- 1College of Urban and Environmental Sciences, Peking University, Beijing, China
- 2College of Resources and Environment, Fujian Agriculture and Forestry University, Fuzhou, China
Syntrophic oxidation of butyrate is catabolized by a few bacteria specialists in the presence of methanogens. In the present study, a highly enriched butyrate-oxidizing consortium was obtained from a wetland sediment in Tibetan Plateau. During continuous transfers of the enrichment, the addition of magnetite nanoparticles (nanoFe3O4) consistently enhanced butyrate oxidation and CH4 production. Molecular analysis revealed that all bacterial sequences from the consortium belonged to Syntrophomonas with the closest relative of Syntrophomonas wolfei and 96% of the archaeal sequences were related to Methanobacteria with the remaining sequences to Methanocella. Addition of graphite and carbon nanotubes for a replacement of nanoFe3O4 caused the similar stimulatory effect. Silica coating of nanoFe3O4 surface, however, completely eliminated the stimulatory effect. The control experiment with axenic cultivation of a Syntrophomonas strain and two methanogen strains showed no effect by nanoFe3O4. Together, the results in the present study support that syntrophic oxidation of butyrate is likely facilitated by direct interspecies electron transfer in the presence of conductive nanomaterials.
Introduction
Methane is an end product of anaerobic food web degrading organic substances in anoxic habitats. Under methanogenic conditions where the electron donors other than protons and CO2 are absent, the complicated organic substances undergo fermentation producing short-chain alcohols and fatty acids as intermediate products (Drake et al., 2009). Secondary fermenters metabolize these products by discharging electrons to protons forming H2 or formate, which are then used by methanogens. This syntrophic cooperation, however, confronts a critical energetic dilemma as the syntrophic bacteria require a sufficiently low concentration of H2 or formate to process electron discharging that is in disfavor of methanogens (Schink, 1997; McInerney et al., 2009; Stams and Plugge, 2009; Schink et al., 2017).
The theory of syntrophic methanogenesis was discovered a half century ago (Bryant et al., 1967). The pioneering work considered H2 as mediator for interspecies electron transfer. Later, formate was found to serve as a similar function (Thiele and Zeikus, 1988; Boone et al., 1989; Dong and Stams, 1995). Using of formate can confer a kinetic advantage due to its faster diffusion rate than H2 in aqueous medium (Boone et al., 1989; Worm et al., 2014). Recently, amino acids like alanine was found to serve as a supplemental carrier for interspecies electron transfer (Walker et al., 2012).Amino acid exchange may not only function for electron transfer but become essential as some syntrophic partners evolve into amino acid auxotrophies over the course of syntrophic cooperation (Embree et al., 2015).
Apart from the mechanisms above, direct interspecies electron transfer (DIET) has been revealed. This process was initially demonstrated in the coculture of two Geobacter species (Summers et al., 2010). Then it was found DIET also occurred between Geobacter and methanogens with ethanol as the sole substrate (Morita et al., 2011). Methanosarcina barkeri and Methanosaeta harundinacea seemed particularly efficient in performing DIET with Geobacter (Rotaru et al., 2014a,b). Strikingly, addition of the electrically conductive granular activated carbon facilitated DIET either between Geobacter species or between Geobacter and Methanosarcina (Liu et al., 2012). In paddy soil enrichments dominated by Geobacter and Methanosarcina, the production of CH4 from ethanol was significantly stimulated in the presence of magnetite nanoparticles (nanoFe3O4) (Kato et al., 2012a). Other conductive materials like biochar, graphite, and carbon cloth were found to promote DIET between Geobacter species and between Geobacter and methanogens (Chen et al., 2014a,b; Zhao et al., 2015a,b). DIET could also occur between Geobacter and nitrate reducer in the presence of conductive nanoFe3O4 (Kato et al., 2012b). Most of these studies, however, used ethanol (occasionally acetate) as substrate with Geobacter as syntrophic bacteria. Geobacter spp. are known to synthesize metallic-like conductive structure or e-pili and outer membrane c type cytochromes for electric conductivity (Lovley and Malvankar, 2015; Malvankar et al., 2015; Rotaru et al., 2015; Lovley, 2017).
Butyrate is a major intermediate during the decomposition of organic residues in anoxic environments. The syntrophic oxidation of butyrate is thermodynamically stricter than ethanol, requiring a much lower H2 partial pressure for the reaction to process (Schink, 1997; Schink et al., 2017). So far, only a few bacteria specialists are found to metabolize butyrate oxidation through obligate syntrophy with methanogens (Sieber et al., 2010, 2012, 2015). The pure cultures of butyrate syntrophs known to date do not contain genomic machinery for e-pili or outer membrane cytochromes found in Geobacter (Sieber et al., 2012, 2014). This information suggests that DIET shall not exist in butyrate oxidation. But the possibility cannot be ruled out if electric connection substitute is provided externally. Such a substitute has in fact been demonstrated in Geobacter-based cocultures. In a coculture of Methanosarcina barkeri with a pilin-deficient Geobacter metallireducens the addition of granular activated carbon restored the otherwise broken DIET activity (Rotaru et al., 2014a). It has also been suggested that nanoFe3O4 can complement the function of pilin-associated c type cytochrome OmcS for DIET in Geobacter sulfurreducens (Liu et al., 2015).
Two kinds of studies with conflict results have been reported on butyrate syntrophy. On the one hand, the studies with environmental enrichments proposed that DIET likely occurred for butyrate oxidation in the presence of the conductive nanoFe3O4 (Li et al., 2015; Zhang and Lu, 2016). On the other hand, the study on the defined coculture showed that the addition of conductive carbon nanotubes (CNTs) stimulated not only the coculture comprising Syntrophomonas wolfei and Methanospirillum hungatei but also some pure culture methanogens (Salvador et al., 2017). Robust conclusions, however, are difficult to obtain from these studies. In case of environment enrichments, microbial compositions were too complicated to tease out explicitly the routes of interspecies electron transfer (Li et al., 2015; Zhang and Lu, 2016). In case of the defined coculture, the influence of CNTs varied depending on methanogen identity (Salvador et al., 2017). Apparently, more researches are needed to evaluate the possibility of DIET in butyrate syntrophy.
In the present study we constructed a highly enriched cultivation from a wetland soil collected from Zoige wetland in Tibetan Plateau. The enrichment was dominated exclusively by Syntrophomonas and Methanobacteria without the detection of Geobacter and other bacteria. We found that addition of nanoFe3O4 and CNTs to the enrichment substantially accelerated syntrophic oxidation of butyrate while the test on a few pure cultures revealed no effect.
Materials and Methods
Soil Sampling
The surface soil samples were collected from an open fen close to the Wetland National Nature Reserve of Zoige located in Qinghai-Tibetan Plateau (33°47′ N, 102°57′ E) (Fu et al., 2015). The Zoige wetland covers a total area of 6,180 km2, with the average altitude of 3,500 m and the mean annual air temperature of around 1°C (Fu et al., 2015). Vegetation and organic debris was removed by hands during the sampling. Ten kilograms of wet soil samples were collected, placed in an ice box and transported to the laboratory within 24 h for immediate processing. The soil sample had the following characteristics: pH 7.5, organic C of 15.26 g kg-1, total N of 1.06 g kg-1, and C:N of 14.4. Soil slurries were prepared by mixing soil samples with autoclaved and degassed water. The slurries were passed through 2-mm sieves to homogenize and remove the coarse materials. Thirty grams of soil slurry was then filled into 50-ml glass bottles with the final soil (d.w.) to water ratio of 1:5. The bottles were closed with butyl stoppers and flushed with N2. Soil slurries were pre-incubated for 21 days at 30°C to reduce electron acceptors prior to the enrichment incubation.
Enrichment Cultivation, Isotope Labeling, and Molecular Analysis
Enrichment incubation was initiated by inoculating 4% (v/v) pre-incubated soil slurry into 60-ml vessels containing 25 ml of Hepes-buffered (30 mM, pH 7.0) fresh medium under a headspace of N2/CO2 (80/20) (Supplementary Figure S4). The basal medium contained MgCl2.6H2O (0.4 g l-1), CaCl2.H2O (0.1 g l-1), NH4Cl (0.1 g l-1), KH2PO4 (0.2 g l-1), KCl (0.5 g l-1), and was supplemented with, vitamin and trace element solutions as described previously (Lü and Lu, 2012). Na2S.9H2O (1.0 mM) was applied to the growth medium together with redox indicator resazurin (0.0005 g l-1). Butyrate was added to a final concentration of 5 mM in the initial four transfers and then increased to 10 mM thereafter. Cysteine was not added to avoid the possible effect of electron shuttle molecules. Magnetite nanoparticles were synthesized as described previously (Kang et al., 1996). Graphite and multi-walled CNTs was purchased from Beijing Dk Nano Technology, China.
