- 1USC 1366 INRA, Institut des Sciences de la Vigne et du Vin, Unité de Recherche Œnologie EA 4577, University of Bordeaux, Bordeaux, France
- 2School of Agriculture, Food and Wine, The University of Adelaide, Adelaide, SA, Australia
- 3Microflora-ADERA, Institut des Sciences de la Vigne et du Vin, Unité de Rrecherche Œnologie EA 4577, Bordeaux, France
- 4Bordeaux Sciences Agro, Gradignan, France
- 5École Nationale Supérieure de Chimie de Biologie et de Physique, Institut Polytechnique de Bordeaux, Bordeaux, France
The yeast species Brettanomyces bruxellensis is associated with important economic losses due to red wine spoilage. The most common method to prevent and/or control B. bruxellensis spoilage in winemaking is the addition of sulfur dioxide into must and wine. However, recently, it was reported that some B. bruxellensis strains could be tolerant to commonly used doses of SO2. In this work, B. bruxellensis response to SO2 was assessed in order to explore the relationship between SO2 tolerance and genotype. We selected 145 isolates representative of the genetic diversity of the species, and from different fermentation niches (roughly 70% from grape wine fermentation environment, and 30% from beer, ethanol, tequila, kombucha, etc.). These isolates were grown in media harboring increasing sulfite concentrations, from 0 to 0.6 mg.L-1 of molecular SO2. Three behaviors were defined: sensitive strains showed longer lag phase and slower growth rate and/or lower maximum population size in presence of increasing concentrations of SO2. Tolerant strains displayed increased lag phase, but maximal growth rate and maximal population size remained unchanged. Finally, resistant strains showed no growth variation whatever the SO2 concentrations. 36% (52/145) of B. bruxellensis isolates were resistant or tolerant to sulfite, and up to 43% (46/107) when considering only wine isolates. Moreover, most of the resistant/tolerant strains belonged to two specific genetic groups, allowing the use of microsatellite genotyping to predict the risk of sulfur dioxide resistance/tolerance with high reliability (>90%). Such molecular diagnosis could help the winemakers to adjust antimicrobial techniques and efficient spoilage prevention with minimal intervention.
Introduction
Winemakers manage the transformation of must into wine through various processes, aiming to obtain high quality product according to their wishes and the expectations of their customers. However, wine chemical and microbiological properties are in constant evolution throughout the winemaking process, and some parameters are difficult to control. Yeast metabolism is one of the multiple factors shaping wine aromatic and flavor properties by contributing to its complexity or, in some cases, leading to undesirable aromas (Fleet, 2003). One example of such phenomenon is wine spoilage by Brettanomyces bruxellensis, a yeast species related to production of off-aromas perceived as barnyard, horse sweat, or medicinal (Heresztyn, 1986; Chatonnet et al., 1992). Prevention methods against B. bruxellensis development include spoilage risk evaluation, SO2 addition, the use of biocontrol agents, e.g., through the inoculation/co-inoculation of various species and/or strains of yeast and bacteria (Berbegal et al., 2017, 2018), etc. If B. bruxellensis is detected, different elimination techniques exist which could be roughly divided in physical (filtering, the use of electric current, pressure, temperature, ultrasonics, etc.) and chemical (SO2, chitosan, DMDC, yeast-derived killer toxins, etc.), see for details (Delfini et al., 2002; Lustrato et al., 2010; Francesca and Maurizio, 2011; Luo et al., 2012; Umiker et al., 2013; Mehlomakulu et al., 2014; Fabrizio et al., 2015; Taillandier et al., 2015; González-Arenzana et al., 2016, 2018; Petrova et al., 2016; Berbegal et al., 2017). Still, the most common method to prevent and/or control B. bruxellensis spoilage remains the addition of sulfur dioxide into must and wine, with regular adjustments if needed. Sulfites are used in winemaking at least since the 18th century and are introduced either through the burning of sulfur tablets in barrels, or in liquid form, mainly through addition of potassium bisulfite solution to must and wine (Ribéreau-Gayon et al., 2006). Sulfur dioxide is broadly used in winemaking not only for its antiseptic action, but also for its antioxidant and antioxidasic properties (Ribéreau-Gayon et al., 2006). Thus, SO2 addition is the preferred choice when it comes to B. bruxellensis spoilage prevention. Unfortunately, over the last years, some B. bruxellensis strains were reported to be tolerant to commonly used doses of SO2, with a high variability amongst isolates (Barata et al., 2008; Curtin et al., 2012; Agnolucci et al., 2014). This variability makes the prediction of B. bruxellensis spoilage potential and the choice of adequate antimicrobial agent a challenge for winemakers. Recently, it was shown that B. bruxellensis SO2 sensitivity correlates with genotype defined by both AFLP and microsatellite markers (Curtin et al., 2012; Avramova et al., 2018). The former study analyzed a total of 41 isolates, with a focus on Australian wine strains. The latter study assessed the intraspecific genetic diversity of a larger number of isolates (1488 strains from 29 countries and 5 types of fermentation niches). Microsatellite genotype analysis revealed that the population was structured according to ploidy level (some clusters being mainly composed of diploid isolates, whereas others – of triploid ones). Statistical analysis of the generated data highlighted that both substrate of isolation and geographical origin of the isolates contribute to the observed population structure. The results suggested an anthropic influence on the spatial biodiversity of B. bruxellensis. The hypothesis of human-related factors effect on the population was further supported by the correlation between genotypic clustering and tolerance to SO2, the main antimicrobial agent used by winemakers. In particular, among the six main clusters of B. bruxellensis population (Avramova et al., 2018), two genetic clusters (AWRI1499-like and L0308-like) were highlighted to comprise isolates with high SO2 tolerance (Avramova et al., 2018). However, SO2 sensitivity was tested on a limited number of isolates (39), particularly for the L0308-like cluster (2 isolates). Thus, the aims of this study were (i) to extend the screening of SO2 sensitivity to 106 additional isolates and thus confirm/infirm the correlation between genetic clusters and SO2 sensitivity to a larger collection representative of the global B. bruxellensis population and (ii) to validate the applicability of a method allowing the prediction of B. bruxellensis SO2 sensitivity through genetic markers analysis.
Materials and Methods
Strains
In this study, 106 strains – in addition to the 39 strains tested previously (Avramova et al., 2018) – from different geographical and industrial fermentation origins were used based on their microsatellite profile (full protocol details and population dendrogram assessment in Avramova et al., 2018). Twelve microsatellite markers were used for genotyping, and a dendrogram was produced using Bruvo’s distance and Neighbor Joining (NJ) clustering. Those strains were evaluated for their tolerance to SO2 using the same protocol as previously described (Avramova et al., 2018) (details in the section “Sulfite Tolerance Assessment”) which made possible the combination of both datasets together to give a total of 145 strains (Table 1 and Figure 1).
