DSM 8720T and B. subtilis IAM 1026 were -192 mV at pH 10 and -122 mV at pH 7, respectively (Goto et al., 2016).
Occurrence of ΔΨ
As described above, ΔΨ is very important for attracting H+, which translocates via the respiratory chain to the outer surface membrane. Generally, the ΔΨ across the membrane is generated by translocation of positively charged H+ from the intracellular to the extracellular region across the membrane. However, the larger ΔΨ in alkaliphilic Bacillus spp. than that in neutralophilic Bacillus spp. cannot be accounted for by the rate of H+ translocation. The large ΔΨ values of alkaliphilic Bacillus spp. may be attributed to the production of high Donnan potentials (Donnan, 1924). The Donnan effect is observed in the presence of membrane-impermeable charged molecules on only one side of the membrane. If a membrane-impermeable negatively charged molecules are present only on the intracellular side of the membrane, these molecules contribute to the formation of the ΔΨ. To estimate the contribution of the Donnan effect to the large ΔΨ in alkaliphilic Bacillus spp., intracellular negative ion capacity was estimated in the obligate alkaliphilic B. clarkii DSM 8720T and facultative alkaliphilic B. cohnii YN-2000 and compared to that of the neutralophilic B. subtilis IAM 1026. To estimate the intracellular negative ion capacity, a cell extract containing inside-out membrane vesicles was prepared and titrated using the positively charged substance clupein sulfate. Negative ion capacity is the amount of negative surface charge of substances in a solution (substances in the intracellular fraction of bacterial cells in this study) estimated by the colloid titration method. This method is one procedure by which the net charge density of surfaces, polyelectrolytes levels, and charge demand of colloidal materials in a solution may be estimated. The measured parameter is the capacity of the mixture to adsorb a polyelectrolyte with the opposite net charge. The intracellular negative ion capacity in alkaliphilic Bacillus spp. increased with increasing pH in the range of pH 6-8, whereas the intracellular negative ion capacity of neutralophilic B. subtilis IAM 1026 changed very little within this pH range. To understand the corresponding negative ion capacity when alkaliphilic Bacillus spp. were grown under alkaline conditions, the intracellular pH of the alkaliphilic strains was estimated at an extracellular pH of 10 (Goto et al., 2016). The intercellular pH of the alkaliphilic Bacillus spp. B. clarkii DSM 8720T and B. cohnii YN-2000 was 8.1, which is comparable to values reported previously using other alkaliphilic Bacillus spp. (Guffanti et al., 1986; Guffanti and Hicks, 1991). At this intracellular pH, the negative ion capacities of B. clarkii DSM 8720T and B. cohnii YN-2000 were 2.9 and 3.3 (×106 eq⋅mg protein-1), respectively. The intracellular pH of neutralophilic B. subtilis IAM 1026 was 6.7 when the extracellular pH was 7. At this intracellular pH, the negative ion capacity of B. subtilis IAM 1026 was 0.7 (×106 eqmg protein-1). This finding indicates that alkaliphilic Bacillus spp. possess a much higher intracellular negative ion capacity than neutralophilic B. subtilis. This high intracellular negative ion capacity contributes to the intrinsic ΔΨ. There may be questions regarding the existence of such a high intracellular negative ion capacity. One explanation is that the intracellularly expressed acidic proteins in alkaliphilic Bacillus spp. are negatively charged at slightly higher intercellular pH values (ca. pH 8) than those in neutralophiles (pH 6-7). Whole-genome analyses have been performed previously in alkaliphilic Bacillus spp. as well as neutralophilic Bacillus spp., and Janto et al. (2011) estimated the average pI values of proteins localized in intracellular and extracellular spaces, the cell wall and the membrane. Despite a tendency toward a high frequency of acidic proteins in the cell wall and extracellular proteins in alkaliphiles, the obligate alkaliphile B. selenitireducens ML10 possesses much higher levels of low pI proteins than neutralophiles. The combination of the acidic nature of the intracellular side chains of acidic membrane proteins and intercellular acidic proteins is predicted to contribute to the high negative ion capacity in alkaliphilic Bacillus spp.
Cytochromes c From Various Bacillus spp. and Related Taxa
The primary role of cytochrome c is to transfer electrons between complex III (cytochrome bc1 complex) and complex IV (cytochrome c oxidase) proteins. A typical class I cytochrome c possesses one low-spin heme c in the N-terminal region bound to the protein by two thioether bonds with cysteine residues (Ambler, 1991). The proximal side and distal side of the iron ligands have a histidine residue and a methionine residue, respectively. The molecular weight of cytochrome c is approximately 8,000-14,000, and cytochrome c is a soluble protein in mitochondria and Gram-negative bacteria (Pettigrew and Moore, 1987; Moore and Pettigrew, 1990; Yamanaka, 1992; Brayer and Murphy, 1996).
Although numerous studies have assessed soluble cytochromes c from various sources, including mitochondria and Gram-negative bacteria, there have been limited examples of cytochrome c from Gram-positive bacteria. Despite having some outer surface membrane space, Gram-positive bacteria do not have outer membranes similar to those of Gram-negative bacteria. Therefore, Gram-positive bacteria do not possess a periplasmic space equivalent to that in Gram-negative bacteria. Consequently, all the cytochrome c in Gram-positive bacteria is membrane-bound. For example, B. subtilis possesses two types of membrane-bound cytochromes: c-550 and c-551. Cytochrome c-550 has a molecular mass of 13 kDa, with a membrane-anchored domain consisting of a single α-helical transmembrane segment of a hydrophobic polypeptide containing 30 amino acids (von Wachenfeldt and Hederstedt, 1990). The midpoint redox potential of cytochrome c-550 is +178 mV (von Wachenfeldt and Hederstedt, 1993). The other cytochrome c, namely, c-551, has a molecular mass of 10 kDa and binds to the membrane via a diacyl-glyceryl-cysteine moiety (Bengtsson et al., 1999). The midpoint redox potential of cytochrome c-551 is >100 mV. The functions of these two membrane-binding cytochromes c have not been characterized. However, it is expected that these proteins are involved in transport of electrons between complexes III and IV.
It has been reported that alkaliphilic Bacillus spp. contain higher amounts of membrane-bound cytochrome c than neutralophilic B. subtilis (Yumoto et al., 1997; Hijikata, 2004). In addition, the amount of membrane-bound cytochrome c is low in mutant strains that lack the ability to grow in alkaline media (Lewis et al., 1980). These findings suggest that membrane-bound cytochrome c in alkaliphiles may contribute to adaptation in alkaline environments. Cytochrome c-552 from B. pseudofirmus RAB was first purified and characterized from alkaliphilic Bacillus spp., exhibiting a molecular mass of 16.5 kDa and midpoint redox potential of +66 mV at pH 7, which decreases as the surrounding pH is increased (Davidson et al., 1988). This cytochrome c is normally membrane bound but was purified as a soluble protein. Cytochrome c proteins are predicted to play a role in electron transport between complexes III and IV.
The primary and 3D structures of cytochrome c were first studied in alkaliphilic Bacillus-related taxa using cytochrome c-553 purified from Sporosarcina pasteurii (formerly B. pasteurii) (Benini et al., 2000). Cytochrome c-553 has a low molecular mass of 9.6 kDa and a low midpoint redox potential (+47 mV) (Benini et al., 1998). The crystal structure of the protein exhibits a highly asymmetric charge distribution. Most of the charges are located on the side opposite to that exposed to the heme edge. The localization of charges is related to H+ transfer on the outer surface membrane. Analyses of the physicochemical parameters reveal that the heme solvent accessibility is correlated with entropy. This finding suggests a direct link between the major determinant of the electrochemical potential (entropy) and a structural parameter (heme solvent exposure). The low midpoint redox potential of cytochrome c-553 could be explained by the decrease in reduction entropy via extrusion of water molecules from the protein hydration shell, affecting a large number of water molecules in the case of increased solvent accessibility, which occurs upon heme reduction.
The abundance of alkaliphilic Bacillus spp.-specific membrane-bound cytochrome c (Yumoto et al., 1991) has also been studied using the facultative alkaliphile B. cohnii YN-2000 (Yumoto et al., 2000). The abundance of membrane-bound cytochrome c is higher during growth at pH 10 than during growth at pH 7. In addition, cytochrome abundance is further increased under low-aeration conditions. Solubilized B. cohnii YN-2000 membranes prepared from cells grown at pH 10 using the detergent Triton X-100 contain larger amounts of cytochrome c-553 than solubilized membranes prepared with cells grown at pH 7. The native molecular mass of cytochrome c-553, which was solubilized by Triton X-100 in a buffer, was determined to be 127 kDa by gel filtration. The molecular mass of Triton X-100, which was used to solubilize cytochrome c-553 in solution is 90 kDa. Therefore, if cytochrome c-553 is contained in micelles of Triton X-100, the actual native molecular mass should be 37 kDa. The stoichiometry of cytochrome c-553 and Triton X-100 is considered to be 1:1. In contrast, the molecular mass of cytochrome c-553 was determined to be 10,500 Da by sodium dodecyl sulfate-polyacrylamide gel electrophoresis (SDS-PAGE). The results described above suggest that cytochrome c-553 forms a tetramer in its native form in solution or in the original membrane. Cytochrome c-553 from B. cohnii YN-2000 also exhibits a low midpoint redox potential of +87 mV at the pH values ranging from 6 to 8.
