- 1Division of Infectious Diseases, School of Medicine, Johns Hopkins University, Baltimore, MD, United States
- 2Division of Pulmonary and Critical Care Medicine, School of Medicine, Johns Hopkins University, Baltimore, MD, United States
β-lactams, the most widely used class of antibiotics, are well-tolerated, and their molecular mechanisms of action against many bacteria are well-documented. Mycobacterium abscessus (Mab) is a highly drug-resistant rapidly-growing nontuberculous mycobacteria (NTM). Only in recent years have we started to gain insight into the unique relationship between β-lactams and their targets in Mab. In this mini-review, we summarize recent findings that have begun to unravel the molecular basis for overall efficacy of β-lactams against Mab and discuss emerging evidence that indicates that we have yet to harness the full potential of this antibiotic class to treat Mab infections.
Introduction
Although Mycobacterium abscessus (Mab) was first discovered in 1953 (Moore and Frerichs, 1953), it was only recently that genomic sequencing differentiated the Mab complex into three subspecies: M. abscessus sensu stricto, M. abscessus subsp. bolletii, and M. abscessus subsp. massiliense (Adekambi et al., 2004, 2006; Viana-Niero et al., 2008). These subspecies exhibit differential susceptibilities to certain antibiotics and differential clinical outcomes.
Mab can cause pulmonary disease in addition to skin and soft tissue infections, lymphadenitis, and disseminated disease. Mab is sometimes considered a respiratory colonizer; however, in the setting of immunosuppression or structural lung disease, such as cystic fibrosis (CF) and bronchiectasis, Mab can cause chronic pulmonary disease. In CF patients, Mab infections are often incurable and associated with rapid lung function decline (Griffith et al., 2007; Esther et al., 2010; Benwill and Wallace, 2014). The cure rate for Mab lung disease is only 30–50% (Jarand et al., 2011), with a recent review reporting sputum culture conversion rates as low as 25% with antibiotic treatment alone (Diel et al., 2017).
Poor treatment outcomes of Mab infection have been ascribed to both innate and acquired drug resistance. Mab is intrinsically resistant to multiple antibiotic classes which has been attributed to various factors (Brown-Elliott and Wallace, 2002; Nessar et al., 2012; van Ingen et al., 2012). Acquired resistance has further limited therapeutic options (Flume, 2016). Current treatment regimens are suboptimal, as they require several months of intravenous multidrug therapy with potentially cytotoxic antibiotics and produce poor outcomes (Wallace et al., 1985; Floto et al., 2016).
In this review, we will briefly summarize Mab treatment recommendations, discuss unique molecular targets of β-lactams in Mab, and highlight emerging insights into how β-lactams may be leveraged to treat individuals infected with Mab.
Current Mab Treatment Recommendations
The US Cystic Fibrosis Foundation and European Cystic Fibrosis Society recently developed consensus guidelines for management of Mab lung disease in CF patients (Floto et al., 2016). Similar to tuberculosis, Mab infection is treated with multidrug regimens divided into an intensive phase, followed by a continuation phase. Per recent guidelines, the intensive phase of Mab therapy should consist of an oral macrolide, combined with 3–12 weeks of intravenous amikacin, plus at least one of the following: intravenous cefoxitin, imipenem, or tigecycline (Floto et al., 2016). Guidelines for the continuation phase include a daily oral macrolide, inhaled amikacin, and two to three additional oral antibiotics, including minocycline, clofazimine, moxifloxacin, and linezolid.
Macrolides have historically been considered the backbone of treatment against Mab. They have relatively low toxicity, are orally bioavailable (Griffith et al., 2007; Floto et al., 2016), and exhibit consistent activity against Mab in vitro (Griffith et al., 2007). However, subspecies abscessus and bolletii harbor a functional erm(41) gene, which confers inducible macrolide resistance and can limit the effectiveness of this drug class. In contrast, subspecies massiliense carries a non-functional erm(41) gene (Nash et al., 2009), thus cannot exhibit inducible macrolide resistance and is associated with improved outcomes on macrolide-based regimens (Koh et al., 2011). Consequently, the CF guidelines recommend subspeciation of Mab complex, which many clinical laboratories are not equipped to perform routinely. Therefore, some CF centers prescribe initial treatment regimens comprised of intravenous amikacin plus either cefoxitin or imipenem, rather than a macrolide (Philley et al., 2016).
Cefoxitin and imipenem are currently the only two β-lactams included in the guidelines for treatment of Mab infections. This antibiotic class has been largely understudied against Mab and may be a potential untapped resource in combating this highly-resistant microbe.
Mechanism of Action of β-LACTAMS Against Mab
β-lactams are the most widely-used antibiotic class to treat bacterial infections (Hamad, 2010) and their safety and efficacy profiles have been well-established. There are five subclasses of β-lactams currently available in the clinical setting: penicillins, cephalosporins, monobactams, carbapenems, and penems. β-lactams have been studied extensively for treatment of drug-resistant Mycobacterium tuberculosis (Mtb) infections, which is summarized elsewhere (Story-Roller and Lamichhane, 2018). Certain β-lactam subclasses also exhibit activity against Mab (Lavollay et al., 2014; Kaushik et al., 2015; Lefebvre et al., 2016). While initial insights into the molecular mechanism of action of β-lactams against mycobacteria were gleaned largely from Mtb, recent studies have begun to elucidate the relationship between Mab and β-lactams (Lavollay et al., 2014; Lefebvre et al., 2016; Kumar et al., 2017a).
β-lactams exert their activity by inhibiting synthesis of an essential component of the bacterial cell wall, the peptidoglycan (PG) (Hartmann et al., 1972). The building block of PG is a disaccharide with a stem peptide comprised of four or five amino acids; specifically N-acetyl-glucosamine-N-acetyl-muramic acid-l-alanyl-d-glutaminyl-meso-diaminopimelyl-d-alanyl-d-alanine in Mab (Lavollay et al., 2011). Polymerization of disaccharides by transglycosylases and stem peptides by transpeptidases produces a three-dimensional macromolecule, the PG (Figure 1).
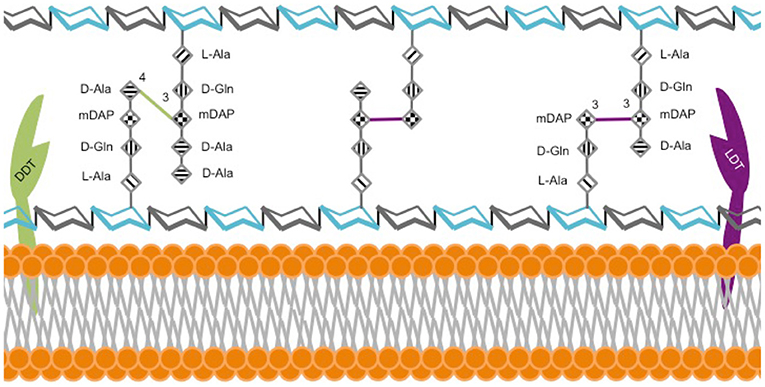
Figure 1. Model of M. abscessus peptidoglycan. The hexagonal structures depict sugars N-acetylglucosamine (gray) and N-acetylmuramic acid (cyan). L-alanine (L-Ala), D-glutamine (D-Gln), meso-diaminopimelic acid (m-DAP) and D-alanine (D-Ala).