Continuous transfers were conducted in the presence of nanoFe3O4 (4.64 mM in Fe in the medium). The inocula for every transfer were taken from the last nanoFe3O4-amended cultivation. For a comparison, same inocula were used to make parallel preparations without nanoFe3O4 in the medium (i.e., the control) (Supplementary Figure S4).
The final cultivation (after 13 transfers) was used to extract microbial DNA following the previous protocol (Ma et al., 2012). DNA samples from both the control and nanoFe3O4 treatment were used to construct bacterial and archaeal clone libraries (Ma et al., 2012). The PCR amplification, cloning, and sequencing followed the previous procedure (Peng et al., 2008; Rui et al., 2009). Phylogenetic trees were constructed using the neighbor-joining algorithm of the MEGA7 program (Kumar et al., 2016), and bootstrap analysis implemented 1,000 replicates. DNA-SIP was performed using the same cultivation. For this purpose, the fully 13C-labeled butyrate (99 atom%; Sigma-Aldrich) was added as substrate. At the end of incubation, the carbon isotopic ratios (δ13C values) of CH4 and CO2 were analyzed by a gas chromatography-isotope ratio mass spectrometry system (Yuan and Lu, 2009). DNA was extracted from the 13C-labeled and non-labeled cultivations and subjected to DNA-SIP procedure through the isopycnic centrifugation and density gradient fractionation of DNA as described previously (Liu et al., 2011; Rui et al., 2011; Gan et al., 2012). The density-resolved DNA gradients were quantified for total bacteria and archaea using real-time quantitative PCR (Qiu et al., 2008; Gan et al., 2012). The fingerprinting of the DNA gradients was conducted using the terminal restriction fragment length polymorphism analysis (T-RFLP) following the protocol described previously (Liu et al., 2011; Rui et al., 2011; Gan et al., 2012).
Tests on the Enrichment
The following materials were used for test on the enriched cultivation: (1) nanoFe3O4; (2) nanoFe3O4 coated with silica prepared as described (Deng et al., 2008; Li et al., 2015); (3) graphite; and (4) CNTs. For the test with CNTs, three further transfers were made. The inocula from the nanoFe3O4 treatment were used to prepare the first transfer (CNT-1) with the addition of 0.4% CNTs (w/v) in the medium. The second transfer (CNT-2) used the inocula from the CNT-1 treatment and incubated with the addition of CNTs or nanoFe3O4 in the medium. The third transfer was prepared to test the effect of CNTs concentration (0.2, 0.4, and 0.8%, w/v) (Supplementary Figure S4).
Tests on Pure Cultures
Three organisms were used for pure culture test. Methanocella conradii (DSM 24694) was isolated and available in our lab (Lü and Lu, 2012). Methanococcus maripaludis (DSM 14266) were purchased from German culture collection DSMZ (Braunschweig, Germany). Syntrophomonas erecta subsp. sporosyntropha (DSM 16215) was a courtesy of Prof. Xiuzhu Dong at the Institute of Microbiology, Chinese Academy of Sciences. The Methanocella conradii was cultivated as described (Lü and Lu, 2012). The Methanococcus maripaludis was grown on 170 kPa of H2/CO2 (80:20, v/v) in a modified DSMZ141 medium containing 100 mM NaCl, 7.87 mM MgCl2.6H2O, and 0.007 mM Fe(NH4)2(SO4)2. The Syntrophomonas erecta was cultivated in medium containing 20 mM sodium crotonate as described previously (Zehnder and Wuhrmann, 1977; Wu et al., 2006). The effect of nanoFe3O4 was tested for these pure culture strains.
Chemical Analyses
Gas samples (0.1 ml) were regularly taken from headspace of incubations with a pressure-lock precision analytical syringe (Baton Rouge, LA, United States). The concentrations of CH4 and CO2 were analyzed using gas chromatographs GC-7890 (Agilent Technologies, United States) equipped with a thermal conductivity detector (Li et al., 2015). Liquid samples (0.5 ml) were taken with sterile syringes and centrifuged 15 min at 17,949 ×g at 4°C. The supernatant was collected, passed through 0.25-μm-pore-size filters, and analyzed for the concentrations of acetate and butyrate with an HPLC-1200 using a Zorbax SB-AQ C18 column (Agilent Technologies, United States) (Zhang et al., 2014).
Nucleotide Sequence Accession Numbers
The sequences of the 16S rRNA clones obtained in this study have been deposited in the EMBL nucleotide sequence database under the following accession numbers: KT203965–KT204256.
Statistical Analysis
Data analyses were carried out using SPSS 16.0 (SPSS Inc., Chicago, IL, United States) software. For all the analyses, the significance level was set at P < 0.05. Sample variability is given as the standard (S.D.) of the mean.
Results
The production of CH4 occurred without a lag in the initial two transfers indicating the readily activity of butyrate oxidation in this wetland sediment (Supplementary Figures S1A,B). Addition of 5 mM butyrate yielded about 10–12 mM CH4 (normalized to liquid volume) in the initial two transfers but then decreased to 2–2.5 mM CH4 in the third and fourth transfers (Supplementary Figures S1C,D). When the concentration of butyrate was increased to 10 mM in the later transfers, about 4 mM of CH4 was obtained (Figure 1). These results indicated that butyrate was stoichiometrically converted to CH4 and CO2 in the first two transfers, while thereafter the aceticlastic methanogens were lost (see more results below, Figures 3A,C) and CH4 was only produced from CO2 reduction by the electrons released from butyrate oxidation (four electrons per butyrate). After this transition, the addition of nanoFe3O4 consistently stimulated CH4 production, with the shorter lag phase and greater maximal rate compared with the control (Figures 1A,B and Supplementary Figures S1C,D). At the third transfer (right after the transition), CH4 production displayed a long lag in the control while it took less than a week before the onset of rapid production in the presence of nanoFe3O4 (Supplementary Figures S1C,D).
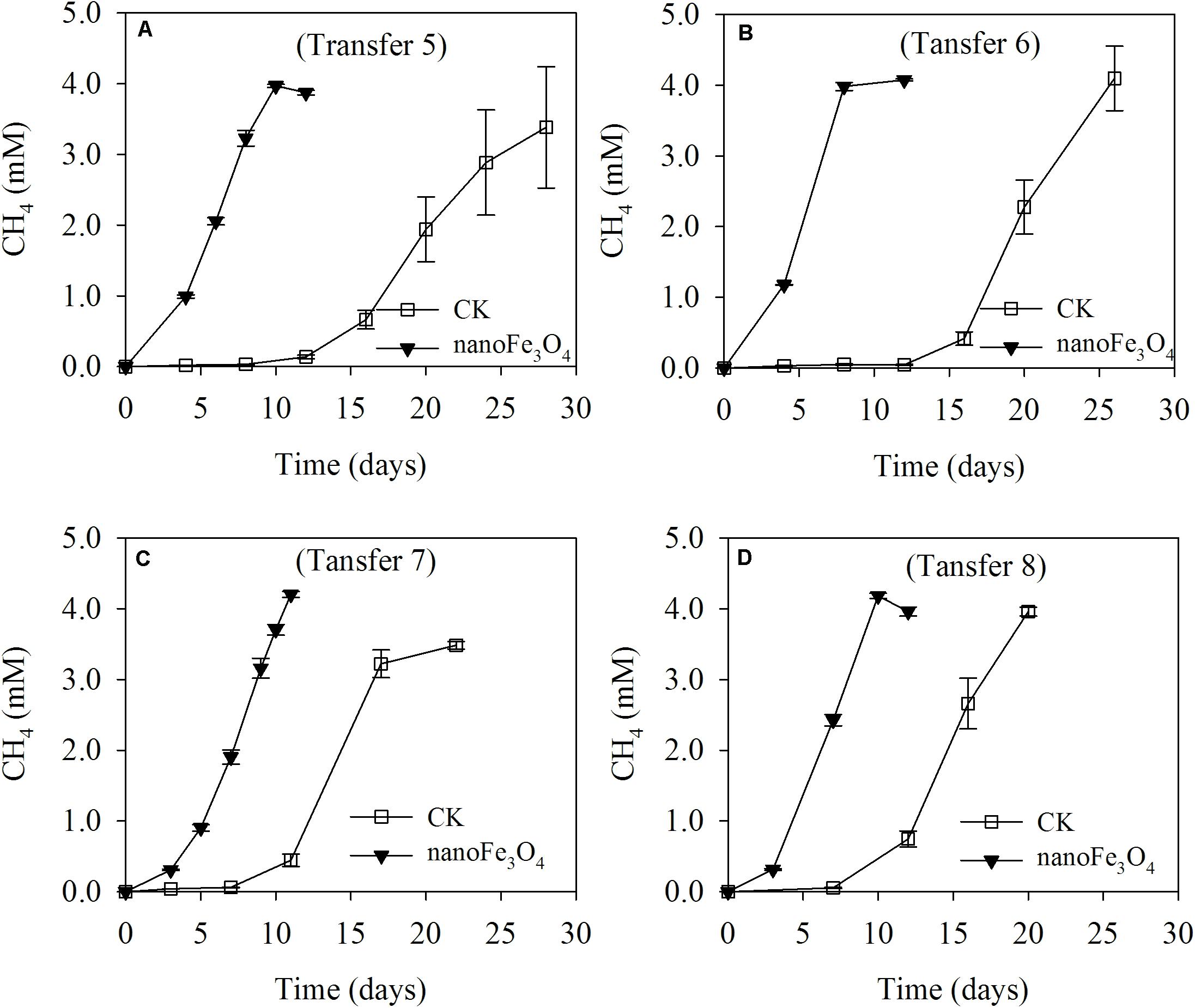
FIGURE 1. Methane production from butyrate oxidation in continuous (the 5th to 8th) transfers (A–D) of wetland enrichment in the presence (nanoFe3O4) and absence (CK) of nanoFe3O4. The concentration of CH4 produced was expressed as micromoles per liter (mM) of incubation medium. The error bars indicate the standard deviations of three replications.