A novel type of cytochrome c oxidase, cytochrome aco3, was purified and characterized from B. cohnii YN-2000 (Qureshi et al., 1990; Yumoto et al., 1993; Denda et al., 2001). Cytochrome c-533 can react with cytochrome aco3, and the reaction is greatly enhanced in the presence of the positively charged substance poly-L-lysine, which may accelerate binding to two negatively charged molecules: cytochrome c-553 and cytochrome aco3. Although cytochrome aco3 was present in equal amounts in cells grown at pH 10 and pH 7, the cytochrome c-553 content was higher in cells grown at pH 10 than in those grown at pH 7. The midpoint redox potential of the attached cytochrome c was +95 mV at pH 7 (Orii et al., 1991). In contrast, cytochrome a exhibited two forms with midpoint redox potentials of +250 mV and +323 mV at pH 7 (Orii et al., 1991). A stopped-flow study on cytochrome aco3 showed that the cytochrome a component exhibited the highest affinity for electrons and only a minimal contribution to O2 reduction among the involved redox components. Therefore, membrane-bound cytochrome c-553 may directly react not only with cytochrome c in cytochrome aco3 but also with the cytochrome a moiety in cytochrome aco3. During electron flow from cytochrome c-553 or cytochrome c to cytochrome a in cytochrome aco3, there is a large midpoint redox potential difference between each component. This significant difference is necessary for electron flow between each cytochrome c and cytochrome a in cytochrome aco3 to overcome the large ΔΨ (Yumoto et al., 1993). Therefore, the large difference in redox potential between the outer surface redox components and intramembrane redox components sustains electron transfer in the respiratory system in alkaliphilic Bacillus spp. In summary, this large difference in midpoint redox potential may be necessary for the generation of produce the large energy potential required for the translocation of intracellular H+ to overcome the hindrance conferred by the high ΔΨ and to retain H+ on the intracellular side of the membrane.
Membrane-Bound Cytochrome c-550 From the Obligate Alkaliphile Bacillus clarkii K24-1U
A previous study suggested that cytochrome c has an important role in the adaptation to alkaline environments based on its abundance (Lewis et al., 1980; Guffanti et al., 1986; Yumoto et al., 1991, 1997). In addition, cytochrome c is likely located at the outer surface membrane and associated with H+ transfer in the vicinity of the membrane. Therefore, we attempted to elucidate the precise structure of membrane-bound cytochrome c in alkaliphilic Bacillus spp. An experiment was performed to isolate intact protein from the obligate alkaliphile B. clarkii K24-1U. Bacillus spp. generally exhibit strong protease activity, making it difficult to isolate intact cytochrome c from their cells. This strong protease activity often causes isolated cytochrome c to exhibit an anchor-less, soluble protein. First, an attempt was made to isolate obligate alkaliphilic Bacillus spp. that exhibit low protease activity. Screening was performed using soil samples from approximately 10 sites in the Hokkaido region of Japan. One strain, K24-1U, isolated from Yuubari in Hokkaido Japan (43°04′ N, 141°58′ E), was found to be an obligate alkaliphile that exhibited very weak protease activity. The cytochrome c-550 gene sequence was determined and cloned, and the protein was purified and characterized (Ogami et al., 2009). Purified cytochrome c-550 was attached to a diacylglycerol-cysteine moiety. According to the analyzed gene sequence of cytochrome c-550, a signal peptide was present at the 5′ end of the gene. During the processing of mature cytochrome c-550, expressed cytochrome c-550 was translocated to the extracellular side of the membrane by the signal peptide. After the signal peptide was dissociated, the terminal cystatin was modified by attaching diacylglycerol and acetyl moieties. Cytochrome c-550 binds to fatty acids with carbon lengths of C15, C16, and C17 via glycerol-Cys18. Although the length of the internal carbon chain is always C15, the external chain length varies from C15-C17. The diacylglycerol moiety exhibits flexibility in its fatty acid molecular species. Therefore, if the expressed amount of cytochrome c-550 is dependent on the culture conditions, the fatty acid composition of the membrane may be minimally influenced.
The amino acid sequence of cytochrome c-550 was deduced from the gene sequence and aligned with the amino acid sequences of cytochromes c from obligate and facultative alkaliphilic and neutralophilic Bacillus spp. and related taxa (Ogami et al., 2009). The results indicated that cytochrome c-550 contains only two basic amino acids, including histidine, in the heme c axial ligand. This scarcity of basic amino acids is more pronounced in obligate alkaliphilic Bacillus spp. than in facultative alkaliphiles and neutralophiles. Cytochrome c-550 contains the distinct amino acid sequence Gly22-Asn34, which is absent in facultative alkaliphilic and neutralophilic Bacillus spp. Thus, this sequence exists specifically for adaptation to alkaline environments. The amino acid sequence Gly22-Asn34 contains the H+-transferable amino acids Asp and Glu at ratios of 3/13 and 1/13, respectively. The most prominent constituent of the Gly22-Asn34 sequence is Asn. Asn is present at a ratio of 7/17 in the Asn21-Asn37 region and may play an important role in the H+ transfer network in the vicinity of the outer surface membrane.
Although Asn is theoretically H+-transferable, there have been a few examples of the contribution of H+ transfer processes due to weak hydrogen binding of the involved residues. Doukov et al. (2007) reported a very interesting hypothetical model of a hydrogen-bond network involving the H+-transferable characteristics of Asn in the methyltetrahydrofolate (MTHF) corrinoid-iron-sulfur protein methyltransferase. The enzyme catalyzes the transfer of the methyl group of MTHF to cob(I)amide. This transfer reaction requires electrophilic activation of the methyl group of MTHF, which includes proton transfer to the N5 group of the pterin ring of MTHF. However, the resolved crystal structure of the methyltransferase revealed no obvious H+ donor within hydrogen-bonding distance of the N5 position of MTHF. Combining kinetic and structural evidence, it was predicted that the extended hydrogen-bond network contributes to H+ transfer to the N5 group of the pterin ring of MTHF. This extended hydrogen-bonding network contains an Asn, a conserved Asp and a water molecule. The evidence from this study suggests that even amino acid residues that exhibit weak hydrogen bonding, such as Asn can contribute to a cumulative hydrogen-bond network such that the overall effect on this transitional state is greater than expected based on the individual components alone. If this knowledge is applied to the Asn21-Asn37 region in cytochrome c-550 in B. clarkii K24-1U, then the region contributes to a cumulative hydrogen-bond network.
Based on the structures of other reported membrane-bound cytochromes c (David et al., 2000), it can be assumed that the region from Asn21 to Asn37 is located outside the α-helical domain from the N-terminus to the surrounding heme c. Thus, the region is located in the vicinity of the outer surface membrane. In addition, we attempted to elucidate the structure of cytochrome c-550 in B. clarkii K24-1U at the original membrane. Cytochrome c-550 with a membrane-anchoring diacylglycerol-cysteine moiety exhibits a tetrameric structure in the presence of the detergent Triton X-100. In addition, a mutant protein containing an N-terminal Cys18 to Met mutation in the mature protein also exists as a tetramer in the absence of Triton X-100. This finding indicates that cytochrome c-550 may exist as a tetramer on the outer surface membrane. This structure may be important not only for the regulation of the redox reaction involving four redox centers, as in the case of cytochrome c3 in Desulfovibrio gigas (Messias et al., 2006) but also for the formation of the hydrogen-bond network.
H+-Coupling Function of Cytochrome c
The above-described H+/O ratio in B. clarkii DSM 8720T (H+/O ratio = 3.6) cannot be explained by the conventional understanding of the function of respiratory chain because the theoretical H+/O ratio in Bacillus spp. should be 6 (complex III, 4 plus complex IV, 2 = 6) (Sone et al., 1999). The H+/O ratio in B. clarkii DSM 8720T, which is lower than the theoretical value, may be attributable to the high amount of energy required to translocate H+ across the membrane under a larger ΔΨ value than those of neutralophiles. Due to the low translocation of H+ to the outer surface of the membrane, mechanisms for retaining H+ and/or regulating H+ behavior on the outer surface membrane are necessary. It is expected that the membrane-bound cytochrome c concomitant with physicochemical factors assumes these functions on the outer surface of the membrane.
Electron transfer-coupled H+ transfer was studied by Murgida and Hildebrandt (2001) via deuterium substitution. Horse heart cytochrome c was bound as a self-assembled monolayer (SAM) on a Ag electrode produced using different chain lengths (C2-C16) of ω-carboxyl alkanethiols. Cytochrome c redox reactions were performed by changing the electrical potential of the Ag electrode and monitoring changes in the Raman spectra. When the distance between cytochrome c and the electrode was short (C2; distance between cytochrome c and the electrode: 6.3 Å), the electron transfer rate between cytochrome c and the Ag electrode was slower in D2O (33 s-1) than that in H2O (132 s-1). However, there was no difference in the electron transfer rate (0.073-0.074 s-1) between cytochrome c and the Ag electrode when the distance between cytochrome c and the electrode was large (C16; distance between cytochrome c and the electrode: 24 Å), regardless of whether the aqueous solvent was D2O or H2O. These results indicate that the D+ exchange rate with amino acids in cytochrome c was not the rate-limiting step when the electron transfer rate between cytochrome c and the Ag electrode was low. H+-exchange-coupled electron transfer between cytochrome c and the Ag electrode may be the rate-limiting step if the rate of electron transfer between cytochrome c and the Ag electrode is high. The above-described rate-limiting H+/D+ transfer-coupled electron transfer was observed during self-assembly but not in solution. Therefore, differentiated H+/D+ transfer-coupled electron transfer can be observed only in the accumulated nanolayers of cytochromes affected by changes in the electric field (Coulomb’s force) of the Ag electrode when an electron is taken in and out concomitant with electron transfer (associated H+/D+ transfer). The fluctuating electric field may be equivariant to the local electric field strength at biological interfaces (Murgida and Hildebrandt, 2004). This finding suggests that the H+ transfer rate between cytochrome c and the H+ transfer network on the outer surface membrane is affected not only by electron transfer to cytochrome c but also by the local electric field strength on the outer surface membrane. Electron transfer and local electric field strength on the outer surface membrane may fluctuate depending on the electron transfer events in the respiratory chain.