The dominant model of PG architecture was largely established by studies using model organisms, such as E. coli. According to this historical model, the final step of PG synthesis is catalyzed by D,D-transpeptidases (DDT), also known as penicillin binding proteins, which link the 4th amino acid of one stem peptide to the 3rd amino acid of the adjacent stem peptide, thereby generating a 4 → 3-linked peptide network. However, as early as 1974, it became clear that the chemical architecture of mycobacterial PG, and therefore the enzymes necessary for its synthesis, were distinct from those described in the historical model. This study reported that stem peptides in the PG of M. smegmatis, Mtb, and M. bovis BCG were predominantly cross-linked with non-canonical linkages between the 3rd amino acid of one peptide and the 3rd amino acid of another (Wietzerbin et al., 1974). That same year, this group also demonstrated that the enzyme, L,D-transpeptidase (LDT), generated these 3 → 3 linkages in Streptococcus faecalis (Coyette et al., 1974).
The first direct evidence demonstrating that stem peptides in Mab PG are predominantly cross-linked by 3 → 3 linkages was reported in 2011 (Lavollay et al., 2011). Subsequently, five putative LDTs, LdtMab1−5, were identified in Mab (Mattoo et al., 2017) and the first crystal structure of one of these enzymes, LdtMab2, was described (Kumar et al., 2017a). These studies confirmed that Mab utilizes both LDTs and DDTs to generate 3 → 3 and 4 → 3 linkages between stem peptides, respectively (Figure 1). The majority of linkages in Mab are 3 → 3, which suggests that LDTs are at least as important as DDTs for synthesis of its PG. Several studies report that genes involved in PG synthesis and remodeling are largely conserved across mycobacteria, implying a similar PG chemical composition, architecture, and metabolism (Sanders et al., 2014; Mattoo et al., 2017). A review of PG biosynthesis in Mtb by Pavelka et al. is recommended for further insight into mycobacterial PG biology (Pavelka et al., 2014).
LDTs are Preferentially Inhibited by Carbapenems and Cephalosporins
β-lactams mimic the C-terminal end of the native stem peptide of PG, bind to the active site of transpeptidases, and irreversibly inhibit their enzymatic activity (Park and Strominger, 1957). As the historical model considered DDTs to be the only enzymes that synthesized PG, they were assumed to be the sole targets of β-lactams. The discovery of LDTs (Mainardi et al., 2005) prompted inquiry into whether β-lactams also interacted with this enzyme class. Subsequent studies have demonstrated that LDTs and DDTs of mycobacteria differ in their binding affinities to β-lactam subclasses and are consequently inhibited by different subclasses to varying degrees (Dubee et al., 2012; Kumar et al., 2017b). DDTs are effectively bound and inhibited by all β-lactam subclasses, whereas Mab LDTs are preferentially bound and inhibited by carbapenems and to a lesser extent by cephalosporins (Kumar et al., 2017a,b).
Although the crystal structures of LDTs of Mab bound to β-lactams are not yet available, several groups have reported crystal structures of LDTs of Mtb bound to carbapenems and penems (Kim et al., 2013; Li et al., 2013; Bianchet et al., 2017; Kumar et al., 2017b; Steiner et al., 2017). As LDTs and DDTs of Mab are differentially inhibited by β-lactam subclasses, comprehensive inhibition of PG synthesis will likely require simultaneous administration of multiple β-lactams belonging to different subclasses to optimally inhibit the two enzyme classes.
Factors That Determine Potency of β-LACTAMS Against Mab
The major molecular factors limiting effectiveness of β-lactams against Mab are β-lactamase activity and the bacterial cell wall. Factors that commonly affect other antibiotic classes, including poor permeability of the cellular envelope, low affinity of antibiotic targets, drug efflux pumps, and chromosomally-encoded neutralizing enzymes, have been elegantly summarized elsewhere (Nessar et al., 2012; van Ingen et al., 2012).
β-Lactamases
The potent activity of the chromosomally-encoded β-lactamase, BlaMab, is primarily responsible for poor efficacy of β-lactams against Mab (Soroka et al., 2014). β-lactamases hydrolyze the β-lactam ring, thereby inactivating these antibiotics (Kasik et al., 1971). Not only does BlaMab degrade several β-lactams with significantly higher efficiency than BlaC of Mtb, BlaMab is not effectively inhibited by common β-lactamase inhibitors (BLI) clavulanate, tazobactam, and sulbactam (Soroka et al., 2017); agents that inhibit BlaC of Mtb (Wang et al., 2006). The observation that these BLIs do not reduce the minimum inhibitory concentration (MIC) of β-lactams against Mab in a whole-cell assay (Kaushik et al., 2017) is additional confirmation that β-lactamase activity in Mab is more robust than in Mtb. Subspecies massiliense harbors an additional β-lactamase, BlaMmas (Ramirez et al., 2017).
BlaMab is inactivated by avibactam (Dubee et al., 2015a), a recently-developed BLI whose core chemical composition differs from older BLIs and lacks a β-lactam ring (Coleman, 2011). Observations that avibactam reduces the MIC of several β-lactams against Mab provides further validation of its efficacy against both the BlaMab protein and whole-cell Mab (Dubee et al., 2015a; Kaushik et al., 2017; Lefebvre et al., 2017). A recent study showed avibactam not only inhibits β-lactamases but also inhibits LDTs (Edoo et al., 2018). A recombinant Mab strain lacking blaMab exhibited increased sensitivity to β-lactams and was rendered susceptible to amoxicillin and ceftaroline (Lefebvre et al., 2016). This study also observed that β-lactams plus avibactam exhibited similar efficacy against the parental Mab strain as compared to each drug alone against ΔblaMab, suggesting that avibactam fully inhibits BlaMab. While BlaMab and BlaMmas hydrolyze penicillins and cephalosporins with similar efficacy, BlaMmas also exhibits mild carbapenemase activity, a potential concern as it suggests an evolutionary movement toward β-lactamases with extended spectra (Ramirez et al., 2017). This study also noted that BlaMmas is structurally similar to other acquired carbapenemases normally found in gram negative bacteria, such as KPC-2 and SFC-1. Avibactam activity against BlaMmas has not yet been assessed and further study is warranted.