DNA-SIP and clone sequence analyses were used to determine microbial composition in the enriched cultivation. Prior to DNA-SIP, two bacterial and two archaeal clone libraries were constructed, with one each for the control and nanoFe3O4 treatment, respectively. All of the bacterial clone sequences from both the nanoFe3O4 treatment and the control were closely related to a Syntrophomonas wolfei strain (Figure 2A). For the archaeal composition, 94 out of 98 sequences were affiliated to Methanobacteriales (Methanobacterium bryantii as the closest pure culture relative) and the remaining four clones to Methanocellales (Figure 2B).
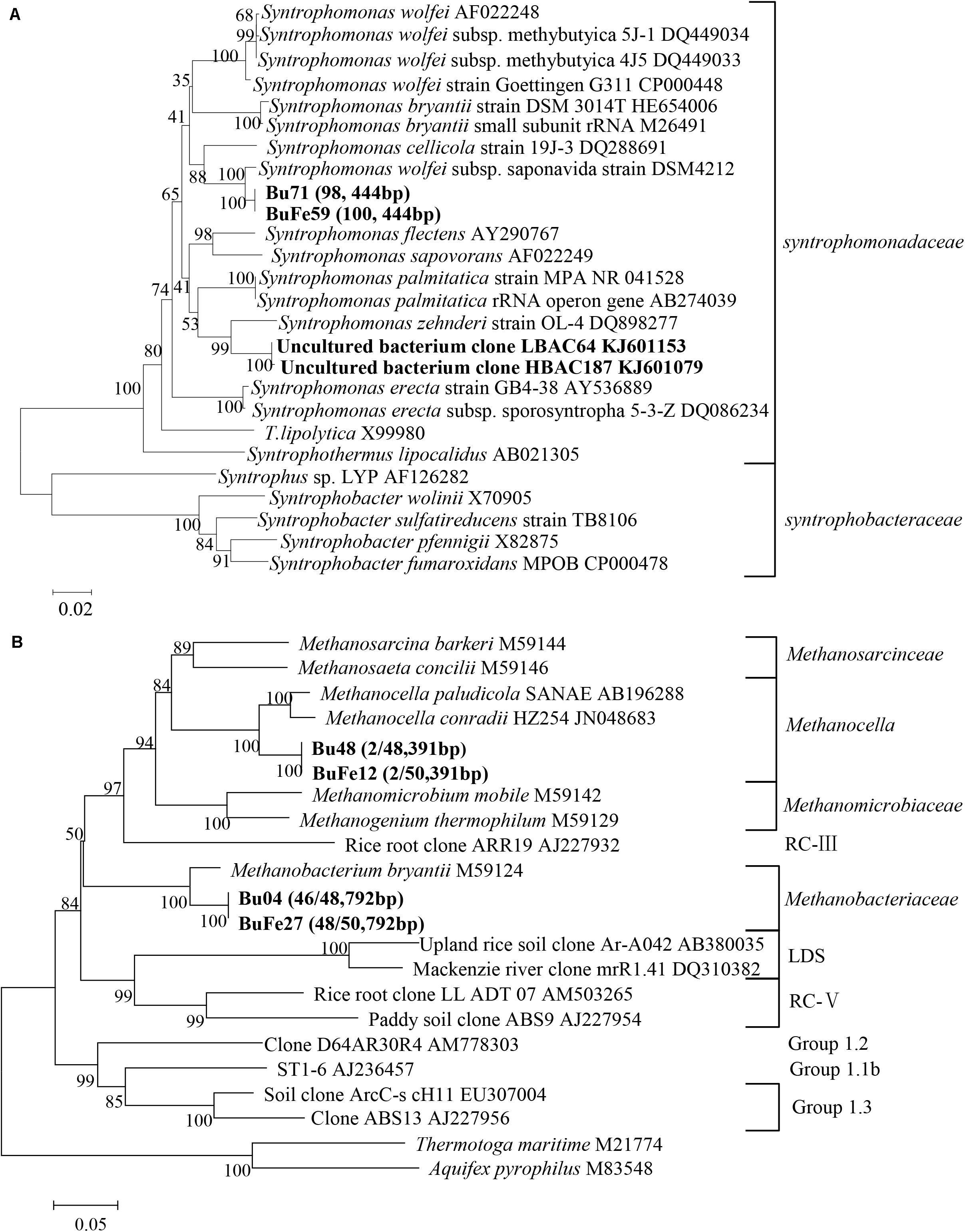
FIGURE 2. Phylogenetic relationship of representative bacterial (A) and archaeal (B) 16S rRNA gene clone sequences. Two bacterial and two archaeal clone libraries were constructed from nanoFe3O4 (BuFe) and the control (Bu) treatment, respectively. Sequences from these libraries were used for constructing the phylogenetic tree. Bootstrap values (%) were generated from 1,000 replications and indicated at individual nodes. The scale bar represents 10% sequence divergence. GenBank accession numbers of the reference sequences are indicated and in silico T-RF sizes are given in parentheses.
Albeit the simplicity of microbial composition in the enrichment, DNA-SIP was still performed by applying the fully 13C-labeled butyrate. Almost identical pattern was observed for the distribution of the density-resolved DNA fragments along the buoyant density gradient between the nanoFe3O4 treatment (Supplementary Figures S2C,D) and the control (Supplementary Figures S2A,B). Notably, the distribution of the archaeal DNA showed no difference between the labeled samples and the non-labeled controls (Supplementary Figures S2A,C), but the distribution of the bacterial DNA shifted to the heavier fractions in the labeled samples compared with the non-labeled control (Supplementary Figures S2B,D). These results indicate that the bacterial populations assimilated 13C from butyrate (regardless of nanoFe3O4 presence), while the archaeal populations did not. The T-RFLP fingerprinting of the density-resolved DNA gradients revealed only one peak for both archaeal and bacterial populations without difference between “heavy” and “light” DNA and between the nanoFe3O4 treatment (Supplementary Figures S3C,D) and the control (Supplementary Figures S3A,B). The T-RF had a size of 792 bp for the archaeal and 444 bp for the bacterial populations, respectively. Comparison to the clone sequences indicates that these T-RFs belong to Methanobacteria spp. and Syntrophomonas spp., respectively. Collectively, the molecular analyses indicate that the enrichment was almost close to be “purified.”
Isotopic and chemical analysis was conducted during the labeling experiment. Incubations with or without labeling showed identical patterns of butyrate consumption, CH4 production and acetate accumulation (Figures 3A,B without and Figures 3C,D with isotopic labeling). In consistence with the transfer incubations, addition of 10 mM butyrate produced about 4.0–4.3 mM CH4 and about 21–24 mM acetate cumulated in the medium (Figure 3). The 13C to 12C ratios of CH4 and CO2 showed no change over the course of incubations, being around 1.0–1.1% of atomic 13C for both CH4 and CO2 (Figures 3B,D) and there was no difference between the 13C labeled incubations (Figure 3D) and the non-labeled control (Figure 3B). These results indicate that CH4 was not labeled albeit the application of the fully 13C-labeled butyrate. Therefore, methanogens utilized only electrons but not carbon from butyrate oxidation.