The sulfate-reducing bacterium D. gigas, which reduces sulfuric acid to hydrogen sulfide, possesses large quantities of cytochrome c3, with four heme molecules in one protein (Coutinho and Xavier, 1994). The redox potential of cytochrome c changes depending on the pH, affecting the electric field and reducing the sequence of these hemes. Cytochrome c3 has been reported to facilitate the transfer of H+ to the electron transfer complex or F1Fo-ATPase by cooperative H+/e- linkage (redox-Bohr effect) (Louro et al., 1997; Messias et al., 2006). The midpoint redox potentials of heme I, heme II, heme III, and heme IV of cytochrome c3 are -306, -327, -308, and -297 mV, respectively, in solution but -332, -384, -381, and -457 mV, respectively, on the SAM on the electrode. The differences in midpoint redox potentials between the solution and the SAM follow the sequence heme IV (160 mV) > heme III (73 mV) > heme II (57 mV) > heme I (16 mV), which is consistent with the sequence for the distance between hemes and the electrode (shorter distances with larger differences in redox potentials) (Rivas et al., 2005). This finding indicates that the midpoint redox potential is affected by structural changes in the protein concomitant with changes in the electric field (Coulomb’s force). Thus, the redox potential of heme c is affected by localized structural changes in the vicinity of heme c. Changes in the intensity of the redox potential may be accounted for by the reduced distances between the hemes, and the electrode may be affected by the enhanced electric field.
To summarize the above-described phenomena regarding the behavior of redox reactions concomitant with H+ exchange in the aqueous phase in cytochrome c (or heme c), H+ transfer via the H+ exchange network is affected not only by the redox change in heme c but also by the change in the intensity of the electric field on the SAM of cytochrome c. In addition, the same cumulative configuration of the structure of the assembled cytochrome c was indispensable for these events.
A H+-Condenser Produced by Cytochrome c-550
In this section, we consider respiratory regulatory mechanisms based on the obtained experimental data for B. clarkii DSM 8720T and B. clarkii K24-1U because both strains belong to the same species. As described above, the cytochromes c from alkaliphilic Bacillus spp. exhibited lower redox potentials (+47-+95 mV) than those from neutralophilic bacteria (+170-+230 mV) (Hicks and Krulwich, 1995; Yumoto, 2002; Goto et al., 2005) due to electron transport across the membrane between the redox center located in the outer membrane to the intramembrane side and/or transport of the heavier H+ from the intracellular to the extracellular side in the presence of a large ΔΨ. Although the midpoint redox potential of cytochrome c-550 in B. clarkii K24-1U was +83 mV, based on redox titration, the potential was even lower when determined by cyclic voltammetric measurements (Ogami et al., 2009), probably because the redox potential was affected by the electric field of the electrode during cyclic voltammetric measurement. In the abovementioned example of cytochrome c in the SAM, the midpoint redox potential and H+ transfer behavior of cytochrome c-550 were also affected by ΔΨ. For example, the ΔΨ of B. clarkii DSM 8720T was lower under low-aeration conditions than under high-aeration conditions (Goto et al., 2016). Thus, electron transport via the respiratory chain concomitant with H+ transport across the membrane is relatively easy under low-aeration conditions, whereas the Δp per H+ is lower under low-aeration conditions than under high-aeration conditions. Under high-aeration conditions, the cytochrome c content of B. clarkii K24-1U (0.23 nmolmg protein-1) in the membrane is lower than that (1.38 nmolmg protein-1) under low-aeration conditions (Figure 1). This finding suggested that the membrane-bound cytochrome c in B. clarkii plays an important role under low-aeration conditions at a pH of approximately 10. Growth characteristics were studied under both conditions for alkaliphilic B. clarkii K24-1U (Table 1). The μmax and OD650,max of B. clarkii under low-aeration conditions were 0.26 h-1 and 0.96 (18 h), respectively, whereas the μmax and OD650,max under high-aeration conditions were 0.21 h-1 and 1.27 (12 h), respectively. These results indicated that although the growth rate and intensity of B. clarkii were greatly influenced by aeration conditions, the bacterium retained a high growth rate under low-aeration conditions. In addition, despite a culture duration of 21 h, the growth intensity remained high. This finding is quite remarkable considering the low H+ concentration at the outer surface membrane and the low number of terminal electron acceptors (low O2 concentration) in the conducting respiratory system. Therefore, it can be assumed that an increased level of membrane-bound cytochrome c affects H+ transfer at the outer surface of the membrane as follows: H+ condensation at the outer surface membrane via both (i) the electrical force (in reduced form) (ii) the hydrogen-bond network produced by the chemical characteristics of the protein (via Asn-rich structures). Thus, we hypothesize that the H+-condensation mechanism produced by cytochrome c in certain alkaliphilic Bacillus spp. plays very significant role under condition of limited aeration (Figure 2).
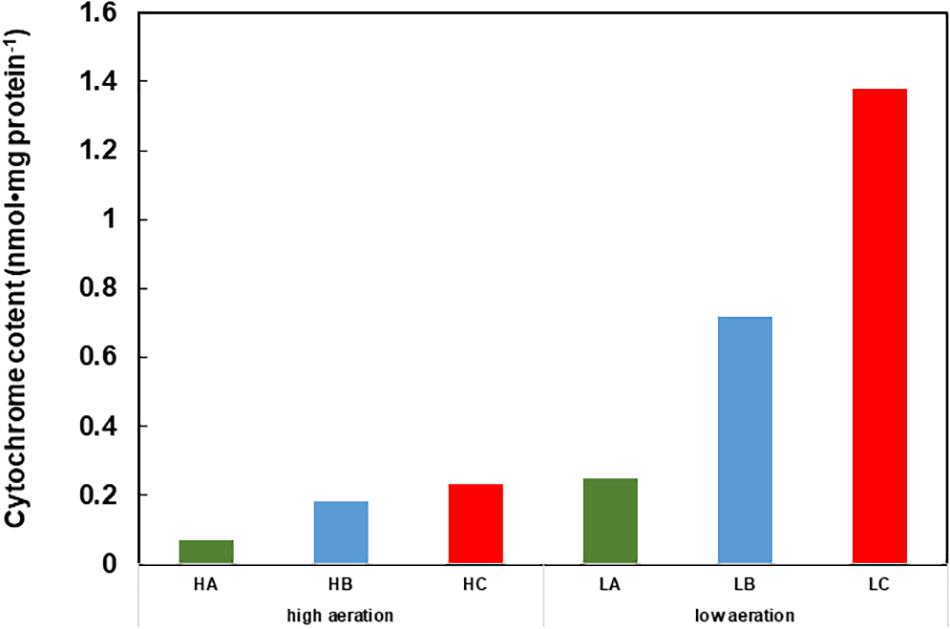
FIGURE 1. Cytochrome content of cell extracts of Gram-positive obligately alkaliphilic Bacillus clarkii K24-1U (grown at pH 10) under low-aeration and high-aeration conditions. HA, HB, and HC are the cytochrome a, b, and c levels, respectively, under high-aeration conditions. LA, LB, and LC are the cytochrome a, b, and c levels, respectively, under low-aeration conditions. This difference in cytochrome c content may indicate that cytochrome c has an important function under low-aeration conditions at pH 10. Low-aeration conditions were produced by using 15 L of a medium in a 20-L stainless-steel fermenter (Takasugi Seisakusho, Tokyo, Japan) with an agitation speed of 106 rpm and an air flow rate of 20 Lmin-1, while high-aeration conditions were produced by using 15 L of a medium in a 30-L stainless-steel fermenter (Marubishi, Tokyo, Japan) with an agitation speed of 250 rpm and an air flow rate of 20 Lmin-1. This figure was made according to the data of Hijikata (2004).
