- Bacterial Cell Biology and Physiology, Swammerdam Institute for Life Sciences, University of Amsterdam, Amsterdam, Netherlands
The morphology of bacterial species shows a wealth of variation from star-shaped to spherical and rod- to spiral-shaped, to mention a few. Their mode of growth and division is also very diverse and flexible ranging from polar growth and lateral surface increase to midcell expansion and from perpendicular to longitudinal asymmetric division. Gammaproteobacterial rod-shaped species such as Escherchia coli divide perpendicularly and grow in length, whereas the genetically very similar rod-shaped symbiotic Thiosymbion divide longitudinally, and some species even divide asynchronously while growing in width. The ovococcal Streptococcus pneumoniae also lengthens and divides perpendicularly, yet it is genetically very different from E. coli. Are these differences as dramatic as is suggested by visual inspection, or can they all be achieved by subtle variation in the regulation of the same protein complexes that synthesize the cell envelope? Most bacteria rely on the cytoskeletal polymer FtsZ to organize cell division, but only a subset of species use the actin homolog MreB for length growth, although some of them are morphologically not that different. Poles are usually negative determinant for cell division. Curved cell poles can be inert or active with respect to peptidoglycan synthesis, can localize chemotaxis and other sensing proteins or other bacterial equipment, such as pili, depending on the species. But what is actually the definition of a pole? This review discusses the possible common denominators for growth and division of distinct and similar bacterial species.
Introduction
Bacteria can grow with a wealth of variations (Kysela et al., 2016) of which only the morphogenesis of the sphere, the ovococ, the rod-shape, and the crescent-shaped species have been thoroughly investigated in the form of Staphyloccocus aureus (Monteiro et al., 2015; Wheeler et al., 2015), Streptococcus pneumoniae (Morlot et al., 2003; Land and Winkler, 2011; Fleurie et al., 2014; Tsui et al., 2014; Bajaj et al., 2016), Escherichia coli (Egan et al., 2015; Blaauwen et al., 2017), Bacillus subtilis (Adams and Errington, 2009), and Caulobacter crescentus (Yakhnina and Gitai, 2013; Collier, 2016; Woldemeskel and Goley, 2017), respectively. More recently, other rod-shaped bacteria such as Myxococcus xanthus that grow by elongation at lateral sides (Lotte Søgaard-Andersen personal communication) and rods that grow by extension of their poles [Mycobacterium tuberculosis (Singh et al., 2013), Corynebacterium glutamicum (Letek et al., 2008), and Agrobacterium tumefaciens (Cameron et al., 2015)], from midcell (Rhizobiales; Brown et al., 2012), or as branched filaments (Streptomyces; Jakimowicz and van Wezel, 2012; Claessen et al., 2014), are receiving more attention (Eswara and Ramamurthi, 2017). This development is applaudable as it is discovered that our laboratory pets are not the standard for morphological organization and regulation we thought them to be.
A better understanding of the available variation in the organization of bacterial growth (see for a review Yang et al., 2016) will enable the discrimination of key factors that determine survival of bacteria. Likely, if new antibiotics are to be discovered they should preferably interfere with multiple of these key factors to postpone resistance as long as possible. In fact, that is what many of the naturally occurring antibiotics such as beta-lactams and fluoroquinolones do (Hooper, 2001; Cho et al., 2014) and why they have been very successful, until we have started to apply them in quantities that were not meant to be used. Consequently, a dramatic increase in antibiotic resistance developed to a point that some bacterial infections have become untreatable (http://www.who.int/mediacentre/news/releases/2017/bacteria-antibiotics-needed). In addition, detailed molecular knowledge of specific species could be of use for the development of new small spectrum antibiotics, to diminish destruction of the composition of the gut microbiota (Gao et al., 2017).
Even among phylogenetically related species, considerable difference in morphology can be observed despite a very high genomic similarity. For instance, the gammaproteobacteria Escherichia coli, Candidatus Thiosymbion oneisti, and Candidatus Thiosymbion hypermnestrae, share most of the genes known to be involved in morphogenesis, whereas their division modes are very different. The Thiosymbion live in marine sediment attached via one of their cell poles to the cuticle of their nematode host, Laxus oneistus and Robbea hypermnestra, respectively. E. coli is a rod-shaped bacterium that divides perpendicularly to produce two identical daughter cells, whereas the rod-shaped symbionts have approximately the same size as E. coli but divide longitudinally (Leisch et al., 2012, 2016; Figure 1A). Presumably, they have evolved to divide longitudinally to ensure the daughter cells have an anchor point on the nematode surface. These bacteria can be seen as very fat and short E. coli that still divide perpendicularly (Figure 1B model 1) or as thin and long bacteria that divide longitudinally (Figure 1B model 2). This review will try to discriminate between the two models.
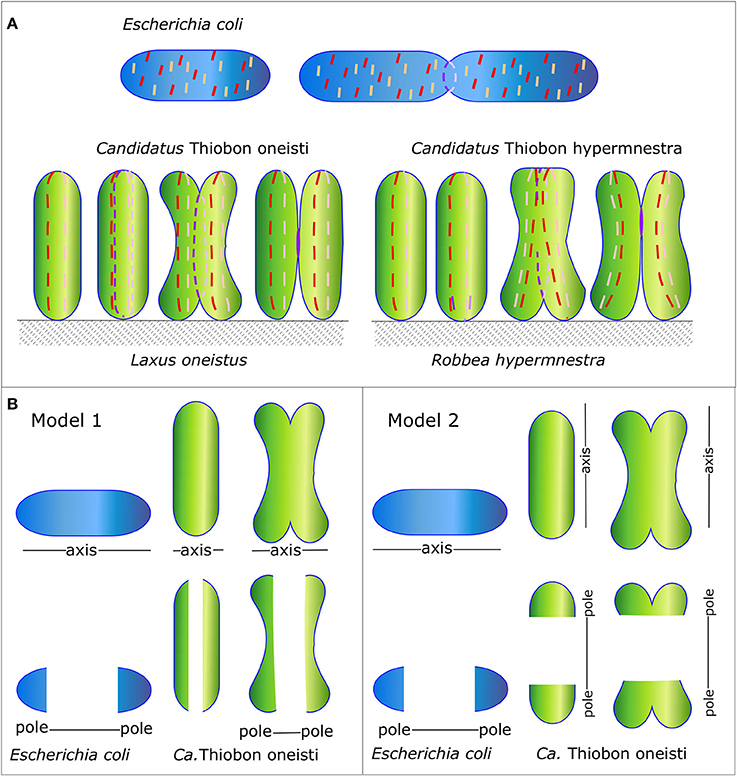
Figure 1. Schematic representation of the Morphology of E. coli and Ca. T. oneisti and Ca. T. hypermnestrae and their MreB and FtsZ localization patterns. (A) Orientation of the division plane in the gammaproteobacteria E. coli (in blue) and Ca.T. oneisti and Ca. T. hypermnestae (in green). The very simplified localization of MreB and FtsZ are shown as a dashed red and purple line, respectively. (B) Two possible models. Ca. T. oneisti can be seen as a short very fat E. coli (model 1) or as a cell that has swapped its major axes in comparison to E. coli (model 2).
