- Department for Farm Animals and Veterinary Public Health, Institute of Animal Nutrition and Functional Plant Compounds, University of Veterinary Medicine Vienna, Vienna, Austria
Dietary deoxynivalenol (DON) impairs the intestinal functions and performance in broiler chickens, whereas little is known about the effect of DON on the gastrointestinal microbiota. This study evaluated the impact of graded levels of dietary DON contamination on the cecal bacterial microbiota, their predicted metabolic abilities and short-chain fatty acid (SCFA) profiles in chickens. In using a single oral lipopolysaccharide (LPS) challenge we further assessed whether an additional intestinal stressor would potentiate DON-related effects on the cecal microbiota. Eighty 1-day-old chicks were fed diets with increasing DON concentrations (0, 2.5, 5, and 10 mg DON per kg diet) for 5 weeks and were sampled after half of the chickens received an oral LPS challenge (1 mg LPS/kg bodyweight) 1 day before sampling. The bacterial composition was investigated by Illumina MiSeq sequencing of the V3–5 region of the 16S rRNA gene. DON-feeding decreased (p < 0.05) the cecal species richness (Chao1) and evenness (Shannon) compared to the non-contaminated diet. The phyla Firmicutes and Proteobacteria tended to linearly increase and decrease with increasing DON-concentrations, respectively. Within the Firmicutes, DON decreased the relative abundance of Oscillospira, Clostridiaceae genus, Clostridium, and Ruminococcaceae genus 2 (p < 0.05), whereas it increased Clostridiales genus 2 (p < 0.05). Moreover, increasing DON levels linearly decreased a high-abundance Enterobacteriaceae genus and an Escherichia/Shigella-OTU (p < 0.05). Changes in the bacterial composition and their imputed metagenomic capabilities may be explained by DON-related changes in host physiology and cecal nutrient availability. The oral LPS challenge only decreased the abundance of an unassigned Clostridiales genus 2 (p = 0.03). Increasing dietary concentrations of DON quadratically increased the cecal total SCFA and butyrate concentration (p < 0.05), whereas a DON × LPS interaction indicated that LPS mainly increased cecal total SCFA, butyrate, and acetate concentrations in chickens fed the diets that were not contaminated with DON. The present findings showed that even the lowest level of dietary DON contamination had modulatory effects on chicken's cecal bacterial microbiota composition and diversity, whereas the additional oral challenge with LPS did not potentiate DON effects on the cecal bacterial composition.
Introduction
The gut microbiota plays a crucial role in host health through various functions like vitamin synthesis, digestion of dietary fiber, modulation of the gut epithelial barrier, and inflammatory responses as well as protecting against colonization with pathogens (Maslowski and Mackay, 2011; Kogut and Arsenault, 2016). In chickens, the ceca are the gut site with the highest microbial colonization (Oakley et al., 2014), with Bacteroidaceae, Ruminococcaceae, Lachnospiraceae, and Clostridiaceae being the dominating bacterial taxa (Oakley et al., 2014). Moreover, the longer retention time of digesta in the ceca allows for a more complete microbial breakdown of complex fiber and enhances short-chain fatty acid (SCFA) production compared to the other gut sites (Oakley et al., 2014).
Because of the widespread contamination of cereal grains with the Fusarium toxin deoxynivalenol (DON) combined with the vast use of cereal grains in livestock diets worldwide, DON-contaminated feed is a very harmful factor for animal health (Escrivá et al., 2015), and causes substantial economic losses in poultry production (Awad et al., 2013). Chickens are believed to be sensitive to moderate DON levels that compromise growth performance (Andretta et al., 2011) and functioning of the immune system (Awad et al., 2013). The current guidance value of the European Union that is applicable for complete feed for poultry is 5 mg DON/kg feed (12% moisture) (2006/576/EC, 2006). Consumption of DON impairs the intestinal morphology, nutrient absorption, barrier function, and the innate immune response in chickens (Awad et al., 2011b; Osselaere et al., 2013), whereas the interaction between DON and the chicken gut microbiota has been hardly elucidated (Ghareeb et al., 2015). Evidence from other species (e.g., pigs, human microbiota-associated rats) demonstrates that exposure to DON modifies the gut bacterial community (Waché et al., 2009; Saint-Cyr et al., 2013; Piotrowska et al., 2014). Accordingly, DON increased the genera Bacteroides and Prevotella while decreasing Escherichia coli in a human microbiota-associated rat model (Saint-Cyr et al., 2013). Moreover, the gut microbiota has been reported to metabolize mycotoxin compounds, which may alter the identity and toxicity of metabolites for the host (Gratz et al., 2013, 2017). Due to the role that the gut microbiota plays in priming of gut functions and immune development (Schokker et al., 2015), DON-induced alterations in the gut bacterial composition and microbe-host interactions may account for some of the adverse effects reported for DON (Robert et al., 2017).