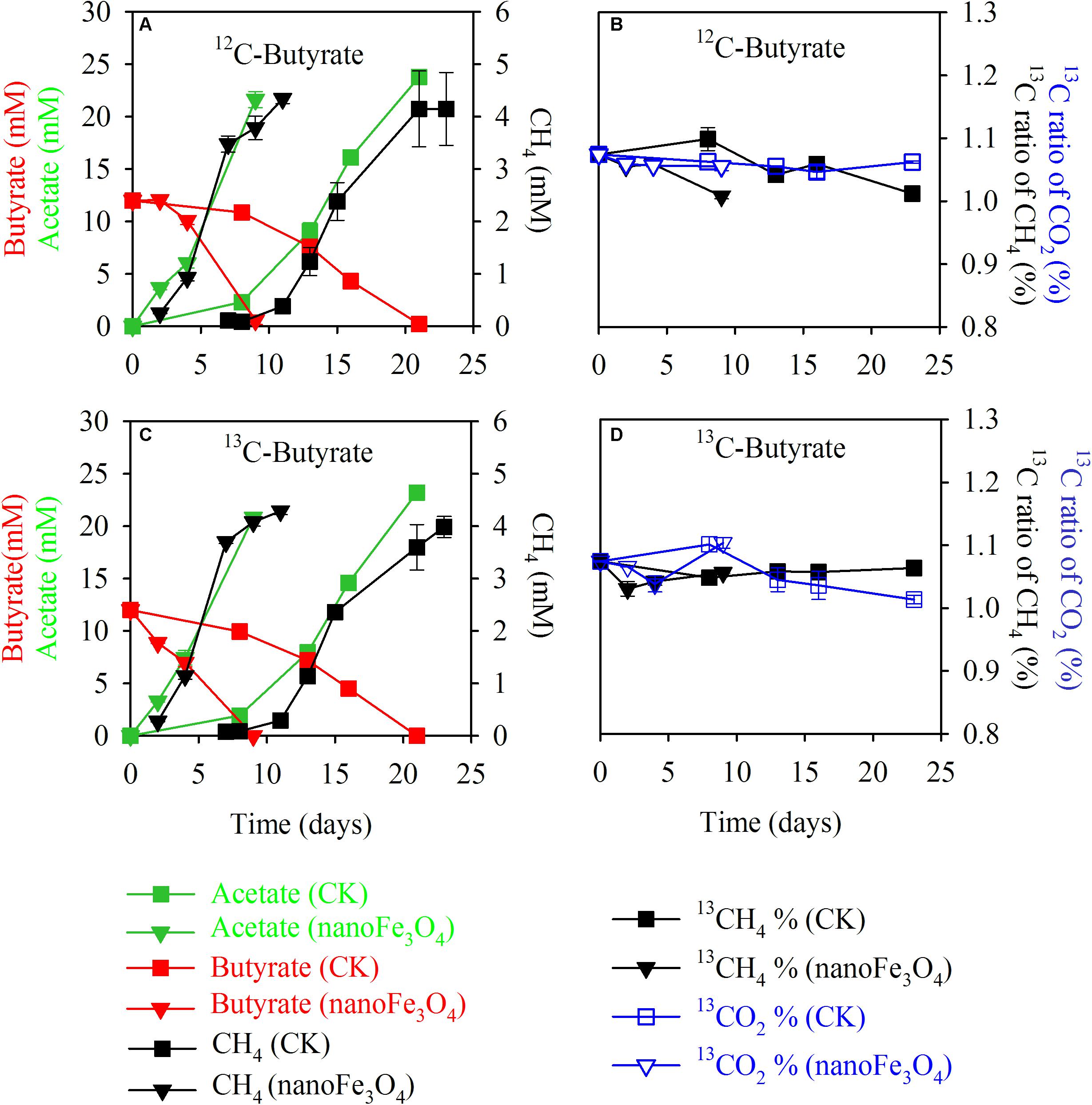
FIGURE 3. Isotopic labeling experiment in the 13th transfer enrichment. The fully 13C-labeled butyrate was applied for the labeling treatment. Shown are the total concentrations of CH4, butyrate, acetate (A,C) and the atomic 13C percentages of CH4 and CO2 (B,D) for the 13C-labeling treatment (C,D) and the non-labeling control (A,B); with nanoFe3O4 (inverted triangles) or without nanoFe3O4 (squares). The total concentrations of acetate, butyrate, and CH4 were colored in green, red, and black, respectively (A,C). The atomic 13C% of CH4 and CO2 were colored in black and blue, respectively (B,D). The error bars indicate the standard deviations of three replications.
Various tests were carried out on the enriched cultivation (Figure 4). Addition of nanoFe3O4 markedly stimulated the production of CH4 as already observed in the transfer incubations. However, silica coating of nanoFe3O4 completely eliminated this stimulatory effect. On the other hand, the addition of graphite retained the stimulatory effect albeit less significant compared with nanoFe3O4. For the CNTs test, three further transfers were performed (Figure 5). A slight positive effect was detected in the first CNTs transfer (Figure 5A). In the second transfer, the first CNTs enrichment was re-inoculated to fresh media in the presence of CNTs or nanoFe3O4. Significant positive effects were observed for both CNTs and nanoFe3O4 (Figure 5B). In the third CNTs transfer, the effect of CNTs concentrations was determined. The stimulatory effect was substantiated at the concentration of 0.4 and 0.8% CNTs (w/v) but diminished when the concentration decreased to 0.2% CNTs.
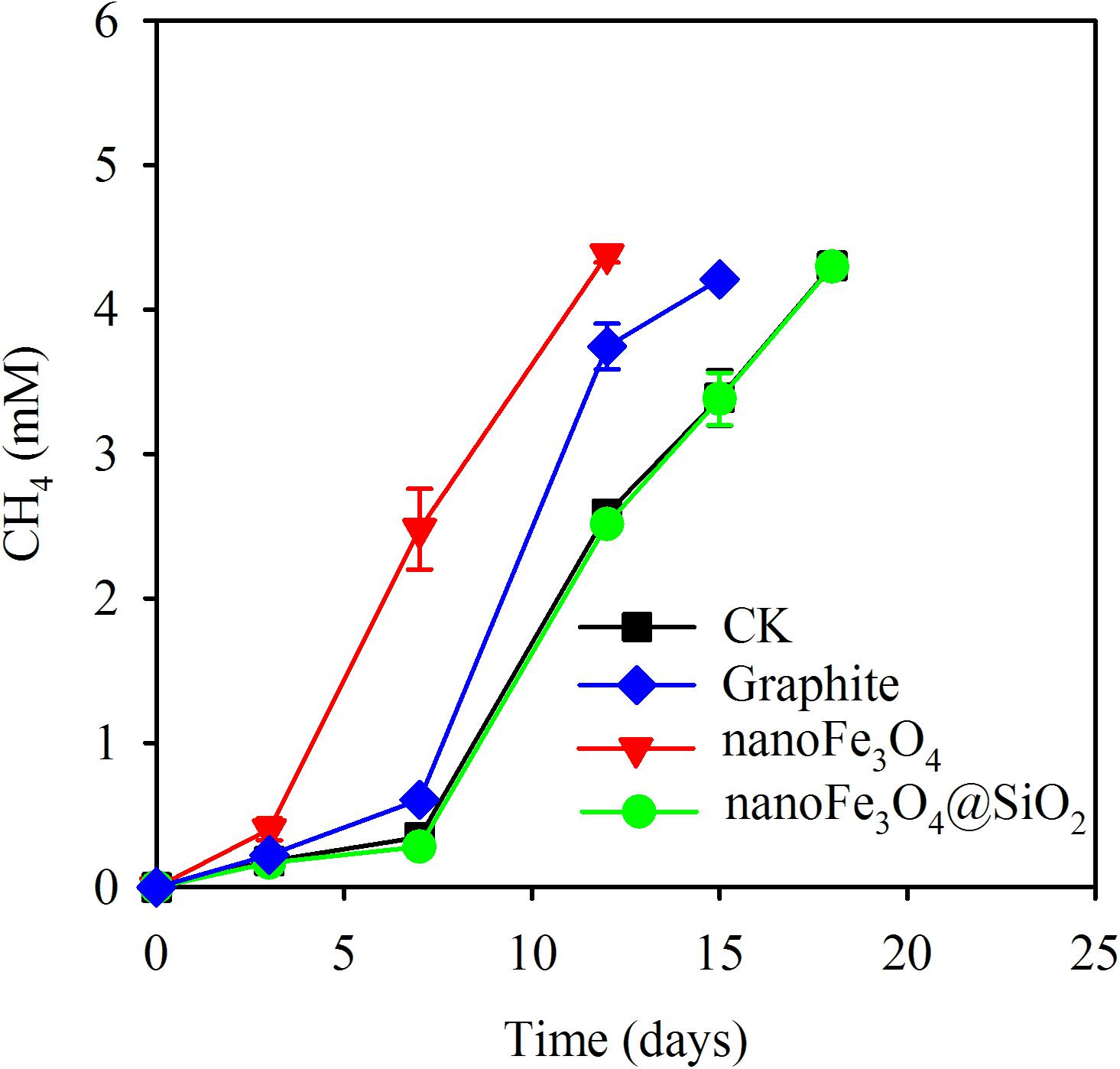
FIGURE 4. Methane production from butyrate oxidation in the 13th transfer of wetland enrichment. Treatments included: (i) addition of nanoFe3O4 (nanoFe3O4); (ii) addition of nanoFe3O4 coated with silica (nanoFe3O4@SiO2); (iii) addition of graphite nanoparticles (Graphite); and (iv) the control without nanomaterials (CK). The error bars indicate the standard deviations of three replications.