Cell Wall
Mycobacteria possess an unusually thick cell wall composed of layers of complex hydrophobic molecules including fatty acids, mycolic acids, lipoproteins, glycopeptidolipids (GPL), and largely insoluble PG and arabinogalactan layers. Although poorly-understood in Mab, epigenetic factors generating differential levels of these molecules, especially GPLs, are associated with two distinct colony morphotypes—rough and smooth—within a clonal population. The rough morphotype tends to be associated with higher rates of antimicrobial resistance, including against β-lactams (Cangelosi et al., 1999; Greendyke and Byrd, 2008; Lavollay et al., 2014). Additionally, glycosylation of lipoproteins limits permeability of the cell wall to antibiotics that inhibit PG synthesis (Becker et al., 2017). Cell wall porins are also partially responsible for β-lactam resistance, as they allow transport of small hydrophilic molecules across the membrane, which interact with targets within the cytoplasm to potentially activate expression of drug resistance genes (Nguyen and Thompson, 2006; Nessar et al., 2012).
Activity of β-LACTAMS Against Mab
We identified thirty-five studies with documented MIC ranges of β-lactams against clinical isolates of Mab globally (Table 1). These data serve to highlight the high degree of variability in observed MIC ranges among clinical isolates, even within each study, and this variability is partially why standardized treatment regimens against Mab are often not practical in the clinical setting. Imipenem and cefoxitin were the most commonly-tested β-lactams and nearly all studies included Mab strains that were resistant to these agents based on established MIC breakpoints (Woods et al., 2011). Only in four studies were all strains susceptible or intermediate to cefoxitin (Lee et al., 2012; Lavollay et al., 2014; Singh et al., 2014; Jeong et al., 2017). Two studies performed subspeciation and observed that all strains of subspecies massiliense and/or bolletii were either susceptible or intermediate to imipenem, whereas subspecies abscessus exhibited higher MICs to this drug (Lavollay et al., 2014; Singh et al., 2014). The reason for this is not currently known. Although seventeen studies also evaluated additional β-lactams, it is evident that this antibiotic class is largely understudied against Mab.
Further Potentiation of β-LACTAMS Against Mab by BLIs
Several studies have investigated the ability of BLIs to potentiate β-lactams against Mab, both in vitro and in vivo. The combination of amoxicillin and avibactam effectively reduced abscess formation and prolonged survival of zebrafish infected with Mab reference strain ATCC 19977 compared to amoxicillin alone (Dubee et al., 2015a). A subsequent study found that a combination of imipenem and avibactam also prolonged zebrafish survival compared to imipenem alone (Lefebvre et al., 2017). Avibactam also decreases the MIC of ceftaroline against Mab (Dubee et al., 2015b). Combinations of carbapenems and avibactam against clinical isolates of Mab showed that avibactam reduced MICs to therapeutically-achievable levels (Kaushik et al., 2017). The greatest MIC reductions were noted with tebipenem, ertapenem, and panipenem; demonstrating that avibactam can successfully overcome β-lactamase activity and further suggests that carbapenems, especially those developed after imipenem, such as doripenem, biapenem and tebipenem, have untapped potential for use against Mab (Kaushik et al., 2017).
Synergy Studies with β-LACTAMS and Other Drugs
As combination regimens are essential for clinical management of Mab infections, several studies have evaluated antibiotic synergy against Mab with mixed results (Cremades et al., 2009; Shen et al., 2010; Bastian et al., 2011; Choi et al., 2012; van Ingen et al., 2012; Oh et al., 2014; Singh et al., 2014; Ferro et al., 2016; Mukherjee et al., 2017; Aziz et al., 2018; Pryjma et al., 2018; Schwartz et al., 2018; Zhang et al., 2018). In vitro studies have shown variable synergy of β-lactams in combination with other drugs. One study found no evidence of synergy among combinations of either imipenem or ertapenem with various other antibiotics (Cremades et al., 2009). However, another study reported high levels of synergy against Mab clinical isolates when clofazimine and amikacin were combined with several β-lactam subclasses (Schwartz et al., 2018). In a final study, rifampin combined with either doripenem or biapenem significantly reduced the MICs of both drugs to within therapeutic levels, compared with each carbapenem alone (Kaushik et al., 2015).
Dual β-LACTAMS for Mab
Given that different subclasses of β-lactams target distinct aspects of mycobacterial cell wall biosynthesis, Mab regimens that contain two β-lactams from different subclasses may have high efficacy in Mab. As mentioned above, mycobacterial DDTs are inhibited by all β-lactams, whereas LDTs are preferentially inhibited by carbapenems and cephalosporins (Kumar et al., 2017a,b). A combination of cefdinir and doripenem was observed to be synergistic against Mab 19977 (Kumar et al., 2017a), demonstrating that dual β-lactams have therapeutic potential against Mab. This promising finding warrants further investigation into the effects of dual β-lactams against clinical isolates of Mab, further potentiation with BLIs, and additional in vivo studies.
Preclinical Models and Clinical Trials
At least two groups have taken initiatives to develop animal models of Mab infection (Lerat et al., 2014; Obregon-Henao et al., 2015). Two studies have assessed efficacy of antibiotic treatment of mice infected with Mab, one of which included a β-lactam, cefoxitin. Lerat et al. assessed regimens containing clarithromycin, amikacin, or cefoxitin monotherapy vs. a three-drug combination in nude mice infected with Mab ATCC 19977. Cefoxitin monotherapy was equally effective as triple therapy, resulting in prolonged survival and reduced splenic bacillary loads compared to untreated controls (Lerat et al., 2014). Several clinical trials assessing efficacy of non-β-lactam antibiotics against NTMs have been undertaken (clinicaltrials.gov). To date, there are no published clinical trials that have specifically investigated β-lactams for the treatment of Mab; however, we are hopeful that an increasing awareness of β-lactams as viable treatment options may lead to clinical trials with this class in the future.
Future Directions and Conclusions
There is a dearth of research exploring β-lactams as potential treatments for Mab. Given the increasing prevalence of highly drug-resistant Mab isolates leading to poor clinical outcomes, new therapeutic approaches are needed to adequately treat these infections. Given our understanding of the differential mechanisms of β-lactam subclasses, and the ability of certain BLIs to overcome β-lactamase activity, currently-available β-lactams are a largely untapped resource for Mab treatment. Of the β-lactam subclasses, carbapenems/penems have the greatest activity against Mab, followed by cephalosporins, then penicillins. As noted above (Kumar et al., 2017a), it is likely that combinations of different β-lactam subclasses are required to fully inhibit PG synthesis in Mab. This insight may partially explain why prior studies evaluating β-lactams individually have not shown significant efficacy against this microbe. Further investigation may identify novel treatments utilizing combinations of β-lactams that optimally inhibit the distinct enzymatic targets present in Mab.
