- 1Institute for Molecular Biosciences, Goethe University Frankfurt, Frankfurt, Germany
- 2Integrative Fungal Research Cluster, Frankfurt, Germany
- 3Institute of Ecology, Evolution and Diversity, Goethe University Frankfurt, Frankfurt, Germany
The plant-growth modulating effect of microbial volatile organic compounds (VOCs) has been demonstrated repeatedly. This has most often been performed by exposing plants to VOC released by microbes grown on nutrient rich media. Here, we used soil instead to grow fungi of the Fusarium genus and investigate how VOCs emitted by this system influenced the development of Arabidopsis plants. The volatile profiles of Fusarium strains grown in soil and malt extract were also compared. Our results demonstrate that distinct volatile signatures can be attributed to different Fusarium genetic clades but also highlight a major influence of the growth medium on volatile emission. Furthermore, all soil-grown Fusarium isolates increased primary root length in Arabidopsis by decreasing VOC concentrations in soil. This result represents a major paradigm shift in plant-microbe interactions since growth modulating effects have been attributed so far to the emission and not the consumption of volatile signals.
Introduction
Volatile organic compounds (VOCs), small molecules with low boiling point and high vapor pressure, include numerous signals involved in plant-microbial interactions (Junker and Tholl, 2013; Schulz-Bohm et al., 2017). To date, a few thousands VOCs have been described in flowering plants (Knudsen et al., 2006) and microbes (Lemfack et al., 2018). These VOCs predominantly include terpenoids, phenylpropanoids/benzenoids, fatty acids, and amino acid derivatives (Dudareva et al., 2013). By contrast to their well-established functions aboveground (Šimpraga et al., 2016), the role of VOCs released belowground has only started to emerge recently.
Rhizosphere microbes might regulate plant growth by emitting VOCs as demonstrated by Bacillus bacteria (Ryu et al., 2003; Zhang et al., 2007) that promote plant growth through the release of 2,3-butanediol and acetoin (Ryu et al., 2003). Similar growth promotion in Arabidopsis was reported for Trichoderma viride fungi with growth regulators putatively identified as isobutyl alcohol, isopentyl alcohol and 3-methylbutanal (Hung et al., 2013). VOCs emitted by the fungal root pathogen Rhizoctonia solani have also been shown to increase shoot and root biomass in Arabidopsis and at the same time compromising resistance to above-ground herbivory (Cordovez et al., 2017). Microbial VOCs might also inhibit plant growth and affect root development as documented for bacteria (Wenke et al., 2012) and mycorrhizal fungi (Splivallo et al., 2007; Ditengou et al., 2015). These examples illustrate the seemingly wide-spread ability of rhizospheric microbes to regulate plant development through VOCs. Regulation of plant growth by secondary metabolites occurs in a concentration-dependent manner, with promotion typically reported at low concentrations and inhibition at higher concentrations as demonstrated for benzaldehyde derivatives (Choi et al., 2016). Bioactive concentrations mimic hormonal levels as shown for some VOCs (i.e., 13-tetradecadien-1-ol, 3-hydroxy-2-butanone, 2,3-butanediol) that promote plant growth in the ng-μg range for microbes grown on non-soil matrices (Ryu et al., 2003; Zou et al., 2010; Blom et al., 2011; Park et al., 2015). As most media used in previous studies are rich in terms of nutrients, it might be more challenging for organisms growing on soil to produce secondary metabolites. In bulk soil and the rhizosphere, bioactivity will eventually depend on the half-life of specific signaling molecules as well as the ability of the emitter to sustain stable VOCs concentrations over longer periods of time.
In terms of phylogeny, the ability to regulate plant-growth via VOCs seems widespread among diverse microbial species (Sánchez-López et al., 2016) and microbial VOCs might act by modulating the auxin and the cytokinine signaling pathways of plants (Ryu et al., 2003; Zhang et al., 2007; Bitas et al., 2015; Sánchez-López et al., 2016). Volatile signals of Trichoderma fungi have been shown to increase the uptake of iron in roots of Arabidopsis and tomato, resulting in the priming of jasmonic acid signaling pathway in plant shoots (Martínez-Medina et al., 2017). It has also been suggested that microbes occupying particular niches (i.e., rhizosphere) or having specific lifestyles (ectomycorrhizal, pathogenic or saprophytic) might produce distinct ecologically relevant VOCs (Müller et al., 2013; Schenkel et al., 2015). If confirmed, this hypothesis might corroborate the importance of specific VOCs in plant/microbial interactions.
The influence of microbial VOCs on plant growth has been tested in various static and flow-through systems where plants and microbes are physically separated but exchanged VOCs via a common headspace (Kai et al., 2016). Static systems such as a split Petri dish have been widely used, with plants in one compartment and microbes in a second compartment (Blom et al., 2011; Kai et al., 2016; Lazazzara et al., 2017). One shortcoming of the static systems described previously is that they used artificial (nutrient rich) media to grow microbes. VOCs profiles emitted by microbes grown on nutrient rich media might drastically differ from those emitted under more natural conditions (i.e., with microbes grown on soil), which might in turn affect plant phenotype. Thus growth conditions should be carefully selected when investigating potential ecologically relevant signaling compounds. Another shortcoming of static systems is that they are prone to the artificial accumulation of humidity (Tholl and Röse, 2006; Kai et al., 2016) and CO2 (Kai and Piechulla, 2009) that must to be controlled for unbiased results (Piechulla and Schnitzler, 2016). Ultimately, bioactive VOCs must be identified and quantified, and their plant growth regulation ability should be demonstrated with pure compounds in concentrations similar to the ones observed in the assays with plants.
The first aim of our study was to investigate the influence of growth medium (a nutrient rich medium versus soil) on the emission of fungal volatiles. The second aim was to test the outcome of VOC-mediated interactions between plants and soil-grown fungi. For this purpose, we employed Arabidopsis thaliana as a model plant and different species belonging to the Fusarium genus. This genus comprises globally distributed ascomycete fungi of the Hypocreales order (Karim et al., 2016) that range in lifestyle from plant pathogens and saprophytes (Karim et al., 2016) to symptomless endophytes (Maciá-Vicente et al., 2008; Glynou et al., 2016). Members of the Fusarium genus cannot be strictly classified as saprophytes or pathogen since their lifestyle is highly dependent on the colonized host plant and possibly other environmental conditions (Kia et al., 2017; Lofgren et al., 2018). Previous work on F. oxysporum grown on nutrient rich media highlighted its ability to alter plant growth via the emission of VOCs (Bitas et al., 2015). In contrast to the latter study, soil was employed here as substrate for fungal growth. Additionally, further species of the same genus were tested to understand whether general patterns of VOC-mediated growth regulation could be inferred from phylogenetic data.
Materials and Methods
Identification of Fungal Strains
Twenty-eight fungal strains, including twenty-seven Fusarium (Hypocreales) and one unclassified Helotiales strains were used in this study. All strains were isolated as endophytes from the roots of two species of the Brassicaceae family, Microthlaspi perfoliatum and Microthlaspi erraticum originating from several locations in Europe and Turkey (Glynou et al., 2016) (Table 1).
The strains, characterized earlier (Glynou et al., 2016) by internal transcribed spacer (ITS) sequencing, were further characterized here through sequencing of the transcription elongation factor 1-α gene (tef1-α), which provides a better species resolution for Fusarium spp. than the ITS (O’Donnell et al., 2015). Using primers ef1/ef2 and PCR conditions reported earlier (Geiser et al., 2004), the partial tef1-α gene was amplified from fungal DNA (Glynou et al., 2016). The resulting amplicons were purified and sequenced by GATC Biotech AG (Konstanz, Germany). The quality-filtered sequences were first manually compared against the FUSARIUM-ID database (Geiser et al., 2004) using BLAST (Altschul et al., 1990). Then a maximum-likelihood (ML) phylogenetic tree was built alongside sequences from reference strains. To do this, all the strains’ tef1-α sequences were compared using BLAST against the full NCBI GenBank database and against subsets of it containing only sequences from vouchered strains or species type specimens. A maximum of ten BLAST best hit sequences were retrieved from each dataset and aligned with the query sequences applying the G-INS-I algorithm of MAFFT v7.123b (Katoh and Standley, 2013). The alignment was trimmed with Gblocks v0.91b (Castresana, 2000) and then a ML tree was constructed with the program RAxML v8.0.0 (Stamatakis, 2014), using a GTRGAMMA model of nucleotide substitution and rate heterogeneity and 1,000 bootstraps. Another ML tree was likewise built using only the strain sequences, to compare the phylogenetic relationships among strains with their VOC production profiles.
Experimental Design and Preparation of Fungal Inocula
Fungal strains, maintained on corn meal agar (CMA, Sigma-Aldrich, St. Louis, MO, United States) at room temperature, were used in a series of experiments aimed at characterizing VOC profiles and VOC mediated interactions of fungi and plants (see Figure 1 for an overview of the experimental design).