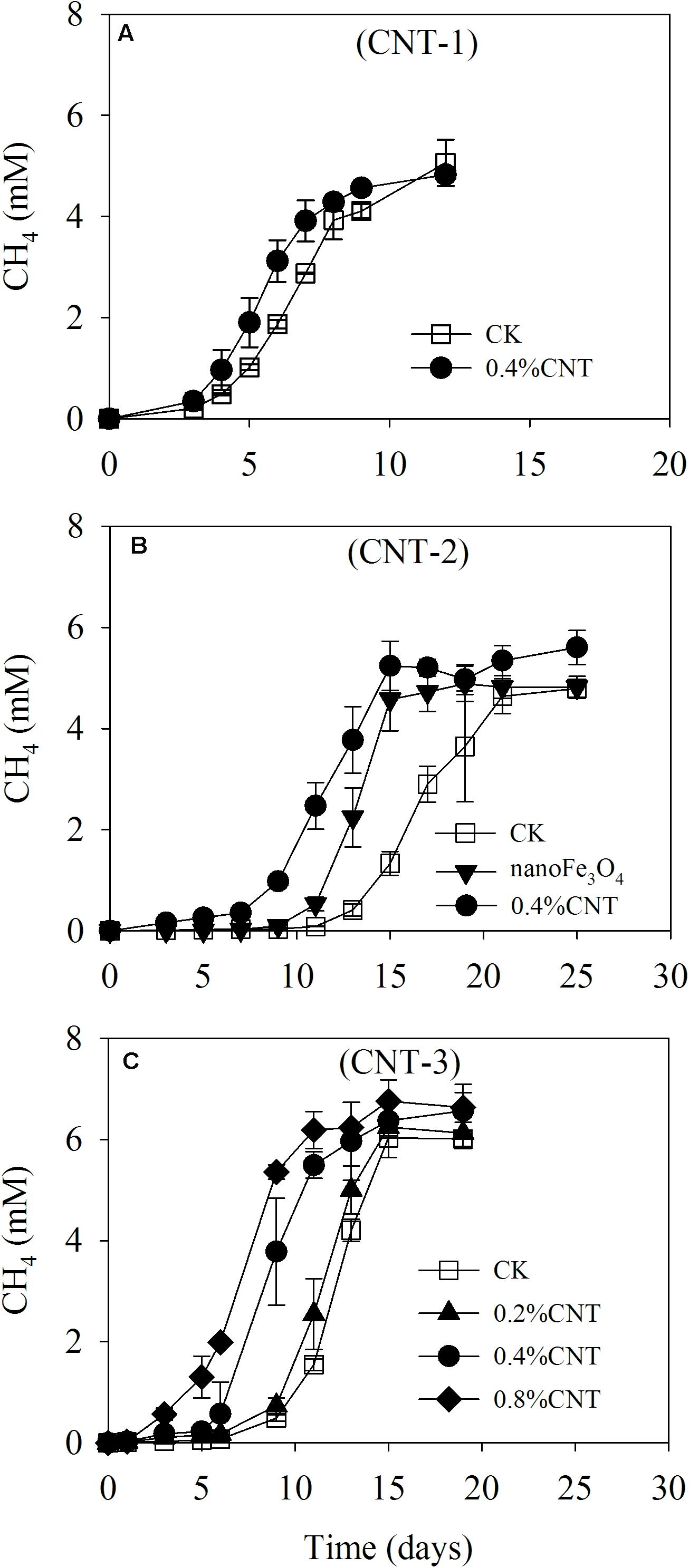
FIGURE 5. Methane production from butyrate oxidation in the enriched incubation in the presence of carbon nanotubes (CNTs). Three continuous transfers were made. The first transfer (A) was inoculated from the 13th transfer of the nanoFe3O4-amended enrichment with the addition of CNTs (0.4% CNT). The second transfer (B) was made by inoculating the first CNT-amended transfer into the fresh media with the addition of nanoFe3O4 (nanoFe3O4) and CNTs (0.4% CNT). The third transfer (C) was prepared by inoculating the second CNT-amended transfer into fresh media of different CNT concentrations, i.e., 0.2, 0.4, and 0.8% (w/v). A control without CNTs (CK) was prepared in parallel for every transfer. The error bars indicate the standard deviations of three replications.
Three pure culture strains were used to test the effect of nanoFe3O4. Incubation of Methanococcus maripaludis (Figure 6A) and Methanocella conradii (Figure 6B) with 170 kPa of H2 produced 43–45 kPa of CH4, indicating the stoichiometric production of CH4 from CO2 reduction by H2. Addition of nanoFe3O4 did not show an effect on CH4 production by these two methanogen strains. Incubation of Syntrophomonas erecta (Figure 6C) with 20 mM crotonate yielded approximately 22 mM acetate and 8.7 mM butyrate, thus more than a half of crotonate was oxidized to acetate and the remaining was reduced to butyrate. Similar to CH4 production, addition of nanoFe3O4 did not reveal an effect on crotonate fermentation in pure culture.
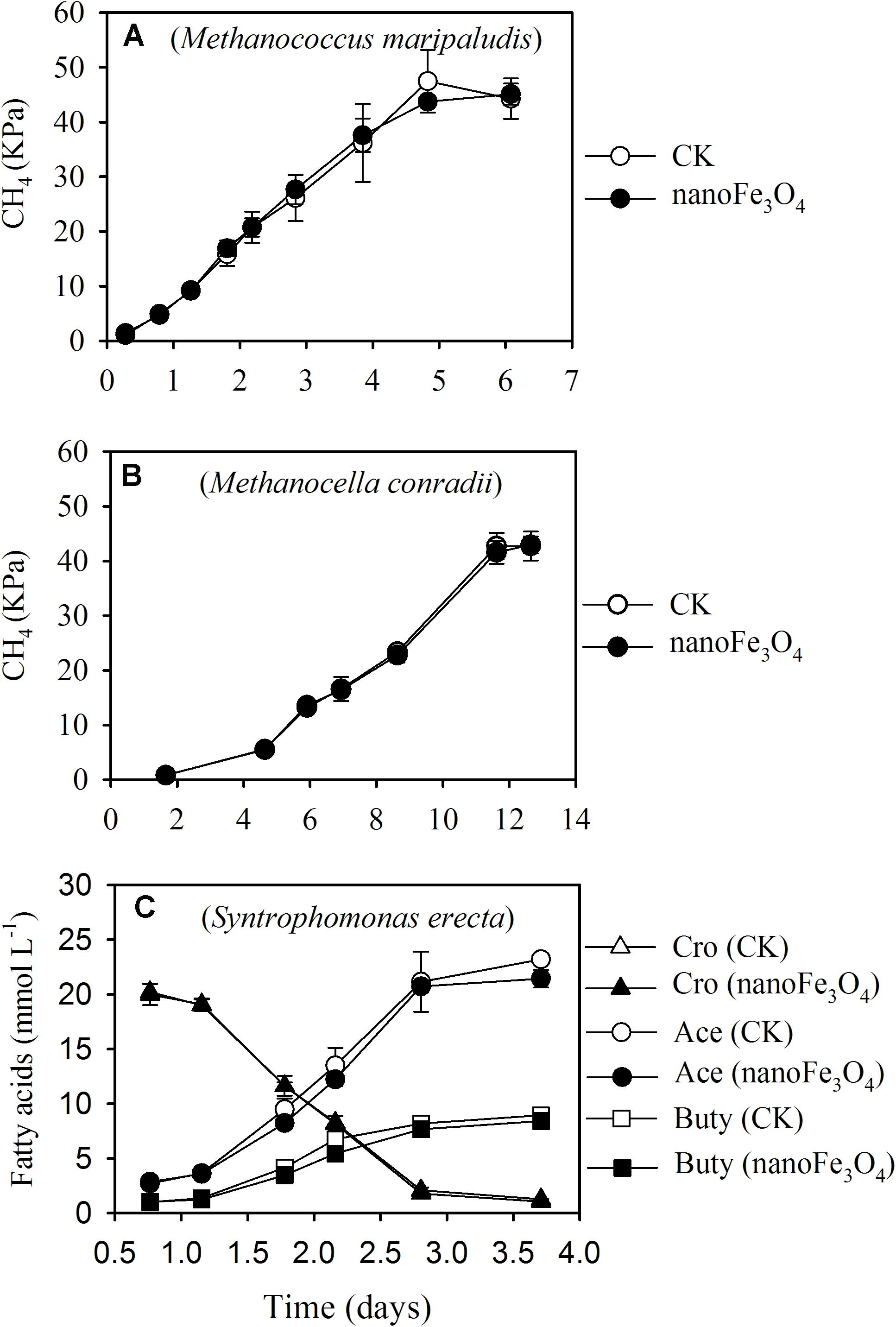
FIGURE 6. Effect of nanoFe3O4 on CH4 production by two hydrogenotrophic methanogens, Methanococcus maripaludis (A) and Methanocella conradii (B), and on crotonate fermentation by a butyrate syntroph Syntrophomonas erecta (C) under axenic conditions. CK, the control without nanoFe3O4. For crotonate fermentation, the concentrations of crotonate (Cro), acetate (Ace), and butyrate (Buty) were monitored. The error bars indicate the standard deviations of three replications.