Appropriate selection of companion BLIs is another area in which β-lactams can be potentiated for use against Mab. Several studies have demonstrated efficacy of the BLI avibactam in inhibiting BlaMab activity, which is a major factor contributing to the high MIC of most β-lactams against Mab. However, avibactam is currently only available as a coformulated combination with ceftazidime, which itself does not appear to have activity against Mab (Dubee et al., 2015a; Kaushik et al., 2017). If avibactam were to be made available as an individual formulation, this would significantly increase its clinical usefulness, as regimens could be tailored to combine it with any β-lactam shown to be effective against a particular microbe or strain. Recently, two novel carbapenem-BLI combinations have been developed. These are meropenem-vaborbactam, which was recently FDA-approved for use against gram-negative organisms, and imipenem-relebactam, which is currently in phase II clinical trials (Zhanel et al., 2018). There are no published studies assessing efficacy of these BLIs against Mab, but their coformulation with carbapenems may confer greater potential for clinical use and further studies with these drugs are certainly warranted. It is possible that β-lactam-BLI combinations will become integral to effective treatment of drug-resistant Mab in the future. Additional animal studies as well as clinical trials with this drug class will be essential for the development of novel treatment regimens with improved clinical outcomes. Furthermore, repurposing already FDA-approved β-lactams for use against Mab may allow for expedited clinical implementation of regimens that show promise in preclinical models.
Author Contributions
ES-R, EM, KC, and GL discussed relevant literature. ES-R and KC focused on clinical aspects of the literature and EM focused on the basic biology. ES-R, EM, KC, and GL wrote the manuscript.
Conflict of Interest Statement
The authors declare that the research was conducted in the absence of any commercial or financial relationships that could be construed as a potential conflict of interest.
Acknowledgments
This work was supported by the Cystic Fibrosis Foundation grant LAMICH17GO and NIH grant R21 AI121805. ES-R was supported by the NIH T32 AI007291. The content is solely the responsibility of the authors and does not necessarily represent the official views of the Cystic Fibrosis Foundation or the National Institutes of Health.
References
Adekambi, T., Berger, P., Raoult, D., and Drancourt, M. (2006). rpoB gene sequence-based characterization of emerging non-tuberculous mycobacteria with descriptions of Mycobacterium bolletii sp. nov., Mycobacterium phocaicum sp. nov. and Mycobacterium aubagnense sp. nov. Int. J. Syst. Evol. Microbiol. 56, 133–143. doi: 10.1099/ijs.0.63969-0
Adekambi, T., Reynaud-Gaubert, M., Greub, G., Gevaudan, M. J., La Scola, B., Raoult, D., et al. (2004). Amoebal coculture of “Mycobacterium massiliense” sp. nov. from the sputum of a patient with hemoptoic pneumonia. J. Clin. Microbiol. 42, 5493–5501. doi: 10.1128/JCM.42.12.5493-5501.2004
Aziz, D. B., Teo, J. W. P., Dartois, V., and Dick, T. (2018). Teicoplanin – tigecycline combination shows synergy against Mycobacterium abscessus. Front. Microbiol. 9:932. doi: 10.3389/fmicb.2018.00932
Bastian, S., Veziris, N., Roux, A. L., Brossier, F., Gaillard, J. L., Jarlier, V., et al. (2011). Assessment of clarithromycin susceptibility in strains belonging to the Mycobacterium abscessus group by erm(41) and rrl sequencing. Antimicrob. Agents Chemother. 55, 775–781. doi: 10.1128/AAC.00861-10
Becker, K., Haldimann, K., Selchow, P., Reinau, L. M., Dal Molin, M., and Sander, P. (2017). Lipoprotein glycosylation by protein-O-mannosyltransferase (MAB_1122c) contributes to low cell envelope permeability and antibiotic resistance of Mycobacterium abscessus. Front. Microbiol. 8:2123. doi: 10.3389/fmicb.2017.02123
Benwill, J. L., and Wallace, R. J. Jr. (2014). Mycobacterium abscessus: challenges in diagnosis and treatment. Curr. Opin. Infect. Dis. 27, 506–510. doi: 10.1097/QCO.0000000000000104
Bianchet, M. A., Pan, Y. H., Basta, L. A. B., Saavedra, H., Lloyd, E. P., and Lamichhane, G. (2017). Structural insight into the inactivation of Mycobacterium tuberculosis non-classical transpeptidase LdtMt2 by biapenem and tebipenem. BMC Biochem. 18:8. doi: 10.1186/s12858-017-0082-4
Broda, A., Jebbari, H., Beaton, K., Mitchell, S., and Drobniewski, F. (2013). Comparative drug resistance of Mycobacterium abscessus and M. chelonae isolates from patients with and without cystic fibrosis in the United Kingdom. J. Clin. Microbiol. 51, 217–223. doi: 10.1128/JCM.02260-12
Brown-Elliott, B. A., Killingley, J., Vasireddy, S., Bridge, L., and Wallace, R. J. Jr. (2016). In vitro comparison of ertapenem, meropenem, and imipenem against isolates of rapidly growing mycobacteria and nocardia by use of broth microdilution and Etest. J. Clin. Microbiol. 54, 1586–1592. doi: 10.1128/JCM.00298-16
Brown-Elliott, B. A., Mann, L. B., Hail, D., Whitney, C., and Wallace, R. J. Jr. (2012). Antimicrobial susceptibility of nontuberculous mycobacteria from eye infections. Cornea 31, 900–906. doi: 10.1097/ICO.0b013e31823f8bb9
Brown-Elliott, B. A., and Wallace, R. J. Jr. (2002). Clinical and taxonomic status of pathogenic nonpigmented or late-pigmenting rapidly growing mycobacteria. Clin. Microbiol. Rev. 15, 716–746. doi: 10.1128/CMR.15.4.716-746.2002
Candido, P. H., Nunes Lde, S., Marques, E. A., Folescu, T. W., Coelho, F. S., De Moura, V. C., et al. (2014). Multidrug-resistant nontuberculous mycobacteria isolated from cystic fibrosis patients. J. Clin. Microbiol. 52, 2990–2997. doi: 10.1128/JCM.00549-14
Cangelosi, G. A., Palermo, C. O., Laurent, J. P., Hamlin, A. M., and Brabant, W. H. (1999). Colony morphotypes on Congo red agar segregate along species and drug susceptibility lines in the Mycobacterium avium-intracellulare complex. Microbiology 145, 1317–1324. doi: 10.1099/13500872-145-6-1317
Chihara, S., Smith, G., and Petti, C. A. (2010). Carbapenem susceptibility patterns for clinical isolates of Mycobacterium abscessus determined by the Etest method. J. Clin. Microbiol. 48, 579–580. doi: 10.1128/JCM.01930-09
Choi, G. E., Min, K. N., Won, C. J., Jeon, K., Shin, S. J., and Koh, W. J. (2012). Activities of moxifloxacin in combination with macrolides against clinical isolates of Mycobacterium abscessus and Mycobacterium massiliense. Antimicrob. Agents Chemother. 56, 3549–3555. doi: 10.1128/AAC.00685-12