Peptidoglycan Determines Shape
The peptidoglycan (PG) layer protects bacteria against osmotic and chemical stress and is an absolute requirement to maintain shape for bacteria that live in a hypo-osmotic environments such as the sea and the soil, in contrast to species such as Chlamydia that live in Eukaryotic cells, which cytosol is osmotically in balance with their own cytosol (Liechti et al., 2016). The PG layer consists of glycan strands of repeating N-acetylglucosamine and N-acetylmuramic acid disaccharides of which the latter are interconnected by peptide side bridges (Egan et al., 2015). The fourth and third amino acid of two stem peptides are crosslinked. Alternatively, some bacterial species such as Mycobacteria (Gupta et al., 2010) and Clostridia (Peltier et al., 2011) make predominantly crosslinks between the third amino acid on both stem peptides (Figure 2). The PG network is present as a single layer in Gram-negative bacteria that have an outer membrane and as multiple layers in Gram-positive bacteria that do not have an outer membrane. The well-known Penicillin Binding Proteins (PBP)s that can be inhibited by beta-lactams are responsible for the synthesis of peptidoglycan (Vollmer and Bertsche, 2008), together with the penicillin insensitive glycosyl transferases (GTases) RodA and possibly FtsW (Meeske et al., 2015, 2016; Cho et al., 2016). The PG disaccharide pentapeptide building units are synthesized in the cytoplasm and coupled to a lipid linker (undecaprenyl pyrophosphate) by the integral membrane protein MraY to become attached to the cytoplasmic membrane as lipid II, and subsequently flipped to the outer side of the cytoplasmic membrane to be used by the PG synthases (Egan et al., 2015). PG forms a covalently closed network, which intactness is essential for the survival of the cells. Therefore, a well-regulated balance between the insertion of new PG and the cleavage of bonds in the existing layer is needed to allow for the growth and survival of the bacteria. This balance is achieved by the formation of large protein complexes, termed elongasome and divisome for cell elongation and division, respectively (Blaauwen et al., 2008).
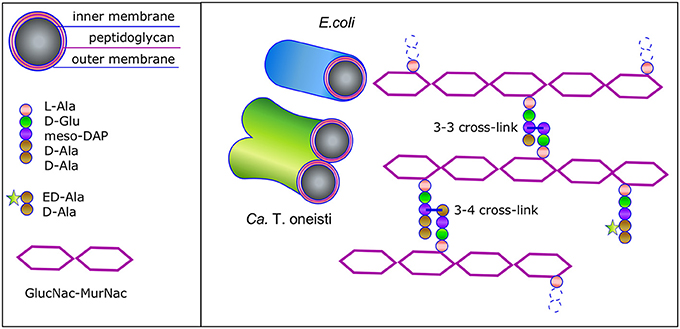
Figure 2. Schematic representation of the Gram-negative envelope and its peptidoglycan structure. Gram-negative bacteria have a three-layered envelope. The outer and inner membrane sandwich the single peptidoglycan (PG) layer. The glycan strands are connected by stem peptides that are either 3-4 or 3-3 crosslinked. ED-Ala-D-Ala (Liechti et al., 2014) is a clickable probe that is imported in the cytosol of the Thiosymbon and incorporated in the N-acetyl muramyl(MurNac)pentapeptide. Next the precursor is coupled to the lipid tail undecaprenyl phosphate by MraY and to N-acetyl glucosamine (GlcNac) by MurG to complete the synthesis of Lipid II. The latter is then flipped across the cytoplasmic membrane and the PG precursor is incorporated in the peptidoglycan layer. Consequently, the nascent peptidoglycan synthesis can be monitored by chemical addition of a fluorophore.
FtsZ
Septum synthesis and cell division is initiated by a cytoskeletal protein, the tubulin homolog FtsZ. It polymerizes in a GTP dependent fashion to form protofilaments of about 100 nm in length underneath the cytoplasmic membrane at midcell. In E. coli, the polymers are attached to the membrane by the bitopic membrane protein ZipA (Hale and de Boer, 1997) and the membrane associated protein FtsA (Tormo and Vicente, 1984). The FtsZ filaments are connected to each other by ZapA and possibly by other Z-ring organizing proteins such as Zap C, D, and E (Blaauwen et al., 2017). This structure has been termed the proto-ring because initially FtsZ was thought to form a continuous ring based on low resolution fluorescence microscopy. High resolution microscopy such as PALM, STET and SIM has revealed that the proto-ring is discontinuous and that FtsZ polymers likely form a loosely organized network of filaments in three dimensions (Strauss et al., 2012; Buss et al., 2013, 2015; Holden et al., 2014; Jacq et al., 2015), which are dynamically kept together by the aforementioned proteins. The FtsZ polymers were shown to grow on one end and release monomers on the opposite end of the filament, causing them to treadmill (Loose and Mitchison, 2014; Bisson-Filho et al., 2017; Yang et al., 2017). After a measurable delay (Aarsman et al., 2005; Gamba et al., 2009; Wu et al., 2018), the proto-ring recruits all proteins that are needed to coordinate the precisely regulated synthesis and cleavage of the septum that will become the new pole of the two daughter cells. The treadmilling of the FtsZ filaments seems to move the PG synthetic complexes in a circumferential direction guiding the synthesis of the septum. Cephalexin-mediated Inhibition of transpeptidase activity, does not inhibit this movement (Yang et al., 2017). When the class B PBP3 responsible for the crosslinking of the glycan strands during cell division is specifically inhibited by aztreonam (Kocaoglu and Carlson, 2015) the cells are not able to divide, and form filaments while the division machinery remains localized at midcell for at least two mass doublings (Pogliano et al., 1997; Blaauwen et al., 2003; Potluri et al., 2010). Possibly the division machinery does not dissociate while PBP3 is inhibited, because glycosyl transferase (GTase) activity continues in a futile cycle of synthesis and hydrolysis of the glycan strands until the lipid II precursors are depleted (Cho et al., 2014). FtsZ mutants that have a reduced GTPase activity and therefore reduced depolymerization rate, slow down the movement of the PG synthases (Yang et al., 2017), suggesting that FtsZ treadmilling directs PG synthesis.
The Gram-negative gammaproteobacteria E. coli, Ca. T. oneisti, and Ca. T. hypermnestrae all have closely related FtsZ proteins (Figure 3) with 74% identical amino acid residues (amino acid residues 1-316, excluding the C-terminal variable region). The longitudinal mode of division of the symbionts is also supported by FtsZ, which forms an interrupted ellipse instead of a ring in Ca.T. oneisti (Leisch et al., 2012) and starts as an arc in the basal pole of Ca. T. hypermnestrae (Leisch et al., 2016; Figure 1A). The localization patterns of the Z-ring in these bacteria exemplify that a closed ring structure is not a requirement for division.
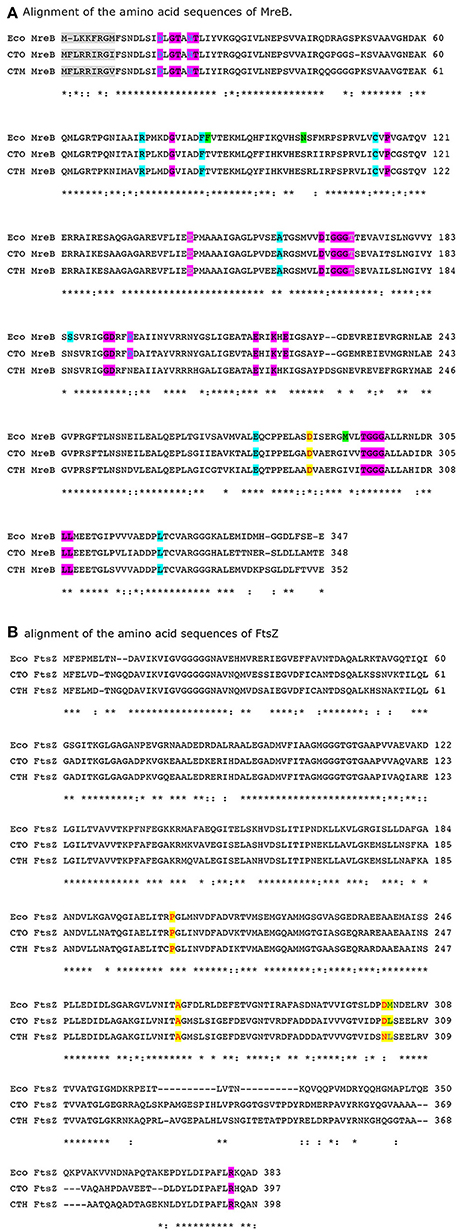
Figure 3. (A,B) Alignment of E. coli (Eco), Ca. T. oneisti (CTO) and Ca. T. hypermnestra (CTH) MreB and FtsZ. Active site residues in MreB are highlighted in magenta (van den Ent et al., 2001). Amino acids that are in direct contact with A22 are shown in gray (Bean et al., 2009). Amino acids that confer A22 resistance are highlighted in cyan or when overlapping with ATP binding shown in magenta (Gitai et al., 2005; Dye et al., 2011; Ouzounov et al., 2016). Amino acids in MreB that increase the width of E. coli are highlighted in green (Shi et al., 2017). Amino acids involved in the interaction of MreB with FtsZ are highlighted in yellow. The amino acid R379 that is essential for degradation of FtsZ by ClpXP in E. coli (highlighted magenta) is also conserved in the symbionts. Alignment was made by Clustal Omega (Sievers et al., 2011).