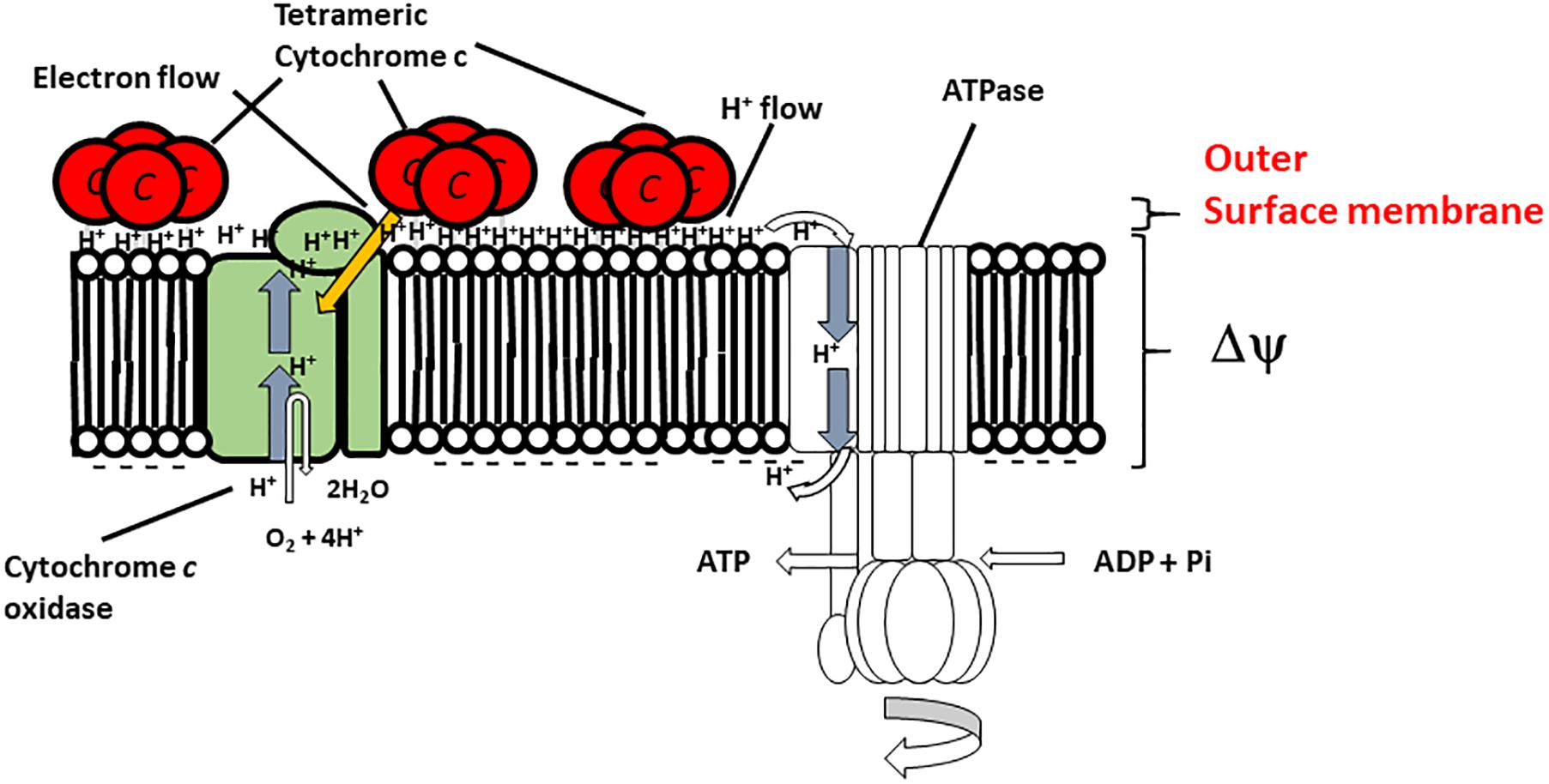
FIGURE 2. Hypothetical model of the function of membrane-bound cytochrome c-550 in the respiratory system of B. clarkii K24-1U. Cytochrome c-550 contains the specific structure Gly22-Asn34 (Asn-rich) at the N-terminal region of its sequence, which may facilitate H+ transfer at the interface of the outer surface membrane. The tetrameric structure is predicted to be important for enhancement of the H-bound network. The production of cytochrome c-550 was enhanced under low-aeration conditions. This enhanced cytochrome c-550 on the outer surface of the membrane led to the accumulation of electrons, H+ and the H+-condenser construct. This structure facilitates the growth of the microorganism, especially under high-pH and low-aeration conditions. This figure was produced as an original hypothetical model for this review.
Soluble Form Cytochrome c in the Gram-Negative Alkaliphile Pseudomonas alcaliphila Al15-21T
Gram-negative alkaliphiles have been studied far less investigated than Gram-positive alkaliphiles, probably because most of the sources for alkaliphile isolation have been terrestrial samples, such as soil. The facultative alkaliphile P. alcaliphila AL15-21T was isolated from seawater obtained from the coast of Rumoi, Hokkaido, Japan (43°56′N 141°38′E) (Yumoto et al., 2001), and the cytochromes c of this bacterium was studied because Gram-negative bacteria possess soluble cytochromes c (Matsuno et al., 2007, 2009; Matsuno and Yumoto, 2015). This property is due to the presence of periplasmic space in Gram-negative bacteria on the outer side of the membrane. Pseudomonas spp. belonging to the same node as P. alcaliphila (such as P. mendocina and P. toyotomiensis) in a phylogenetic tree based on the 16S rRNA gene sequence are able to grow at pH 10 (Hirota et al., 2011). This relationship between alkaline adaptation and phylogenetic position based on 16S rRNA gene sequences is similar to that observed for alkaliphilic Bacillus spp.
The soluble cytochrome c content in cells grown at pH 7-10 under low- or high-aeration conditions was estimated (Matsuno et al., 2007). The highest amount of cytochrome c was observed in cells grown at pH 10 under low-aeration conditions. The cytochrome c content in cells grown at pH 10 under low-aeration conditions (0.47 ± 0.05 nmolmg protein-1) was 3.6 times higher than that in cells grown at pH 7 under high-aeration conditions, which was the lowest cytochrome c content among the tested samples (0.13 ± 0.05 nmolmg protein-1) (Matsuno et al., 2009). The increased cytochrome c content at high pH under low-aeration conditions was similar to that observed for facultative alkaliphilic Bacillus spp. such as B. clarkia K24-1U (Figure 1; Hijikata, 2004).
The soluble fraction of P. alcaliphila AL15-21T contains three types of cytochrome c: cytochrome c-552, cytochrome c-554, and cytochrome c-551. Cytochrome c-522 is the major soluble cytochrome c component, constituting 64% of the total cytochrome c content in P. alcaliphila AL15-21T (Matsuno and Yumoto, 2015). One particular characteristic of cytochrome c-552 is that the resting state of this protein is similar to its fully reduced state (Matsuno et al., 2009). Thus, cytochrome c-552 possesses electron-retention characteristics. The molecular mass of this protein is 7.5 kDa, as determined by SDS-PAGE, which is somewhat smaller than the reported masses of cytochrome c proteins isolated from neutralophilic P. aeruginosa (9-15 kDa). A phylogenetic analysis performed using the amino acid sequence of cytochrome c-552 classified it as a small cytochrome c5 belonging to group 4 of class I cytochrome c proteins (Matsuno et al., 2007; Matsuno and Yumoto, 2015). Class I cytochromes c consist of six groups, and group four contains monoheme cytochromes c form Gram-negative bacteria such as Pseudomonas spp. and Shewanella spp. (Sone and Toh, 1994; Bertini et al., 2006; Matsuno and Yumoto, 2015). The midpoint redox potential of cytochrome c-552 determined by redox titration (+228 mV) was almost the same as that determined by cyclic voltammetry (+224 mV) (Matsuno et al., 2009). Cytochrome c-552 reacts with the terminal oxidase in the respiratory system (Matsuno et al., 2009).
The pH dependence of the cytochrome c-552 reduction rate was determined by estimating the reduction rate under anaerobic conditions (Matsuno et al., 2009). Cytochrome c-552 was fully reduced after 40 h at pH 8.5 but was fully reduced after 4 h at pH 10 in the presence of the electron mediator TMPD (N,N,N′,N′-tetramethyl-p-phenylenediamine). The reduction rate exhibited first-order reaction constants of 0.07 and 0.56 h-1 at pH 8.5 and pH 10, respectively. The oxidation rates of cytochrome c-552 and horse heart cytochrome c were estimated at pH 6-10 under ambient conditions. Cytochrome c-552 was oxidized very slowly at pH 8-10, with the slowest rate observed at pH 8, but was oxidized rapidly from pH 6-7. The oxidation rates of horse heart cytochrome c were consistently high at pH 6-10. The results demonstrated that cytochrome c-552 possessed distinctive electron retention characteristics. If electron-transfer-coupled H+ transfer (redox-Bohr effect) is possible, then cytochrome c-552 retains H+ in the periplasmic space.
To understand the physiological function of P. alcaliphila AL15-21T cytochrome c-552, an antibiotic-marker-less cytochrome c-552-deficient mutant was constructed to exclude the effects of antibiotics (Matsuno et al., 2011). The growth features of the wild-type P. alcaliphila AL15-21T and cytochrome c-552 deletion mutant strains were compared at pH 10 and 7 under low- and high-aeration conditions by estimating the maximum specific growth rate (μmax [h-1]) and maximum cell turbidity (OD660,max) (Figure 3 and Table 2). The most significant differences in the growth parameters were observed at pH 10 under low-aeration conditions between the wild-type (μmax [h-1] = 0.85, OD660,max = 0.27) and the cytochrome c-522 deletion mutant (μmax [h-1] = 0.69, OD660,max = 0.20). The μmax (h-1) of the deletion mutant was 1.5 times higher, whereas the OD660,max was 26% lower than that of the wild-type strain (Table 2).