Chua, K. Y., Bustamante, A., Jelfs, P., Chen, S. C., and Sintchenko, V. (2015). Antibiotic susceptibility of diverse Mycobacterium abscessus complex strains in New South Wales, Australia. Pathology 47, 678–682. doi: 10.1097/PAT.0000000000000327
Coleman, K. (2011). Diazabicyclooctanes (DBOs): a potent new class of non-β-lactam β-lactamase inhibitors. Curr. Opin. Microbiol. 14, 550–555. doi: 10.1016/j.mib.2011.07.026
Coyette, J., Perkins, H. R., Polacheck, I., Shockman, G. D., and Ghuysen, J. M. (1974). Membrane-bound DD-carboxypeptidase and LD-transpeptidase of Streptococcus faecalis ATCC 9790. Eur. J. Biochem. 44, 459–468. doi: 10.1111/j.1432-1033.1974.tb03504.x
Cremades, R., Santos, A., Rodriguez, J. C., Garcia-Pachon, E., Ruiz, M., and Royo, G. (2009). Mycobacterium abscessus from respiratory isolates: activities of drug combinations. J. Infect. Chemother. 15, 46–48. doi: 10.1007/s10156-008-0651-Y
Diel, R., Ringshausen, F., Richter, E., Welker, L., Schmitz, J., and Nienhaus, A. (2017). Microbiological and clinical outcomes of treating non-Mycobacterium avium complex nontuberculous mycobacterial pulmonary disease: a systematic review and meta-analysis. Chest 152, 120–142. doi: 10.1016/j.chest.2017.04.166
Dubee, V., Bernut, A., Cortes, M., Lesne, T., Dorchene, D., Lefebvre, A. L., et al. (2015a). β-Lactamase inhibition by avibactam in Mycobacterium abscessus. J. Antimicrob. Chemother. 70, 1051–1058. doi: 10.1093/jac/dku510
Dubee, V., Soroka, D., Cortes, M., Lefebvre, A. L., Gutmann, L., Hugonnet, J. E., et al. (2015b). Impact of β-lactamase inhibition on the activity of ceftaroline against Mycobacterium tuberculosis and Mycobacterium abscessus. Antimicrob. Agents Chemother. 59, 2938–2941. doi: 10.1128/AAC.05080-14
Dubee, V., Triboulet, S., Mainardi, J. L., Etheve-Quelquejeu, M., Gutmann, L., Marie, A., et al. (2012). Inactivation of Mycobacterium tuberculosis L,D-transpeptidase LdtMt1 by carbapenems and cephalosporins. Antimicrob. Agents Chemother. 56, 4189–4195. doi: 10.1128/AAC.00665-12
Edoo, Z., Iannazzo, L., Compain, F., Li De La Sierra Gallay, I., Van Tilbeurgh, H., Fonvielle, M., et al. (2018). Synthesis of avibactam derivatives and activity on β-lactamases and peptidoglycan biosynthesis enzymes of mycobacteria. Chemistry 24, 8081–8086. doi: 10.1002/chem.201800923
Esther, C. R. Jr., Esserman, D. A., Gilligan, P., Kerr, A., and Noone, P. G. (2010). Chronic Mycobacterium abscessus infection and lung function decline in cystic fibrosis. J. Cyst. Fibros. 9, 117–123. doi: 10.1016/j.jcf.2009.12.001
Ferro, B. E., Srivastava, S., Deshpande, D., Pasipanodya, J. G., Van Soolingen, D., Mouton, J. W., et al. (2016). Failure of the amikacin, cefoxitin, and clarithromycin combination regimen for treating pulmonary Mycobacterium abscessus infection. Antimicrob. Agents Chemother. 60, 6374–6376. doi: 10.1128/AAC.00990-16
Floto, R. A., Olivier, K. N., Saiman, L., Daley, C. L., Herrmann, J. L., Nick, J. A., et al. (2016). US cystic fibrosis foundation and European Cystic Fibrosis Society consensus recommendations for the management of non-tuberculous mycobacteria in individuals with cystic fibrosis. Thorax 71, i1–22. doi: 10.1136/thoraxjnl-2015-207360
Flume, P. A. (2016). US cystic fibrosis foundation and European Cystic Fibrosis Society consensus recommendations for the management of non-tuberculous mycobacteria in individuals with cystic fibrosis. J. Cyst. Fibros. 15, 139–140. doi: 10.1016/S1569-1993(16)00018-7
Greendyke, R., and Byrd, T. F. (2008). Differential antibiotic susceptibility of Mycobacterium abscessus variants in biofilms and macrophages compared to that of planktonic bacteria. Antimicrob. Agents Chemother. 52, 2019–2026. doi: 10.1128/AAC.00986-07
Griffith, D. E., Aksamit, T., Brown-Elliott, B. A., Catanzaro, A., Daley, C., Gordin, F., et al. (2007). An official ATS/IDSA statement: diagnosis, treatment, and prevention of nontuberculous mycobacterial diseases. Am. J. Respir. Crit. Care Med. 175, 367–416. doi: 10.1164/rccm.200604-571ST
Harada, T., Akiyama, Y., Kurashima, A., Nagai, H., Tsuyuguchi, K., Fujii, T., et al. (2012). Clinical and microbiological differences between Mycobacterium abscessus and Mycobacterium massiliense lung diseases. J. Clin. Microbiol. 50, 3556–3561. doi: 10.1128/JCM.01175-12
Hartmann, R., Holtje, J. V., and Schwarz, U. (1972). Targets of penicillin action in Escherichia coli. Nature 235, 426–429. doi: 10.1038/235426a0
Hatakeyama, S., Ohama, Y., Okazaki, M., Nukui, Y., and Moriya, K. (2017). Antimicrobial susceptibility testing of rapidly growing mycobacteria isolated in Japan. BMC Infect. Dis. 17:197. doi: 10.1186/s12879-017-2298-8
Heidarieh, P., Mirsaeidi, M., Hashemzadeh, M., Feizabadi, M. M., Bostanabad, S. Z., Nobar, M. G., et al. (2016). In vitro antimicrobial susceptibility of nontuberculous mycobacteria in Iran. Microb. Drug Resist. 22, 172–178. doi: 10.1089/mdr.2015.0134
Huang, T. S., Lee, S. S., Hsueh, P. R., Tsai, H. C., Chen, Y. S., Wann, S. R., et al. (2008). Antimicrobial resistance of rapidly growing mycobacteria in western Taiwan: SMART program 2002. J. Formos. Med. Assoc. 107, 281–287. doi: 10.1016/S0929-6646(08)60088-1
Huang, Y. C., Liu, M. F., Shen, G. H., Lin, C. F., Kao, C. C., Liu, P. Y., et al. (2010). Clinical outcome of Mycobacterium abscessus infection and antimicrobial susceptibility testing. J. Microbiol. Immunol. Infect. 43, 401–406. doi: 10.1016/S1684-1182(10)60063-1
Ito, K., Hashimoto, K., and Ogata, H. (2003). Activity of cephems and carbapenems against clinically isolated Mycobacterium abscessus. Kekkaku 78, 587–590. doi: 10.11400/kekkaku1923.78.587
Jarand, J., Levin, A., Zhang, L., Huitt, G., Mitchell, J. D., and Daley, C. L. (2011). Clinical and microbiologic outcomes in patients receiving treatment for Mycobacterium abscessus pulmonary disease. Clin. Infect. Dis. 52, 565–571. doi: 10.1093/cid/ciq237
Jeon, K., Kwon, O. J., Lee, N. Y., Kim, B. J., Kook, Y. H., Lee, S. H., et al. (2009). Antibiotic treatment of Mycobacterium abscessus lung disease: a retrospective analysis of 65 patients. Am. J. Respir. Crit. Care Med. 180, 896–902. doi: 10.1164/rccm.200905-0704OC
Jeong, S. H., Kim, S. Y., Huh, H. J., Ki, C. S., Lee, N. Y., Kang, C. I., et al. (2017). Mycobacteriological characteristics and treatment outcomes in extrapulmonary Mycobacterium abscessus complex infections. Int. J. Infect. Dis. 60, 49–56. doi: 10.1016/j.ijid.2017.05.007
Kasik, J. E., Severson, C. D., Stearns, N. A., and Thompson, J. S. (1971). Immunologic distinction of mycobacterial β-lactamase. J. Lab. Clin. Med. 78, 982.