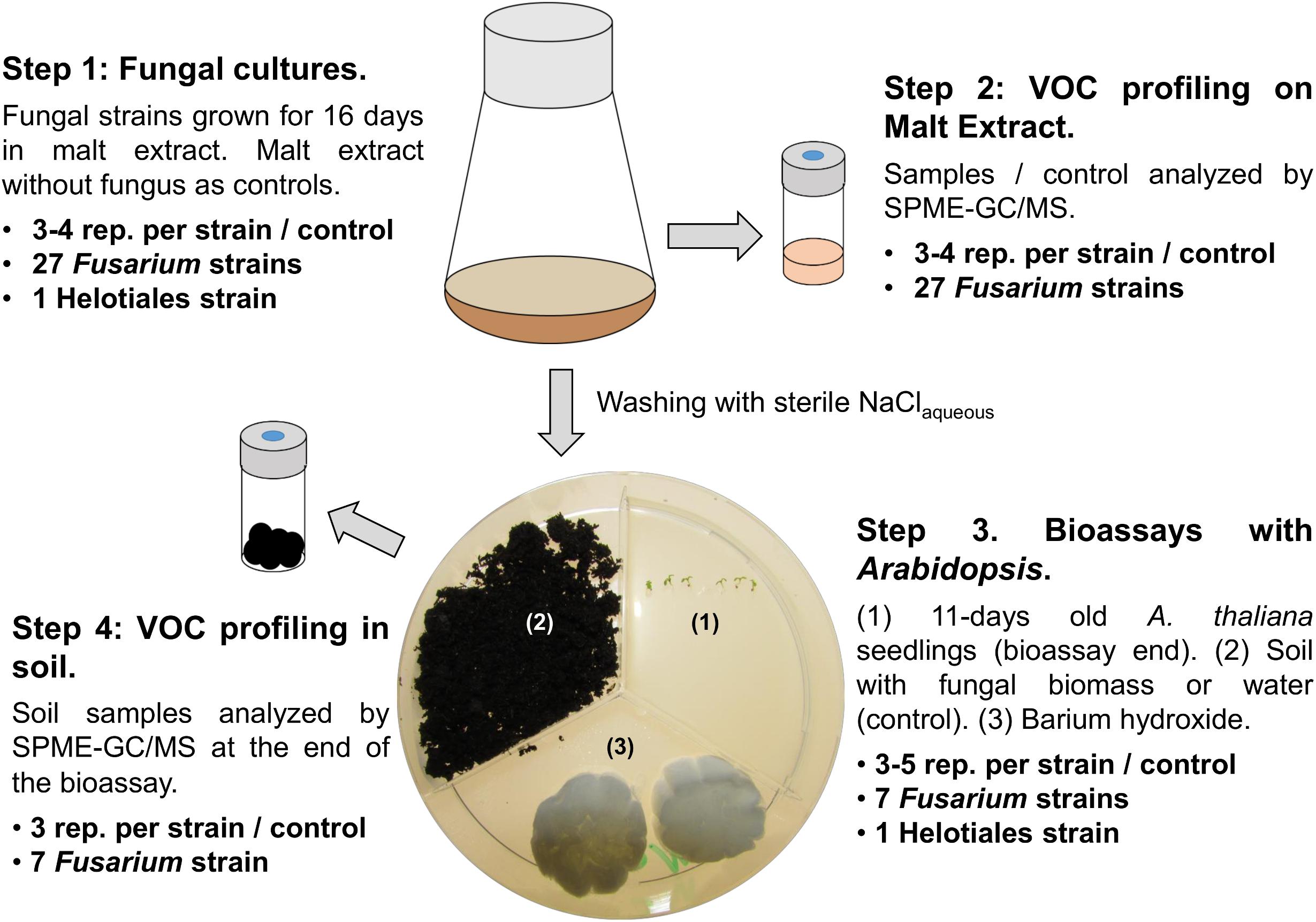
FIGURE 1. Experimental design. The figure illustrates the four experimental steps as well as the specific fungal strains and numbers of replicates used for each experiment. Step 1: Fungal strains were grown in liquid medium; sterile medium was used as control. Step 2: Fungi were harvested by centrifugation, one part of the mycelium or control medium was analyzed by GC/MS, one part was used to inoculate sterilized soil for the bioassays (step 3). Bioassay setup: six seeds of A. thaliana were grown exposed to VOCs released from the soil compartment. Plant morphology was determined at the end of the experiment and soil samples were analyzed by GC/MS (step 4).
Fungal inocula for VOC profiling and bioassays were prepared by growing each strain for 16 days in 100 ml Erlenmeyer flasks containing 30 ml of 15 gl-1 malt extract broth, pH 7.0 (Becton, Dickinson and Company, Heidelberg, Germany) at 24°C (step 1, Figure 1). Resulting fungal VOC profiles were characterized by gas chromatography/mass spectroscopy (GC/MS) (step 2, Figure 1 and “VOC profiling” hereafter) while the remaining fungal biomass was used to test the effect of VOCs emitted by selected Fusarium and the Helotiales strains on the model plant Arabidopsis thaliana ecotype Col-0 (Arabidopsis onward; step 3, Figure 1). These assays were also employed to profile the VOCs emitted by Fusarium grown in soil (step 4, Figure 1). Details about each experimental step is given hereafter.
VOC Profiling
Volatile organic compound profiling was performed in 20 ml solid-phase micro extraction (SPME) vials for fungal cultures or soil samples. Specifically, samples in malt extract contained either pelleted fungal biomass (1.25 g harvested after centrifugation at 12,000 ×g for 10 min) or culture medium without fungal inoculum (1.00 ± 0.05 ml). Alternatively, samples contained 1.50 ± 0.05 g of soil either inoculated with Fusarium or without fungus (control), taken directly at the end of the bioassays from the plate harboring soil (refer to “Bioassays with plants and fungi grown in soil”).
Solid-phase micro extraction vials, sealed with a silicon/polytetrafluoroethylene septum, were incubated at 60°C for 20 min prior to VOC sampling. VOCs from the head-space were subsequently extracted for 15 min at 60°C using a Divinylbenzene/Carboxen/Polydimethylsiloxane SPME fiber (DVB/CARB/PDMS) pre-conditioned following the manufacturer’s instructions (Sigma-Aldrich, Munich, Germany). Empty vials were regularly included in between runs to check for carry-over and at the beginning of each run to generate a blank. GC/MS analysis was performed on an Agilent 7890B GC coupled to an Agilent 5977B MSD (Agilent, Waldbronn, Germany). VOCs were desorbed from the SPME fiber at 250°C (GC inlet temperature) in splitless mode and were separated on an HP-5ms Ultra Inert capillary column (30 m × 0.25 mm with 0.25 μm coating) using the following program: 40°C for 5 min, ramp 3°C min-1 until 160°C, ramp 50°C min-1 until 260°C, hold 260°C for 6 min. Helium was used as a carrier gas (1.2 ml min-1). The MS scan range was 50–350 atomic mass units and ionization was performed at 70 eV. An EI 350 XTR was used as MS source. Source temperature was 230°C, MS quad temperature 150°C.
Chromatograms derived from the GC/MS analysis of fungal cultures and soil samples were aligned with the TagFinder 4.1 software (Luedemann et al., 2008) using parameters described earlier (Sherif et al., 2016), with the exception of the threshold which was set to 500. This resulted in a data matrix comprised of TAGs equivalent to single m/z values within a specific time range [(m/z, RT range)]. TAGs (made of one or more VOCs) present in empty SPME vials were subtracted from the data (3× blank removal). The data were normalized by dividing specific TAGs in each sample by the sum of all TAGs in that sample. This procedure is equivalent to normalizing the peak areas of single VOCs to the Total Ion Current (TIC).
Volatile organic compounds in TAGs of interest were either tentatively identified by comparison of their mass spectra to the ones found in the NIST Mass Spectral Search Program 2.0 (National Institute of Standards and Technology, Gaithersburg, MD, United States) or fully identified based on MS spectra, Kovats retention indices (n-alkane) and injection of an authentic standard. Specific VOCs that were fully identified were purchased from Sigma-Aldrich and Merck (Darmstadt, Germany), including (CAS number in parenthesis): furfural (98-01-1), 4-heptanone (123-19-3), 5-methyl-3-heptanone (541-85-5), benzaldehyde (100-52-7), 5-methyl-2-furancarboxaldehyde (620-02-0), 3-octanone (106-68-3), octanal (124-13-0), 2-ethyl-1-hexanol (104-76-7), benzeneacetaldehyde (122-78-1), nonanal (124-19-6), camphor (76-22-2), 1,2-dimethoxy-benzene (91-16-7) and decanal (112-31-2).
Quantification of VOCs Emitted From Soil
The concentration of seven VOCs (namely benzaldehyde, decanal, 5-methyl-2-furancarboxaldehyde, furfural, 5-methyl-3-heptanone, 2-ethyl-1-hexanol, nonanal) were quantified in soil by standard addition. These seven compounds were selected based on a linear model suggesting that they might be responsible for the root shortening of Arabidopsis observed in non-inoculated soil (see for details “Fusarium spp. decrease the emission of soil VOCs in the results section). For this purpose, water was first added to dried soil to adjust water content to the one determined at the end of the bioassays with Arabidopsis. The resulting sample (1.50 g wet soil = 0.26 g soil + 1.24 g water) was placed into a 20 ml SPME vial, and spiked either with 20 μl pure dichloromethane, or 20 μl dichloromethane with varying concentrations of single VOCs. Quantification was obtained by calculating the x-intercept of the resulting linear calibration curves.
Bioassays With Plants and Fungi Grown in Soil
Bioassays in tripartite Petri dishes (Greiner Bio One, Frickenhausen, Germany) were used to test the influence of fungi on root development. The partitioning in the Petri dishes ensured that interactions among organisms were strictly mediated by VOCs (see the illustration in Figure 1). The setup was based on Kai and Piechulla (2009), reviewed in Piechulla and Schnitzler (2016) and Kai et al. (2016). One compartment of each plate contained sterile soil inoculated with a single fungal strain (or non-inoculated as control). A sterile cotton piece with barium hydroxide (3 ml of a 25.7 g l-1 BaOH aqueous solution) was placed in another compartment to mitigate CO2 buildup (Piechulla and Schnitzler, 2016), and the third compartment harbored six seeds of Arabidopsis.
The plant compartment of the tripartite plates was filled with half strength Murashige and Skoog basal medium with 15 g l-1 sucrose (M9274, Sigma) and 1.5% agar (w/v). The Arabidopsis seeds were surface-sterilized for 2 min with 70% ethanol (v/v) followed by 20 min in a 1% sodium hypochlorite (NaHClO3) solution in water (v/v) and rinsed three times with sterile water. Seeds on Murashige and Skoog medium were stratified for 2 days at 4°C prior to the bioassays. Thereafter, the remaining Petri dish compartments were completed with barium hydroxide and soil/fungal biomass. Specifically, twice autoclaved commercial soil (Vermehrungssubstrat C200, Stender AG, Schermbeck) (4 g) was added to one compartment of the Petri dish.