MreB
In rod-shaped bacteria such as E. coli and B. subtilis two modes of PG synthesis can be discriminated. Elongation occurs by dispersed insertion of new material into the existing PG layer, and division is achieved by the synthesis of a division septum, which is later converted to yield two entirely new cell poles (De Pedro et al., 1997). The second cytoskeletal protein in most rod shaped bacterial species is MreB, an actin homolog that polymerizes as short filaments in an ATP dependent fashion, which attaches to the cytoplasmic membrane with its amphipathic helix (Salje et al., 2011). The distribution of MreB filaments over the entire length of the cell is even (Jones et al., 2001; Carballido-López and Errington, 2003). The filaments themselves are almost perpendicularly oriented to the width axis of the cell (Wang et al., 2012; Olshausen et al., 2013). It is not known what exactly determines this organization. In addition to its own interaction with the membrane, MreB is also attached by its interaction with the bitopic membrane protein RodZ (Shiomi et al., 2008), the integral protein MreD and the bitopic protein MreC that forms a similar organization as MreB in the periplasm (Leaver and Errington, 2005; van den Ent et al., 2006). The MreB filaments of about 300–500 nm move along the membrane with an average speed of half a cell circumference per min in B. subtilis and in E. coli (Domínguez-Escobar et al., 2011; Garner et al., 2011; van Teeffelen et al., 2011; Olshausen et al., 2013; Ouzounov et al., 2016). In contrast to the treadmilling of FtsZ, inhibition by mecillinam of the transpeptidase activity of the class B PBP2, responsible for the crosslinking of the glycan strands during elongation, stops this movement despite the ongoing futile cycle of GTase and turnover activity (van Teeffelen et al., 2011; Cho et al., 2016). Depletion of Lipid II or induction of the expression of a GTase defective RodA mutant also inhibits the MreB movement (Cho et al., 2016), indicating that MreB movement and PG insertion in the lateral cell wall are coupled. In contrast, depolymerization of MreB by its inhibitor A22 does only affect the number of filaments but not the speed of movement of the filaments that were still intact (van Teeffelen et al., 2011; Olshausen et al., 2013; Lee et al., 2014). The overall consensus is that MreB recruits and organizes the PG synthesis machinery needed for elongation and that insertion of new PG is responsible for the movement of MreB.
Escherchia coli, Ca. T. oneisti, and Ca. T. hypermnestrae all have closely related MreB proteins (Figure 3) with up to 77% identical and 88% similar residues. Surprisingly, the localization of MreB in the symbiotic bacteria is quite different from the well-known patchy distribution observed in E. coli, B. subtilis and C. crescentus. In non-dividing symbiont cells MreB was found in a very thin and restricted area in medially (parallel to the cell long axis) arranged patches (Pende et al., 2018). In dividing cells MreB seemed to associate initially with the Z-ellipse and both proteins co-localize. Upon invagination of the envelope, MreB and FtsZ separate in the proximal and distal poles where MreB reassumes its medial localization and FtsZ follows the constricting septum forming a ring and finally a dot in the almost separated daughter cells (Pende et al., 2018). Because of the large surface of the septum (Figure 1) in these longitudinally dividing bacteria, the doubling in volume assuming exponential growth comes to a large extent from the added surface area during cells division (see Figure 4 and Supplementary Video 1).
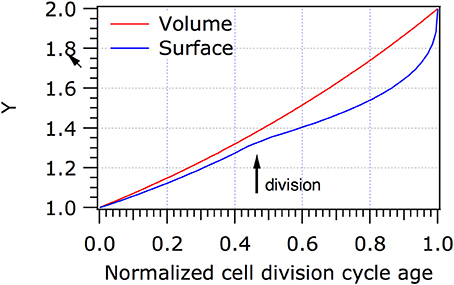
Figure 4. Increase in volume and surface of Ca.T. oneisti. When it is assumed that Ca. T. onesti is growing exponentially, it increases predominantly (70%) in surface during division. The normalized cell division cycle age is plotted against the volume (red) or the surface (blue) of the cells. Y represents the volume or the surface in arbitrary units. It should be noted that we have no information on the growth mode of the symbiont because they cannot be cultivated, apart from that they clearly grow in width only. The model is purely to show the putative contribution of division to the increase in width.
The Role of MreB in Cell Division
MreB filaments also run perpendicular to the long axis of C. crescentus cells, like in E. coli. In addition, MreB accumulates at midcell in dividing cells in an FtsZ dependent fashion (Figge et al., 2004; Gitai et al., 2004). In bacterial two hybrid experiments FtsZ was found to interact with MreB (White et al., 2010). Upon depletion of MreB, cell division was impaired and diameter control at midcell seemed to be lost implying that MreB is needed for cell division in C. crescentus. However, MreB leaves the cell division site at about the same time as the late localizing proteins such as FtsL and FtsW arrive (Goley et al., 2011), suggesting that MreB is only needed at an early stage in which the cells are preparing for cell division. A similar temporal midcell localization of MreB was observed in E. coli (Vats and Rothfield, 2007; van der Ploeg et al., 2013), and a specific interaction between FtsZ and MreB was shown in vivo and in vitro (Fenton and Gerdes, 2013). It was hypothesized that midcell localization of MreB might help to position the lipid II synthesizing complex at midcell because MurG, the last protein involved in lipid II synthesis, could be immunoprecipitated together with MreB in E. coli (Mohammadi et al., 2007; van der Ploeg et al., 2013). In Caulobacter, MurG and MreB are both dependent on the presence of a FtsZ ring for midcell localization and treatment of the cells with A22, which inhibits the polymerization of MreB does not affect the localization of MurG (Aaron et al., 2007). MurG midcell localization is not dependent on active PG synthesis in Caulobacter (Aaron et al., 2007). These data suggest that if MreB helps to recruit the lipid II synthesis complex to midcell its role is only temporarily.
Cells that do not possess FtsZ, such as Chlamydia trachomatis, require MreB to synthesize a temporal thin band of PG at the division plane (Liechti et al., 2016). The deltaproteobacterium Bdellovibrio bacteriovorus is a very small Gram-negative bacterial species that preys on Gram-negative species like E. coli (Kuru et al., 2017). It has two MreB proteins, one involved in elongation and one involved in division (Fenton et al., 2010). Interestingly, bacteria that grow by dispersed insertion of PG, do not always use MreB for this purpose. For instance, in Rhodobacter sphaeroides MreB localizes predominantly at a predivisional stage at the septum in elongating cells, and moves to future division sites when septation starts (Slovak et al., 2005). In the symbiont cells Ca. T. oneisti and Ca. T. hypermnestrae the MreB inhibitor A22 completely abolished the synthesis of peptidoglycan as monitored by the lack of incorporation of fluorescent PG precursors (Figure 2; Pende et al., 2018). In addition, the specific localization and assemblage of FtsZ is lost (Pende et al., 2018). Inhibition by A22 of MreB in E. coli results in spherical cells that increase in diameter (Karczmarek et al., 2007; Ouzounov et al., 2016). No change in the width of the symbiont cells was observed after prolonged incubation with A22. Since all PG synthesis stops under these conditions, also no shape changes are observed in the symbionts.