Kaushik, A., Gupta, C., Fisher, S., Story-Roller, E., Galanis, C., Parrish, N., et al. (2017). Combinations of avibactam and carbapenems exhibit enhanced potencies against drug-resistant Mycobacterium abscessus. Fut. Microbiol. 12, 473–480. doi: 10.2217/fmb-2016-0234
Kaushik, A., Makkar, N., Pandey, P., Parrish, N., Singh, U., and Lamichhane, G. (2015). Carbapenems and rifampin exhibit synergy against Mycobacterium tuberculosis and Mycobacterium abscessus. Antimicrob. Agents Chemother. 59, 6561–6567. doi: 10.1128/AAC.01158-15
Kim, H. S., Kim, J., Im, H. N., Yoon, J. Y., An, D. R., Yoon, H. J., et al. (2013). Structural basis for the inhibition of Mycobacterium tuberculosis L,D-transpeptidase by meropenem, a drug effective against extensively drug-resistant strains. Acta Crystallogr. D Biol. Crystallogr. 69, 420–431. doi: 10.1107/S0907444912048998
Koh, W. J., Jeon, K., Lee, N. Y., Kim, B. J., Kook, Y. H., Lee, S. H., et al. (2011). Clinical significance of differentiation of Mycobacterium massiliense from Mycobacterium abscessus. Am. J. Respir. Crit. Care Med. 183, 405–410. doi: 10.1164/rccm.201003-0395OC
Kumar, P., Chauhan, V., Silva, J. R. A., Lameira, J., D'andrea, F. B., Li, S. G., et al. (2017a). Mycobacterium abscessus L,D-transpeptidases are susceptible to inactivation by carbapenems and cephalosporins but not penicillins. Antimicrob. Agents Chemother. 61:e00866-17. doi: 10.1128/AAC.00866-17
Kumar, P., Kaushik, A., Lloyd, E. P., Li, S. G., Mattoo, R., Ammerman, N. C., et al. (2017b). Non-classical transpeptidases yield insight into new antibacterials. Nat. Chem. Biol. 13, 54–61. doi: 10.1038/nchembio.2237
Lavollay, M., Dubee, V., Heym, B., Herrmann, J. L., Gaillard, J. L., Gutmann, L., et al. (2014). In vitro activity of cefoxitin and imipenem against Mycobacterium abscessus complex. Clin. Microbiol. Infect. 20, O297–O300. doi: 10.1111/1469-0691.12405
Lavollay, M., Fourgeaud, M., Herrmann, J. L., Dubost, L., Marie, A., Gutmann, L., et al. (2011). The peptidoglycan of Mycobacterium abscessus is predominantly cross-linked by L,D-transpeptidases. J. Bacteriol. 193, 778–782. doi: 10.1128/JB.00606-10
Lee, M. C., Sun, P. L., Wu, T. L., Wang, L. H., Yang, C. H., Chung, W. H., et al. (2017). Antimicrobial resistance in Mycobacterium abscessus complex isolated from patients with skin and soft tissue infections at a tertiary teaching hospital in Taiwan. J. Antimicrob. Chemother. 72, 2782–2786. doi: 10.1093/jac/dkx212
Lee, M. R., Cheng, A., Lee, Y. C., Yang, C. Y., Lai, C. C., Huang, Y. T., et al. (2012). CNS infections caused by Mycobacterium abscessus complex: clinical features and antimicrobial susceptibilities of isolates. J. Antimicrob. Chemother. 67, 222–225. doi: 10.1093/jac/dkr420
Lee, M. R., Ko, J. C., Liang, S. K., Lee, S. W., Yen, D. H., and Hsueh, P. R. (2014). Bacteraemia caused by Mycobacterium abscessus subsp. abscessus and M. abscessus subsp. bolletii: clinical features and susceptibilities of the isolates. Int. J. Antimicrob. Agents 43, 438–441. doi: 10.1016/j.ijantimicag.2014.02.007
Lee, S. M., Kim, J., Jeong, J., Park, Y. K., Bai, G. H., Lee, E. Y., et al. (2007). Evaluation of the broth microdilution method using 2,3-diphenyl-5-thienyl-(2)-tetrazolium chloride for rapidly growing mycobacteria susceptibility testing. J. Korean Med. Sci. 22, 784–790. doi: 10.3346/jkms.2007.22.5.784
Lefebvre, A. L., Dubee, V., Cortes, M., Dorchene, D., Arthur, M., and Mainardi, J. L. (2016). Bactericidal and intracellular activity of β-lactams against Mycobacterium abscessus. J. Antimicrob. Chemother. 71, 1556–1563. doi: 10.1093/jac/dkw022
Lefebvre, A. L., Le Moigne, V., Bernut, A., Veckerle, C., Compain, F., Herrmann, J. L., et al. (2017). Inhibition of the β-lactamase BlaMab by avibactam improves the in vitro and in vivo efficacy of imipenem against Mycobacterium abscessus. Antimicrob. Agents Chemother. 61:e02440-16. doi: 10.1128/AAC.02440-16
Lerat, I., Cambau, E., Roth Dit Bettoni, R., Gaillard, J. L., Jarlier, V., Truffot, C., et al. (2014). In vivo evaluation of antibiotic activity against Mycobacterium abscessus. J. Infect. Dis. 209, 905–912. doi: 10.1093/infdis/jit614
Li, W. J., Li, D. F., Hu, Y. L., Zhang, X. E., Bi, L. J., and Wang, D. C. (2013). Crystal structure of L,D-transpeptidase LdtMt2 in complex with meropenem reveals the mechanism of carbapenem against Mycobacterium tuberculosis. Cell Res. 23, 728–731. doi: 10.1038/cr.2013.53
Li, Y. M., Tong, X. L., Xu, H. T., Ju, Y., Cai, M., and Wang, C. (2016). Prevalence and antimicrobial susceptibility of Mycobacterium abscessus in a General Hospital, China. Biomed. Environ. Sci. 29, 85–90. doi: 10.3967/bes2016.009