To test the effect of fungal volatiles on early plant development, mycelium pre-grown for 16 days of one of seven selected Fusarium strains was placed on soil in the remaining compartment (Figure 1). These seven strains were selected from the three genetic clades I, II, and III (Figure 2A) based on the similarities and differences observed on their VOC profiles on malt extract (Figure 2B). To evaluate specificity of effects observed, one Helotiales strain was included in the bioassay. Fungal biomass (Figure 1, step 1 and Table 1) was homogenized for 1 min with a blender (IKA T18 digital Ultra-Turrax IKA-Werke GMBH, Staufen, Germany), washed twice with 0.85% (w/v) NaClaq to remove traces of malt extract, and pelleted by centrifugation at 12,000 ×g for 10 min. A wet fungal biomass of 50.0 ± 0.5 mg was transferred to fresh tubes and resuspended in 1 ml 0.85% (w/v) NaClaq. These were homogenously distributed on the soil surface (Figure 1, Step 3). The same volume of 0.85% (w/v) NaClaq without fungus was used for control Petri dishes.
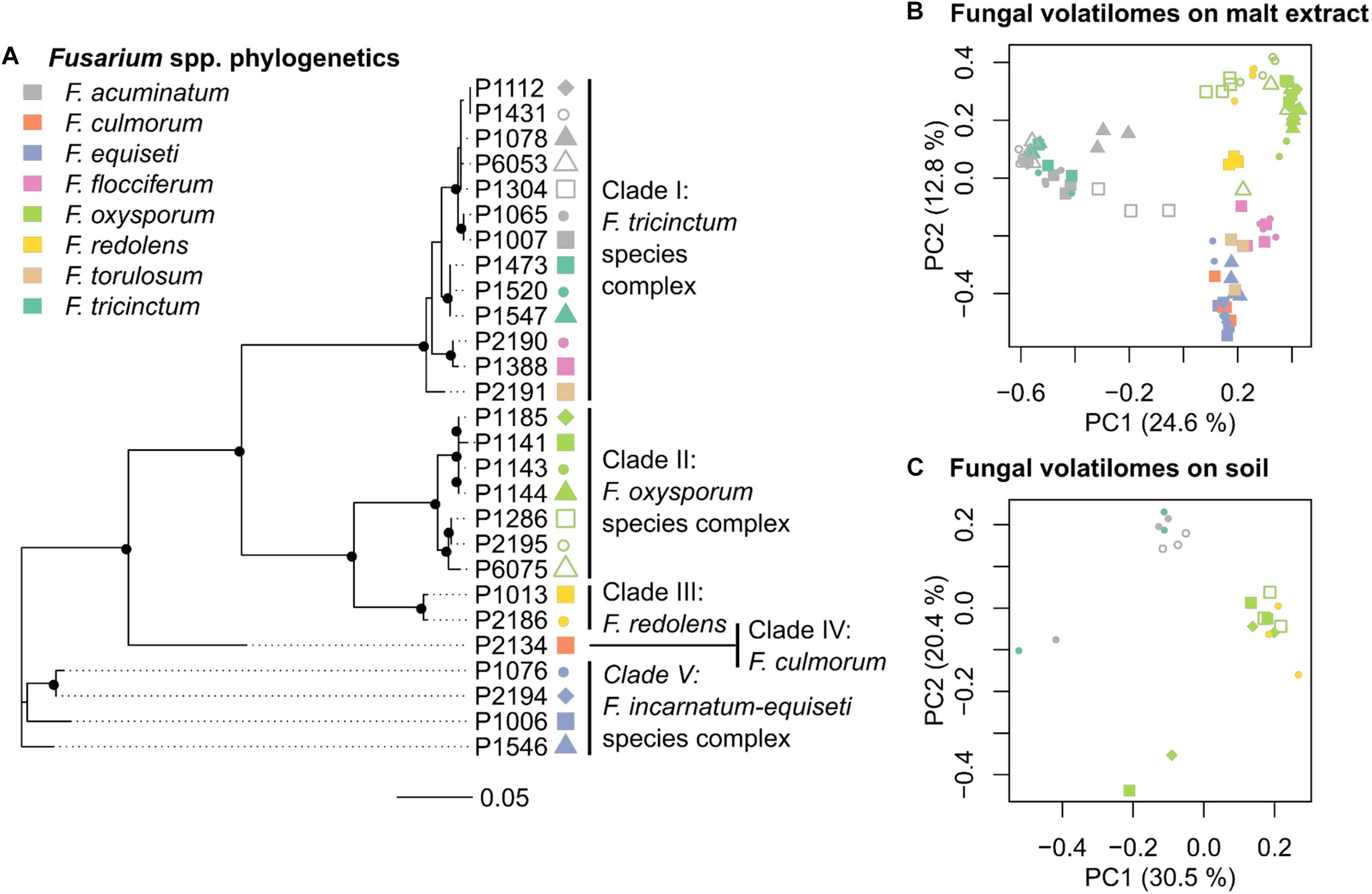
FIGURE 2. Phylogeny and volatilome of Fusarium spp. (A) Phylogenetic tree of 27 Fusarium strains based on partial tef-1α sequences (Table 1). Black dots in the tree nodes indicate bootstrap values ≥70% based on 1,000 replicates. (B) Principle Coordinate Analysis (PCoA) based on the VOC profiles of 27 Fusarium strains grown in malt extract (n = 3–4 replicates per strain) (C) PCoA based on the VOC profiles of 7 Fusarium strains grown in soil (n = 3 replicates per strain). Each point in PCoAs correspond to an independently measured volatile profile, with point color indicating the species (see color key), and different point types within each clade denoting different strains. Both PCoAs highlight that differences in VOC profiles among strains correspond by large to genotypic differences.
Plates, sealed with Parafilm, were incubated in an upright position in a growth chamber (Binder KBW 400, Tuttlingen, Germany) for 11 days (23°C, a 16 h photo period, 8,140 lux). Plates were digitalized at the end of the assays with a flat-bed scanner and leaf size and primary root length were measured with the ImageJ (v1.51q) software (Schneider et al., 2012). Secondary root branching was determined under a stereomicroscope (Leica MZ16, Leica Microsystems, Wetzlar, Germany).
Bioassays With Plants and Synthetic VOCs
Bioassays with mixtures of seven synthetic VOCs were performed similarly to the ones with soil, except that soil was replaced with a piece of sterile cotton harboring mixtures of synthetic VOCs in 20 μl dichloromethane. The mixture of compounds tested in these assays were benzaldehyde, decanal, 5-methyl-2-furancarboxaldehyde, furfural, 5-methyl-3-heptanone, 2-ethyl-1-hexanol, and nonanal. Specific mixtures contained 0.00015, 0.15, 1.5, 10, 20, and 100 μg of each volatile compound in 20 μl dichloromethane. Quantities were selected to reflect the concentrations of single VOCs determined in soil (Table 2). These mixtures were added to the Petri dish at the beginning of the bioassay and once again after 6 days. Control Petri dishes were prepared with 2× 20 μl dichloromethane similarly added at the start of the assay and on day six. Plant morphology was evaluated as in the earlier assays after eleven days of exposure to VOCs.
Statistical Analysis
Statistical tests were conducted at several stages using Past 3.04 (Hammer et al., 2001) or R version 3.4.3 (R Core Team, 2017) and packages within. Nonparametric Kruskal-Wallis tests were performed in Past to identify VOCs (TAGs) which concentrations significantly differed among samples and to assess differences in plant morphology (Arabidopsis primary root length, leaf size and root branching) resulting from different treatments. Correlation of VOCs and Arabidopsis primary root length was tested via a linear model with Past. Boxplots of primary root length were generated with R, as well as phylogenetic trees (using the package “ape” 5.0 (Paradis et al., 2004)) and Principle Coordinate analyses (PCoAs) (using the package “vegan” 2.4-5 (Oksanen et al., 2017)). For PCoAs all VOC matrices were square root transformed. Details about statistics and number of replicates are given in figure legends.
Results
Fungal VOC Profiles Depend Both on Genotype and Culture Media
The first objective was to genetically characterize fungal isolates to relate genotypic diversity to variability in VOC profiles. Partial sequencing of the tef1-α gene indicates that the twenty-seven Fusarium strains used in this study belong to five genetic clades (Figure 2 and Supplementary Figure S1 for a phylogenetic tree including reference strains). These clades harbor members of several Fusarium species complexes, namely F. tricinctum (clade I - F. tricinctum, F. acuminatum, F. flocciferum and F. torulosum), F. oxysporum (clade II), F. redolens (clade III), F. culmorum (clade IV) and F. incarnatum-equiseti (clade V) (Figure 2A).
Volatile organic compounds profiles were generated by SPME-GC/MS for all 27 Fusarium strains on malt extract and for seven selected strains grown in soil (Table 1). Chromatograms were processed with the Tagfinder software (Luedemann et al., 2008) for alignment. Multivariate analysis was applied to normalized VOC profiles after removal of the signal emitted by the respective control samples (either pure malt extract or non-inoculated soil). A PCoA plot showing all replicates (n ≥ 3) per strains illustrates that the VOC profiles of the 27 Fusarium strains on malt extract varied by large in accordance to genetic identity (Figure 2B). Those strains formed three major clusters. The first cluster included several strains from the F. tricinctum species complex, the second cluster all strains of the F. oxysporum and F. redolens complex and the third complex all remaining strains, including F. torulosum and F. flocciferum from the F. tricinctum complex. Given the axes displayed, 37.4% of total variation could be explained by the model displayed in Figure 2B. The same conclusion, that volatilomes depend on genetic identities, can be reached for strains grown in soil (Figure 2C). Strains from the F. tricinctum species complex were separated from F. oxysporum and F. redolens strains. Explained variation of the model was 50.9% in Figure 2C. Overall this confirms that, regardless of the medium used for fungal growth, species identity has the biggest impact on the VOC profiles of Fusarium strains.