The concentration of FtsZ in non-dividing Ca. T. oneisti is only 10–20% of that in dividing cells (Leisch et al., 2012), which could indicate that it is specifically synthesized at the moment the cells are ready to divide. Amino acid residue R379, involved in degradation of FtsZ by the proteolytic system ClpXP in E. coli (Viola et al., 2017) is also conserved in the symbionts (Figure 3). Possibly a large part of FtsZ is degraded after the symbionts have finished division. As MreB is known to interact with FtsZ in E. coli and the involved amino acids in MreB as well as in FtsZ are conserved in Ca. T. oneisti, it is conceivable that MreB recruits FtsZ to the potential division site. Because these symbionts double in width predominantly by dividing (Pende et al., 2018), MreB might synthesize a band of preseptal PG and recruit FtsZ. Inhibition of MreB by A22 would not provide the band of preseptal peptidoglycan nor recruit FtsZ and perhaps fails to activate FtsZ synthesis. Consequently, all new peptidoglycan synthesis is completely abrogated by inhibiting MreB. The examples presented above suggest that MreB is directing specific PG synthesis at midcell in at least many Gram-negative bacterial species. The activity of MreB at midcell likely contributes to the maintenance of the correct diameter of the cells and to the precise positioning of their new division plane.
In C. T. oneisti FtsZ forms an ellipse that initially colocalizes with MreB. When constriction progresses, FtsZ remains concentrated at the midcell where new PG is synthesized, whereas the MreB signal splits in two, occupying the medial and future division sites of both daughter cells (Pende et al., 2018). PG synthesis during constriction requires a highly active synthesizing machinery to produce the new cell pole, which comprises 22% of the surface of new-born E. coli cells. In the symbionts, septal synthesis can surmount to an estimated 45–70% of the surface of the newborn cell (Figure 4 and Supplementary Video 1). Consequently, regions of active PG synthesis were predominantly observed at the leading edge of the constriction where FtsZ was localized (Pende et al., 2018).
What's in a Pole?
Conceivably, the symbiotic bacterial species have reoriented their PG synthesizing machinery such that the Z-ring is positioned parallel to the long axis of the cell, which would then correspond to the cylindrical part of an E. coli cell (Figure 1B model 2). The position where constriction is initiated would then be the equivalent of the cell poles in E. coli. This would require the reorientation of the major cytoskeletal elements MreB and FtsZ in the symbiont cells. An alternative interpretation could be that the symbionts are just very fat short E. coli cells (Figure 1B model 1). This would not require any reorganization of MreB and FtsZ. The medial position of MreB in the symbionts would then correspond to the cylindrical part of E. coli. Recently a study has been described in which MreB was randomly mutagenized and cells were selected based on their morphology by FACS (Shi et al., 2017). The mutations in MreB that resulted in very fat E. coli cells (almost 2 μm in width) are similar in the symbionts. For instance, the two mutations that cause the largest increase in width in E. coli M291V and F86S are an isoleucine (similar to valine) and a threonine (similar to serine) in Ca. T. oneisti as well as in Ca. T. hypermnestra. This illustrates that subtle changes might be sufficient to change at least one dimension of the morphology of the cells.
Since the symbionts in the latter interpretation would consist of almost only cell pole, the medial part in which MreB is localizing in a thin band would have the same orientation as the cylindrical part in E. coli. Since there is very little PG to be synthesized during elongation of the symbionts, MreB has obtained predominantly the function of marking the midcell position by the synthesis of a band of preseptal PG. Not excluding that it might contribute to the synthesis of the new cell poles during septation. FtsZ, is doing exactly what it is doing in E. coli, only it occupies a much larger circumference. By now it is clear that this is not really an issue as the Z-ring is discontinuous in all thus far investigated bacterial species (Blaauwen et al., 2017).
The cell poles in E. coli are thought to be inert with respect to PG synthesis (De Pedro et al., 1997) and so could be the very large putative poles of the symbionts. The observation in E. coli is based on the incorporation of gold labeled D-cysteine, which was diluted in density in the cylindrical wall but not in the cell poles during a chase for two mass doublings (De Pedro et al., 1997). Indicating that these D-cysteines were not accessible to PG turnover enzymes. Although many proteins involved in length growth are not present in the cell poles, many specialized sensor proteins are (for instance, the serine chemoreceptor, the DcuS/DcuR two-component system, the chemoreceptor Tar, and the Osmosensing transporter ProP; Scheu et al., 2014; Neeli-Venkata et al., 2016; Romantsov et al., 2017; Saaki et al., 2018). Thus, although poles of E. coli are inert for PG recycling, they function fully in perception of their environment. Ca. T. oneisti and Ca. T. hypermnestra cannot be cultivated, which makes experiments with living cells very limited. Consequently, at this moment it is not known whether the very large putative poles of the symbionts are inert with respect to PG turnover. Even if they were, proteins required for nutrient uptake and environmental sensing could be inserted during new cell pole synthesis. In addition, it is not known whether limited reorganization of polar PG occurs in E. coli. For instance, for the insertion of envelope spanning proteins. The wealth of available PG hydrolases in E. coli (Vollmer et al., 2008) and in the symbionts, could allow the bacteria to make new pores in the PG layer by hydrolysis of the 3–4 crosslinks and insert new protein complexes or remove proteins complexes and subsequently repair the PG layer by creating 3–3 crosslinks (Figure 2; Hugonnet et al., 2016; Kuru et al., 2017). This provides sufficient flexibility to insert whatever is needed in the envelope of the symbionts. This leaves the very short length axis of the symbionts of which the top and bottom look like a pole as the only available place for nascent PG synthesis, which is exactly what is observed in the symbiont cells (Pende et al., 2018).
MreB is reported to avoid the poles of E. coli because of the presence of anionic phospholipids such as cardiolipin and phosphatidylglycerol, which are there because of the increased curvature of the poles (Renner et al., 2013; Billings et al., 2014; Ursell et al., 2014; Kawazura et al., 2017). However, in a case where MreB was forced to bind to the poles it would stimulate PG synthesis and create Y-shaped cells, which is how division starts in Ca T. hypermnestra (Kawazura et al., 2017). Presently it is unknown what the lipid composition is of symbiont envelopes, but marine gammaproteobacteria, especially sulfuroxidizing bacteria, can have a very different lipid composition (Sebastián et al., 2016; Favre et al., 2018). For MreB to localize in the top or bottom of the symbiont cell, where the curvature is high, possibly one of the requirements is to adapt the lipid composition. The amount of RodZ is reported to affect the sensitivity of MreB for curvature (Shi et al., 2018). The organization and amount of RodZ and MreCD (only ± 15% amino acid identity with E. coli versions) in the symbionts might be able to overrule the negative influence of the membrane curvature for MreB localization. For the symbionts to survive on the cuticle of the host longitudinal division was an essential adaptation. In general, evolution works best if increased fitness can be achieved by relatively small changes. MreB and FtsZ of the symbionts are very conserved and their minor changes might explain some of the changes in morphology, but likely the adaptation are to be found in the other proteins that are part of the elongasome and divisome. The alternative model (Figure 1B model 2) in which the symbionts have swapped their axes and the orientation of their cytoskeletal proteins would require different adaptations. For instance, what would prevent MreB to orient perpendicular to their short axis and what would restrict MreB to the medial position only? During septation, the synthetic machinery should discriminate between the top and bottom of the cells, which would have to become inactive with respect to PG synthesis and the envelope in between these parts should remain active areas of PG synthesis. Due to our present lack of knowledge on the morphogenesis of the symbionts, it is not possible to choose between the two models. However, from an evolutionary point of view, Model 1 in Figure 1B might be realized with the least complicated adaptations.
Author Contributions
The author confirms being the sole contributor of this work and approved it for publication.