The gut microbiota itself releases immune-stimulatory compounds, such as lipopolysaccharides (LPS) (Saadia et al., 1990; Ge et al., 2000; Ghareeb et al., 2016), which are part of the outer membrane of Gram negative bacteria and may depress growth performance in poultry by diverting energy for an elevated immune response (Ghareeb et al., 2016). Oral challenges with LPS have been reported to cause gut mucosal tissue damage (Wu et al., 2013) and modify mucus secretion and composition in vitro (Dohrman et al., 1998; Smirnova et al., 2003; Cornick et al., 2015; Zhang et al., 2017). Moreover, evidence suggests that LPS can interfere with the response to other xenobiotic agents. In doing so, LPS has been shown to increase the toxicity of trichothecenes such as DON in rodent models (Zhou et al., 2000; Ganey and Roth, 2001; Islam and Pestka, 2006). Damage to the gut barrier in duodenum and jejunum may modify intestinal nutrient flows with consequences for the microbial composition and metabolism in the ceca. Therefore, gaining knowledge about potential interactions between common stressors, such as DON and LPS, on gut microbial profiles may help in the development of effective strategies to reduce their deleterious effects in broiler production.
The main objective of the present study was to investigate the effects of a chronic exposure to graded levels of dietary DON contamination on the cecal bacterial microbiota, their predicted metabolic functions and SCFA profile in broiler chickens. Our hypothesis was that the exposure to increasing levels of DON from the first day of life would alter the bacterial community composition and metabolic capabilities in growing chickens, leading to alterations in metabolic fermentation profile and host performance and health. In using a single oral LPS-challenge we further aimed to assess whether an additional stressor for the gut would potentiate DON-related effects on the cecal microbiota. The dietary levels of DON used in this study were chosen on the basis of the current guidance values in the European Union and are therefore of practical relevance. Data of feed intake and performance can be found in our companion paper (Lucke et al., 2017a).
Materials and Methods
Ethics Statement
The animal procedures were approved by the institutional ethics committee of the University of Veterinary Medicine Vienna and the Austrian national authority according to paragraph 26 of Law for Animal Experiments, Tierversuchsgesetz 2012—TVG 2012 (GZ 68.205/0062–WF/V/3b/2015).
Experimental Design
The feeding experiment, housing conditions and diets are described in detail in Lucke et al. (2017a). Briefly, a total of 80 one-day-old broiler chicks (ROSS 308) obtained from a commercial hatchery (Brüterei Schlierbach GmbH, Pettenbach, Austria) were randomly allocated to four feeding groups: (1) control diet without DON (0 DON), (2) control diet experimentally contaminated with 2.5 mg DON/kg diet (2.5 DON; Romer Labs, Tulln, Austria; Supplementary Table S1), (3) control diet with 5 mg DON/kg diet (5 DON), and (4) control diet with 10 mg DON/kg diet (10 DON). The natural DON contamination in the control diet amounted to 0.16 mg/kg diet (Lucke et al., 2017a). The diets were fed from the first day of life until the end of the experimental period (d 34–37 of life). The chickens were housed in flatdeck cages (0.36 m2 each) in groups of 2–4 animals per cage from week 1 to 3 of the experiment and 1–2 birds per cage for the remaining time of the experiment (Lucke et al., 2017a,b). Cage floors were covered with clean cardboard paper every 2 days. One day before (d 33–36) cecal digesta collection, half of the chickens orally received 1 mg LPS per kg body weight (LPS L2880 from E. coli O55:B5, Sigma-Aldrich, St. Louis, Missouri, USA) dissolved in distilled water, via crop gavage, whereas the other half received distilled water as placebo (negative control). This resulted in the following eight treatment groups: 0 DON+con, 0 DON+lps, 2.5 DON+con, 2.5 DON+lps, 5 DON+con, 5 DON+lps, 10 DON+con, 10 DON+lps. On d 34–37, chickens were euthanized by an overdose of Thiopental (50–100 mg/kg body weight medicamentum pharma GmbH, Allerheiligen im Mürztal, Austria) into the wing vein followed by exsanguination. Immediately thereafter, the entire intestine was carefully removed from the abdomen and the ceca were separated. The ceca were opened longitudinally and cecal digesta were collected, homogenized with a sterile spatula, transferred to CryoPure Tubes (Sarstedt AG + Co., Nümbrecht, Germany), snap-frozen in liquid nitrogen and stored at −80°C until analysis.
Genomic DNA Isolation and 16S rRNA Gene Sequencing
Total DNA was isolated from 250 to 300 mg of frozen cecal digesta using the Power Soil DNA Isolation Kit (MoBio Laboratories Inc., Carlsbad, CA, USA) with few modifications as described previously (Metzler-Zebeli et al., 2016). The isolated DNA was stored at −20°C. The DNA concentration was measured using the Qubit 2.0 Fluorometer (Life Technologies, Carlsbad, CA, USA) and the Qubit dsDNA HS Assay Kit (Life technologies, Carlsbad, CA, USA).