Discussion
A highly enriched syntrophic consortium was obtained from the wetland sediment after enrichment incubation with continuous transfers in the presence of nanoFe3O4. Mass balance and isotopic labeling indicated that the conversion of butyrate in the enrichment followed the stoichiometric reduction of CO2 by electrons released from butyrate oxidation. Molecular analyses revealed that Syntrophomonas closely related to a Syntrophomonas wolfei strain were left as the only bacteria and methanogens were dominated exclusively by Methanobacteria in addition to a few Methanocella in the enrichment. The aceticlastic methanogens existing initially was possibly lost after a few continuous transfers and Geobacter were not detected. Thus, it can be assumed that butyrate oxidation and CH4 production resulted solely from the syntrophic interaction between Syntrophomonas and Methanobacteria together with a few Methanocella in the enrichment.
The addition of nanoFe3O4 consistently shortened the lag period and enhanced the maximum rate of CH4 production compared with the control without nanoFe3O4. This is consistent with our previous observation that nanoFe3O4 promoted syntrophic oxidation of butyrate in paddy field soil and lake sediment enrichments (Li et al., 2015; Zhang and Lu, 2016). A few other studies showed that nanoFe3O4 promoted syntrophic oxidation of ethanol and propionate in paddy soil and anaerobic bioreactors (Kato et al., 2012a; Viggi et al., 2014; Jing et al., 2017). But microbial compositions in previous enrichments were more complex and often Geobacter species were present. Due to the complicated microbial compositions, it was difficult to pinpoint explicitly the microbial interaction and figure out where the stimulatory effect of nanoFe3O4 was located. The enrichment obtained in the present study, however, was highly enriched without the presence of Geobacter and other bacteria. It can be concluded that the stimulatory effect of nanoFe3O4 is directly due to the response of Syntrophomonas and Methanobacteria/Methanocella.
Different mechanisms may be involved in the stimulatory effect of nanoFe3O4. Firstly, nanoFe3O4 has a relatively low redox potential (Straub et al., 2001). It has been argued that the stimulatory effect by nanomaterials like CNTs on syntrophic coculture and pure culture of methanogens can be due to the decrease in redox potential (Salvador et al., 2017). Similar effect may be postulated for nanoFe3O4. Therefore, we tested its effect on three pure culture strains. The production of CH4 by two hydrogenotrophic methanogens and the fermentation of crotonate by a Syntrophomonas strain revealed no effect by nanoFe3O4. Though not the identical representatives of syntrophs and methanogens in the enrichment, the effect of nanoFe3O4 on individual organisms by reducing the redox potential might be excluded in the present study. Secondly, the physical support of nanoparticles for microbial aggregate formation through adsorption of microbial cells may also result in a stimulatory effect on syntrophy. Accordingly, we modified nanoFe3O4 with silica coating which insulated the electric conductivity of nanoFe3O4 but otherwise retained the physical support for microbial aggregation. Silica coating, however, completely eliminated the stimulatory effect of nanoFe3O4, in consistence with previous study (Li et al., 2015). More tests were then conducted concerning the effect of electric conductivity. We substituted nanoFe3O4 with CNTs and graphite in the enriched consortium. Three transfers were made using CNTs, which all showed the stimulatory effect. Moreover, the effect appeared increasing with the concentration of CNTs in the medium, and re-inoculation of CNT-amended enrichment into nanoFe3O4 medium did not influence the later effect. Application of graphite also showed the enhancement of CH4 production compared with the control, consistent with previous study (Li et al., 2015). Apart from the common property in electric conductivity, nanoFe3O4, CNTs and graphite are different chemically and physically. Together, the results from above tests suggest that the electric conductivity of nanomaterials plays the key role in promoting the syntrophic oxidation of butyrate.
Geobacter species have e-pili and outer membrane c-type cytochromes. Some of Methanosarcina and Methanosaeta species have been demonstrated to perform DIET with Geobacter (Morita et al., 2011; Liu et al., 2012; Rotaru et al., 2014a,b). Recently, it was proposed that DIET also occurred in the anaerobic methanotrophic consortia consisting of ANME-2 with the putative mechanism linked to the presence of large multi-heme cytochromes (McGlynn et al., 2015; Wegener et al., 2015). However, Syntrophomonas species do not contain genomic inventories coding for conductive e-pili and outer membrane cytochromes (Sieber et al., 2012) and unlike Methanosarcina, Methanosaeta, and ANME-2, the Methanobacteria represent methanogens without cytochromes (Thauer et al., 2008). Therefore, it appears hard to conceive that DIET occurs between Syntrophomonas and Methanobacteria. But the essentiality of biological electric connection has been challenged in the experiments using Geobacter mutants (Liu et al., 2012; Rotaru et al., 2014a; Liu et al., 2015). Supplementation of conductive granular activated carbon and magnetite nanoparticles restored DIET in mutants depleted of biological electric connections. Before a better alternate explanation can be uncovered for the stimulatory effect of nanomaterials observed in the present study, we assume that DIET is induced for butyrate oxidation by the biologically compatible conductive nanomaterials. Recently, the membrane associated (reverse) electron transfer chain in Syntrophomonas wolfei has been proposed (Sieber et al., 2015) and the surface-oriented hydrogenases and formate dehydrogenases were abundant in both Syntrophomonas and hydrogenotrophic methanogens (Thauer et al., 2008; Sieber et al., 2012; Worm et al., 2014). It warrants further investigations whether these components can be involved in DIET in concert with H2/formate transfer for the syntrophic oxidation of butyrate.
Conclusion
A highly enriched consortium comprising Syntrophomonas and Methanobacteria-Methanocella was obtained from Tibetan Plateau wetland sediment. The syntrophic production of CH4 from butyrate oxidation was substantially accelerated in the presence of nanoFe3O4. We propose that DIET is likely induced by the added conductive materials in butyrate syntrophy. Mechanisms different from those in Geobacter species may operate in syntrophic butyrate oxidation and shall deserve further investigations. The conductive minerals like magnetite and pyrite are ubiquitous in soils and sediments. Further investigations shall also pay an attention to the effect of these materials on the anaerobic decomposition of organic substances and methanogenesis in those habitats.
Author Contributions
YL conceived the research. LF performed the enrichment cultivation, isotope labeling, and molecular analysis. TS and JZ tested the effect of CNTs on the enrichment. WZ performed the pure culture test. YL wrote the manuscript. YL and LF edited the manuscript. All authors reviewed and approved the manuscript.
Funding
This work was funded by grants from the National Natural Science Foundation (NFSC, no. 41630857) and the National Key Research and Development Program of China (no. 2016YFD0200306) awarded to YL and a grant from NSFC (no. 41601241) awarded to LF.