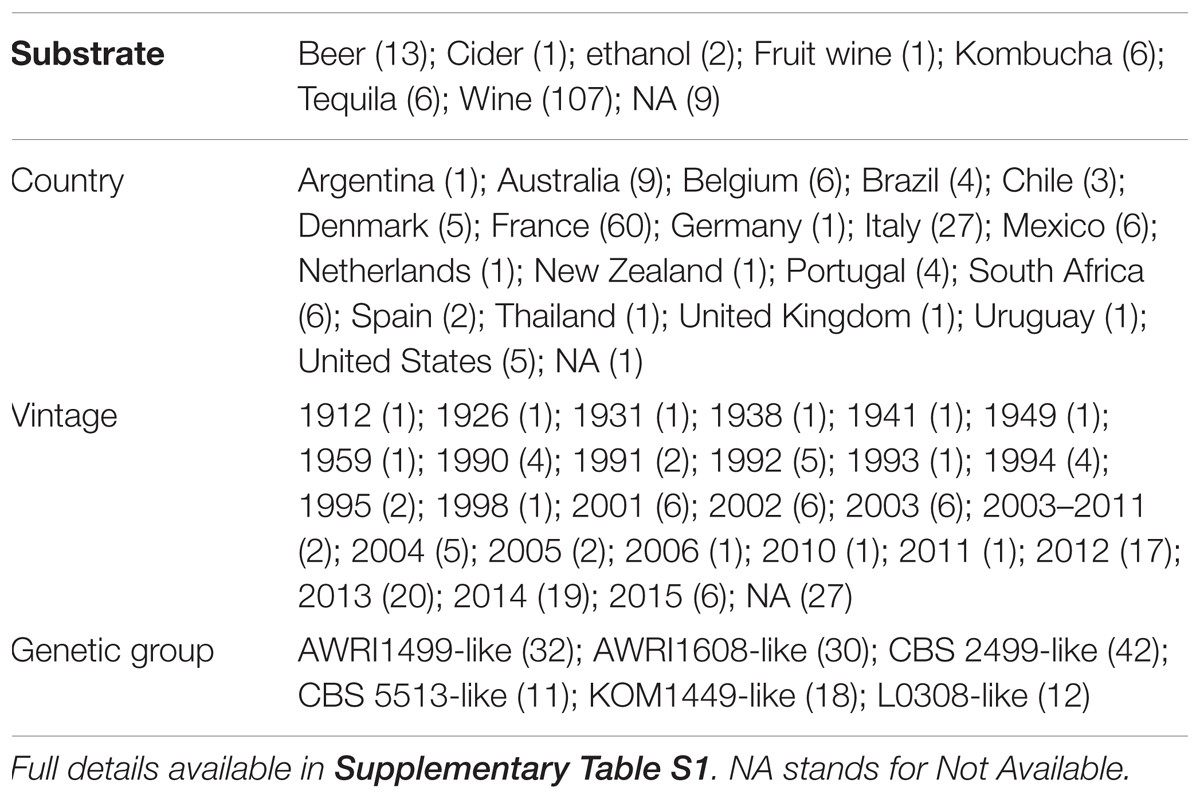
TABLE 1. Summary of the collection of 145 Brettanomyces bruxellensis strains used for sulfur dioxide tolerance assay.
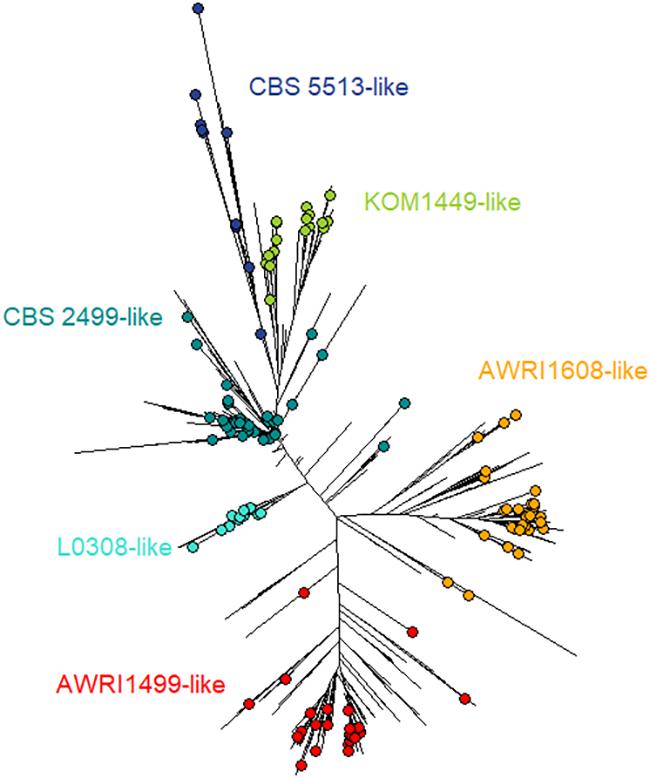
FIGURE 1. Dendrogram tree showing the 145 phenotyped B. bruxellensis isolates. The dendrogram tree includes 1488 isolates, and was built using 12 microsatellite markers, Bruvo’s distance, and NJ clustering, as described previously (Avramova et al., 2018). The 145 isolates used in this work are represented by colored circles. The six different colors correspond to the main genetic groups identified and were named from one isolate (e.g., L0308-like means genetic group close to L0308 strain).
Sulfite Tolerance Assessment
The assay was performed in liquid medium containing 6.7 g.L-1 of YNB (DifcoTM Yeast Nitrogen Base, Becton, Dickinson and Company), 2.5 g.L-1 D-glucose, 2.5 g.L-1 D-Fructose, 5% (v/v) ethanol and increasing concentrations of potassium metabisulfite (PMB, K2S2O5, Thermo Fischer Scientific) in order to obtain 0, 0.2, 0.4, and 0.6 mg.L-1 mSO2 final concentrations. For the calculation of mSO2 it was considered that K2S2O5 corresponds to about 50% of total SO2 (therefore a solution of 10 g.L-1 K2S2O5 corresponds to approximately 5 g.L-1 total SO2). In order to deduce the final mSO2 concentration, the free SO2 concentration was assessed by aspiration/titration method. Then, the mSO2 was calculated by using the Henderson–Hasselbalch equation on dissociation constant pK1 (Divol et al., 2012). Ethanol concentration (5%) was chosen to allow growth of all strains, isolated from wine as well as from other fermentation niches with lower initial ethanol content. Final pH was adjusted to 3.5 (corresponding to an average value for pH generally encountered in red winemaking conditions) with phosphoric acid (1 M H3PO4) and the four media (corresponding to the four different concentrations of SO2) were filtered separately with 0.22 μm pore filter (Millipore).