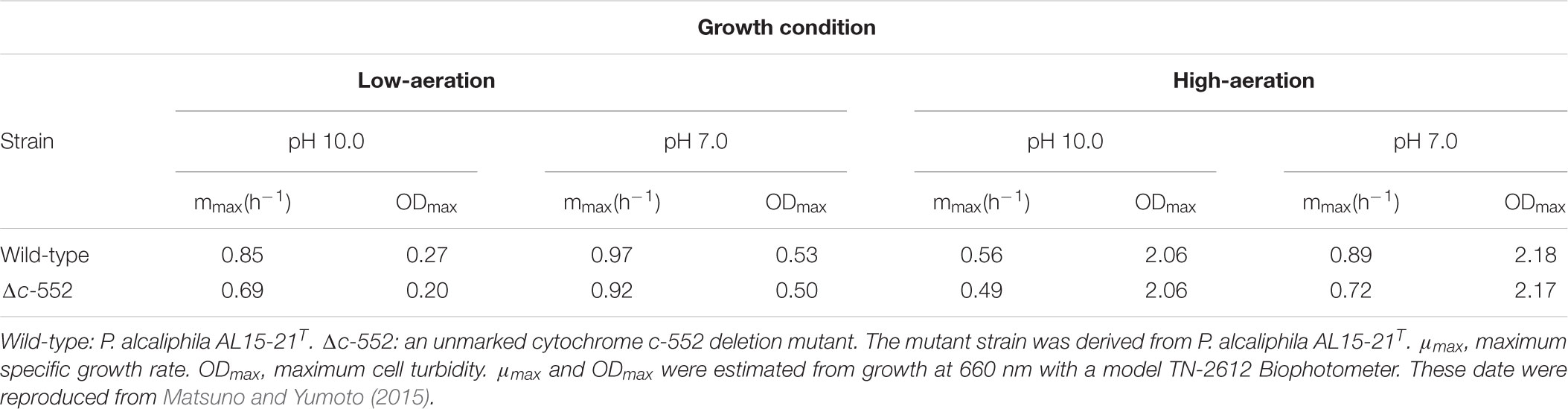
TABLE 2. Growth characteristics of wild-type and cytochrome c-552 deletion mutant strains of P. alcaliphila AL15-21T.
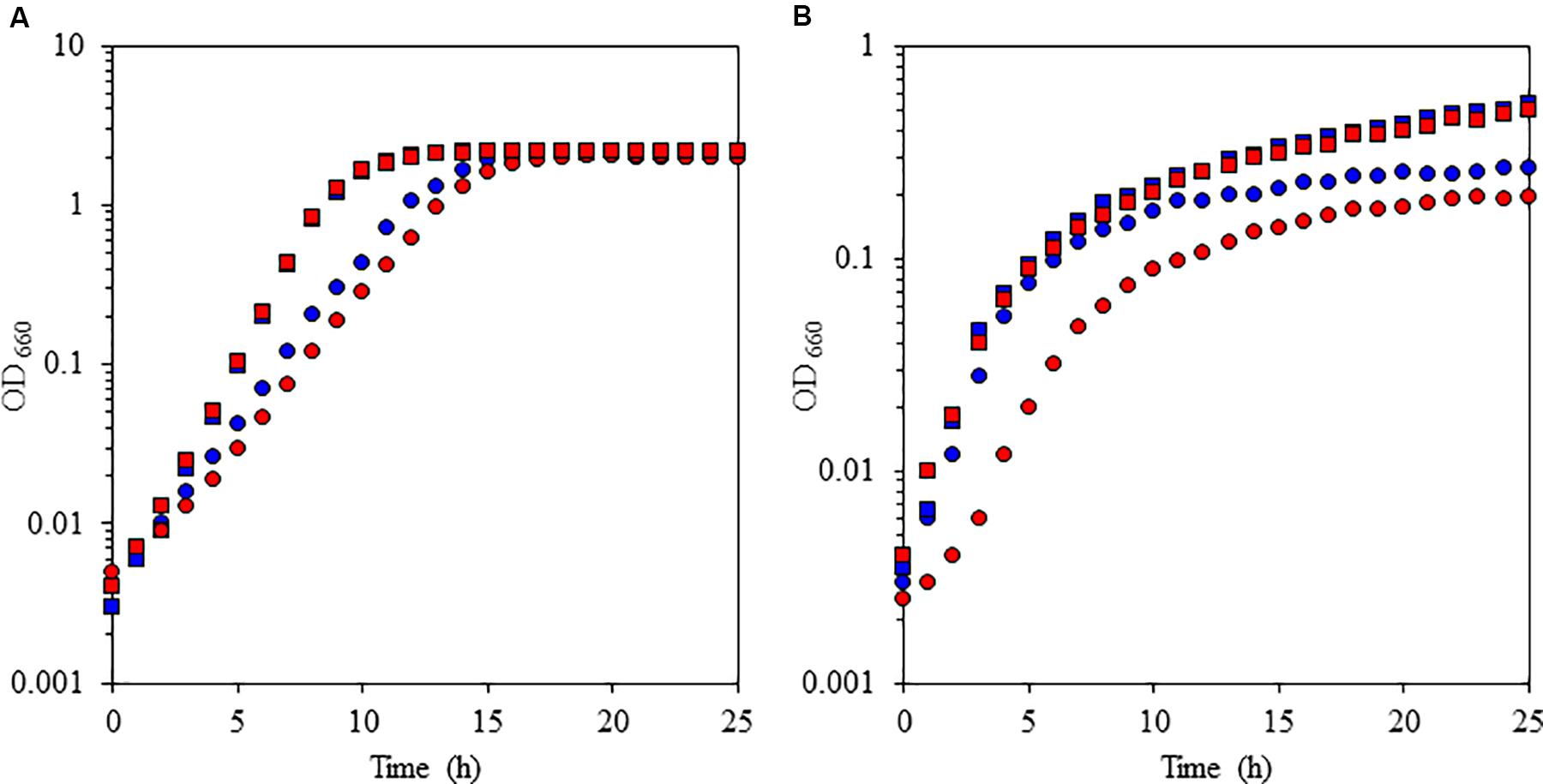
FIGURE 3. Growth characteristics of the facultative alkaliphile Pseudomonas alcaliphila AL15-1T (wild type: blue symbols) and the cytochrome c-522 deletion mutant derived from the wild-type strain (mutant: red symbols) under high-aeration (A) and low-aeration (B) conditions at pH 10 (squares) and pH 7 (circles). The reproducibility of the results was confirmed by performing three independent experiments. This figure was reproduced from Matsuno and Yumoto (2015).
The oxygen consumption rates of cell suspensions of the wild-type strain and the cytochrome c-522 deletion mutant of P. alcaliphila AL15-21T were assessed under different conditions (i.e., pH 7 or pH 10; high or low aeration) (Matsuno et al., 2011). The oxygen consumption rates in the cytochrome c-522 deletion mutant cells under low- and high-aeration conditions increased by 12 and 17%, respectively, compared to those in the wild type. This finding indicates that cytochrome c-552 hinders the direct electron transfer in the respiratory chain. A possible role of this protein might be the formation of an electron bypass to construct an electron reservoir in the periplasmic space. This hypothesis is consistent with the finding that cytochrome c-552 has strong electron retention ability at a high pH values (Figure 4).
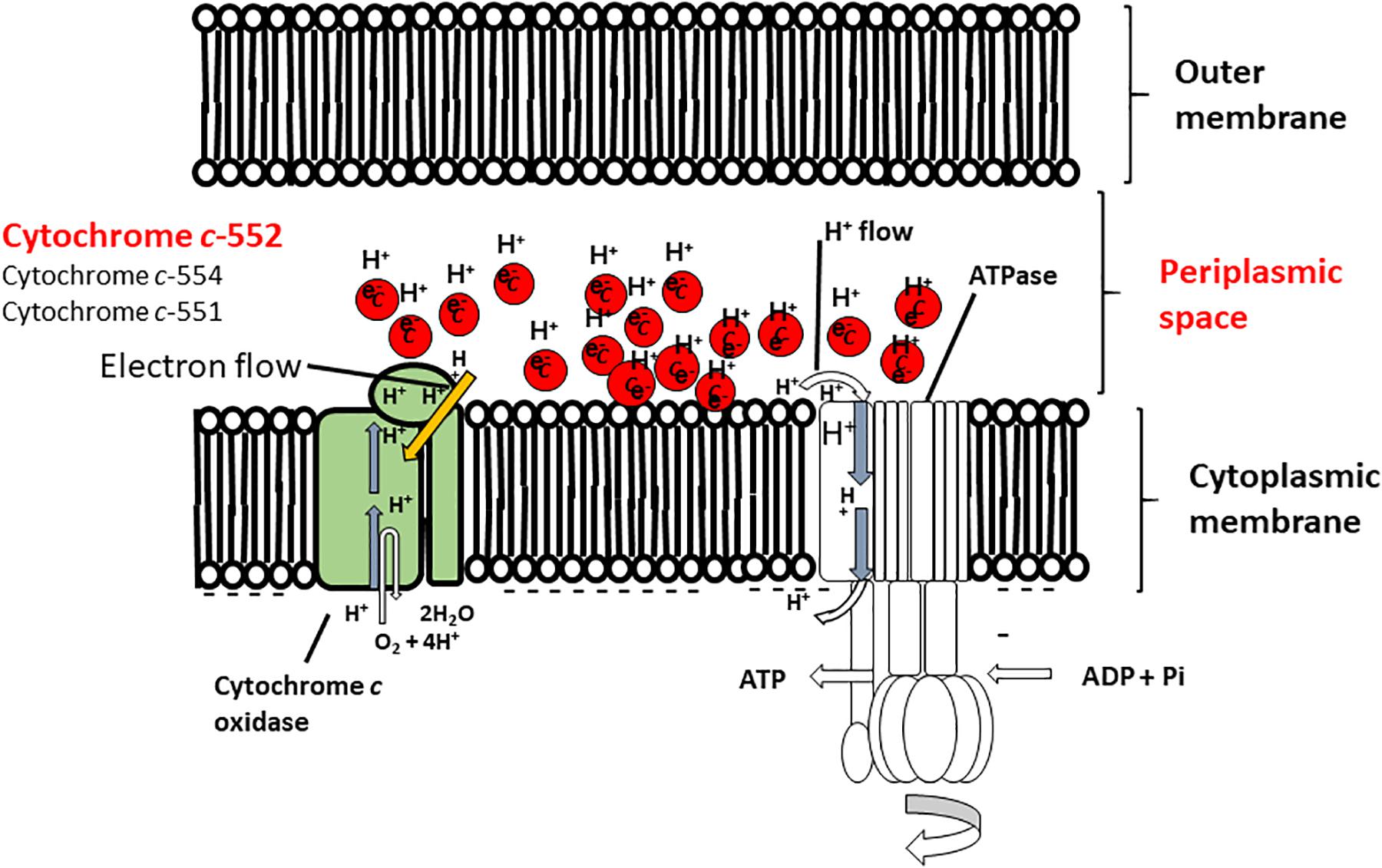
FIGURE 4. Hypothetical model of the function of cytochrome c-552 in the respiratory system of the Gram-negative facultative alkaliphilic P. alcaliphila AL15-1T. This bacterium possesses cytochrome c-552 (monoheme), cytochrome c-554 (monoheme), and cytochrome 551 (diheme), all of which tend to retain the reduced state. Cytochrome c-552 is a major soluble cytochrome c constituent in the periplasmic space. The features of cytochrome c-552 cause in high electron retention and attract H+, and the development of electron- and H+ -retention conditions occurs in the periplasmic space of microorganisms concomitant with an abundance of this cytochrome c under low-aeration conditions at pH 10. Thus, Gram-negative P. alcaliphila AL15-1T expresses an electron and H+ condenser in the periplasmic space. This figure was reproduced based on a hypothetical model presented in Matsuno and Yumoto (2015).