Conflict of Interest Statement
The authors declare that the research was conducted in the absence of any commercial or financial relationships that could be construed as a potential conflict of interest.
Supplementary Materials
The Supplementary Material for this article can be found online at: https://www.frontiersin.org/articles/10.3389/fmicb.2018.01480/full#supplementary-material
References
Boone, D. R., Johnson, R. L., and Liu, Y. (1989). Diffusion of the interspecies electron carriers H2 and formate in methanogenic ecosystems and its implications in the measurement of Km for H2 or formate Uptake. Appl. Environ. Microbiol. 55, 1735–1741.
Bryant, M. P., Wolin, E. A., Wolin, M. J., and Wolfe, R. S. (1967). Methanobacillus omelianskii, a symbiotic association of two species of bacteria. Arch. Mikrobiol. 59, 20–31. doi: 10.1007/bf00406313
Chen, S., Rotaru, A. E., Liu, F., Philips, J., Woodard, T. L., Nevin, K. P., et al. (2014a). Carbon cloth stimulates direct interspecies electron transfer in syntrophic co-cultures. Bioresour. Technol. 173, 82–86. doi: 10.1016/j.biortech.2014.09.009
Chen, S., Rotaru, A. E., Shrestha, P. M., Malvankar, N. S., Liu, F., Fan, W., et al. (2014b). Promoting interspecies electron transfer with biochar. Sci. Rep. 4:5019. doi: 10.1038/srep05019
Deng, Y., Qi, D., Deng, C., Zhang, X., and Zhao, D. (2008). Superparamagnetic high-magnetization microspheres with an Fe3O4@SiO2 core and perpen-dicularly aligned mesoporous SiO2 shell for removal of microcystins. J. Am. Chem. Soc. 130, 28–29. doi: 10.1021/ja0777584
Dong, X., and Stams, A. J. M. (1995). Evidence for H2 and formate formation during syntrophic butyrate and propionate degradation. Anaerobe 1, 35–39. doi: 10.1016/S1075-9964(95)80405-6
Drake, H. L., Horn, M. A., and Wuest, P. K. (2009). Intermediary ecosystem metabolism as a main driver of methanogenesis in acidic wetland soil. Environ. Microbiol. Rep. 1, 307–318. doi: 10.1111/j.1758-2229.2009.00050.x
Embree, M., Liu, J. K., Al-Bassam, M. M., and Zengler, K. (2015). Networks of energetic and metabolic interactions define dynamics in microbial communities. Proc. Nati. Acad. Sci. U.S.A. 112, 15450–15455. doi: 10.1073/pnas.1506034112
Fu, L., Song, T., and Lu, Y. (2015). Snapshot of methanogen sensitivity to temperature in Zoige wetland from Tibetan plateau. Front. Microbiol. 6:131. doi: 10.3389/fmicb.2015.00131
Gan, Y. L., Qiu, Q. F., Liu, P. F., Rui, J. P., and Lu, Y. H. (2012). Syntrophic oxidation of propionate in rice field soil at 15 and 30°C under methanogenic conditions. Appl. Environ. Microbiol. 78, 4923–4932. doi: 10.1128/aem.00688-12
Jing, Y., Wan, J., Angelidaki, I., Zhang, S., and Luo, G. (2017). iTRAQ quantitative proteomic analysis reveals the pathways for methanation of propionate facilitated by magnetite. Water Res. 108, 212–221. doi: 10.1016/j.watres.2016.10.077
Kang, Y. S., Risbud, S., Rabolt, J. F., and Stroeve, P. (1996). Synthesis and characterization of nanometer-size Fe3O4 and γ-Fe2O3 particles. Chem. Mater. 8, 2209–2211. doi: 10.1021/cm960157j
Kato, S., Hashimoto, K., and Watanabe, K. (2012a). Methanogenesis facilitated by electric syntrophy via (semi)conductive iron-oxide minerals. Environ. Microbiol. 14, 1646–1654. doi: 10.1111/j.1462-2920.2011.02611.x
Kato, S., Hashimoto, K., and Watanabe, K. (2012b). Microbial interspecies electron transfer via electric currents through conductive minerals. Proc. Nati. Acad. Sci. U.S.A. 109, 10042–10046. doi: 10.1073/pnas.1117592109
Kumar, S., Stecher, G., and Tamura, K. (2016). MEGA7: molecular evolutionary genetics analysis version 7.0 for bigger datasets. Mol. Biol. Evol. 33, 1870–1874. doi: 10.1093/molbev/msw054
Lü, Z., and Lu, Y. (2012). Methanocella conradii sp. nov., a thermophilic, obligate hydrogenotrophic methanogen, isolated from Chinese rice field soil. PLoS One 7:e35279. doi: 10.1371/journal.pone.0035279
Li, H., Chang, J., Liu, P., Fu, L., Ding, D., and Lu, Y. (2015). Direct interspecies electron transfer accelerates syntrophic oxidation of butyrate in paddy soil enrichments. Environ. Microbiol. 17, 1533–1547. doi: 10.1111/1462-2920.12576
Liu, F., Rotaru, A. E., Shrestha, P. M., Malvankar, N. S., Nevin, K. P., and Lovley, D. R. (2012). Promoting direct interspecies electron transfer with activated carbon. Energy Environ. Sci. 5, 8982–8989. doi: 10.1039/c2ee22459c
Liu, F., Rotaru, A. E., Shrestha, P. M., Malvankar, N. S., Nevin, K. P., and Lovley, D. R. (2015). Magnetite compensates for the lack of a pilin-associated c-type cytochrome in extracellular electron exchange. Environ. Microbiol. 17, 648–655. doi: 10.1111/1462-2920.12485
Liu, P., Qiu, Q., and Lu, Y. (2011). Syntrophomonadaceae-affiliated species as active butyrate-utilizing syntrophs in paddy field soil. Appl. Environ. Microbiol. 77, 3884–3887. doi: 10.1128/AEM.00190-11
Lovley, D. R. (2017). Syntrophy goes electric: direct interspecies electron transfer. Annu. Rev. Microbiol. 71, 643–664. doi: 10.1146/annurev-micro-030117-20420
Lovley, D. R., and Malvankar, N. S. (2015). Seeing is believing: novel imaging techniques help clarify microbial nanowire structure and function. Environ. Microbiol. 17, 2209–2215. doi: 10.1111/1462-2920.12708
Ma, K., Conrad, R., and Lu, Y. H. (2012). Responses of methanogen mcrA genes and their transcripts to an alternate dry/wet cycle of paddy field soil. Appl. Environ. Microbiol. 78, 445–454. doi: 10.1128/aem.06934-6911
Malvankar, N. S., Vargas, M., Nevin, K., Tremblay, P. L., Evans-Lutterodt, K., Nykypanchuk, D., et al. (2015). Structural basis for metallic-like conductivity in microbial nanowires. Mbio 6:e00084-15. doi: 10.1128/mBio.00084-15
McGlynn, S. E., Chadwick, G. L., Kempes, C. P., and Orphan, V. J. (2015). Single cell activity reveals direct electron transfer in methanotrophic consortia. Nature 526, 531–535. doi: 10.1038/nature15512
McInerney, M. J., Sieber, J. R., and Gunsalus, R. P. (2009). Syntrophy in anaerobic global carbon cycles. Curr. Opin. Biotechnol. 20, 623–632. doi: 10.1016/j.copbio.2009.10.001
Morita, M., Malvankar, N. S., Franks, A. E., Summers, Z. M., Giloteaux, L., Rotaru, A. E., et al. (2011). Potential for direct interspecies electron transfer in methanogenic wastewater digester aggregates. MBio 2:e00159-11. doi: 10.1128/mBio.00159-11
Peng, J., Lu, Z., Rui, J., and Lu, Y. (2008). Dynamics of the methanogenic archaeal community during plant residue decomposition in an anoxic rice field soil. Appl. Environ. Microbiol. 74, 2894–2901. doi: 10.1128/AEM.00070-08
Qiu, Q., Noll, M., Abraham, W. R., Lu, Y., and Conrad, R. (2008). Applying stable isotope probing of phospholipid fatty acids and rRNA in a Chinese rice field to study activity and composition of the methanotrophic bacterial communities in situ. ISME J. 2, 602–614. doi: 10.1038/ismej.2008.34
Rotaru, A. E., Shrestha, P. M., Liu, F., Markovaite, B., Chen, S., Nevin, K. P., et al. (2014a). Direct interspecies electron transfer between Geobacter metallireducens and Methanosarcina barkeri. Appl. Environ. Microbiol. 80, 4599–4605. doi: 10.1128/aem.00895-814
Rotaru, A. E., Shrestha, P. M., Liu, F., Shrestha, M., Shrestha, D., Embree, M., et al. (2014b). A new model for electron flow during anaerobic digestion: direct interspecies electron transfer to Methanosaeta for the reduction of carbon dioxide to methane. Energy Environ. Sci. 7, 408–415. doi: 10.1039/c3ee42189a
Rotaru, A. E., Woodard, T. L., Nevin, K. P., and Lovley, D. R. (2015). Link between capacity for current production and syntrophic growth in Geobacter species. Front. Microbiol. 6:744. doi: 10.3389/fmicb.2015.00744
Rui, J., Qiu, Q., and Lu, Y. (2011). Syntrophic acetate oxidation under thermophilic methanogenic condition in Chinese paddy field soil. FEMS Microbiol. Ecol. 77, 264–273. doi: 10.1111/j.1574-6941.2011.01104.x
Rui, J. P., Peng, J. J., and Lu, Y. H. (2009). Succession of bacterial populations during plant residue decomposition in rice field soil. Appl. Environ. Microbiol. 75, 4879–4886. doi: 10.1128/AEM.00702-09
Salvador, A. F., Martins, G., Melle-Franco, M., Serpa, R., Stams, A. J. M., Cavaleiro, A. J., et al. (2017). Carbon nanotubes accelerate methane production in pure cultures of methanogens and in a syntrophic coculture. Environ. Microbiol. 19, 2727–2739. doi: 10.1111/1462-2920.13774
Schink, B. (1997). Energetics of syntrophic cooperation in methanogenic degradation. Microbiol. Mol. Biol. Rev. 61, 262–280.