Fusarium Strains Increase Primary Root Length in Arabidopsis Seedlings
Since genetically distinct Fusarium strains differed in their VOC profiles, we hypothesized that these differences might also affect plant growth in specific ways (i.e., stimulation in some cases and inhibition in others). Hence, the influence of selected soil-grown Fusarium strains on plant development was tested in a system which prevents physical contact between the test fungus and plant but allows indirect interactions through VOCs (Figure 1). The effect of seven Fusarium strains and one unclassified Helotiales strain on Arabidopsis development (primary root length, root branching and leaf size) was recorded and compared to control seedlings grown in the presence of soil only (no fungus).
Due to high variability between time independent replicates, primary root length and leaf surface area were normalized to control plants (not exposed to fungi). Similarly, root branching was normalized to primary root length. Most fungal strains strongly influenced primary root growth but no significant differences were detected in terms of leaf surface area or root branching (Supplementary Figure S2). Specifically, seedlings grown in the presence of all Fusarium strains had significantly longer roots than control plants, while the Helotiales strain did not affect primary root development (Figure 3). Overall this indicates that all Fusarium strains tested had the ability to increase primary root growth. In contrast, the unidentified Helotiales strain did not.
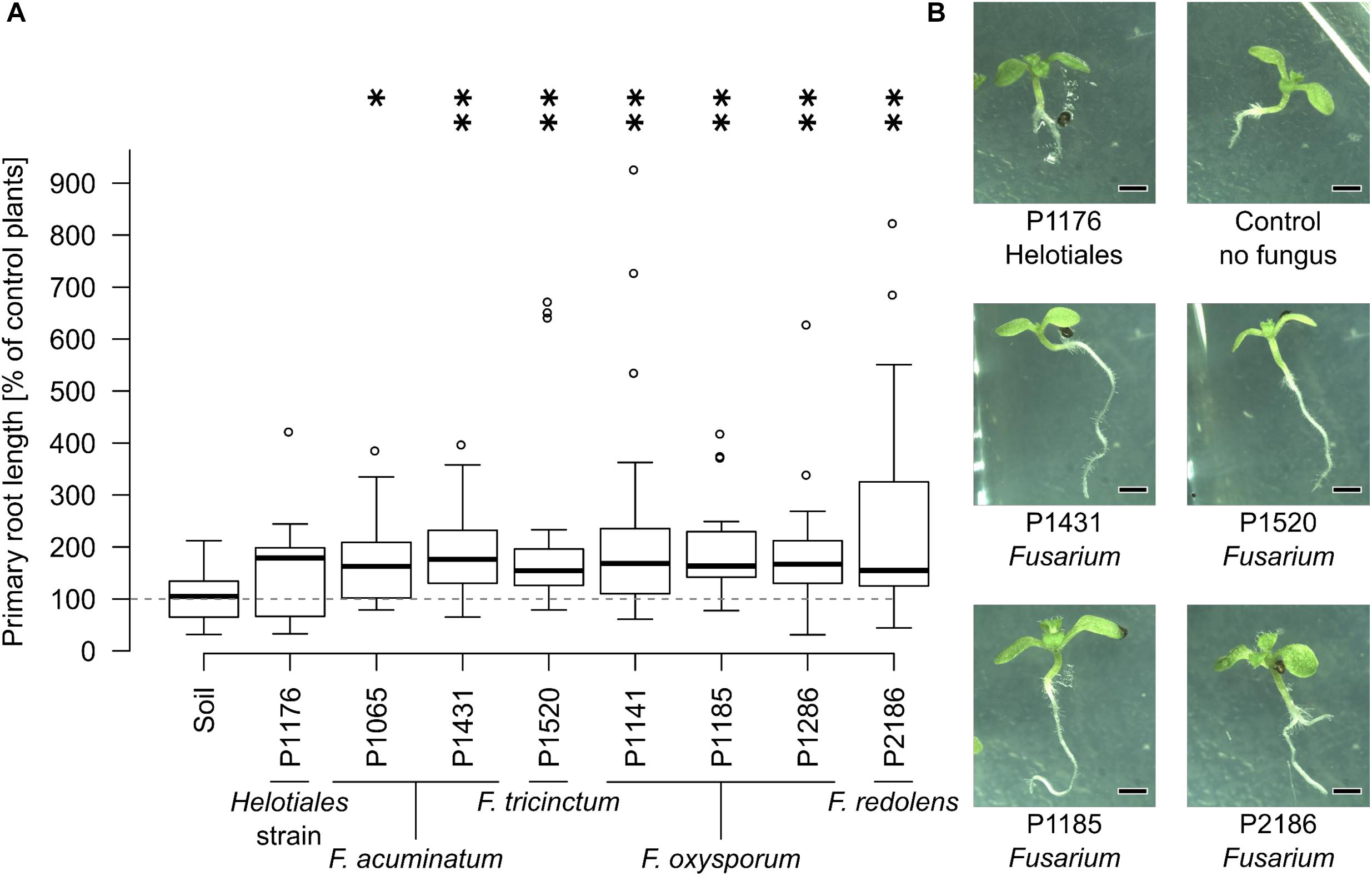
FIGURE 3. The presence of Fusarium increases Arabidopsis primary root length. Bioassays were conducted as described in Figure 1. (A) All soil-grown Fusarium strains but not the Helotiales one significantly increased primary root length compared to control seedlings (exposed to soil VOCs only) (Kruskal-Wallis test, ∗indicates a p-value of ≤0.05, ∗∗≤ 0.01, n = 14 to 20 seedlings pooled from 3 to 5 Petri dishes). (B) Representative 11 days old seedlings grown with or without fungi (scale bar = 1 mm).
Fusarium spp. Decrease the Emission of Soil VOCs
Given the primary root growth stimulation observed with all Fusarium strains, we aimed at indentifying the VOC(s) responsible for this effect. VOC profiles of soil-grown Fusarium were compared to the one of non-inoculated soil (samples were taken at the end of the biossays documented in Figure 3). Chromatograms for soil-grown strains and non-inoculated soil are visible in Figure 4 (GC/MS data files are available as Supplementary Data Sheet S3). Specifically, VOC(s) (TAGs) whose concentration significantly differed among strains and non-inoculated soil were identified by a Kruskal-Wallis test (p < 0.05). The resulting data matrix was used to generate a PCoA ordination showing that the VOC profile of non-inoculated soil drastically differed from that of soil inoculated with Fusarium (Figure 5A). The model overall explained 51% of the data varability. The PCoA scores of individual TAGs (Figure 5B) highlight that specific VOCs were responsible for the differences between non-inoculated soil and Fusarium inoculated soil seen in Figure 5A. TAGs representing VOCs identified by authentic standards are indicatied as red numbered dots in Figure 5B.
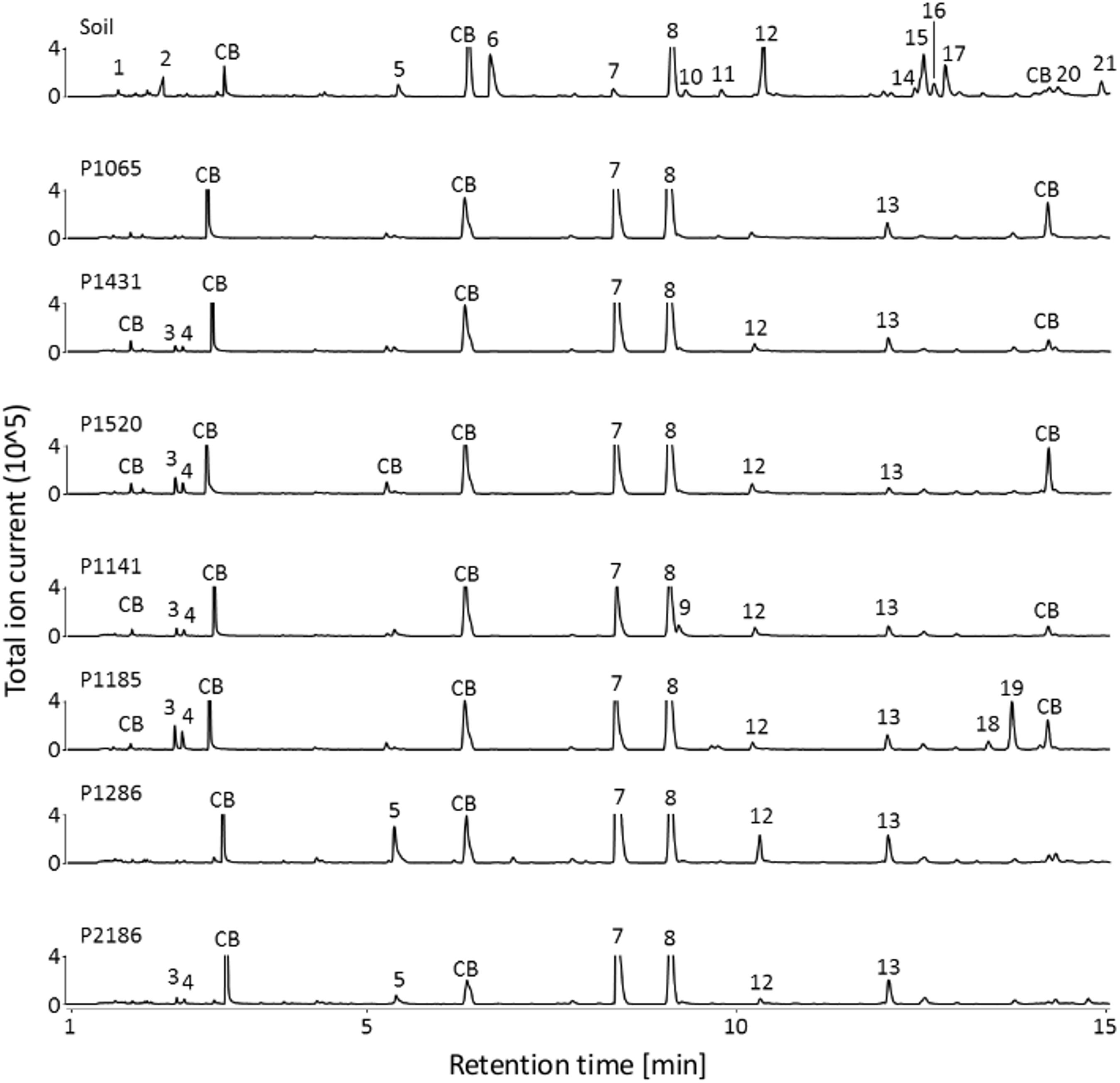
FIGURE 4. VOCs detected in non-inoculated and Fusarium-inoculated soil samples. GC/MS chromatograms (TIC) illustrating the volatile profiles of non-inoculated soil samples and of Fusarium inoculated soil samples. Fusarium strains overall decreased the number of VOCs detected in the chromatograms compared to non-inoculated soil. CB: column bleed (siloxane derivatives), 1: unidentified, 2: unidentified, 3: 3-methylbutanal, 4: 2-methylbutanal, 5: hexanal, 6: 3-furancarbaldehyde, 7: 4-heptanone∗, 8: styrene, 9: 4-heptanol, 10: 2-heptanone, 11: heptanal, 12: methyl-(Z)-N-hydroxybenzenecarboximidate, 13: 7-ethyl-4-nonanone, 14: 2-ethylhexanal, 15: benzaldehyde∗, 16: unidentified, 17: 5-methyl-2-furancarboxaldehyde∗, 18: unidentified, 19: 1-octen-3-ol, 20: unidentified, 21: octanal∗. ∗ Fully identified compounds (based on authentic standard, Kovats-retention indices, mass fragmentation), all other compounds were tentatively identified based on Kovats-retention indices and mass fragmentation).