Conflict of Interest Statement
The author declares that the research was conducted in the absence of any commercial or financial relationships that could be construed as a potential conflict of interest.
Acknowledgments
I would like to thank Norbert O. E. Vischer for modeling the growth of Ca. T. oneisti. I would like to thank Silvia Bulgheresi and Leendert Hamoen for critically reading the manuscript. Special thanks to Silvia Bulgheresi for sharing the symbionts and their wonderful world with me.
Supplementary Material
The Supplementary Material for this article can be found online at: https://www.frontiersin.org/articles/10.3389/fmicb.2018.00822/full#supplementary-material
Supplementary Video 1. Simulation of the growth and division of a Ca. Thiosymbion oneisti cell.
References
Aaron, M., Charbon, G., Lam, H., Schwarz, H., Vollmer, W., and Jacobs-Wagner, C. (2007). The tubulin homologue FtsZ contributes to cell elongation by guiding cell wall precursor synthesis in Caulobacter crescentus. Mol. Microbiol. 64, 938–952. doi: 10.1111/j.1365-2958.2007.05720.x
Aarsman, M. E., Piette, A., Fraipont, C., Vinkenvleugel, T. M., Nguyen-Distèche, M., and Blaauwen, d. T. (2005). Maturation of the Escherichia coli divisome occurs in two steps. Mol. Microbiol. 55, 1631–1645. doi: 10.1111/j.1365-2958.2005.04502.x
Adams, D. W., and Errington, J. (2009). Bacterial cell division: assembly, maintenance and disassembly of the Z ring. Nat. Rev. Microbiol. 7, 642–653. doi: 10.1038/nrmicro2198
Bajaj, R., Bruce, K. E., Davidson, A. L., Rued, B. E., Stauffacher, C. V., and Winkler, M. E. (2016). Biochemical characterization of essential cell division proteins FtsX and FtsE that mediate peptidoglycan hydrolysis by PcsB in Streptococcus pneumoniae. Microbiologyopen 5, 738–752. doi: 10.1002/mbo3.366
Bean, G. J., Flickinger, S. T., Westler, W. M., McCully, M. E., Sept, D., Weibel, D. B., et al. (2009). A22 disrupts the bacterial actin cytoskeleton by directly binding and inducing a low-affinity state in MreB. Biochemistry 48, 4852–4857. doi: 10.1021/bi900014d
Billings, G., Ouzounov, N., Ursell, T., Desmarais, S. M., Shaevitz, J., Gitai, Z., et al. (2014). De novo morphogenesis in L-forms via geometric control of cell growth. Mol. Microbiol. 93, 883–896. doi: 10.1111/mmi.12703
Bisson-Filho, A. W., Hsu, Y. P., Squyres, G. R., Kuru, E., Wu, F., Jukes, C., et al. (2017). Treadmilling by FtsZ filaments drives peptidoglycan synthesis and bacterial cell division. Science 355, 739–743. doi: 10.1126/science.aak9973
Brown, P. J. B., De Pedro, M. A., Kysela, D. T., Van der Henst, C., Kim, J., De Bolle, X., et al. (2012). Polar growth in the Alphaproteobacterial order Rhizobiales. Proc. Natl. Acad. Sci. U.S.A. 109, 1697–1701. doi: 10.1073/pnas.1114476109
Buss, J., Coltharp, C., Huang, T., Pohlmeyer, C., Wang, S. C., Hatem, C., et al. (2013). In vivo organization of the FtsZ-ring by ZapA and ZapB revealed by quantitative super-resolution microscopy. Mol. Microbiol. 89, 1099–1120. doi: 10.1111/mmi.12331
Buss, J., Coltharp, C., Shtengel, G., Yang, X., Hess, H., and Xiao, J. (2015). A multi-layered protein network stabilizes the Escherichia coli FtsZ-ring and modulates constriction dynamics. PLoS Genet. 11:e1005128. doi: 10.1371/journal.pgen.1005128
Cameron, T. A., Zupan, J. R., and Zambryski, P. C. (2015). The essential features and modes of bacterial polar growth. Trends Microbiol. 23, 347–353. doi: 10.1016/j.tim.2015.01.003
Carballido-López, R., and Errington, J. (2003). The bacterial cytoskeleton: in vivo dynamics of the actin-like protein Mbl of Bacillus subtilis. Dev. Cell 4, 19–28. doi: 10.1016/S1534-5807(02)00403-3
Cho, H., Uehara, T., and Bernhardt, T. G. (2014). Beta-lactam antibiotics induce a lethal malfunctioning of the bacterial cell wall synthesis machinery. Cell 159, 1300–1311. doi: 10.1016/j.cell.2014.11.017
Cho, H., Wivagg, C. N., Kapoor, M., Barry, Z., Rohs, P. D. A., Suh, H., et al. (2016). Bacterial cell wall biogenesis is mediated by SEDS and PBP polymerase families functioning semi-autonomously. Nat. Microbiol. 1:16172. doi: 10.1038/nmicrobiol.2016.172
Claessen, D., Rozen, D. E., Kuipers, O. P., Søgaard-Andersen, L., and van Wezel, G. P. (2014). Bacterial solutions to multicellularity: a tale of biofilms, filaments and fruiting bodies. Nat. Rev. Microbiol. 12, 115–124. doi: 10.1038/nrmicro3178
Collier, J. (2016). Cell cycle control in Alphaproteobacteria. Curr. Opin. Microbiol. 30, 107–113. doi: 10.1016/j.mib.2016.01.010
de Pedro, M. A., Quintela, J. C., Höltje, J. V., and Schwarz, H. (1997). Murein segregation in Escherichia coli. J. Bacteriol. 179, 2823–2834. doi: 10.1128/jb.179.9.2823-2834.1997
Domínguez-Escobar, J., Chastanet, A., Crevenna, A. H., Fromion, V., Wedlich-Söldner, R., and Carballido-López, R. (2011). Processive movement of MreB-associated cell wall biosynthetic complexes in bacteria. Science 333, 225–228. doi: 10.1126/science.1203466
Dye, N. A., Pincus, Z., Fisher, I. C., Shapiro, L., and Theriot, J. A. (2011). Mutations in the nucleotide binding pocket of MreB can alter cell curvature and polar morphology in Caulobacter. Mol. Microbiol. 81, 368–394. doi: 10.1111/j.1365-2958.2011.07698.x
Egan, A. J., Biboy, J., van't Veer, I., Breukink, E., and Vollmer, W. (2015). Activities and regulation of peptidoglycan synthases. Philos. Trans. R. Soc. Lond. B. Biol. Sci. 370:20150031. doi: 10.1098/rstb.2015.0031
Eswara, P. J., and Ramamurthi, K. S. (2017). Bacterial cell division: nonmodels poised to take the spotlight. Annu. Rev. Microbiol. 71, 393–411. doi: 10.1146/annurev-micro-102215-095657
Favre, L., Ortalo-Magné, A., Pichereaux, C., Gargaros, A., Burlet-Schiltz, O., Cotelle, V., et al. (2018). Metabolome and proteome changes between biofilm and planktonic phenotypes of the marine bacterium Pseudoalteromonas lipolytica TC8. Biofouling 50, 1–17. doi: 10.1080/08927014.2017.1413551
Fenton, A. K., and Gerdes, K. (2013). Direct interaction of FtsZ and MreB is required for septum synthesis and cell division in Escherichia coli. EMBO J. 32, 1953–1965. doi: 10.1038/emboj.2013.129
Fenton, A. K., Lambert, C., Wagstaff, P. C., and Sockett, R. E. (2010). Manipulating Each MreB of Bdellovibrio bacteriovorus gives diverse morphological and predatory phenotypes. J. Bacteriol. 192, 1299–1311. doi: 10.1128/JB.01157-09
Figge, R. M., Divakaruni, A. V., and Gober, J. W. (2004). MreB, the cell shape-determining bacterial actin homologue, co-ordinates cell wall morphogenesis in Caulobacter crescentus. Mol. Microbiol. 51, 1321–1332. doi: 10.1111/j.1365-2958.2003.03936.x
Fleurie, A., Lesterlin, C., Manuse, S., Zhao, C., Cluzel, C., Lavergne, J. P., et al. (2014). MapZ marks the division sites and positions FtsZ rings in Streptococcus pneumoniae. Nature 516, 259–262. doi: 10.1038/nature13966