Small-scale fermentations were performed in sterile 4 mL spectrophotometer cuvettes containing a sterile magnet stirrer (Dutscher, France). The cells were grown on YPD agar and inoculated into the YNB-based medium without SO2. After 96 h of pre-culture (the point at which all strains reached stationary phase), the cells were inoculated at OD600nm 0.1 in a final volume of 3 mL. The inoculated medium was then covered with 300 μL of sterile silicone oil (Sigma-Aldrich) to avoid oxidation of the medium which could favor the free SO2 consumption. Then, the cuvette was capped with a plastic cap (Dutscher) and sealed with parafilm. A sterile needle was added by piercing the cap to allow CO2 release. These so-called nano-fermenters were then placed in a spectrophotometer cuvettes container box and on a 15 multi-positions magnetic stirrer plate at 25°C (the final temperature in the nano-fermenters was therefore 29°C due to the stirrer heating). Optical density (OD600nm) was measured every 24 h during at least 150 h to follow cell population growth until stationary phase was reached.
Growth Parameter Calculation and Statistical Analyses
For each growth curve, the following three parameters were calculated: ODmax was the maximal OD reached at 600 nm and corresponded to the maximal population size, the lag phase (in hours) was the time between inoculation and the beginning of cell growth (5% maximal OD increase), and finally, the maximal growth rate was calculated (maximal number of division per hour based on the OD measurement divided by time).
Non-parametric Kruskal–Wallis tests were performed (α = 5%) to identify the means that were significantly different. All statistical analyses and graphs were produced using R language (R Development Core Team, 2010).
Results
Growth Behavior in Presence of SO2
The growth behavior of 145 strains of B. bruxellensis was evaluated regarding sensitivity to sulfite treatment. The selected strains were distributed amongst the six main genetic groups defined using microsatellite markers and were representative of the genetic diversity of the species (Figure 1): CBS 2499-like, KOM1449-like, AWRI1608-like, AWRI1499-like, CBS 5513-like, and L0308-like groups were represented by 42, 18, 30, 32, 11, and 12 strains, respectively (Table 2). A total of >2050 small-scale fermentations were performed, corresponding to each strain tested at increasing concentrations of mSO2 (0, 0.2, 0.4, and 0.6 mg.L-1) at least in triplicate. The strains had different response to sulfur dioxide concentrations in means of lag phase, maximal growth rate, and maximum OD. Depending on the growth parameters’ variation (Supplementary Table S1), three growth behaviors were defined (Figure 2). Sensitive strains showed significantly longer lag phase and slower growth rate and/or lower maximum OD in presence of increasing concentrations of SO2: for example, strain B002-14 T14 7 (Figure 2) showed 22.4, 39.7, 99.2, and 173.4 h of lag phase with 0, 0.2, 0.4, and 0.6 mg.L-1 mSO2, respectively. Maximal growth rate decreased along sulfite concentration with 0.09, 0.06, 0.02, and 0.01 division/h, and ODmax decreased drastically with 1.42, 1.27, 0.77, and 0.09 OD. The same pattern (increased lag-phase, decreased growth rate, and decreased ODmax) was observed for strains 12AVB1 and 2OT14_02 (Figure 2). The degree of sensitivity varied depending on the isolates: some strains showed low growth in presence of 0.2 mg.L-1 mSO2 like strain CBS 3025 which ODmax drops from 1.92 to 0.13 at 0 and 0.2 mg.L-1 mSO2, respectively, or strain 12AVB1 that shows a twofold decrease of ODmax between 0 and 0.2 mg.L-1 mSO2 (1.46 to 0.63, see Supplementary Table S1). Other isolates showed close to normal growth at 0.2 mg.L-1 mSO2 (ODmax > 1), but low/no growth at 0.4 mg.L-1 mSO2 (AWRI1615, L02/E2 AZ, L14160, L14186, YJS5447, etc.). Finally, other strains, although showing a significant growth decrease, were still able to show moderate growth at 0.6 mg.L-1 mSO2: for example, lag-phase of UWOPS 92–297.4 was drastically impacted, from 7 and 10 h (0 and 0.2 mg.L-1 mSO2) to 154 and 171 h (0.4 and 0.6 mg.L-1 mSO2). Its ODmax was also clearly impacted, ranging from 1.29 to 0.54 (at 0 and 0.6 mg.L-1 mSO2, respectively), yet with a residual growth. In conclusion, all strains considered to be sensitive had significantly longer lag phase and slower growth rate and/or lower maximum OD in presence of increasing concentrations of SO2. However, the sulfite concentration at which growth began to be impacted varied, as well as the level of growth’s decrease.
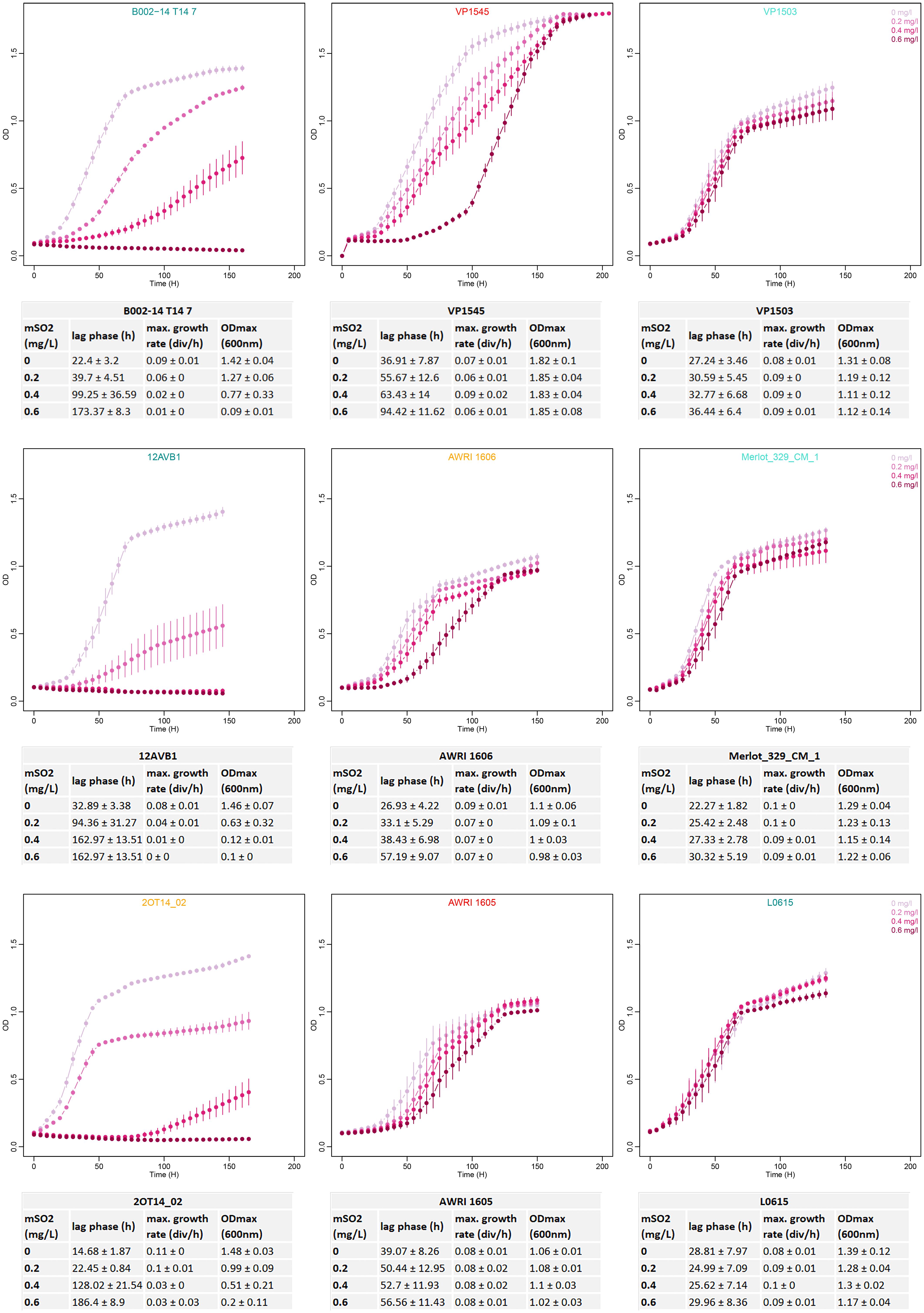
FIGURE 2. Examples of B. bruxellensis sensitive, tolerant, and resistant behavior at four mSO2 concentrations. Strains B002-14 T14 7, 12AVB1, and 2OT14_02 represent sensitive strains. VP1545, AWRI 1606, and AWRI 1605 are tolerant isolates and VP1503, Merlot_329_CM_1, and L0615 are examples of resistant strains. Each curve is built using the mean of three to four replicates, and error bars represent standard deviations and curve colors correspond to increasing SO2 concentration (light pink 0 mg/L mSO2 to dark pink 0.6 mg/L mSO2). The estimated growth parameters (lag phase, maximal growth rate, and maximal OD) are shown below each curve, with mean ± standard deviation.