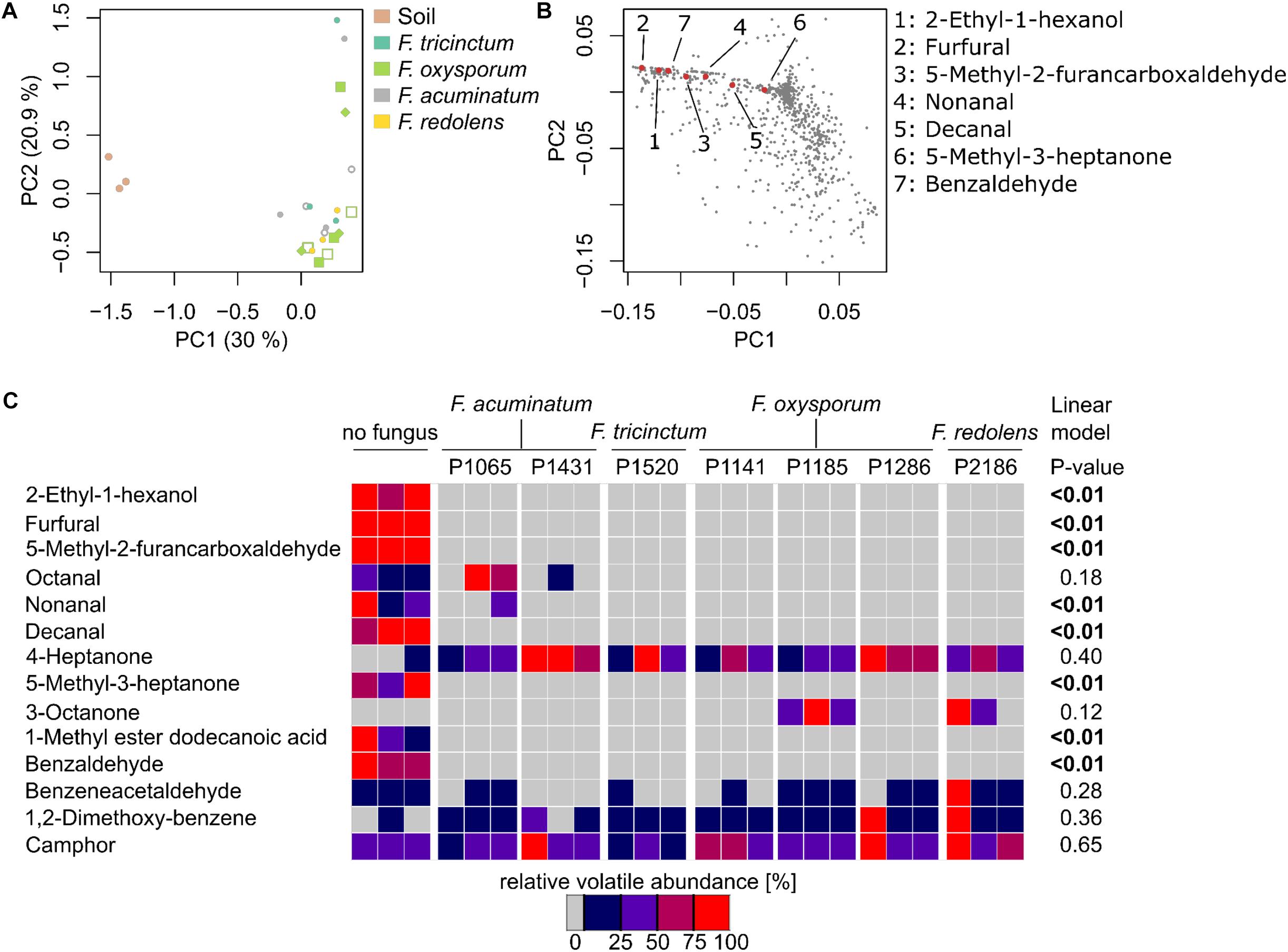
FIGURE 5. Fusarium spp. reduces the emission of soil VOCs. (A) Principle Coordinate Analysis ordination (PCoA) based on VOCs emitted by non-inoculated soil and Fusarium-inoculated soil samples highlights major differences among non-inoculated and Fusarium-inoculated soil (n = 3 replicates per strain). (B) PCoA illustrating the distribution of TAGs (VOCs) across samples, showing those responsible of the differences observed in (a). Red, numbered dots correspond to identified VOCs. (C) Heatmap illustrating the concentration of 14 identified VOCs in n = 3 replicates (Supplementary Figure S5 includes unidentified VOCs as well) and highlighting that non-inoculated soil tends to have a higher concentration of VOCs compared to Fusarium-inoculated soil. P-values reported on the right side of the heatmap correspond to significant negative correlations among VOC concentration and primary root length (reported in Figure 3) obtained through a linear model.
A heatmap was constructed to vizualize how the concentration of 14 VOCs that could be identified differed among treatments (Figure 5C). The corresponding heatmap with an extra 33 unidentified compounds in shown in Supplementary Figure S5 and corresponding mass spectras can be obtained from GC/MS data files available as Supplementary Data Sheet S3. It shows that the concentrations of these VOCs significantly differed between Fusarium-inoculated and non-inoculated soil, in most cases due to a reduction of the concentration of numerous VOCs in respect to non-inoculated soil. By contrast, only 3-octanone was absent from non-inoculated soil but present only in two Fusarium treatments (Figure 5C). Overall this indicates that Fusarium spp. have the ability to drastically reduce the concentration of soil VOCs.
To pinpoint VOCs that might be responsible for the root length stimulation described herewith, a linear model was used to test for significant correlations among the concentrations of specific VOCs and primary root length. Seven VOCs, with maximal concentrations in non-inoculated soil, were significantly and negatively correlated to primary root length (Figure 5C) and might thus explain root length stimulation observed in the presence of Fusarium.
Mixtures of Synthetic VOCs Decrease Primary Root Length at Concentrations Comparable to Those Emitted by Soil
The linear model of Figure 5C suggests that, among identified VOC, seven might be specifically responsible for the primary root length stimulation observed with Fusarium (Figure 3). The concentrations of these VOCs were quantified from soil by standard addition and ranged at the end of the bioassays from 26 ng g-1 to 16 μg g-1 soil (wet weight) (Table 2).
Bioassays with Arabidopsis were subsequently performed using mixtures of the former seven VOCs dissolved in dichloromethane at varying concentrations. Since all those VOCs were emitted by sterilized and non-inoculated soil, a piece of cotton was used in compartment 2 (Figure 1) of the bioassay instead of soil. This mixture of VOCs significantly inhibited growth of primary roots at quantities corresponding to 10–20 μg and fully blocked germination from 100 μg onward (Figure 6). Overall this demonstrates that a mixture of synthetic soil VOCs effectively decreased primary root length at concentrations comparable to those emitted by soil.