Gamba, P., Veening, J.-W., Saunders, N. J., Hamoen, L. W., and Daniel, R. A. (2009). Two-step assembly dynamics of the Bacillus subtilis divisome. J. Bacteriol. 191, 4186–4194. doi: 10.1128/JB.01758-08
Gao, P., Ma, C., Sun, Z., Wang, L., Huang, S., Su, X., et al. (2017). Feed-additive probiotics accelerate yet antibiotics delay intestinal microbiota maturation in broiler chicken. Microbiome 5:91. doi: 10.1186/s40168-017-0315-1
Garner, E. C., Bernard, R., Wang, W., Zhuang, X., Rudner, D. Z., and Mitchison, T. (2011). Coupled, circumferential motions of the cell wall synthesis machinery and MreB filaments in B. subtilis. Science 333, 222–225. doi: 10.1126/science.1203285
Gitai, Z., Dye, N. A., Reisenauer, A., Wachi, M., and Shapiro, L. (2005). MreB actin-mediated segregation of a specific region of a bacterial chromosome. Cell 120, 329–341. doi: 10.1016/j.cell.2005.01.007
Gitai, Z., Dye, N., and Shapiro, L. (2004). An actin-like gene can determine cell polarity in bacteria. Proc. Natl. Acad. Sci. U.S.A. 101, 8643–8648. doi: 10.1073/pnas.0402638101
Goley, E. D., Yeh, Y. C., Hong, S. H., Fero, M. J., Abeliuk, E., McAdams, H. H., et al. (2011). Assembly of the Caulobacter cell division machine. Mol. Microbiol. 80, 1680–1698. doi: 10.1111/j.1365-2958.2011.07677.x
Gupta, R., Lavollay, M., Mainardi, J.-L., Arthur, M., Bishai, W. R., and Lamichhane, G. (2010). The Mycobacterium tuberculosis protein Ldt Mt2 is a nonclassical transpeptidase required for virulence and resistance to amoxicillin. Nat. Med. 16, 466–469. doi: 10.1038/nm.2120
Hale, C. A., and de Boer, P. A. (1997). Direct binding of FtsZ to ZipA, an essential component of the septal ring structure that mediates cell division in E. coli. Cell 88, 175–185. doi: 10.1016/S0092-8674(00)81838-3
Holden, S. J., Pengo, T., Meibom, K. L., Fernandez Fernandez, C., Collier, J., and Manley, S. (2014). High throughput 3D super-resolution microscopy reveals Caulobacter crescentus in vivo Z-ring organization. Proc. Natl. Acad. Sci. U.S.A. 111, 4566–4571. doi: 10.1073/pnas.1313368111
Hooper, D. C. (2001). Mechanisms of action of antimicrobials: focus on fluoroquinolones. Clin. Infect. Dis. 32, S9–S15. doi: 10.1086/319370
Hugonnet, J. E., Mengin-Lecreulx, D., Monton, A., Blaauwen, d. T., Carbonnelle, E., Veckerlé, C., et al. (2016). Factors essential for L,D-transpeptidase-mediated peptidoglycan cross-linking and β-lactam resistance in Escherichia coli. Elife 5:e19469. doi: 10.7554/eLife.19469
Jacq, M., Adam, V., Bourgeois, D., Moriscot, C., Di Guilmi, A. M., Vernet, T., et al. (2015). Remodeling of the Z-Ring Nanostructure during the Streptococcus pneumoniae cell cycle revealed by photoactivated localization microscopy. MBio 6:e01108-15. doi: 10.1128/mBio.01108-15
Jakimowicz, D., and van Wezel, G. P. (2012). Cell division and DNA segregation in Streptomyces: how to build a septum in the middle of nowhere? Mol. Microbiol. 85, 393–404. doi: 10.1111/j.1365-2958.2012.08107.x
Jones, L. J., Carballido-López, R., and Errington, J. (2001). Control of cell shape in bacteria: helical, actin-like filaments in Bacillus subtilis. Cell 104, 913–922. doi: 10.1016/S0092-8674(01)00287-2
Karczmarek, A., Martínez-Arteaga, R., Baselga, R. M.-A., Alexeeva, S., Hansen, F. G., Vicente, M., et al. (2007). DNA and origin region segregation are not affected by the transition from rod to sphere after inhibition of Escherichia coli MreB by A22. Mol. Microbiol. 65, 51–63. doi: 10.1111/j.1365-2958.2007.05777.x
Kawazura, T., Matsumoto, K., Kojima, K., Kato, F., Kanai, T., Niki, H., et al. (2017). Exclusion of assembled MreB by anionic phospholipids at cell poles confers cell polarity for bidirectional growth. Mol. Microbiol. 104, 472–486. doi: 10.1111/mmi.13639
Kocaoglu, O., and Carlson, E. E. (2015). Profiling of β-lactam selectivity for penicillin-binding proteins in Escherichia coli strain DC2. Antimicrob. Agents Chemother. 59, 2785–2790. doi: 10.1128/AAC.04552-14
Kuru, E., Lambert, C., Rittichier, J., Till, R., Ducret, A., Derouaux, A., et al. (2017). Fluorescent D -amino-acids reveal bi-cellular cell wall modifications important for Bdellovibrio bacteriovorus predation. Nat. Microbiol. 2, 1648–1657. doi: 10.1038/s41564-017-0029-y
Kysela, D. T., Randich, A. M., Caccamo, P. D., and Brun, Y. V. (2016). Diversity takes shape: understanding the mechanistic and adaptive basis of bacterial morphology. PLoS Biol. 14:e1002565. doi: 10.1371/journal.pbio.1002565
Land, A. D., and Winkler, M. E. (2011). The requirement for pneumococcal MreC and MreD is relieved by inactivation of the gene encoding PBP1a. J. Bacteriol. 193, 4166–4179. doi: 10.1128/JB.05245-11
Leaver, M., and Errington, J. (2005). Roles for MreC and MreD proteins in helical growth of the cylindrical cell wall in Bacillus subtilis. Mol. Microbiol. 57, 1196–1209. doi: 10.1111/j.1365-2958.2005.04736.x
Lee, T. K., Tropini, C., Hsin, J., Desmarais, S. M., Ursell, T. S., Gong, E., et al. (2014). A dynamically assembled cell wall synthesis machinery buffers cell growth. Proc. Natl. Acad. Sci. U.S.A. 111, 4554–4559. doi: 10.1073/pnas.1313826111
Leisch, N., Pende, N., Weber, P. M., Gruber-Vodicka, H. R., Verheul, J., Vischer, N. O., et al. (2016). Asynchronous division by non-ring FtsZ in the gammaproteobacterial symbiont of Robbea hypermnestra. Nat. Microbiol. 2:16182. doi: 10.1038/nmicrobiol.2016.182
Leisch, N., Verheul, J., Heindl, N. R., Gruber-Vodicka, H. R., Pende, N., den Blaauwen, T., et al. (2012). Growth in width and FtsZ ring longitudinal positioning in a gammaproteobacterial symbiont. Curr. Biol. 22, R831–R832. doi: 10.1016/j.cub.2012.08.033
Letek, M., Fiuza, M., Ordóñez, E., Villadangos, A. F., Ramos, A., Mateos, L. M., et al. (2008). Cell growth and cell division in the rod-shaped actinomycete Corynebacterium glutamicum. Antonie Van Leeuwenhoek 94, 99–109. doi: 10.1007/s10482-008-9224-4
Liechti, G., Kuru, E., Packiam, M., Hsu, Y. P., Tekkam, S., Hall, E., et al. (2016). Pathogenic Chlamydia lack a classical sacculus but synthesize a narrow, mid-cell peptidoglycan ring, regulated by MreB, for cell division. PLoS Pathog. 12:e1005590. doi: 10.1371/journal.ppat.1005590
Liechti, G. W., Kuru, E., Hall, E., Kalinda, A., Brun, Y. V., VanNieuwenhze, M., et al. (2014). A new metabolic cell-wall labelling method reveals peptidoglycan in Chlamydia trachomatis. Nature 506, 507–510. doi: 10.1038/nature12892
Loose, M., and Mitchison, T. J. (2014). The bacterial cell division proteins FtsA and FtsZ self-organize into dynamic cytoskeletal patterns. Nat. Cell Biol. 16, 38–46. doi: 10.1038/ncb2885
Meeske, A. J., Riley, E. P., Robins, W. P., Uehara, T., Mekalanos, J. J., Kahne, D., et al. (2016). SEDS proteins are a widespread family of bacterial cell wall polymerases. Nature 537, 634–638. doi: 10.1038/nature19331