By contrast, tolerant strains displayed increased lag phase with SO2 increase, while others growth parameters (maximal growth rate and maximal OD) remained statistically unchanged (Kruskal–Wallis test, α = 0.05). For example, strain VP1545 (Figure 2) showed varying lag phase (36.9, 55.7, 63.4, and 94.4 h at 0, 0.2, 0.4, and 0.6 mg.L-1 mSO2, respectively), but unchanged maximal growth rate (0.07–0.09 division/h) and ODmax (1.82–1.85 OD). The same pattern is observed for AWRI 1606 (lag-phase ranging from 27 to 57 h) or AWRI 1605 (lag-phase between 39 and 57 h). Finally, strains for which none parameters were significantly impacted whatever the SO2 concentrations were considered as resistant: VP1503 (Figure 2) had unchanged lag phase of 27.2 to 36.4 h, maximal growth rate of 0.08–0.09 division/h and ODmax of 1.11–1.31 OD. Identically, Merlot_329_M_1 and L0615 showed identical growth’s kinetics whatever the SO2 concentrations tested.
Relationship Between SO2 Sensitivity and Genetic Groups
When analyzed globally, clear differences between the different genetic groups were observed (Figure 3): the L0308-like group showed mostly resistant behavior (invariant growth parameters whatever sulfite concentration). The AWRI1499-like group showed mostly unchanged maximal growth rate and OD, and showed either unchanged lag phase (resistant strains) or poorly increased lag phase (tolerant strains). All other groups were mostly sensitive to sulfite treatments, with an important variability amongst strains regarding to their degree of sensitivity.
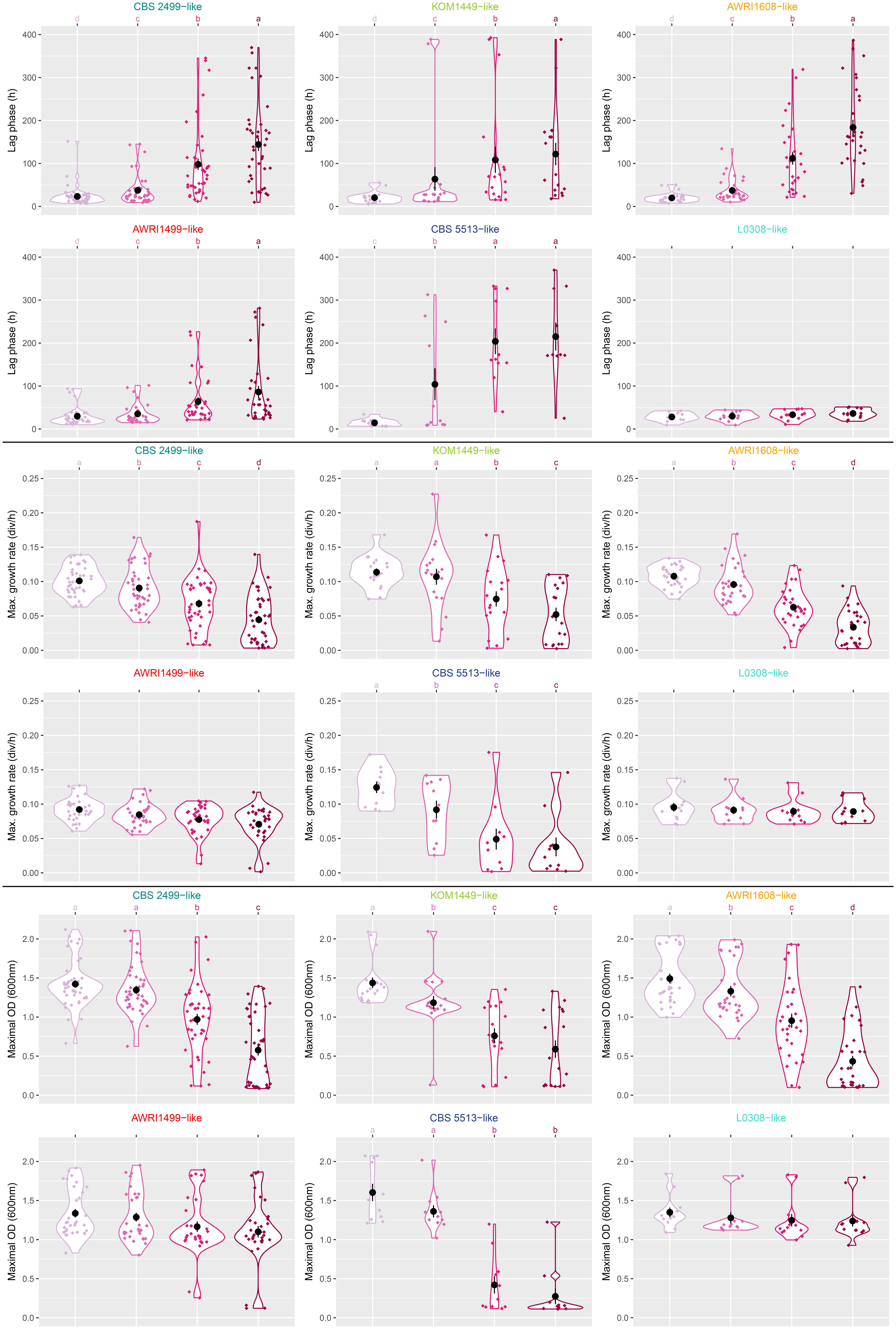
FIGURE 3. Violin plots for three growth parameters and six genetic groups of B. bruxellensis. Three growth parameters were represented: lag phase (h), maximum growth rate (division per hour), and maximum OD (600 nm). For each genetic group, numeric values (corresponding to the different strains) are represented as diamonds, the corresponding probability densities are represented as plain traits, means, and standard errors are represented by black circles and segments, respectively. Increasing SO2 concentrations are represented by the same coloring (pink shades, light pink corresponding to 0 mg/L and darker color representing increasing SO2 concentrations) as in Figure 2. The plots were obtained using ggplot2 package (R). Top letters represent significance groups as defined by Kruskal–Wallis test (agricolae package, p-value < 0.05). Absence of top letters indicates non-significantly different sulfur conditions.