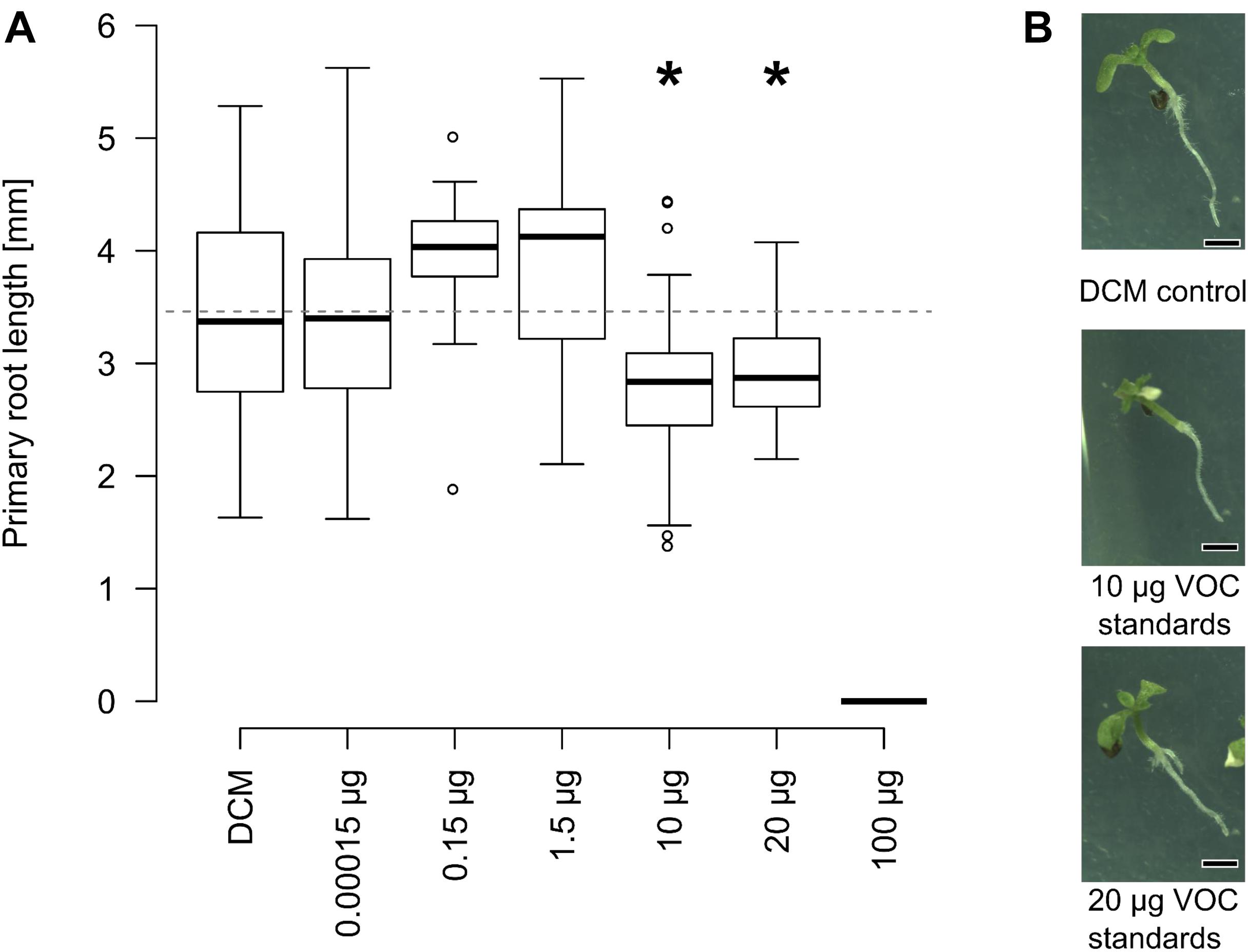
FIGURE 6. Effect of VOC standards identified in soil on root growth. (A) Arabidopsis plants were grown on tripartite plates, as described in Figure 1. Instead of soil, the second compartment harbored cotton with mixtures of 2-ethyl-1-hexanol, furfural, 5-methyl-2-furancarboxaldehyde, nonanal, decanal, 5-methyl-3-heptanone and benzaldehyde in dichloromethane. Primary root length was mostly unaffected by low concentration, significantly smaller between 10 and 20 μg than plants exposed to dichloromethane without additional VOCs. Germination was fully suppressed at 100 μg (∗p ≤ 0.05, Kruskal Wallis, n = 15–45 seedlings pooled from 6 to 15 Petri dishes). (B) Speciemen of 11-days old Arabidopsis plants exposed to different volatile blends at the end of the experiment (scale bar = 1 mm).
Discussion
Here we investigated the variability in the VOC profiles of various Fusarium fungal species and demonstrated that microbial genetic background and media composition influenced volatile profiles. VOC fingerprints were generated on malt extract for all isolates and on soil for representative members of dominant Fusarium clades (Figure 2). Medium composition and species identity impacted Fusarium VOC emission (Supplementary Figure S3), which is in line with previous results illustrating that biotic and abiotic factors can greatly impact secondary metabolism (Blom et al., 2011; Brakhage and Schroeckh, 2011; Schenkel et al., 2015; Schmidt et al., 2016; Lazazzara et al., 2017). Specifically, emission of fungal VOCs might for instance depend on the age/growth stage of mycelium (Naznin et al., 2013; Lee et al., 2015). The influence of growth medium on secondary metabolism can be attributed to the direct availability of precursors in the growth medium or the modulating effects of specific metabolites on secondary metabolism (Zain et al., 2011). Whereas malt extract is a sugar-rich medium (Balcerek et al., 2016), soil is nutrient poor and rich in amino acids and lipids (Pétriacq et al., 2017). These dissimilarities might explain the drastic differences in VOC profiles observed here for the same species grown on soil or malt extract (Figures 2B, 5A, Supplementary Figures S3, S4). Furthermore, the fact that soil contains a large pool of uncharacterized metabolites (Pétriacq et al., 2017) might explain why the structure of numerous VOCs could not be elucidated based on existing mass spectral databases. VOCs emitted by soil indeed depend on a plethora of variables including environmental and soil physico-chemical factors (Insam and Seewald, 2010). Elucidating the structure of novel soil VOCs and understanding how they are formed will be a challenge for future studies.
Bioassays investigating VOC-based interactions between soil-grown Fusarium and Arabidopsis highlighted the ability of the Fusarium genus to increase primary root length. This growth promoting effect was not observed for the unidentified Helotiales strain, suggesting a high impact of genetic background. It has been hypothesized that fungi with different lifestyles (i.e., pathogens, saprophytes, symbiotic) might emit different volatiles (Müller et al., 2013; Schenkel et al., 2015), which could in turn partially explain plant phenotype. The dataset presented here is however not optimal to validate this hypothesis, first because the Helotiales strain used here has not been fully characterized and, second because numerous Fusarium isolates can switch their lifestyle depending on plant hosts and environmental conditions (Kia et al., 2017; Lofgren et al., 2018). Thus, attributing a strict pathogenic/saprotrophic/endophytic lifestyle to these strains would be difficult and likely misleading.
Volatile organic compound-based root length stimulation comparable to the one reported here (i.e., 49–126% increase compared to control plants) have been described by others (Bitas et al., 2015; Ditengou et al., 2015). By contrast to primary root length, leaf area and root branching of the 11-days-old seedlings were not significantly affected by the presence of Fusarium strains in this study. This is in opposition with earlier results that reported increased leaf size and root branching in Arabidopsis seedlings of comparable age along with primary root length stimulation (Ryu et al., 2003; Splivallo et al., 2009; Bitas et al., 2015; Ditengou et al., 2015). This might indicate that under our experimental conditions, Fusarium specifically stimulated primary root length or that root branching and leaf surface would only have been affected at a later plant developmental stage. The root length shortening observed with Arabidopsis could be attributed to seven VOCs that showed bioactivity from 70 μg (a mixture of 10 μg each) up. This is comparable to quantities of bioactive VOCs reported by others (Ryu et al., 2003; Zou et al., 2010; Blom et al., 2011; Park et al., 2015). Additionally, one of the bioactive VOC tested here (2-ethyl-1-hexanol) is phytotoxic to ornamental plants (Hörmann et al., 2018). Demonstrating whether similar effects occur under field conditions will be a challenge that will require to carefully take soil parameters as well as source and sink mechanisms under consideration.
Lastly, our results indicate that Fusarium has the capacity to decrease soil VOCs. The mechanism behind this phenomenon is elusive, yet the ability of microbes to consume VOCs has been occasionally described. For instance, microbial communities are able to degrade VOCs in soil including benzene and toluene under aerobic conditions (Yoshikawa et al., 2017). Tracer experiments with radioactive carbon revealed that the labelled monoterpene geraniol was metabolized by unidentified soil/rhisophere microbes and transformed into another monoterpene (limonene) (Owen et al., 2007). Mono- and sesquiterpenes can also serve as carbon source to rhizobacteria (Best et al., 1987; Kleinheinz et al., 1999; Del Giudice et al., 2008; Schulz-Bohm et al., 2015, 2017). It has also been suggested that VOCs might act as carbon source for fungal growth on carbon-poor substrate (Cale et al., 2016). Besides being metabolized or transformed, soil VOCs might also be adsorbed to fungal hyphae. Demonstrating the exact mechanism that leads to the decrease of specific VOCs by Fusarium will require combining labeling experiments to metabolomics in order to understand the fate of specific VOCs.
Overall our results constitute an important paradigm shift in plant-microbial interactions since they suggest that microbes shall not only be considered as the possible emitter of bioactive VOCs, but also as a possible sink. Shedding light on the implications of VOC degradation by microbes will require understanding their breakdown mechanisms which remains elusive. Nevertheless, this finding might have important implications in plant-microbial interactions and suggest that considering the soil volatilome might help to predict plant productivity.
Author Contributions
RS and DS designed all the experiments. DS performed all the experiments with input from AB for the bioassays on soil. JM-V provided plant and fungal material and contributed to fungal characterization. DS, RS, and JM-V analyzed the data and wrote the manuscript.
Funding
We thankfully acknowledge the LOEWE research program of the government of Hesse, Germany, in the framework of the Integrative Fungal Research Cluster (IPF) for funding and access to the fungal collection.
Conflict of Interest Statement
The authors declare that the research was conducted in the absence of any commercial or financial relationships that could be construed as a potential conflict of interest.
Supplementary Material
The Supplementary Material for this article can be found online at: https://www.frontiersin.org/articles/10.3389/fmicb.2018.01847/full#supplementary-material
FIGURE S1 | Phylogenetic tree of Fusarium strains used in this study and reference strains. Phylogenetic tree of 27 Fusarium strains (Table 1) and reference strains [FUSARIUM-ID database (Geiser et al., 2004)] based on tef-1α sequences. Numbers at nodes indicates bootstrap values (1,000 replications).
FIGURE S2 | The presence of Fusarium does not affect Arabidopsis root branching or leaf surface area. Bioassays were conducted as described in Figure 1. No significant differences in leaf surface area or root branching (number of secondary roots) were observed between Arabidopsis seedlings exposed to VOCs from non-inoculated soil or Fusarium or Helotiales-inoculated soil (statistics: Kruskal-Wallis test, p ≤ 0.05).