Meeske, A. J., Sham, L. T., Kimsey, H., Koo, B.-M., Gross, C. A., Bernhardt, T. G., et al. (2015). MurJ and a novel lipid II flippase are required for cell wall biogenesis in Bacillus subtilis. Proc. Natl. Acad. Sci. U.S.A. 112, 6437–6442. doi: 10.1073/pnas.1504967112
Mohammadi, T., Karczmarek, A., Crouvoisier, M., Bouhss, A., Mengin-Lecreulx, D., and den Blaauwen, T. (2007). The essential peptidoglycan glycosyltransferase MurG forms a complex with proteins involved in lateral envelope growth as well as with proteins involved in cell division in Escherichia coli. Mol. Microbiol. 65, 1106–1121. doi: 10.1111/j.1365-2958.2007.05851.x
Monteiro, J. M., Fernandes, P. B., Vaz, F., Pereira, A. R., Tavares, A. C., Ferreira, M. T., et al. (2015). Cell shape dynamics during the staphylococcal cell cycle. Nat. Commun. 6:8055. doi: 10.1038/ncomms9055
Morlot, C., Zapun, A., Dideberg, O., and Vernet, T. (2003). Growth and division of Streptococcus pneumoniae: localization of the high molecular weight penicillin-binding proteins during the cell cycle. Mol. Microbiol. 50, 845–855. doi: 10.1046/j.1365-2958.2003.03767.x
Neeli-Venkata, R., Startceva, S., Annila, T., and Ribeiro, A. S. (2016). Polar localization of the serine chemoreceptor of Escherichia coli is nucleoid exclusion-dependent. Biophys. J. 111, 2512–2522. doi: 10.1016/j.bpj.2016.10.024
Olshausen, P. V., Defeu Soufo, H. J., Wicker, K., Heintzmann, R., Graumann, P. L., and Rohrbach, A. (2013). Superresolution imaging of dynamic MreB filaments in B. subtilis-a multiple-motor-driven transport? Biophys. J. 105, 1171–1181. doi: 10.1016/j.bpj.2013.07.038
Ouzounov, N., Nguyen, J. P., Bratton, B. P., Jacobowitz, D., Gitai, Z., and Shaevitz, J. W. (2016). MreB orientation correlates with cell diameter in Escherichia coli. Biophys. J. 111, 1035–1043. doi: 10.1016/j.bpj.2016.07.017
Peltier, J., Courtin, P., Meouche El, I., Lemée, L., Chapot-Chartier, M. P., and Pons, J. L. (2011). Clostridium difficile has an original peptidoglycan structure with a high level of N-acetylglucosamine deacetylation and mainly 3-3 cross-links. J. Biol. Chem. 286, 29053–29062. doi: 10.1074/jbc.M111.259150
Pende, N., Wang, J., Weber, P. M., Verheul, J., Kuru, E., Rittmann, S. K. M. R., et al. (2018). Host-Polarized cell growth in animal symbionts. Curr. Biol. 28, 1039.e5–1051.e5. doi: 10.1016/j.cub.2018.02.028
Pogliano, J., Pogliano, K., Weiss, D. S., Losick, R., and Beckwith, J. (1997). Inactivation of FtsI inhibits constriction of the FtsZ cytokinetic ring and delays the assembly of FtsZ rings at potential division sites. Proc. Natl. Acad. Sci. U.S.A. 94, 559–564. doi: 10.1073/pnas.94.2.559
Potluri, L., Karczmarek, A., Verheul, J., Piette, A., Wilkin, J. M., Werth, N., et al. (2010). Septal and lateral wall localization of PBP5, the major D,D-carboxypeptidase of Escherichia coli, requires substrate recognition and membrane attachment. Mol. Microbiol. 77, 300–323. doi: 10.1111/j.1365-2958.2010.07205.x
Renner, L. D., Eswaramoorthy, P., Ramamurthi, K. S., and Weibel, D. B. (2013). Studying biomolecule localization by engineering bacterial cell wall curvature. PLoS ONE 8:e84143. doi: 10.1371/journal.pone.0084143
Romantsov, T., Culham, D. E., Caplan, T., Garner, J., Hodges, R. S., and Wood, J. M. (2017). ProP-ProP and ProP-phospholipid interactions determine the subcellular distribution of osmosensing transporter ProP in Escherichia coli. Mol. Microbiol. 103, 469–482. doi: 10.1111/mmi.13569
Saaki, T. N. V., Strahl, H., and Hamoen, L. W. (2018). Membrane curvature and the Tol-Pal complex determine polar localization of the chemoreceptor Tar in E. coli. J. Bacteriol. doi: 10.1128/JB.00658-17. [Epub ahead of print].
Salje, J., van den Ent, F., De Boer, P., and Löwe, J. (2011). Direct membrane binding by bacterial actin MreB. Mol. Cell 43, 478–487. doi: 10.1016/j.molcel.2011.07.008
Scheu, P. D., Steinmetz, P. A., Dempwolff, F., Graumann, P. L., and Unden, G. (2014). Polar localization of a tripartite complex of the two-component system DcuS/DcuR and the transporter DctA in Escherichia coli depends on the sensor kinase DcuS. PLoS ONE 9:e115534. doi: 10.1371/journal.pone.0115534
Sebastián, M., Smith, A. F., González, J. M., Fredricks, H. F., Van Mooy, B., Koblížek, M., et al. (2016). Lipid remodelling is a widespread strategy in marine heterotrophic bacteria upon phosphorus deficiency. ISME J. 10, 968–978. doi: 10.1038/ismej.2015.172
Shi, H., Bratton, B. P., Gitai, Z., and Huang, K. C. (2018). How to Build a Bacterial Cell: MreB as the Foreman of E. coli Construction. Cell 172, 1294–1305. doi: 10.1016/j.cell.2018.02.050
Shi, H., Colavin, A., Bigos, M., Tropini, C., Monds, R. D., and Huang, K. C. (2017). Deep phenotypic mapping of bacterial cytoskeletal mutants reveals physiological robustness to cell size. Curr. Biol. 27, 3419.e4–3429.e4. doi: 10.1016/j.cub.2017.09.065
Shiomi, D., Sakai, M., and Niki, H. (2008). Determination of bacterial rod shape by a novel cytoskeletal membrane protein. EMBO J. 27, 3081–3091. doi: 10.1038/emboj.2008.234
Sievers, F., Wilm, A., Dineen, D., Gibson, T. J., Karplus, K., Li, W., et al. (2011). Fast, scalable generation of high-quality protein multiple sequence alignments using Clustal Omega. Mol. Syst. Biol. 7, 539–539. doi: 10.1038/msb.2011.75
Singh, B., Nitharwal, R. G., Ramesh, M., Pettersson, B. M., Kirsebom, L. A., and Dasgupta, S. (2013). Asymmetric growth and division in Mycobacterium spp.: compensatory mechanisms for non-medial septa. Mol. Microbiol. 88, 64–76. doi: 10.1111/mmi.12169
Slovak, P. M., Wadhams, G. H., and Armitage, J. P. (2005). Localization of MreB in Rhodobacter sphaeroides under conditions causing changes in cell shape and membrane structure. J. Bacteriol. 187, 54–64. doi: 10.1128/JB.187.1.54-64.2005
Strauss, M. P., Liew, A. T., Turnbull, L., Whitchurch, C. B., Monahan, L. G., and Harry, E. J. (2012). 3D-SIM super resolution microscopy reveals a bead-like arrangement for FtsZ and the division machinery: implications for triggering cytokinesis. PLoS Biol. 10:e1001389. doi: 10.1371/journal.pbio.1001389
Blaauwen, d. T., Aarsman, M. E. G., Vischer, N. O. E., and Nanninga, N. (2003). Penicillin-binding protein PBP2 of Escherichia coli localizes preferentially in the lateral wall and at mid-cell in comparison with the old cell pole. Mol. Microbiol. 47, 539–547. doi: 10.1046/j.1365-2958.2003.03316.x
den Blaauwen, T., de Pedro, M. A., Nguyen-Distèche, M., and Ayala, J. A. (2008). Morphogenesis of rod-shaped sacculi. FEMS Microbiol. Rev. 32, 321–344. doi: 10.1111/j.1574-6976.2007.00090.x
den Blaauwen, T., Hamoen, L. W., and Levin, P. A. (2017). The divisome at 25: the road ahead. Curr. Opin. Microbiol. 36, 85–94. doi: 10.1016/j.mib.2017.01.007
Tormo, A., and Vicente, M. (1984). The ftsA gene product participates in formation of the Escherichia coli septum structure. J. Bacteriol. 157, 779–784.