Mainardi, J. L., Fourgeaud, M., Hugonnet, J. E., Dubost, L., Brouard, J. P., Ouazzani, J., et al. (2005). A novel peptidoglycan cross-linking enzyme for a β-lactam-resistant transpeptidation pathway. J. Biol. Chem. 280, 38146–38152. doi: 10.1074/jbc.M507384200
Mattoo, R., Lloyd, E. P., Kaushik, A., Kumar, P., Brunelle, J. L., Townsend, C. A., et al. (2017). LdtMav2, a nonclassical transpeptidase and susceptibility of Mycobacterium avium to carbapenems. Fut. Microbiol. 12, 595–607. doi: 10.2217/fmb-2016-0208
Moore, M., and Frerichs, J. B. (1953). An unusual acid-fast infection of the knee with subcutaneous, abscess-like lesions of the gluteal region; report of a case with a study of the organism, Mycobacterium abscessus. J. Invest. Dermatol. 20, 133–169. doi: 10.1038/jid.1953.18
Mougari, F., Amarsy, R., Veziris, N., Bastian, S., Brossier, F., Bercot, B., et al. (2016). Standardized interpretation of antibiotic susceptibility testing and resistance genotyping for Mycobacterium abscessus with regard to subspecies and erm41 sequevar. J. Antimicrob. Chemother. 71, 2208–2212. doi: 10.1093/jac/dkw130
Mukherjee, D., Wu, M. L., Teo, J. W. P., and Dick, T. (2017). Vancomycin and clarithromycin show synergy against Mycobacterium abscessus in vitro. Antimicrob. Agents Chemother. 61:e01298-17. doi: 10.1128/AAC.01298-17
Nash, K. A., Brown-Elliott, B. A., and Wallace, R. J. Jr. (2009). A novel gene, erm(41), confers inducible macrolide resistance to clinical isolates of Mycobacterium abscessus but is absent from Mycobacterium chelonae. Antimicrob. Agents Chemother. 53, 1367–1376. doi: 10.1128/AAC.01275-08
Nessar, R., Cambau, E., Reyrat, J. M., Murray, A., and Gicquel, B. (2012). Mycobacterium abscessus: a new antibiotic nightmare. J. Antimicrob Chemother. 67, 810–818. doi: 10.1093/jac/dkr578
Nguyen, L., and Thompson, C. J. (2006). Foundations of antibiotic resistance in bacterial physiology: the mycobacterial paradigm. Trends Microbiol 14, 304–312. doi: 10.1016/j.tim.2006.05.005
Nie, W., Duan, H., Huang, H., Lu, Y., Bi, D., and Chu, N. (2014). Species identification of Mycobacterium abscessus subsp. abscessus and Mycobacterium abscessus subsp. bolletii using rpoB and hsp65, and susceptibility testing to eight antibiotics. Int. J. Infect. Dis. 25, 170–174. doi: 10.1016/j.ijid.2014.02.014
Obregon-Henao, A., Arnett, K. A., Henao-Tamayo, M., Massoudi, L., Creissen, E., Andries, K., et al. (2015). Susceptibility of Mycobacterium abscessus to antimycobacterial drugs in preclinical models. Antimicrob. Agents Chemother. 59, 6904–6912. doi: 10.1128/AAC.00459-15
Oh, C. T., Moon, C., Park, O. K., Kwon, S. H., and Jang, J. (2014). Novel drug combination for Mycobacterium abscessus disease therapy identified in a Drosophila infection model. J. Antimicrob. Chemother. 69, 1599–1607. doi: 10.1093/jac/dku024
Pang, H., Li, G., Zhao, X., Liu, H., Wan, K., and Yu, P. (2015). Drug susceptibility testing of 31 antimicrobial agents on rapidly growing mycobacteria isolates from China. Biomed. Res. Int. 2015:419392. doi: 10.1155/2015/419392
Park, J. T., and Strominger, J. L. (1957). Mode of action of penicillin. Science 125, 99–101. doi: 10.1126/science.125.3238.99
Park, S., Kim, S., Park, E. M., Kim, H., Kwon, O. J., Chang, C. L., et al. (2008). In vitro antimicrobial susceptibility of Mycobacterium abscessus in Korea. J. Korean Med. Sci. 23, 49–52. doi: 10.3346/jkms.2008.23.1.49
Pavelka, M. S. Jr., Mahapatra, S., and Crick, D. C. (2014). Genetics of peptidoglycan biosynthesis. Microbiol. Spectr. 2:MGM2-0034-2013. doi: 10.1128/microbiolspec.MGM2-0034-2013
Philley, J. V., Degroote, M. A., Honda, J. R., Chan, M. M., Kasperbauer, S., Walter, N. D., et al. (2016). Treatment of non-tuberculous mycobacterial lung disease. Curr. Treat Options Infect. Dis. 8, 275–296. doi: 10.1007/s40506-016-0086-4
Pryjma, M., Burian, J., and Thompson, C. J. (2018). Rifabutin acts in synergy and is bactericidal with frontline Mycobacterium abscessus antibiotics clarithromycin and tigecycline, suggesting a potent treatment combination. Antimicrob. Agents Chemother. 62:e00283-18. doi: 10.1128/AAC.00283-18
Ramirez, A., Ruggiero, M., Aranaga, C., Cataldi, A., Gutkind, G., De Waard, J. H., et al. (2017). Biochemical characterization of β-lactamases from Mycobacterium abscessus complex and genetic environment of the β-lactamase-encoding gene. Microb. Drug Resist. 23, 294–300. doi: 10.1089/mdr.2016.0047
Sanders, A. N., Wright, L. F., and Pavelka, M. S. Jr. (2014). Genetic characterization of mycobacterial L,D-transpeptidases. Microbiology 160, 1795–1806. doi: 10.1099/mic.0.078980-0
Schwartz, M., Fisher, S., Story-Roller, E., Lamichhane, G., and Parrish, N. (2018). Activities of dual combinations of antibiotics against multidrug-resistant nontuberculous mycobacteria recovered from patients with cystic fibrosis. Microb. Drug Resist. doi: 10.1089/mdr.2017.0286. [Epub ahead of print].