A more precise analysis, strain by strain, was performed (Table 2 and Supplementary Figure S1). An important proportion of the tested isolates (52/145, 36%) were either tolerant or resistant to sulfite treatments, and this was strongly related to genetic groups. For example, all 12 isolates of the L0308-like group were either resistant (11) or tolerant (1) to sulfite treatments. Similarly, amongst the 32 isolates tested for the AWRI1499-like group, 21 were resistant, 7 tolerant, and only 4 sensitive to sulfite treatments. This confirms that, globally, most isolates from L0308-like and AWRI1499-like groups are resistant/tolerant to sulfite. By contrast, the other groups contained mostly sensitive strains (38/42 for CBS 2499-like; 14/18 for KOM1449-like; 27/30 for AWRI1608-like; 10/11 for CBS 5513-like).
In addition, 46 out of 52 tolerant or resistant strains were isolated from wine (Supplementary Table S1). Indeed, the proportion of tolerant/resistant isolates from wine represented 43% (46/107).
Discussion
Sulfur dioxide is usually used by winemakers as preventive or curative treatment for spoilage microorganisms including B. bruxellensis contamination. Concentrations of 0.2 to 0.5 mg.L-1 molecular SO2 are typically reported to inhibit growth in wine (Conterno et al., 2006; Barata et al., 2008). However, some B. bruxellensis strains were shown to be rather sulfite tolerant (Barata et al., 2008; Vigentini et al., 2008; Curtin et al., 2012; Agnolucci et al., 2014; Avramova et al., 2018) and sulfite efficiency was elucidated as population level dependent (Longin et al., 2016). Previous studies highlighted genotype-dependent tolerance to sulfur dioxide for B. bruxellensis among Australian isolates with AFLP markers (Curtin et al., 2012), and this was recently confirmed for 39 isolates analyzed with microsatellite markers (Avramova et al., 2018). Taking into account the high intra-species genetic diversity of B. bruxellensis, 106 additional isolates from various origins were included to the previous phenotypic test to confirm the link between genotype and SO2 tolerance at larger and finer scale. Here, we show that 36% of B. bruxellensis isolates are resistant/tolerant to sulfite (up to 43% amongst wine isolates), and we confirm the relationship between genetic groups and survival patterns in presence of sulfite treatments.
In our previous study, it was noticed that representatives of the L0308-like group exhibited a peculiar profile characterized by unmodified growth parameters at all tested SO2 concentrations. However, these observations were based on only two isolates with similar origin (Avramova et al., 2018). To complete these results, we analyzed 9 additional L0308-like strains from different origins and confirmed their (mostly) resistant phenotype. Here, a resistant phenotype corresponds to behavior for which there were no significant differences for all studied growth parameters at increasing SO2 concentration. On the other hand, tolerant strains were those for which lag phase was modified with SO2 increase. Those two terms are used in clinical microbiology, where they serve to describe microbial pathogenicity (Anderson, 2005; Brauner et al., 2016). Often, tolerance is related to the capacity of the organism to survive under inhibition by an agent, whereas resistance is linked to the capacity to actively proliferate in presence of antibiotic, and is measured as minimum inhibitory concentration or fitness (Anderson, 2005). The peculiarity of SO2 application, however, is that the main active antimicrobial fraction (mSO2) of this agent depends on environmental parameters (such as temperature, alcohol content, and mainly pH) and that the active fraction decreases over time due to free SO2 combination. Furthermore, B. bruxellensis is able to enter a VBNC (viable but not cultivable) state after sulfites addition (du Toit et al., 2005; Agnolucci et al., 2010; Serpaggi et al., 2012; Capozzi et al., 2016; Longin et al., 2016), followed by growth recovery when sulfites decrease over time. In winemaking, sulfite levels are regularly re-adjusted at different time intervals, thus creating seasonality in SO2 administration during the winemaking process. In these conditions, the actual survival of B. bruxellensis in wine could be related to (i) survival and growth besides initial “hit” with SO2, that could be related to resistant-type mechanism and (ii) survival at the initial SO2 “hit” and until a stage when mSO2 concentration is lower in the medium, followed by growth recovery that could be described as tolerance mechanism. Indeed, resistant and tolerant phenotypes are often interconnected and related to different types of metabolism and cell structure differences. In clinical microbiology, it is suggested that tolerant and resistant strains should be treated differently: resistant should be treated with higher doses and shorter treatment, whereas tolerant strains should be treated with lower doses but extended treatment duration (Brauner et al., 2016). The detection of both resistant and tolerant growth profiles in the present dataset suggests that B. bruxellensis strains have developed not one, but multiple strategies to cope with SO2 present in wine.
Here, the majority of tolerant or resistant strains were isolated from wine (46 out of 52). This suggests a strong link between SO2 exposure related to the winemaking industry and B. bruxellensis survival in presence of SO2 (Curtin et al., 2012). This data highlights the role of SO2, and therefore human activity, in shaping B. bruxellensis population structure, which was also suggested in previous studies (Curtin et al., 2012; Avramova et al., 2018). Sulfur dioxide resistance is broadly studied in S. cerevisiae and the main molecular mechanisms explaining this phenotype is efflux through Ssu1p active pump (Park and Bakalinsky, 2000; Perez-Ortin et al., 2002; Nardi et al., 2010). It was demonstrated that SSU1-R allele, which is involved in SO2 resistance, is the product of reciprocal translocation between chromosomes VII and XVI, thus highlighting the importance of gross chromosomal rearrangements in the adaptive evolution of S. cerevisiae (Perez-Ortin et al., 2002). Later, another translocation involved in SO2 tolerance (XV-t-XVI) was shown to shorten lag phase in presence of SO2, thus conferring relative selective advantage compared to non-translocated XVI strains (Zimmer et al., 2014). Following those studies, it was suggested that those translocations were empirically selected by humans (Perez-Ortin et al., 2002; Zimmer et al., 2014). The lack of effect of SO2 on lag phase observed for the resistant B. bruxellensis strains could be related to similar mechanisms. Indeed, allele specific expression of efflux pump BbSSU1 was detected by comparative transcriptomics (Curtin et al., 2015). However, the molecular mechanisms underlying resistant phenotype in B. bruxellensis remain to be elucidated. As for the tolerant strains, the longer lag phase would reflect the time needed for the adaptation through complex mechanisms or the survival until a lower mSO2 concentration is attained in the medium. Using staining with propidium iodide detection by flow cytometer analysis, Longin et al. (2016) showed that sulfite induces increased yeast cell permeability, which probably leads to cell death. The ability of cells to restore functional cell permeability could constitute another sulfite adaptation mechanism for B. bruxellensis. The SO2 molecule has various effects on the cell structure, metabolism, and genome (Divol et al., 2012), and the corresponding mechanisms could include synthesis of binding molecules (like acetaldehyde), specific membrane structure, etc (Divol et al., 2012).