Tsui, H. C. T., Boersma, M. J., Vella, S. A., Kocaoglu, O., Kuru, E., Peceny, J. K., et al. (2014). Pbp2x localizes separately from Pbp2b and other peptidoglycan synthesis proteins during later stages of cell division of Streptococcus pneumoniae D39. Mol. Microbiol. 94, 21–40. doi: 10.1111/mmi.12745
Ursell, T. S., Nguyen, J., Monds, R. D., Colavin, A., Billings, G., Ouzounov, N., et al. (2014). Rod-like bacterial shape is maintained by feedback between cell curvature and cytoskeletal localization. Proc. Natl. Acad. Sci. U.S.A. 111, E1025–E1034. doi: 10.1073/pnas.1317174111
van den Ent, F., Amos, L. A., and Löwe, J. (2001). Prokaryotic origin of the actin cytoskeleton. Nature 413, 39–44. doi: 10.1038/35092500
van den Ent, F., Leaver, M., Bendezu, F., Errington, J., de Boer, P., and Löwe, J. (2006). Dimeric structure of the cell shape protein MreC and its functional implications. Mol. Microbiol. 62, 1631–1642. doi: 10.1111/j.1365-2958.2006.05485.x
van der Ploeg, R., Verheul, J., Vischer, N. O. E., Alexeeva, S., Hoogendoorn, E., Postma, M., et al. (2013). Colocalization and interaction between elongasome and divisome during a preparative cell division phase in Escherichia coli. Mol. Microbiol. 87, 1074–1087. doi: 10.1111/mmi.12150
van Teeffelen, S., Wang, S., Furchtgott, L., Huang, K. C., Wingreen, N. S., Shaevitz, J. W., et al. (2011). The bacterial actin MreB rotates, and rotation depends on cell-wall assembly. Proc. Natl. Acad. Sci. U.S.A. 108, 15822–15827. doi: 10.1073/pnas.1108999108
Vats, P., and Rothfield, L. (2007). Duplication and segregation of the actin (MreB) cytoskeleton during the prokaryotic cell cycle. Proc. Natl. Acad. Sci. U.S.A. 104, 17795–17800. doi: 10.1073/pnas.0708739104
Viola, M. G., LaBreck, C. J., Conti, J., and Camberg, J. L. (2017). Proteolysis-dependent remodeling of the tubulin homolog FtsZ at the division septum in Escherichia coli. PLoS ONE 12:e0170505. doi: 10.1371/journal.pone.0170505
Vollmer, W., and Bertsche, U. (2008). Murein (peptidoglycan) structure, architecture and biosynthesis in Escherichia coli. Biochim. Biophys. Acta 1778, 1714–1734. doi: 10.1016/j.bbamem.2007.06.007
Vollmer, W., Joris, B., Charlier, P., and Foster, S. (2008). Bacterial peptidoglycan (murein) hydrolases. FEMS Microbiol. Rev. 32, 259–286. doi: 10.1111/j.1574-6976.2007.00099.x
Wang, S., Furchtgott, L., Huang, K. C., and Shaevitz, J. W. (2012). Helical insertion of peptidoglycan produces chiral ordering of the bacterial cell wall. Proc. Natl. Acad. Sci. U.S.A. 109, E595–E604. doi: 10.1073/pnas.1117132109
Wheeler, R., Turner, R. D., Bailey, R. G., Salamaga, B., Mesnage, S., Mohamad, S. A., et al. (2015). Bacterial cell enlargement requires control of cell wall stiffness mediated by peptidoglycan hydrolases. MBio 6:e00660. doi: 10.1128/mBio.00660-15
White, C. L., Kitich, A., and Gober, J. W. (2010). Positioning cell wall synthetic complexes by the bacterial morphogenetic proteins MreB and MreD. Mol. Microbiol. 76, 616–633. doi: 10.1111/j.1365-2958.2010.07108.x
Woldemeskel, S. A., and Goley, E. D. (2017). Shapeshifting to survive: shape determination and regulation in Caulobacter crescentus. Trends Microbiol. 25, 673–687. doi: 10.1016/j.tim.2017.03.006
Wu, K. J., Zhang, J., Baranowski, C., Leung, V., Rego, E. H., Morita, Y. S., et al. (2018). Characterization of conserved and novel septal factors in Mycobacterium smegmatis. J. Bacteriol. 200:e00649-17. doi: 10.1128/JB.00649-17
Yakhnina, A. A., and Gitai, Z. (2013). Diverse functions for six glycosyltransferases in Caulobacter crescentus cell wall assembly. J. Bacteriol. 195, 4527–4535. doi: 10.1128/JB.00600-13
Yang, D. C., Blair, K. M., and Salama, N. R. (2016). Staying in shape: the impact of cell shape on bacterial survival in diverse environments. Microbiol. Mol. Biol. Rev. 80, 187–203. doi: 10.1128/MMBR.00031-15
Keywords: division, FtsZ, morphology, MreB, peptidoglycan, symbionts
Citation: den Blaauwen T (2018) Is Longitudinal Division in Rod-Shaped Bacteria a Matter of Swapping Axis? Front. Microbiol. 9:822. doi: 10.3389/fmicb.2018.00822
Received: 04 February 2018; Accepted: 11 April 2018;
Published: 08 May 2018.
Edited by:
Ariel Amir, Harvard University, United StatesReviewed by:
Richard A. Daniel, Newcastle University, United KingdomPeter Graumann, Philipps University of Marburg, Germany
Lars Renner, Leibniz Institute of Polymer Research (LG), Germany
Copyright © 2018 den Blaauwen. This is an open-access article distributed under the terms of the Creative Commons Attribution License (CC BY). The use, distribution or reproduction in other forums is permitted, provided the original author(s) and the copyright owner are credited and that the original publication in this journal is cited, in accordance with accepted academic practice. No use, distribution or reproduction is permitted which does not comply with these terms.
*Correspondence: Tanneke den Blaauwen, dC5kZW5ibGFhdXdlbkB1dmEubmw=