Conclusion and Perspectives
Bacteria utilize several strategies to adapt to high pH, avoid OH- and manage scarce H+ resources via secondary cell wall components, the Na+-based transportation system and flagellar rotation. However, for the respiratory system, the management of energy production under one-thousandth of the ambient concentrations of H+ is difficult. In the case of alkaliphilic Bacillus spp., a large ΔΨ is indispensable for adaptation at high pH. This high value not only compensates for the deficient ΔpH but also attracts H+ moieties that are translocated by the respiratory chain and affect the redox potential of the cytochrome c bound to the outer surface membrane. However, although we have not determined the ΔΨ of alkaliphilic Pseudomonas spp., ΔΨ is not believed to greatly influence the cytochrome c in the periplasmic space. This difference may be attributable to differences in the proteins present in the periplasmic space in Gram-negative Pseudomonas spp. Thus, the effect of ΔΨ is assumed to encompass the outer surface membrane, although this parameter hardly affects the entire periplasmic space. The main region involved in alkaline adaptation in alkaliphilic Bacillus spp. is the outer surface membrane, comprising the ΔΨ (physical parameter) and proteins (associated with the acidic nature and retention and transportation of H+ and electrons) located at the outer surface membrane. In contrast, the main region involved in adaptation in alkaliphilic Pseudomonas spp. is the periplasmic space and the corresponding localized proteins (associated with the acidic nature and retention and transportation of H+ and electrons).
Cytochrome c is a well-known electron carrier in the respiratory chain. However, as described above, despite several reports regarding the H+-transferring characteristics of cytochrome c, it is difficult to conclude that these characteristics are well understood. Studies of cytochrome c in alkaliphilic bacteria have shown that not only the H+-transferring characteristics but also the H+-retention features of cytochrome c are important for energy production, especially under low-aeration conditions. We propose that cytochrome c participates in the “H+ capacitor mechanism” as an energy production strategy under low-aeration and alkaline conditions. Surprisingly, although the cell surface structure is completely different between Gram-positive and Gram-negative bacteria, the H+ and electron retention characteristics of cytochrome c are important. However, there are several differences (the charge in redox potential depends on ΔΨ, the value of the redox potential and electron retention ability) among cytochrome c proteins. These changes may be attributable to strategic differences in the reaction site, which is an interfacial surface (outer surface membrane) or space (periplasmic space). As described above, cytochrome c has multiple functions (electron carrier or reservoir, H+ carrier or reservoir and acidic nature). However, pronounced expression of cytochrome c is not considered indispensable for adaptation under alkaline conditions because some alkaliphilic Bacillus spp. do not express large amounts of cytochrome c. According to the experimental results described above, alkaliphiles that express large amounts of cytochrome c likely exhibit superior growth features at a high pH at low-oxygen concentrations.
Author Contributions
KoY, NI, HiM, KaY, and IY designed the research. TM, TG, SO, and HaM performed the research. KaY and IY analyzed the data and wrote the paper.
Funding
This work was supported by an internal grant from the National Institute of Advanced Industrial Science and Technology (AIST) (to IY).
Conflict of Interest Statement
The authors declare that the research was conducted in the absence of any commercial or financial relationships that could be construed as a potential conflict of interest.
Acknowledgments
We wish to thank Dr. Tamotsu Tsukahara, Mr. Nozomu Morishita, Mr. Shoichi Hijikata, and Mr. Toshikazu Hirabayashi for providing technical assistance.
References
Ambler, R. P. (1991). Sequence variability in bacterial cytochrome c. Biochim. Biophys. Acta 1058, 42–47. doi: 10.1016/S0005-2728(05)80266-X
Aono, R., and Horikoshi, K. (1983). Chemical composition of cell walls of alkalophilic strains of alkalophilic strains of Bacillus. J. Gen. Microbiol. 129, 1083–1087.
Aono, R., Ito, M., and Horikoshi, K. (1993). Occurrence of teichurono peptide in cell walls of group 2 alkaliphilic Bacillus sp. J. Gen. Microbiol. 139, 2738–2744. doi: 10.1099/00221287-139-11-2739
Aono, R., Ito, M., Joblin, K. N., and Horikoshi, K. (1995). A high cell wall negative charge is necessary for the growth of alkaliphile Bacillus lentus C-125 at elevated pH. Microbiology 141, 2955–2964. doi: 10.1099/13500872-141-11-2955
Aono, R., Ito, M., and Machida, T. (1999). Contribution of the cell wall component teichuronopeptide to pH homeostasis and alkaliphily in the alkaliphilic Bacillus lentus C-125. J. Bacteriol. 181, 6600–6606.
Aono, R., Kaneko, H., and Horikoshi, K. (1996). Alkaline growth pH-dependent increase of respiratory and NADH-oxidation activities of the facultatively alkaliphilic strain Bacillus lentus C-125. Biosci. Biotech. Biochem. 60, 1243–1247. doi: 10.1271/bbb.60.1243
Bengtsson, J., Rivolta, C., Hederstedt, L., and Karamata, D. (1999). Bacillus subtilis contains two small c-type cytochromes with homologous heme domains but different type of membrane anchors. J. Biol. Chem. 274, 26179–26184. doi: 10.1074/jbc.274.37.26179
Benini, S., Borsari, M., Ciurli, S., Dikiy, A., and Lamborghini, M. (1998). Modulation of Bacillus pasteurii cytochrome c553 reduction potential by structural and solution-parameters. J. Biol. Inorg. Chem. 3, 371–382. doi: 10.1007/s007750050247
Benini, S., González, A., Rypniewski, W. R., Wilson, K. S., Van Beeumen, J. J., and Ciurli, S. (2000). Crystal structure of oxidized Bacillus pasteurii cytochrome c553 at 0.97-Å resolution. Biochemistry 39, 13115–13126. doi: 10.1021/bi000402j
Bertini, I., Cavallaro, G., and Rosato, A. (2006). Cytochrome c: occurrence and functions. Chem. Rev. 106, 90–115. doi: 10.1021/cr050241v
Brayer, G. D., and Murphy, M. P. (1996). “Structural studies of eukaryotic cytochromes c,” in Cytochrome c. A Multidisciplinary Approach, eds R. A. Scott and A. G. Mauk (Sausalio, CA: University Science Books), 103–166.