The sensitivity/survival phenotype in presence of SO2 correlates with genotypic profiles defined by microsatellite analysis in a set of 145 representative strains (Avramova et al., 2018). The groups CBS 2499-like, KOM1449-like, AWRI1608-like, and CBS 5513-like are all susceptible to SO2 presence in synthetic medium. On the contrary, AWRI1499-like and L0308-like survived in presence of high concentrations of mSO2. This behavior was confirmed by independent study (Longin et al., 2016) performed in wine medium, where the strain L0417 (AWRI1499-like) was demonstrated to be more tolerant than L02E2 (CBS 2499-like). The use of microsatellites as selection markers was previously proposed for S. cerevisiae wine strains (Franco-Duarte et al., 2009, 2014). In the latter work, 30 different phenotypes were analyzed, and SO2 tolerance was one of the factors that correlate the most with microsatellite patterns. In the winemaking context, SO2 tolerance is a positive trait for the selection of S. cerevisiae, whereas it is the opposite for B. bruxellensis strains, for which it is directly related to spoilage potential. Defining SO2 tolerance through genetic markers can therefore be used as an efficient tool to adapt antimicrobial treatment in winery. Similar methods are used for resistance prediction for pathogenic fungi (Park and Perlin, 2005; Irinyi et al., 2015). Namely, in the case of C. albicans, PCR-based methods were proposed for the detection of mutations related to fluconazole resistance (Park and Perlin, 2005). This method allows the adoption of alternative techniques to cope with this microorganism. Contrary to fluconazole, SO2 has a very broad range of actions on the cell at structural, genetic, and metabolic level (White et al., 2002; Divol et al., 2012), and detection method of specific mutation responsible for resistance would be a challenge. Therefore, the strong correlation between genotype and SO2 tolerance presents a reliable alternative for the prediction of this phenotype through microsatellite analysis. Indeed, resistant/tolerant genotypes can be reliably predicted: 91% (40/44 strains) of the AWRI1499-like and L0308-like isolates are actually tolerant or resistant to sulfite. For comparison, this percentage was 91% for C. albicans (based on 32 isolates) when using targeted PCR (Park and Perlin, 2005). Combined with the fact that clonal populations of B. bruxellensis strains were isolated over a long period of time in the same winery (Albertin et al., 2014), the use of microsatellite markers is also applicable as a prediction method based on spoilage populations from previous vintages. Hence, the use of microsatellite markers is a reliable method for predicting spoilage potential in means of SO2 tolerance for B. bruxellensis populations, although a bit expensive and time-consuming for routine analysis. Therefore, we developed an alternative analysis, based on a single duplex PCR and classical gel electrophoresis migration that indicates (i) whether the isolates belong to B. bruxellensis species and (ii) their sulfur dioxide sensitivity (Albertin et al., 2017a, 2018). This approach was patented (Albertin et al., 2017b) and is compatible with day-to-day analysis by oenological laboratories. Such diagnosis could allow application of adequate antimicrobial techniques according to the survival mechanism in presence of SO2 of the contaminating B. bruxellensis population, and thus to assure efficient spoilage prevention with minimal intervention.
Author Contributions
IM-P and WA conceived the study. MA, AV-C, and JM performed the experiments. All authors analyzed the data and wrote the manuscript.
Funding
This work was partly funded by AST (Aquitaine Science Transfert), as ‘Typebrett’ Project.
Conflict of Interest Statement
The authors declare that the research was conducted in the absence of any commercial or financial relationships that could be construed as a potential conflict of interest.
Supplementary Material
The Supplementary Material for this article can be found online at: https://www.frontiersin.org/articles/10.3389/fmicb.2018.01260/full#supplementary-material
FIGURE S1 | Growth parameters of 145 B. bruxellensis isolates grown at different SO2 concentrations. Growth parameters lag phase (h), maximum growth rate (division per hour), and maximum OD (600 nm) are presented for 145 isolates. Isolates are clustered by genetic group as defined previously (Avramova et al., 2018), in order: CBS 2499-like group (dark cyan), KOM1449-like (light green), AWRI1608-like (orange), AWRI1499-like (red), dark blue (CBS 5513-like), turquoise (L0308-like). Vertical traits present standard deviations.
TABLE S1 | Growth parameters of B. bruxellensis strains in different concentrations of sulphur dioxide. aAWRI, The Australian Wine Research Institute, Glen Osmond, SA, Australia; CBS, Centraalbureau voor Schimmelcultures, Fungal Biodiversity Centre, Utrecht, Netherlands; CRBO, Centre de Ressources Biologiques Œnologie, Villenave d’Ornon, France; HGU, Hochschule Geisenheim University, Geisenheim, Germany; ICV, Institut coopératif du vin, Lattes, France; Inter-Rhone, Inter Rhône, Avignon, France; ISA, Instituto Superior de Agronomia, Lisbon, Portugal; ISVV, Institut des Sciences de la Vigne et du Vin, Villenave d’Ornon, France; IUVV, Institut Universitaire de la Vigne et du Vin Jules Guyot, Dijon, France; Microflora, Microflora, Villenave d’Ornon, France; UFPE, Federal University of Pernambuco, Recife, Brazil; UNIB, Université de Brest, Brest, France; UNIFG, University of Foggia, Foggia, Italy; UNINA, University of Naples Federico II, Napoli, Italy; UNISTRA, University of Strasbourg, Strasbourg, France; UWOPS, Culture collection of the University of Western Ontario, London, On, Canada; NA, Not Available.
References
Agnolucci, M., Cristani, C., Maggini, S., Rea, F., Cossu, A., Tirelli, A., et al. (2014). Impact of sulphur dioxide on the viability, culturability, and volatile phenol production of Dekkera bruxellensis in wine. Ann. Microbiol. 64, 653–659. doi: 10.1007/s13213-013-0698-6
Agnolucci, M., Rea, F., Sbrana, C., Cristani, C., Fracassetti, D., Tirelli, A., et al. (2010). Sulphur dioxide affects culturability and volatile phenol production by Brettanomyces/Dekkera bruxellensis. Int. J. Food Microbiol. 143, 76–80. doi: 10.1016/j.ijfoodmicro.2010.07.022
Albertin, W., Avramova, M., Cibrario, A., Ballestra, P., Dols-Lafargue, M., Curtin, C., et al. (2017a). Brettanomyces bruxellensis: diversité génétique et sensibilité aux sulfites. Rev. Oenol. Tech. Vitivinicoles Oenol. 44, 31–33.