FIGURE S3 | Comparison the effect of substrate on the VOC profile of Fusarium. Cluster analysis of the VOC profile of seven Fusarium strains grown either on malt extract (3–4 replicates per strain) or soil (3 replicates per strain). A representative VOC profile for each strain was generated by considering only TAGs (VOCs) which consistently appeared in all replicates, and these representative profiles were used to compute the dendrogram presented here. Signal present in the respective controls (i.e., soil or pure malt extract) was removed. TAGs transformed into binary data (i.e., presence–absence) were used to generate a cluster tree using Jaccard similarity index. ∙ represent nodes with ≥70% (1,000 bootstraps).
FIGURE S4 | VOC profiles of Fusarium strain P1065 on malt extract and soil. The two upper chromatograms represent the VOC profile of strain P1065 on malt extract and the profile of the control (malt extract without fungus), whereas the two lower chromatograms illustrate the VOC emitted by the same fungus on soil (or the soil control). These results exemplify the influence of the medium (malt extract versus soil) on microbial VOC emission. VOCs were identified or tentatively identified as: (1) 1-hexanol; (2) 4-heptanone; (3) styrene; (4) 7-ethyl-4-noanone; (5) benzaldehyde; (6) 1,3,5-trimethylbenzene; (7) 5-methyl-2-furancarboxaldehyde; (8) 1-heptanol.
FIGURE S5 | VOC profiles of soil samples at the end of the bioassay. Heatmap illustrating the concentration of 14 identified VOCs and 33 not identified VOCs in n = 3 replicates per fungal strain and highlighting that non-inoculated soil tends to have a higher concentration of VOCs compared to Fusarium-inoculated soil.
DATA SHEET S3 | GC/MS data for non-inoculated and Fusarium inoculated soil.
References
Altschul, S. F., Gish, W., Miller, W., Myers, E. W., and Lipman, D. J. (1990). Basic local alignment search tool. J. Mol. Biol. 215, 403–410. doi: 10.1016/S0022-2836(05)80360-2
Balcerek, M., Pielech-Przybylska, K., Strąk, E., Patelski, P., and Dziekońska, U. (2016). Comparison of fermentation results and quality of the agricultural distillates obtained by application of commercial amylolytic preparations and cereal malts. Eur. Food Res. Technol. 242, 321–335. doi: 10.1007/s00217-015-2542-7
Best, D. J., Floyd, N. C., Magalhaes, A., Burfield, A., and Rhodes, P. M. (1987). Initial enzymatic steps in the degradation of alpha-pinene by Pseudomonas fluorescens Ncimb 11671. Biocatalysis 1, 147–159. doi: 10.3109/10242428709040139
Bitas, V., McCartney, N., Li, N., Demers, J., Kim, J.-E., Kim, H.-S., et al. (2015). Fusarium oxysporum volatiles enhance plant growth via affecting auxin transport and signaling. Front. Microbiol. 6:1248. doi: 10.3389/fmicb.2015.01248
Blom, D., Fabbri, C., Connor, E. C., Schiestl, F. P., Klauser, D. R., Boller, T., et al. (2011). Production of plant growth modulating volatiles is widespread among rhizosphere bacteria and strongly depends on culture conditions: volatile-mediated impact of bacteria on Arabidopsis thaliana. Environ. Microbiol. 13, 3047–3058. doi: 10.1111/j.1462-2920.2011.02582.x
Brakhage, A. A., and Schroeckh, V. (2011). Fungal secondary metabolites – strategies to activate silent gene clusters. Fungal Genet. Biol. 48, 15–22. doi: 10.1016/j.fgb.2010.04.004
Cale, J. A., Collignon, R. M., Klutsch, J. G., Kanekar, S. S., Hussain, A., and Erbilgin, N. (2016). Fungal volatiles can act as carbon sources and semiochemicals to mediate interspecific interactions amongbark beetle-associated fungal symbionts. PLoS One 11:e0162197. doi: 10.1371/journal.pone.0162197
Castresana, J. (2000). Selection of conserved blocks from multiple alignments for their use in phylogenetic analysis. Mol. Biol. Evol. 17, 540–552. doi: 10.1093/oxfordjournals.molbev.a026334
Choi, G.-H., Ro, J.-H., Park, B.-J., Lee, D.-Y., Cheong, M.-S., Lee, D.-Y., et al. (2016). Benzaldehyde as a new class plant growth regulator on Brassica campestris. J. Appl. Biol. Chem. 59, 159–164. doi: 10.3839/jabc.2016.029
Cordovez, V., Mommer, L., Moisan, K., Lucas-Barbosa, D., Pierik, R., Mumm, R., et al. (2017). Plant phenotypic and transcriptional changes induced by volatiles from the fungal root pathogen Rhizoctonia solani. Front. Plant Sci. 8:1262. doi: 10.3389/fpls.2017.01262
R Core Team (2017). R: A Language and Environment for Statistical Computing. Vienna: R Foundation for Statistical Computing.
Del Giudice, L., Massardo, D. R., Pontieri, P., Bertea, C. M., Mombello, D., Carata, E., et al. (2008). The microbial community of Vetiver root and its involvement into essential oil biogenesis: vetiver root bacteria and essential oil biogenesis. Environ. Microbiol. 10, 2824–2841. doi: 10.1111/j.1462-2920.2008.01703.x
Ditengou, F. A., Müller, A., Rosenkranz, M., Felten, J., Lasok, H., van Doorn, M. M., et al. (2015). Volatile signalling by sesquiterpenes from ectomycorrhizal fungi reprogrammes root architecture. Nat. Commun. 6:6279. doi: 10.1038/ncomms7279
Dudareva, N., Klempien, A., Muhlemann, J. K., and Kaplan, I. (2013). Biosynthesis, function and metabolic engineering of plant volatile organic compounds. New Phytol. 198, 16–32. doi: 10.1111/nph.12145
Geiser, D. M., del Mar Jiménez-Gasco, M., Kang, S., Makalowska, I., Veeraraghavan, N., Ward, T. J., et al. (2004). FUSARIUM-ID v. 1.0: a DNA sequence database for identifying Fusarium. Eur. J. Plant Pathol. 110, 473–479. doi: 10.1023/B:EJPP.0000032386.75915.a0
Glynou, K., Ali, T., Buch, A.-K., Haghi Kia, S., Ploch, S., Xia, X., et al. (2016). The local environment determines the assembly of root endophytic fungi at a continental scale. Environ. Microbiol. 18, 2418–2434. doi: 10.1111/1462-2920.13112
Hammer,Ø, David, A., Harper, T., and Ryan, P. D. (2001). Past: paleontological statistics software package for education and data analysis. Palaeontol. Electron. 4, 1–9.
Hörmann, V., Brenske, K.-R., and Ulrichs, C. (2018). Assessment of filtration efficiency and physiological responses of selected plant species to indoor air pollutants (toluene and 2-ethylhexanol) under chamber conditions. Environ. Sci. Pollut. Res. 25, 447–458. doi: 10.1007/s11356-017-0453-9
Hung, R., Lee, S., and Bennett, J. W. (2013). Arabidopsis thaliana as a model system for testing the effect of Trichoderma volatile organic compounds. Fungal Ecol. 6, 19–26. doi: 10.1016/j.funeco.2012.09.005
Insam, H., and Seewald, M. S. A. (2010). Volatile organic compounds (VOCs) in soils. Biol. Fertil. Soils 46, 199–213. doi: 10.1007/s00374-010-0442-3
Junker, R. R., and Tholl, D. (2013). Volatile organic compound mediated interactions at the plant-microbe interface. J. Chem. Ecol. 39, 810–825. doi: 10.1007/s10886-013-0325-9
Kai, M., Effmert, U., and Piechulla, B. (2016). Bacterial-plant-interactions: approaches to unravel the biological function of bacterial volatiles in the rhizosphere. Front. Microbiol. 7:108. doi: 10.3389/fmicb.2016.00108
Kai, M., and Piechulla, B. (2009). Plant growth promotion due to rhizobacterial volatiles–an effect of CO2? FEBS Lett. 583, 3473–3477. doi: 10.1016/j.febslet.2009.09.053
Karim, N. F. A., Mohd, M., Nor, N. M. I. M., and Zakaria, L. (2016). Saprophytic and potentially pathogenic Fusarium species from peat soil in Perak and Pahang. Trop. Life Sci. Res. 27, 1–20.
Katoh, K., and Standley, D. M. (2013). MAFFT multiple sequence alignment software version 7: improvements in performance and usability. Mol. Biol. Evol. 30, 772–780. doi: 10.1093/molbev/mst010
Kia, S. H., Glynou, K., Nau, T., Thines, M., Piepenbring, M., and Maciá-Vicente, J. G. (2017). Influence of phylogenetic conservatism and trait convergence on the interactions between fungal root endophytes and plants. ISME J. 11, 777–790. doi: 10.1038/ismej.2016.140
Kleinheinz, G. T., Bagley, S. T., John, W. P. S., Rughani, J. R., and McGinnis, G. D. (1999). Characterization of alpha-pinene-degrading microorganisms and application to a bench-scale biofiltration system for VOC degradation. Arch. Environ. Contam. Toxicol. 37, 151–157. doi: 10.1007/s002449900500
Knudsen, J. T., Eriksson, R., Gershenzon, J., and Ståhl, B. (2006). Diversity and distribution of floral scent. Bot. Rev. 72, 1–120.