Coutinho, I. B., and Xavier, A. V. (1994). Inorganic microbial sulfur metabolism. Methods Enzymol. 234, 119–140. doi: 10.1016/0076-6879(94)43011-X
David, P. S., Dutt, P. S., Wathen, B., Jia, Z., and Hill, B. C. (2000). Characterization of structural model of membrane bound cytochrome c-550 from Bacillus subtilis. Arch. Biochem. Biophys. 377, 22–30. doi: 10.1006/abbi.2000.1732
Davidson, M. W., Gray, K. A., Knaff, D. B., and Krulwich, T. A. (1988). Purification and characterization of two soluble cytochromes from the alkalophile Bacillus firmus RAB. Biochim. Biophys. Acta 933, 470–477. doi: 10.1016/0005-2728(88)90082-5
Denda, K., Oshima, A., and Fukumori, Y. (2001). Structural analyses of the deduced amino acid sequences of a novel type heme-copper terminal oxidase, cytochrome aco3, from alkalophilic Bacillus YN-2000. Can. J. Microbiol. 47, 1075–1081. doi: 10.1139/w01-114
Dimroth, P., and Cook, G. M. (2004). Bacterial Na+- or H+-coupled ATP synthases operating at low electrochemical potential. Adv. Microb. Physiol. 49, 175–218. doi: 10.1016/S0065-2911(04)49004-3
Donnan, F. G. (1924). The theory of membrane equilibria. Chem. Rev. 1, 73–90. doi: 10.1021/cr60001a003
Doukov, T. I., Hemmi, H., Drenman, C. L., and Ragsdale, S. W. (2007). Structural and kinetic evidence for extended hydrogen-bonding network in catalysis of methyl group transfer. Role of active site asparagine residue in activation of methyl transfer by methyltransferase. J. Biol. Chem. 282, 6609–6618. doi: 10.1074/jbc.M609828200
Gennis, R. B. (2016). Proton dynamics at the membrane surface. Biophys. J. 110, 1909–1911. doi: 10.1074/jbc.M609828200
Gilmore, R., Messner, P., Guffanti, A. A., Kent, R., Scheberl, A., Kendrick, N., et al. (2000). Two-dimensional gel electrophoresis of pH-dependent protein expression in facultatively alkaliphilic Bacillus pseudofirmus OF4 lead to characterization of an S-layer protein with a role in alkaliphily. J. Bacteriol. 182, 5969–5981. doi: 10.1016/j.bpj.2016.04.001
Goto, T., Hirabayashi, T., Morimoto, H., Yamazaki, K., Inoue, N., Matsuyama, H., et al. (2016). Contribution of intracellular negative ion capacity to Donnan effect across the membrane in alkaliphilic Bacillus spp. J. Bioeng. Biomembr. 48, 87–96. doi: 10.1007/s10863-015-9641-9
Goto, T., Matsuno, T., Hishinuma-Narisawa, M., Yamazaki, K., Matsuyama, H., Inoue, N., et al. (2005). Cytochrome c and bioenergetic hypothetical model for alkaliphilic Bacillus spp. J. Biosci. Bioeng. 100, 365–379. doi: 10.1007/s10863-015-9641-9
Guffanti, A. A., Finkelthal, O., Hicks, D. B., Falk, L., Sidhu, A., Garro, A., et al. (1986). Isolation and characterization of new facultatively alkalophilic strains of Bacillus species. J. Bacteriol. 167, 766–773. doi: 10.1263/jbb.100.365
Guffanti, A. A., and Krulwich, T. A. (1992). Features of apparent nonchemiosmotic energization of oxidative phosphorylation by alkaliphilic Bacillus firmus OF4. J. Biol. Chem. 267, 9580–9588. doi: 10.1128/jb.167.3.766-773.1986
Guffanti, A. A., and Hicks, D. B. (1991). Molar growth yields and bioenergetic parameters of extremely alkaliphilic Bacillus species in batch cultures, and growth in a chemostat at pH 10.5. J. Gen. Microbiol. 137, 2375–2379. doi: 10.1099/00221287-137-10-2375
Hicks, D. B., and Krulwich, T. A. (1995). The respiratory chain of alkaliphilic bacteria. Biochim. Biophys. Acta 1229, 303–314. doi: 10.1016/0005-2728(95)00024-D
Hijikata, S. (2004). Purification and Characterization of Cytochrome c-550 from Obligate Alkaliphilc Bacillus Clarkii K24-1U. Master’s thesis, Hokkaido University, Sapporo.
Hirabayashi, T., Goto, T., Morimoto, H., Yoshimune, K., Matsuyama, H., and Yumoto, I. (2012). Relationship between rates of respiratory proton extrusion and ATP synthesis in obligately alkaliphilic Bacillus clarkii DSM 8720T. J. Bioeng. Biomembr. 44, 265–272. doi: 10.1007/s10863-012-9430-7
Hirota, K., Aino, K., and Yumoto, I. (2013). Amphibacillus iburiensis sp. nov., an alkaliphile that reduces an indigo dye. Int. J. Syst. Evol. Microbiol. 63, 4303–4308. doi: 10.1099/ijs.0.048009-0
Hirota, K., Yamahira, K., Nakajima, K., Nodasaka, Y., Okuyama, H., and Yumoto,, I. (2011). Pseudomonas toyotomiensis sp. nov., a psychrotolerant facultative alkaliphile that utilizes hydrocarbons. Int. J. Syst. Evol. Microbiol. 61, 1842–1848. doi: 10.1099/ijs.0.024612-0
Hoffmann, A., and Dimroth, P. (1991). The electrochemical proton potential of Bacillus alcalophilus. Eur. J. Biochem. 201, 467–473. doi: 10.1111/j.1432-1033.1991.tb16304.x
Horikoshi, K. (2011). “Enzymes isolated from alkaliphiles,” in Extremophiles Handbook, eds K. Horikoshi, G. Antranikian, A. T. Bull, F. T. Robb, and K. O. Stetter (Tokyo: Springer), 163–181. doi: 10.1007/978-4-431-53898-1_9
Horikoshi, K., and Akiba, T. (1982). Alkaliphilic Microorganisms. A New Microbial World. Berlin: Springer-Verlag.
Horikoshi, K., and Grant, W. D. (1998). Extremophiles, Microbial Life in Extreme Environments. Hoboken, NJ: John Wiley Sons, 322.
Ito, M., Hicks, D. B., Henkin, T. M., Guffanti, A. A., Powers, B. D., Zvi, L., et al. (2004a). MotPS is the stator-force generator for motility of alkaliphilic Bacillus, and its homologue is a second functional Mot in Bacillus subtilis. Mol. Microbiol. 53, 1035–1049.
Ito, M., Xu, H. X., Guffanti, A. A., Wei, Y., Zvi, L., Clapham, D. E., et al. (2004b). The voltage-gated Na+ NavBP has a role in motility, chemotaxis, and pH homeostasis of an alkaliphilic Bacillus. Proc. Natl. Acad. Sci. U.S.A. 101, 10566–10571.
Janto, B., Ahmed, A., Ito, M., Liu, J., Hicks, D. B., Pagni, S., et al. (2011). Genome of alkaliphilic Bacillus pseudomonas OF4 reveals adaptation that support the ability to grown in external pH range from 7.5 to 11.4. Environ. Microbiol. 13, 3289–3309. doi: 10.1111/j.1462-2920.2011.02591.x
Kitada, M., Guffanti, A. A., and Krulwich, T. A. (1982). Bioenergetic properties and viability of alkalophilic Bacillus firmus RAB as a function of pH and Na+ contents of the incubation medium. J. Bacteriol. 152, 1096–1104.
Krulwich, T. A. (1995). Alkaliphiles: ‘basic’ molecular problems of pH tolerance and bioenergetics. Mol. Microbiol. 15, 403–410. doi: 10.1111/j.1365-2958.1995.tb02253.x
Lane, N., and Martin, W. F. (2012). The origin of membrane bioenergetics. Cell 151, 1406–1416. doi: 10.1016/j.cell.2012.11.050
Lewis, R. J., Belkina, S., and Krulwich, T. A. (1980). Alkalophiles have much higher cytochrome contents than conventional bacteria and than their own non-alkaliphilic mutant derivatives. Biochem. Biophys. Res. Commun. 95, 857–863. doi: 10.1016/0006-291X(80)90866-9
Louro, R. O., Catario, T., LaGall, J., and Xavier, A. V. (1997). Redox-Bohr effect in electron/proton energy transduction: cytochrome c3 coupled to hydrogenase works as a ‘proton thruster’ in Desulfivibrio vulgais. J. Biol. Inorg. Chem. 2, 488–491. doi: 10.1007/s007750050160
Lyu, H., and Lazár, D. (2017). Modeling the light-induced electric potential difference (ΔΨ), the pH difference (ΔpH) and the proton motive force across the thylakoid membrane in C3 leaves. J. Theor. Biol. 413, 11–23. doi: 10.1016/j.jtbi.2016.10.017
Matsuno, T., Mie, Y., Yoshimune, K., and Yumoto, I. (2009). Physiological role and redox properties of a small cytochrome c5, cytochrome c-552, from alkaliphile, Pseudomonas alcaliphila AL15-21T. J. Biosci. Bioeng. 108, 465–470. doi: 10.1016/j.jbiosc.2009.06.008
Matsuno, T., Morishita, N., Yamazaki, K., Inoue, N., Sato, Y., Ichise, N., et al. (2007). Cytochrome c-552 from gram-negative alkaliphilic Pseudomonas alcaliphila AL15-21T alters the redox properties at high pH. J. Biosci. Bioeng. 103, 247–254. doi: 10.1263/jbb.103.247
Matsuno, T., Yoshimune, K., and Yumoto, I. (2011). Physiological function of soluble cytochrome c-552 from alkaliphilic Pseudomonas alcaliphila AL15-21T. J. Bioeng. Biomembr. 43, 473–481. doi: 10.1007/s10863-011-9376-1
Matsuno, T., and Yumoto, I. (2015). Bioenergetics and the role of soluble cytochromes c for alkaline adaptation in Gram-negative alkaliphilic Pseudomonas. Bio. Med. Res. Int. 14:847945. doi: 10.1155/2015/847945
Messias, A. C., Aguiar, A. P., Brennan, L., Salgueiro, C. A., Saraiva, L. M., Xavier, A. V., et al. (2006). Solution structures of tetrahaem ferricytochrome c3 from Desulfovibrio vulgaris (Hildenborough) and its K45Q mutant: the molecular basis of cooperativity. Biochim. Biophys. Acta 1757, 143–153. doi: 10.1016/j.bbabio.2006.01.007
Mitchell, P. (1961). Coupling of phosphorylation to electron and hydrogen transfer by a chemi-osmotic type of mechanism. Nature 191, 144–148. doi: 10.1038/191144a0
Moore, G. R., and Pettigrew, G. W. (1990). Cytochrome c: Evolutionary, Structural and Physicochemical Aspects. Belin: Springer-Verlag. doi: 10.1007/978-3-642-74536-2
Mulkidjanian, A. Y., Heberle, J., and Cherepanov, D. V. (2006). Protons @ interfaces: implications for biological energy conversion. Biochim. Biophys. Acta 1757, 913–930. doi: 10.1016/j.bbabio.2006.02.015
Murgida, D. H., and Hildebrandt, P. (2001). Proton-coupled electron transfer of cytochrome c. J. Am. Chem. Soc. 123, 4062–4068. doi: 10.1021/ja004165j
Murgida, D. H., and Hildebrandt, P. (2004). Electron-transfer processes of cytochrome c at interfaces. New insights by surface-enhanced resonance Raman spectroscopy. Acc. Chem. Res. 47, 854–861. doi: 10.1021/ar0400443
Nielsen, P., Fritze, D., and Priest, F. G. (1995). Phenetic diversity of alkaliphilic Bacillus strains: proposal for nine new species. Microbiology 141, 1745–1761. doi: 10.1099/13500872-141-7-1745
Ogami, S., Higikata, S., Tsukahara, T., Mie, Y., Matsuno, T., Morita, N., et al. (2009). A novel membrane-anchored cytochrome c-550 of alkaliphilic Bacillus clarkii K24-1U: expression, molecular features and properties of redox potential. Extremophiles 13, 491–504. doi: 10.1007/s00792-009-0234-6
Orii, Y., Yumoto, I., Fukumori, Y., and Yamanaka, T. (1991). Stopped-flow and rapid-scan studies of the redox behavior of cytochrome aco from faculataive alkalophilc Bacillus. J. Biol. Chem. 266, 14310–14316.