Albertin, W., Masneuf-Pomarede, I., and Peltier, E. (2017b). Method for Analysing a Sample to Detect the Presence of Sulphite-Resistant Yeasts of the Brettanomyces bruxellensis Species and Kit for Implementing Same. France patent no. PCT/FR2016/052701.
Albertin, W., Avramova, M., Maupeu, J., Vallet-Courbin, A., Cibrario, A., Dols-Lafargue, M., et al. (2018). Pratique du sulfitage. Brettanomyces bruxellensis s’adapte ! Union Girondine Vins Bord. 1153, 46–48.
Albertin, W., Panfili, A., Miot-Sertier, C., Goulielmakis, A., Delcamp, A., Salin, F., et al. (2014). Development of microsatellite markers for the rapid and reliable genotyping of Brettanomyces bruxellensis at strain level. Food Microbiol. 42, 188–195. doi: 10.1016/j.fm.2014.03.012
Anderson, J. B. (2005). Evolution of antifungal-drug resistance: mechanisms and pathogen fitness. Nat. Rev. Microbiol. 3, 547–556. doi: 10.1038/nrmicro1179
Avramova, M., Cibrario, A., Peltier, E., Coton, M., Coton, E., Schacherer, J., et al. (2018). Brettanomyces bruxellensis population survey reveals a diploid-triploid complex structured according to substrate of isolation and geographical distribution. Sci. Rep. 8:4136. doi: 10.1038/s41598-018-22580-7
Barata, A., Caldeira, J., Botelheiro, R., Pagliara, D., Malfeito-Ferreira, M., and Loureiro, V. (2008). Survival patterns of Dekkera bruxellensis in wines and inhibitory effect of sulphur dioxide. Int. J. Food Microbiol. 121, 201–207. doi: 10.1016/j.ijfoodmicro.2007.11.020
Berbegal, C., Garofalo, C., Russo, P., Pati, S., Capozzi, V., and Spano, G. (2017). Use of autochthonous yeasts and bacteria in order to control Brettanomyces bruxellensis in wine. Fermentation 3:65. doi: 10.3390/fermentation3040065
Berbegal, C., Spano, G., Fragasso, M., Grieco, F., Russo, P., and Capozzi, V. (2018). Starter cultures as biocontrol strategy to prevent Brettanomyces bruxellensis proliferation in wine. Appl. Microbiol. Biotechnol. 102, 569–576. doi: 10.1007/s00253-017-8666-x
Brauner, A., Fridman, O., Gefen, O., and Balaban, N. Q. (2016). Distinguishing between resistance, tolerance and persistence to antibiotic treatment. Nat. Rev. Microbiol. 14, 320–330. doi: 10.1038/nrmicro.2016.34
Capozzi, V., Di Toro, M. R., Grieco, F., Michelotti, V., Salma, M., Lamontanara, A., et al. (2016). Viable But Not Culturable (VBNC) state of Brettanomyces bruxellensis in wine: new insights on molecular basis of VBNC behaviour using a transcriptomic approach. Food Microbiol. 59, 196–204. doi: 10.1016/j.fm.2016.06.007
Chatonnet, P., Dubourdieu, D., Boidron, J.-N., and Pons, M. (1992). The origin of ethylphenols in wines. J. Sci. Food Agric. 60, 165–178. doi: 10.1002/jsfa.2740600205
Conterno, L., Joseph, C. M. L., Arvik, T. J., Henick-Kling, T., and Bisson, L. F. (2006). Genetic and physiological characterization of Brettanomyces bruxellensis strains isolated from wines. Am. J. Enol. Vitic. 57, 139–147.
Curtin, C., Joseph, L., Zeppel, R., Albertin, W., Masneuf-Pomarede, I., Bisson, L. F., et al. (2015). “Genomic and transcriptomic landscape of the industrial yeast species Brettanomyces bruxellensis,” in The 32nd International Specialized Symposium on Yeasts (ISSY32), Perugia [accessed September 13–17, 2015].
Curtin, C., Kennedy, E., and Henschke, P. A. (2012). Genotype-dependent sulphite tolerance of Australian Dekkera (Brettanomyces) bruxellensis wine isolates. Lett. Appl. Microbiol. 55, 56–61. doi: 10.1111/j.1472-765X.2012.03257.x
Delfini, C., Gaia, P., Schellino, R., Strano, M., Pagliara, A., and Ambrò, S. (2002). Fermentability of grape must after inhibition with dimethyl dicarbonate (DMDC). J. Agric. Food Chem. 50, 5605–5611. doi: 10.1021/jf0256337
Divol, B., Toit, M., and Duckitt, E. (2012). Surviving in the presence of sulphur dioxide: strategies developed by wine yeasts. Appl. Microbiol. Biotechnol. 95, 601–613. doi: 10.1007/s00253-012-4186-x
du Toit, W. J., Pretorius, I. S., and Lonvaud-Funel, A. (2005). The effect of sulphur dioxide and oxygen on the viability and culturability of a strain of Acetobacter pasteurianus and a strain of Brettanomyces bruxellensis isolated from wine. J. Appl. Microbiol. 98, 862–871. doi: 10.1111/j.1365-2672.2004.02549.x
Fabrizio, V., Vigentini, I., Parisi, N., Picozzi, C., Compagno, C., and Foschino, R. (2015). Heat inactivation of wine spoilage yeast Dekkera bruxellensis by hot water treatment. Lett. Appl. Microbiol. 61, 186–191. doi: 10.1111/lam.12444
Fleet, G. H. (2003). Yeast interactions and wine flavour. Int. J. Food Microbiol. 86, 11–22. doi: 10.1016/S0168-1605(03)00245-9
Francesca, C., and Maurizio, C. (2011). Kluyveromyces wickerhamii killer toxin: purification and activity towards Brettanomyces/Dekkera yeasts in grape must. FEMS Microbiol. Lett. 316, 77–82. doi: 10.1111/j.1574-6968.2010.02194.x
Franco-Duarte, R., Mendes, I., Umek, L., Drumonde-Neves, J., Zupan, B., and Schuller, D. (2014). Computational models reveal genotype-phenotype associations in Saccharomyces cerevisiae. Yeast 31, 265–277. doi: 10.1002/yea.3016
Franco-Duarte, R., Umek, L., Zupan, B., and Schuller, D. (2009). Computational approaches for the genetic and phenotypic characterization of a Saccharomyces cerevisiae wine yeast collection. Yeast 26, 675–692. doi: 10.1002/yea.1728
González-Arenzana, L., López-Alfaro, I., Garde-Cerdán, T., Portu, J., López, R., and Santamaría, P. (2018). Microbial inactivation and MLF performances of Tempranillo Rioja wines treated with PEF after alcoholic fermentation. Int. J. Food Microbiol. 269, 19–26. doi: 10.1016/j.ijfoodmicro.2018.01.008