Schink, B., Montag, D., Keller, A., and Müller, N. (2017). Hydrogen or formate: alternative key players in methanogenic degradation. Environ. Microbiol. Rep. 9, 189–202. doi: 10.1111/1758-2229.12524
Sieber, J. R., Crable, B. R., Sheik, C. S., Hurst, G. B., Rohlin, L., Gunsalus, R. P., et al. (2015). Proteomic analysis reveals metabolic and regulatory systems involved in the syntrophic and axenic lifestyle of Syntrophomonas wolfei. Front. Microbiol. 6:115. doi: 10.3389/fmicb.2015.00115
Sieber, J. R., Le, H. M., and Mcinerney, M. J. (2014). The importance of hydrogen and formate transfer for syntrophic fatty, aromatic and alicyclic metabolism. Environ. Microbiol. 16, 177–188. doi: 10.1111/1462-2920.12269
Sieber, J. R., Mcinerney, M. J., and Gunsalus, R. P. (2012). Genomic insights into syntrophy: the paradigm for anaerobic metabolic cooperation. Annu. Rev. Microbiol. 66, 429–452. doi: 10.1146/annurev-micro-090110-102844
Sieber, J. R., Sims, D. R., Han, C., Kim, E., Lykidis, A., Lapidus, A. L., et al. (2010). The genome of Syntrophomonas wolfei: new insights into syntrophic metabolism and biohydrogen production. Environ. Microbiol. 12, 2289–2301. doi: 10.1111/j.1462-2920.2010.02237.x
Straub, K. L., Benz, M., and Schink, B. (2001). Iron metabolism in anoxic environments at near neutral pH. FEMS Microbiol. Ecol. 34, 181–186. doi: 10.1111/j.1574-6941.2001.tb00768.x
Stams, A. J., and Plugge, C. M. (2009). Electron transfer in syntrophic communities of anaerobic bacteria and archaea. Nat. Rev. Microbiol. 7, 568–577. doi: 10.1038/nrmicro2166
Summers, Z. M., Fogarty, H. E., Leang, C., Franks, A. E., Malvankar, N. S., and Lovley, D. R. (2010). Direct exchange of electrons within aggregates of an evolved syntrophic coculture of anaerobic bacteria. Science 330, 1413–1415. doi: 10.1126/science.1196526
Thauer, R. K., Kaster, A. K., Seedorf, H., Buckel, W., and Hedderich, R. (2008). Methanogenic archaea: ecologically relevant differences in energy conservation. Nat. Rev. Microbiol. 6, 579–591. doi: 10.1038/nrmicro1931
Thiele, J. H., and Zeikus, J. G. (1988). Control of interspecies electron flow during snaerobic-digestion-significance of formate transfer versus hydrogen transfer during syntrophic methanogenesis in flocs. Appl. Environ. Microbiol. 5, 20–29.
Viggi, C. C., Rossetti, S., Fazi, S., Paiano, P., Majone, M., and Aulenta, F. (2014). Magnetite particles triggering a faster and more robust syntrophic pathway of methanogenic propionate degradation. Environ. Sci. Technol. 48, 7536–7543. doi: 10.1021/es5016789
Walker, C. B., Redding-Johanson, A. M., Baidoo, E. E., Rajeev, L., He, Z., Hendrickson, E. L., et al. (2012). Functional responses of methanogenic archaea to syntrophic growth. ISME J. 6, 2045–2055. doi: 10.1038/ismej.2012.60
Wegener, G., Krukenberg, V., Riedel, D., Tegetmeyer, H. E., and Boetius, A. (2015). Intercellular wiring enables electron transfer between methanotrophic archaea and bacteria. Nature 526, 587–590. doi: 10.1038/nature15733
Worm, P., Koehorst, J. J., Visser, M., Sedano-Nunez, V. T., Schaap, P. J., Plugge, C. M., et al. (2014). A genomic view on syntrophic versus non-syntrophic lifestyle in anaerobic fatty acid degrading communities. Biochim. Biophys. Acta 1837, 2004–2016. doi: 10.1016/j.bbabio.2014.06.005
Wu, C., Liu, X., and Dong, X. (2006). Syntrophomonas erecta subsp. sporosyntropha subsp. nov., a spore-forming bacterium that degrades short chain fatty acids in co-culture with methanogens. Syst. Appl. Microbiol. 29, 457–462. doi: 10.1016/j.syapm.2006.01.003
Yuan, Q., and Lu, Y. (2009). Response of methanogenic archaeal community to nitrate addition in rice field soil. Environ. Microbiol. Rep. 1, 362–369. doi: 10.1111/j.1758-2229.2009.00065.x
Zehnder, A. J. B., and Wuhrmann, K. (1977). Physiology of a Methanobacterium strain AZ. Arch. Microbiol. 111, 199–205. doi: 10.1007/BF00549357
Zhang, C., Yuan, Q., and Lu, Y. H. (2014). Inhibitory effects of ammonia on methanogen mcrA transcripts in anaerobic digester sludge. FEMS Microbiol. Ecol. 87, 368–377. doi: 10.1111/1574-6941.12229
Zhang, J., and Lu, Y. (2016). Conductive Fe3O4 nanoparticles accelerate syntrophic methane production from butyrate oxidation in two different lake sediments. Front. Microbiol. 7:1316. doi: 10.3389/fmicb.2016.01316
Zhao, Z., Zhang, Y., Wang, L., and Quan, X. (2015a). Potential for direct interspecies electron transfer in an electric-anaerobic system to increase methane production from sludge digestion. Sci. Rep. 5:11094. doi: 10.1038/srep11094
Keywords: syntrophy, butyrate, direct interspecies electron transfer, methanogenesis, wetland, Tibetan Plateau
Citation: Fu L, Song T, Zhang W, Zhang J and Lu Y (2018) Stimulatory Effect of Magnetite Nanoparticles on a Highly Enriched Butyrate-Oxidizing Consortium. Front. Microbiol. 9:1480. doi: 10.3389/fmicb.2018.01480
Received: 27 February 2018; Accepted: 13 June 2018;
Published: 05 July 2018.
Edited by:
Dennis A. Bazylinski, University of Nevada, Las Vegas, United StatesReviewed by:
Isao Yumoto, National Institute of Advanced Industrial Science and Technology (AIST), JapanCaroline M. Plugge, Wageningen University & Research, Netherlands
Laudemir Carlos Varanda, Universidade de São Paulo, Brazil
Copyright © 2018 Fu, Song, Zhang, Zhang and Lu. This is an open-access article distributed under the terms of the Creative Commons Attribution License (CC BY). The use, distribution or reproduction in other forums is permitted, provided the original author(s) and the copyright owner(s) are credited and that the original publication in this journal is cited, in accordance with accepted academic practice. No use, distribution or reproduction is permitted which does not comply with these terms.
*Correspondence: Yahai Lu, bHV5aEBwa3UuZWR1LmNu