Padan, E., Bibi, E., Ito, M., and Krulwich, T. A. (2005). Alkaline pH homeostasis in bacteria: new insight. Biochim. Biophys. Acta 1717, 67–88. doi: 10.1016/j.bbamem.2005.09.010
Pettigrew, G. W., and Moore, G. R. (1987). Cytochrome c: Biological Aspects. Berlin: Springer-Verlag. doi: 10.1007/978-3-642-72698-9
Qureshi, M. H., Yumoto, I., Fujiwara, T., Fukumori, Y., and Yamanaka, T. (1990). A novel aco-type cytochrome-c oxidase from a facultative alkalophilic Bacillus: purification, and some molecular and enzymatic features. J. Biochem. 107, 480–485. doi: 10.1093/oxfordjournals.jbchem.a123071
Rivas, L., Soares, C. M., Baptista, A. M., Simaan, J., Di Palo, R. E., Murgida, D. H., et al. (2005). Electric-field-induced redox potential shifts of tetraheme cytochrome c3 immobilized on self-assembled monolayers: surface-enhanced resonance Raman spectroscopy and simulation studies. Biophys. J. 88,4188–4199. doi: 10.1529/biophysj.104.057232
Russell, M. J., and Hall, A. J. (1997). The emergence of life from iron monosulphide bubbles at a submarine hydrothermal redox and pH front. J. Geol. Soc. London 154, 377–402. doi: 10.1144/gsjgs.154.3.0377
Sjöholm, J., Bergstrand, J., Nilsson, T., Šachl, R., Christoph, C. V., von Ballmoos, C., et al. (2017). The lateral distance between a proton pump and ATP synthase determines the ATP-synthesis rate. Sci. Rep. 7:2926. doi: 10.1038/s41598-017-02836-4
Sone, N., and Toh, H. (1994). Membrane-bound Bacillus cytochromes c and their phylogenetic position among bacterial class I cytochromes c. FEMS Microbiol. Lett. 122, 203–210. doi: 10.1111/j.1574-6968.1994.tb07168.x
Sone, N., Tsukita, S., and Sakamoto, J. (1999). Direct correlation between proton translocation and growth yield: an analysis of the respiratory chain of Bacillus stearothermophilus. J. Biosci. Bioeng. 87, 495–499. doi: 10.1016/S1389-1723(99)80099-1
Thongaram, T., Kosono, S., Ohkuma, M., Hongoh, Y., Kitada, M., Yoshinaka, T., et al. (2003). Gut of higher termites as a niche for alkaliphiles as shown by culture-based and culture-Independent studies. Microbes. Environ. 18, 152–159. doi: 10.1264/jsme2.18.152
Tsujii, K. (2002). Donnan equilibria in microbial cell walls: a pH-homeostatic mechanism in alkaliphiles. Coll. Surf. B Biointerfaces 24, 247–251. doi: 10.1016/S0927-7765(01)00244-2
Vedder, A. (1934). Bacillus alcalophilus n. sp benevens enkele ervaringen met sterk alcalische voedingsbodems. Antonie Leeuwenhoek 1, 141–147. doi: 10.1007/BF02543931
von Wachenfeldt, C., and Hederstedt, L. (1990). Bacillus subtilis 13-kilodalton cytochrome c-550 encoded by cccA consists of a membrane-anchor and a heme domain. J. Biol. Chem. 265, 13939–13948.
von Wachenfeldt, C., and Hederstedt, L. (1993). Physico-chemical characterisation of membrane-bound and water-soluble forms of Bacillus subtilis cytochrome c-550. Eur. J. Biochem. 212, 499–509. doi: 10.1111/j.1432-1033.1993.tb17687.x
Xiong, J.-W., Zhu, L., Jiao, X., and Liu, S.-S. (2010). Evidence for ΔpH surface component (ΔpHs) of proton motive force in ATP synthesis of mitochondria. Biochim. Biophys. Acta 1800, 213–222. doi: 10.1016/j.bbagen.2009.07.032
Yamanaka, T. (1992). The Biochemistry of Bacterial Cytochromes. Tokyo: Japan Scientific Society Press, 91–168.
Yoshimune, K., Morimoto, H., Hirano, Y., Sakamoto, J., Matsumoto, H., and Yumoto, I. (2010). The obligate alkaliphile Bacillus clarkii K24-1U retains extruded protons at the beginning of respiration. J. Bioeng. Biomembr. 42, 111–116. doi: 10.1007/s10863-010-9278-7
Yumoto, I. (2002). Bioenergetics of alkaliphilic Bacillus spp. J. Biosci. Bioeng. 93, 343–353. doi: 10.1016/S1389-1723(02)80066-4
Yumoto, I. (2003). Electron transport system in alkaliphilic Bacillus spp. Recent Res. Dev. Bacteriol. 1, 131–149.
Yumoto, I., Fukumori, Y., and Yamanaka, T. (1991). Purification and characterization of two membrane-bound c-type cytochromes from a facultative alkalophilic Bacillus. J. Biochem. 110, 267–273. doi: 10.1093/oxfordjournals.jbchem.a123569
Yumoto, I., Nakajima, K., and Ikeda, K. (1997). Comparative study on cytochrome content of alkaliphilic Bacillus strains. J. Ferment. Bioeng. 83, 466–469. doi: 10.1016/S0922-338X(97)83002-4
Yumoto, I., Takahashi, S., Kitagawa, T., Fukumori, Y., and Yamanaka, T. (1993). The molecular features and catalytic activity of CuA-containing aco3-type cytochrome c oxidase from a facultative alkalophilic Bacillus. J. Biochem. 114, 88–95. doi: 10.1093/oxfordjournals.jbchem.a124145
Yumoto, I., Yamazaki, K., Hishinuma, M., Nodasaka, Y., Inoue, N., and Kawasaki, K. (2000). Identification of facultatively alkaliphilic Bacillus sp. strain YN-2000 and its fatty acid composition and cell-surface aspects depending on culture pH. Extremophiles 4, 285–290. doi: 10.1007/s007920070015
Yumoto, I., Yamazaki, K., Hishinuma, M., Nodasaka, Y., Suemori, A., Nakajima, K., et al. (2001). Pseudomonas alcaliphila sp. nov., a novel facultatively psychrophilic alkaliphile isolated from seawater. Int. J. Syst. Evol. Microbiol. 51, 349–355. doi: 10.1099/00207713-51-2-349
Keywords: alkaliphilic, bioenergetic mechanism, cytochrome c, membrane electrical potential, Donnan effect, proton condenser, Bacillus, Pseudomonas
Citation: Matsuno T, Goto T, Ogami S, Morimoto H, Yamazaki K, Inoue N, Matsuyama H, Yoshimune K and Yumoto I (2018) Formation of Proton Motive Force Under Low-Aeration Alkaline Conditions in Alkaliphilic Bacteria. Front. Microbiol. 9:2331. doi: 10.3389/fmicb.2018.02331
Received: 29 May 2018; Accepted: 11 September 2018;
Published: 02 October 2018.
Edited by:
Masahiro Ito, Toyo University, JapanReviewed by:
Jun Liu, Tianjin Institute of Industrial Biotechnology (CAS), ChinaSaori Kosono, The University of Tokyo, Japan
Copyright © 2018 Matsuno, Goto, Ogami, Morimoto, Yamazaki, Inoue, Matsuyama, Yoshimune and Yumoto. This is an open-access article distributed under the terms of the Creative Commons Attribution License (CC BY). The use, distribution or reproduction in other forums is permitted, provided the original author(s) and the copyright owner(s) are credited and that the original publication in this journal is cited, in accordance with accepted academic practice. No use, distribution or reproduction is permitted which does not comply with these terms.
*Correspondence: Isao Yumoto, aS55dW1vdG9AYWlzdC5nby5qcA==