González-Arenzana, L., Sevenich, R., Rauh, C., López, R., Knorr, D., and López-Alfaro, I. (2016). Inactivation of Brettanomyces bruxellensis by high hydrostatic pressure technology. Food Control 59, 188–195. doi: 10.1016/j.foodcont.2015.04.038
Heresztyn, T. (1986). Metabolism of volatile phenolic compounds from hydroxycinnamic acids by Brettanomyces yeast. Arch. Microbiol. 146, 96–98. doi: 10.1007/bf00690165
Irinyi, L., Serena, C., Garcia-Hermoso, D., Arabatzis, M., Desnos-Ollivier, M., Vu, D., et al. (2015). International society of human and animal mycology (ISHAM)-ITS reference DNA barcoding database–the quality controlled standard tool for routine identification of human and animal pathogenic fungi. Med. Mycol. 53, 313–337. doi: 10.1093/mmy/myv008
Longin, C., Degueurce, C., Julliat, F., Guilloux-Benatier, M., Rousseaux, S., and Alexandre, H. (2016). Efficiency of population-dependent sulfite against Brettanomyces bruxellensis in red wine. Food Res. Int. 89(Pt 1), 620–630. doi: 10.1016/j.foodres.2016.09.019
Luo, H., Schmid, F., Grbin, P. R., and Jiranek, V. (2012). Viability of common wine spoilage organisms after exposure to high power ultrasonics. Ultrason. Sonochem. 19, 415–420. doi: 10.1016/j.ultsonch.2011.06.009
Lustrato, G., Vigentini, I., De Leonardis, A., Alfano, G., Tirelli, A., Foschino, R., et al. (2010). Inactivation of wine spoilage yeasts Dekkera bruxellensis using low electric current treatment (LEC). J. Appl. Microbiol. 109, 594–604. doi: 10.1111/j.1365-2672.2010.04686.x
Mehlomakulu, N. N., Setati, M. E., and Divol, B. (2014). Characterization of novel killer toxins secreted by wine-related non-Saccharomyces yeasts and their action on Brettanomyces spp. Int. J. Food Microbiol. 188, 83–91. doi: 10.1016/j.ijfoodmicro.2014.07.015
Nardi, T., Corich, V., Giacomini, A., and Blondin, B. (2010). A sulphite-inducible form of the sulphite efflux gene SSU1 in a Saccharomyces cerevisiae wine yeast. Microbiology 156(Pt 6), 1686–1696. doi: 10.1099/mic.0.036723-0
Park, H., and Bakalinsky, A. T. (2000). SSU1 mediates sulphite efflux in Saccharomyces cerevisiae. Yeast 16, 881–888. doi: 10.1002/1097-0061(200007)16:10<881::AID-YEA576>3.0.CO;2-3
Park, S., and Perlin, D. S. (2005). Establishing surrogate markers for fluconazole resistance in Candida albicans. Microb. Drug Resist. 11, 232–238. doi: 10.1089/mdr.2005.11.232
Perez-Ortin, J. E., Querol, A., Puig, S., and Barrio, E. (2002). Molecular characterization of a chromosomal rearrangement involved in the adaptive evolution of yeast strains. Genome Res. 12, 1533–1539. doi: 10.1101/gr.436602
Petrova, B., Cartwright, Z. M., and Edwards, C. G. (2016). Effectiveness of chitosan preparations against Brettanomyces bruxellensis grown in culture media and red wines. OENO One 50, 49–56. doi: 10.20870/oeno-one.2016.50.1.54
R Development Core Team (2010). R: A Language and Environment for Statistical Computing. Vienna: R Foundation for Statistical Computing.
Ribéreau-Gayon, P., Dubourdieu, D., Donèche, B., and Lonvaud, A. (2006). Handbook of Enology: The Microbiology of Wine and Vinifications. Hoboken, NJ: John Wiley & Sons, Ltd. doi: 10.1002/0470010398
Serpaggi, V., Remize, F., Recorbet, G., Gaudot-Dumas, E., Sequeira-Le Grand, A., and Alexandre, H. (2012). Characterization of the “viable but nonculturable” (VBNC) state in the wine spoilage yeast Brettanomyces. Food Microbiol. 30, 438–447. doi: 10.1016/j.fm.2011.12.020
Taillandier, P., Joannis-Cassan, C., Jentzer, J. B., Gautier, S., Sieczkowski, N., Granes, D., et al. (2015). Effect of a fungal chitosan preparation on Brettanomyces bruxellensis, a wine contaminant. J. Appl. Microbiol. 118,123–131. doi: 10.1111/jam.12682
Umiker, N. L., Descenzo, R. A., Lee, J., and Edwards, C. G. (2013). Removal of Brettanomyces bruxellensis from red wine using membrane filtration. J. Food Process. Preserv. 37, 799–805. doi: 10.1111/j.1745-4549.2012.00702.x
Vigentini, I., Romano, A., Compagno, C., Merico, A., Molinari, F., Tirelli, A., et al. (2008). Physiological and oenological traits of different Dekkera/Brettanomyces bruxellensis strains under wine-model conditions. FEMS Yeast Res. 8,1087–1096. doi: 10.1111/j.1567-1364.2008.00395.x
White, T. C., Holleman, S., Dy, F., Mirels, L. F., and Stevens, D. A. (2002). Resistance mechanisms in clinical isolates of Candida albicans. Antimicrob. Agents Chemother. 46, 1704–1713. doi: 10.1128/AAC.46.6.1704-1713.2002
Keywords: Brettanomyces bruxellensis, resistance, tolerance, sulfur dioxide, wine, spoilage yeast
Citation: Avramova M, Vallet-Courbin A, Maupeu J, Masneuf-Pomarède I and Albertin W (2018) Molecular Diagnosis of Brettanomyces bruxellensis’ Sulfur Dioxide Sensitivity Through Genotype Specific Method. Front. Microbiol. 9:1260. doi: 10.3389/fmicb.2018.01260
Received: 15 March 2018; Accepted: 24 May 2018;
Published: 11 June 2018.
Edited by:
Linda F. Bisson, University of California, Davis, United StatesReviewed by:
Mariagiovanna Fragasso, Consiglio per la Ricerca e la sperimentazione in Agricoltura, ItalyLucía González-Arenzana, Instituto de Ciencias de la Vid y del Vino (ICVV), Spain
Copyright © 2018 Avramova, Vallet-Courbin, Maupeu, Masneuf-Pomarède and Albertin. This is an open-access article distributed under the terms of the Creative Commons Attribution License (CC BY). The use, distribution or reproduction in other forums is permitted, provided the original author(s) and the copyright owner are credited and that the original publication in this journal is cited, in accordance with accepted academic practice. No use, distribution or reproduction is permitted which does not comply with these terms.
*Correspondence: Warren Albertin, d2FycmVuLmFsYmVydGluQHUtYm9yZGVhdXguZnI=