Lazazzara, V., Perazzolli, M., Pertot, I., Biasioli, F., Puopolo, G., and Cappellin, L. (2017). Growth media affect the volatilome and antimicrobial activity against Phytophthora infestans in four Lysobacter type strains. Microbiol. Res. 201, 52–62. doi: 10.1016/j.micres.2017.04.015
Lee, S., Hung, R., Yap, M., and Bennett, J. W. (2015). Age matters: the effects of volatile organic compounds emitted by Trichoderma atroviride on plant growth. Arch. Microbiol. 197, 723–727. doi: 10.1007/s00203-015-1104-5
Lemfack, M. C., Gohlke, B.-O., Toguem, S. M. T., Preissner, S., Piechulla, B., and Preissner, R. (2018). mVOC 2.0: a database of microbial volatiles. Nucleic Acids Res. 46, D1261–D1265. doi: 10.1093/nar/gkx1016
Lofgren, L. A., LeBlanc, N. R., Certano, A. K., Nachtigall, J., LaBine, K. M., Riddle, J., et al. (2018). Fusarium graminearum: pathogen or endophyte of North American grasses? New Phytol. 217, 1203–1212. doi: 10.1111/nph.14894
Luedemann, A., Strassburg, K., Erban, A., and Kopka, J. (2008). TagFinder for the quantitative analysis of gas chromatography–mass spectrometry (GC-MS)-based metabolite profiling experiments. Bioinformatics 24, 732–737. doi: 10.1093/bioinformatics/btn023
Maciá-Vicente, J. G., Jansson, H.-B., Abdullah, S. K., Descals, E., Salinas, J., and Lopez-Llorca, L. V. (2008). Fungal root endophytes from natural vegetation in Mediterranean environments with special reference to Fusarium spp. FEMS Microbiol. Ecol. 64, 90–105. doi: 10.1111/j.1574-6941.2007.00443.x
Martínez-Medina, A., Van Wees, S. C. M., and Pieterse, C. M. J. (2017). Airborne signals from Trichoderma fungi stimulate iron uptake responses in roots resulting in priming of jasmonic acid-dependent defences in shoots of Arabidopsis thaliana and Solanum lycopersicum: fungal volatiles trigger iron uptake responses and ISR. Plant Cell Environ. 40, 2691–2705. doi: 10.1111/pce.13016
Müller, A., Faubert, P., Hagen, M., Zu Castell, W., Polle, A., Schnitzler, J.-P., et al. (2013). Volatile profiles of fungi–Chemotyping of species and ecological functions. Fungal Genet. Biol. 54, 25–33. doi: 10.1016/j.fgb.2013.02.005
Naznin, H. A., Kimura, M., Miyazawa, M., and Hyakumachi, M. (2013). Analysis of volatile organic compounds emitted by plant growth-promoting fungus Phoma sp. GS8-3 for growth promotion effects on Tobacco. Microbes Environ. 28, 42–49. doi: 10.1264/jsme2.ME12085
O’Donnell, K., Ward, T. J., Robert, V. A. R. G., Crous, P. W., Geiser, D. M., and Kang, S. (2015). DNA sequence-based identification of Fusarium: current status and future directions. Phytoparasitica 43, 583–595. doi: 10.1007/s12600-015-0484-z
Oksanen, J., Blanchet, F. G., Michael, F., Kindt, R., Legendre, P., McGlinn, D., et al. (2017). Vegan: Community Ecology Package. R Package Version 2.4–5.
Owen, S. M., Clark, S., Pompe, M., and Semple, K. T. (2007). Biogenic volatile organic compounds as potential carbon sources for microbial communities in soil from the rhizosphere of Populus tremula. FEMS Microbiol. Lett. 268, 34–39. doi: 10.1111/j.1574-6968.2006.00602.x
Paradis, E., Claude, J., and Strimmer, K. (2004). APE: analyses of phylogenetics and evolution in R language. Bioinforma. Oxf. Engl. 20, 289–290.
Park, Y.-S., Dutta, S., Ann, M., Raaijmakers, J. M., and Park, K. (2015). Promotion of plant growth by Pseudomonas fluorescens strain SS101 via novel volatile organic compounds. Biochem. Biophys. Res. Commun. 461, 361–365. doi: 10.1016/j.bbrc.2015.04.039
Pétriacq, P., Williams, A., Cotton, A., McFarlane, A. E., Rolfe, S. A., and Ton, J. (2017). Metabolite profiling of non-sterile rhizosphere soil. Plant J. 92, 147–162. doi: 10.1111/tpj.13639
Piechulla, B., and Schnitzler, J.-P. (2016). Circumvent CO2 effects in volatile-based microbe–plant interactions. Trends Plant Sci. 21, 541–543. doi: 10.1016/j.tplants.2016.05.001
Ryu, C.-M., Farag, M. A., Hu, C.-H., Reddy, M. S., Wei, H.-X., Pare, P. W., et al. (2003). Bacterial volatiles promote growth in Arabidopsis. Proc. Natl. Acad. Sci. U.S.A. 100, 4927–4932. doi: 10.1073/pnas.0730845100
Sánchez-López,ÁM., Baslam, M., De Diego, N., Muñoz, F. J., Bahaji, A., Almagro, G., et al. (2016). Volatile compounds emitted by diverse phytopathogenic microorganisms promote plant growth and flowering through cytokinin action: VCs from microbial phytopathogens promote growth. Plant Cell Environ. 39, 2592–2608. doi: 10.1111/pce.12759
Schenkel, D., Lemfack, M. C., Piechulla, B., and Splivallo, R. (2015). A meta-analysis approach for assessing the diversity and specificity of belowground root and microbial volatiles. Front. Plant Sci. 6:707. doi: 10.3389/fpls.2015.00707
Schmidt, R., Etalo, D. W., de Jager, V., Gerards, S., Zweers, H., de Boer, W., et al. (2016). Microbial small talk: volatiles in fungal–bacterial interactions. Front. Microbiol. 6:1495. doi: 10.3389/fmicb.2015.01495
Schneider, C. A., Rasband, W. S., and Eliceiri, K. W. (2012). NIH Image to ImageJ: 25 years of image analysis. Nat. Methods 9, 671–675. doi: 10.1038/nmeth.2089
Schulz-Bohm, K., Martín-Sánchez, L., and Garbeva, P. (2017). Microbial Volatiles: small molecules with an important role in intra- and inter-kingdom interactions. Front. Microbiol. 8:2484. doi: 10.3389/fmicb.2017.02484
Schulz-Bohm, K., Zweers, H., de Boer, W., and Garbeva, P. (2015). A fragrant neighborhood: volatile mediated bacterial interactions in soil. Front. Microbiol. 6:1212. doi: 10.3389/fmicb.2015.01212
Sherif, M., Becker, E.-M., Herrfurth, C., Feussner, I., Karlovsky, P., and Splivallo, R. (2016). Volatiles emitted from maize ears simultaneously infected with two Fusarium species mirror the most competitive fungal pathogen. Front. Plant Sci. 7:1460. doi: 10.3389/fpls.2016.01460
Šimpraga, M., Takabayashi, J., and Holopainen, J. K. (2016). Language of plants: Where is the word? Language of plants. J. Integr. Plant Biol. 58, 343–349. doi: 10.1111/jipb.12447
Splivallo, R., Fischer, U., Gobel, C., Feussner, I., and Karlovsky, P. (2009). Truffles regulate plant root morphogenesis via the production of auxin and ethylene. Plant Physiol. 150, 2018–2029. doi: 10.1104/pp.109.141325
Splivallo, R., Novero, M., Bertea, C. M., Bossi, S., and Bonfante, P. (2007). Truffle volatiles inhibit growth and induce an oxidative burst in Arabidopsis thaliana. New Phytol. 175, 417–424. doi: 10.1111/j.1469-8137.2007.02141.x
Stamatakis, A. (2014). RAxML version 8: a tool for phylogenetic analysis and post-analysis of large phylogenies. Bioinformatics 30, 1312–1313. doi: 10.1093/bioinformatics/btu033
Tholl, D., and Röse, U. (2006). “Detection and identification of floral scent compounds,” in Biology of Floral Scent, eds E. Pichersky and N. Dudareva (Boca Raton, FL: CRC Press), 3–25. doi: 10.1201/9781420004007.sec1
Wenke, K., Wanke, D., Kilian, J., Berendzen, K., Harter, K., and Piechulla, B. (2012). Volatiles of two growth-inhibiting rhizobacteria commonly engage AtWRKY18 function: bacterial volatiles: a new form of stress elicitor. Plant J. 70, 445–459. doi: 10.1111/j.1365-313X.2011.04891.x
Yoshikawa, M., Zhang, M., Kurisu, F., and Toyota, K. (2017). Bacterial degraders of coexisting dichloromethane, benzene, and toluene, identified by stable-isotope probing. Water Air Soil Pollut. 228:418. doi: 10.1007/s11270-017-3604-1
Zain, M. E., El-Sheikh, H. H., Soliman, H. G., and Khalil, A. M. (2011). Effect of certain chemical compounds on secondary metabolites of Penicillium janthinellum and P. duclauxii. J. Saudi Chem. Soc. 15, 239–246. doi: 10.1016/j.jscs.2010.09.004
Zhang, H., Kim, M.-S., Krishnamachari, V., Payton, P., Sun, Y., Grimson, M., et al. (2007). Rhizobacterial volatile emissions regulate auxin homeostasis and cell expansion in Arabidopsis. Planta 226, 839–851. doi: 10.1007/s00425-007-0530-2
Keywords: Fusarium, Arabidopsis, volatile organic compounds, plant-microbe interactions, soil VOCs
Citation: Schenkel D, Maciá-Vicente JG, Bissell A and Splivallo R (2018) Fungi Indirectly Affect Plant Root Architecture by Modulating Soil Volatile Organic Compounds. Front. Microbiol. 9:1847. doi: 10.3389/fmicb.2018.01847
Received: 16 May 2018; Accepted: 24 July 2018;
Published: 13 August 2018.
Edited by:
Caroline Gutjahr, Technische Universität München, GermanyReviewed by:
Ruth Lydia Schmidt, University of Quebec, CanadaMartin Heil, Centro de Investigación y de Estudios Avanzados del Instituto Politécnico Nacional (CINVESTAV-IPN), Mexico
Copyright © 2018 Schenkel, Maciá-Vicente, Bissell and Splivallo. This is an open-access article distributed under the terms of the Creative Commons Attribution License (CC BY). The use, distribution or reproduction in other forums is permitted, provided the original author(s) and the copyright owner(s) are credited and that the original publication in this journal is cited, in accordance with accepted academic practice. No use, distribution or reproduction is permitted which does not comply with these terms.
*Correspondence: Richard Splivallo, cmljaGFyZC5zcGxpdmFsbG9AYTMuZXBmbC5jaA==