Set, R., Rokade, S., Agrawal, S., and Shastri, J. (2010). Antimicrobial susceptibility testing of rapidly growing mycobacteria by microdilution–experience of a tertiary care centre. Indian J. Med. Microbiol. 28, 48–50. doi: 10.4103/0255-0857.58729
Sfeir, M., Walsh, M., Rosa, R., Aragon, L., Liu, S. Y., Cleary, T., et al. (2018). Mycobacterium abscessus complex infections: a retrospective cohort study. Open Forum Infect. Dis. 5:ofy022. doi: 10.1093/ofid/ofy022
Shen, G. H., Wu, B. D., Hu, S. T., Lin, C. F., Wu, K. M., and Chen, J. H. (2010). High efficacy of clofazimine and its synergistic effect with amikacin against rapidly growing mycobacteria. Int. J. Antimicrob. Agents 35, 400–404. doi: 10.1016/j.ijantimicag.2009.12.008
Singh, S., Bouzinbi, N., Chaturvedi, V., Godreuil, S., and Kremer, L. (2014). In vitro evaluation of a new drug combination against clinical isolates belonging to the Mycobacterium abscessus complex. Clin. Microbiol. Infect. 20, O1124–O1127. doi: 10.1111/1469-0691.12780
Soroka, D., Dubee, V., Soulier-Escrihuela, O., Cuinet, G., Hugonnet, J. E., Gutmann, L., et al. (2014). Characterization of broad-spectrum Mycobacterium abscessus class A β-lactamase. J. Antimicrob. Chemother. 69, 691–696. doi: 10.1093/jac/dkt410
Soroka, D., Ourghanlian, C., Compain, F., Fichini, M., Dubee, V., Mainardi, J. L., et al. (2017). Inhibition of β-lactamases of mycobacteria by avibactam and clavulanate. J. Antimicrob. Chemother. 72, 1081–1088. doi: 10.1093/jac/dkw546
Steiner, E. M., Schneider, G., and Schnell, R. (2017). Binding and processing of β-lactam antibiotics by the transpeptidase LdtMt2 from Mycobacterium tuberculosis. FEBS J. 284, 725–741. doi: 10.1111/febs.14010
Story-Roller, E., and Lamichhane, G. (2018). Have we realized the full potential of β-lactams for treating drug-resistant TB? IUBMB Life 70, 881–888. doi: 10.1002/iub.1875.
Tang, S. S., Lye, D. C., Jureen, R., Sng, L. H., and Hsu, L. Y. (2015). Rapidly growing mycobacteria in Singapore, 2006–2011. Clin. Microbiol. Infect. 21, 236–241. doi: 10.1016/j.cmi.2014.10.018
van Ingen, J., Boeree, M. J., Van Soolingen, D., and Mouton, J. W. (2012). Resistance mechanisms and drug susceptibility testing of nontuberculous mycobacteria. Drug Resist. Updat 15, 149–161. doi: 10.1016/j.drup.2012.04.001
Viana-Niero, C., Lima, K. V., Lopes, M. L., Rabello, M. C., Marsola, L. R., Brilhante, V. C., et al. (2008). Molecular characterization of Mycobacterium massiliense and Mycobacterium bolletii in isolates collected from outbreaks of infections after laparoscopic surgeries and cosmetic procedures. J. Clin. Microbiol. 46, 850–855. doi: 10.1128/JCM.02052-07
Wallace, R. J. Jr., Swenson, J. M., Silcox, V. A., and Bullen, M. G. (1985). Treatment of nonpulmonary infections due to Mycobacterium fortuitum and Mycobacterium chelonei on the basis of in vitro susceptibilities. J. Infect. Dis. 152, 500–514. doi: 10.1093/infdis/152.3.500
Wang, F., Cassidy, C., and Sacchettini, J. C. (2006). Crystal structure and activity studies of the Mycobacterium tuberculosis β-lactamase reveal its critical role in resistance to β-lactam antibiotics. Antimicrob. Agents Chemother. 50, 2762–2771. doi: 10.1128/AAC.00320-06
Wietzerbin, J., Das, B. C., Petit, J. F., Lederer, E., Leyh-Bouille, M., and Ghuysen, J. M. (1974). Occurrence of D-alanyl-(D)-meso-diaminopimelic acid and meso-diaminopimelyl-meso-diaminopimelic acid interpeptide linkages in the peptidoglycan of Mycobacteria. Biochemistry 13, 3471–3476. doi: 10.1021/bi00714a008
Woods, G. L. B. A, Brown-Elliot, P. S., Conville, E. P., Desmond, G. S., Hall, G., et al. (2011). Susceptibility Testing of Mycobacteria, Nocardiae, and Other Aerobic Actinomycetes, 2nd Edn. Wayne, PA: Clinical and Laboratory Standards Institute.
Woods, G. L., Bergmann, J. S., Witebsky, F. G., Fahle, G. A., Boulet, B., Plaunt, M., et al. (2000). Multisite reproducibility of Etest for susceptibility testing of Mycobacterium abscessus, Mycobacterium chelonae, and Mycobacterium fortuitum. J. Clin. Microbiol. 38, 656–661.
Yang, S. C., Hsueh, P. R., Lai, H. C., Teng, L. J., Huang, L. M., Chen, J. M., et al. (2003). High prevalence of antimicrobial resistance in rapidly growing mycobacteria in Taiwan. Antimicrob. Agents Chemother. 47, 1958–1962. doi: 10.1128/AAC.47.6.1958-1962.2003
Yoshida, S., Tsuyuguchi, K., Suzuki, K., Tomita, M., Okada, M., Hayashi, S., et al. (2013). Further isolation of Mycobacterium abscessus subsp. abscessus and subsp. bolletii in different regions of Japan and susceptibility of these isolates to antimicrobial agents. Int. J. Antimicrob. Agents 42, 226–231. doi: 10.1016/j.ijantimicag.2013.04.029
Zhanel, G. G., Lawrence, C. K., Adam, H., Schweizer, F., Zelenitsky, S., Zhanel, M., et al. (2018). Imipenem-relebactam and meropenem-vaborbactam: two novel carbapenem-β-lactamase inhibitor combinations. Drugs 78, 65–98. doi: 10.1007/s40265-017-0851-9
Zhang, Z., Lu, J., Song, Y., and Pang, Y. (2018). In vitro activity between linezolid and other antimicrobial agents against Mycobacterium abscessus complex. Diagn. Microbiol. Infect. Dis. 90, 31–34. doi: 10.1016/j.diagmicrobio.2017.09.013
Keywords: Mycobacterium abscessus, β-lactams, peptidoglycan, LD-transpeptidase, β-lactamase inhibitor
Citation: Story-Roller E, Maggioncalda EC, Cohen KA and Lamichhane G (2018) Mycobacterium abscessus and β-Lactams: Emerging Insights and Potential Opportunities. Front. Microbiol. 9:2273. doi: 10.3389/fmicb.2018.02273
Received: 30 May 2018; Accepted: 05 September 2018;
Published: 25 September 2018.
Edited by:
Thomas Dick, Rutgers, The State University of New Jersey, Newark, United StatesReviewed by:
Marcel Behr, McGill University, CanadaBavesh Davandra Kana, University of the Witwatersrand, South Africa
Copyright © 2018 Story-Roller, Maggioncalda, Cohen and Lamichhane. This is an open-access article distributed under the terms of the Creative Commons Attribution License (CC BY). The use, distribution or reproduction in other forums is permitted, provided the original author(s) and the copyright owner(s) are credited and that the original publication in this journal is cited, in accordance with accepted academic practice. No use, distribution or reproduction is permitted which does not comply with these terms.
*Correspondence: Gyanu Lamichhane, bGFtaWNoaGFuZUBqaHUuZWR1