The 16S rRNA gene PCR of the V3–V5 hypervariable region (primer set 357F_hmp (CCTACGGGAGGCAGCAG) and 926R_hmp (CCGTCAATTCMTTTRAGT), product length ~570 bp), library preparation and Illumina MiSeq sequencing were carried out by Microsynth AG (Balgach, Switzerland) (Metzler-Zebeli et al., 2015). Libraries were constructed by ligating sequencing adapters and indices onto purified PCR products using the Nextera XT sample preparation kit (Illumina Inc., San Diego, CA, USA) according to the recommendations of the manufacturer. For each of the libraries, equimolar amounts were pooled and sequenced on an Illumina MiSeq Personal Sequencer using a 300 bp read length paired-end protocol. The resultant overlapping paired-end reads were demultiplexed, trimmed using cutadapt (Martin, 2011; https://cutadapt.readthedocs.org/), stitched using Fast Length Adjustment of SHort reads (FLASH; http://www.cbcb.umd.edu/software/flash; Magoč and Salzberg, 2011) by Microsynth. In total, 3,627,930 stitched reads for the 80 cecal samples with a mean Phred score of 34–36 were obtained from the commercial provider.
Bioinformatic Analysis
Sequencing data were analyzed using QIIME (version 1.9.1; Siegerstetter et al., 2017). Fastq files were quality filtered using a quality score of 15. Chimera were detected and removed by the UCHIME method using the 64-bit version of USEARCH and the GOLD database (drive5.com; Edgar, 2010; Edgar et al., 2011). Open-reference operational taxonomic unit (OTU) picking was performed at 97% similarity level using UCLUST (Edgar, 2010). OTU taxonomy was assigned against the Greengenes database (gg_13_8; http://qiime.org/home_static/dataFiles.html). Rare OTUs with less than 10 sequences were removed. After quality control and removal of chimeras, 2,860,912 sequences remained, with a mean of 35,761 reads per sample and mean read length of 536 bp which were classified into 2,989 OTUs. For α-diversity analyses a rarefaction depth of 17,500 sequences per sample was used. OTUs being differently abundant between treatments were additionally checked using the Greengenes database (http://greengenes.lbl.gov). Proposed species obtained by BLAST sharing the highest percentage of similarity were reported. The raw sequence reads were uploaded to the NCBI BioProject databank under the project ID: PRJNA419703.
Microbial function prediction for each cecal sample based on 16S rRNA gene sequencing data was determined using Phylogenetic Investigation of Communities by Reconstruction of Unobserved States (PICRUSt; Langille et al., 2013). For this, closed-reference OTU picking was performed at 97% similarity level against the Greengenes database (downloaded from http://greengenes.secondgenome.com/downloads/database/13_5), clustered based on a 0.03 distance limit, and processed in the online Galaxy PICRUSt interface (http://galaxyproject.org/) with a workflow described by the developers (http://picrust.github.com/picrust/tutorials/quickstart.html#quickstartguide). Sequences were categorized by function based on cluster of orthologous groups of proteins (COG) and Kyoto Encyclopedia of Genes and Genomes (KEGG) pathways in PICRUSt. Non-bacteria related COG and KEGG orthology functions were dismissed.
Microbial Fermentation
The SCFA (i.e., acetic acid, propionic acid, isobutyric acid, n-butyric acid, isovaleric acid, n-valeric acid, and caproic acid) in cecal digesta were determined using gas chromatography (GC). For this, 0.5 g of cecal digesta was mixed with 0.5 g H20 (double-distilled), 200 μl phosphoric acid (25%), and 300 μl of an internal standard (4-methylvalerian acid). Samples were vortexed and centrifuged at 16,300 × g for 20 min. The supernatant was collected into a fresh tube. Centrifugation steps were repeated until the supernatant became clear. Afterwards, the supernatant was stored at −80°C and analyzed on the GC (Fisons GC Model 8060 MS DFPC, Rodano, Italy) equipped with a 30 m × 0.53 mm × 0.5 μm capillary column, a flame-ionization detector (Fisons EL980) and an autosampler (Fisons autosampler Modell AS 800 C.U.). Helium was used as carrier gas (flow rate: 2 ml/min) and the temperatures of the injector and detector were set at 170 and 190°C, respectively. The GC oven program was defined as follows: The starting temperature was set at 65°C and was heated with a heating rate of 15 K/min to 170°C. The program continued with a heating rate of 35 K/min to 190°C and afterwards with a heating rate of 40 K/min to 200°C.
Statistical Analysis
The Shapiro-Wilk test was firstly used to test for normality of data distribution for all variables using the PROC UNIVARIATE procedure in SAS (version 9.4; SAS Inst. Inc., Cary, NC). After establishing normality for all data, relative bacterial abundances, relative predicted microbial function abundances and SCFA concentrations were analyzed by ANOVA using the MIXED procedure of SAS. DON treatment, LPS and their interaction were considered as fixed effects. The experimental run was included as random effect with chicken nested within group and day as the experimental unit. Orthogonal contrasts were used to test linear and quadratic relationships between control feeding and the 3 increasing levels of DON as well as the overall difference of 0 DON vs. all DON groups. Degrees of freedom were approximated using Kenward-Rogers method (ddfm = kr). Least squares means and the standard error of the mean are presented. Pairwise comparisons among least squares means were performed using the probability of difference (pdiff) option in SAS. Significance was declared if p < 0.05 and a trend was reported if p < 0.10. Fold-changes were calculated by dividing the difference between a treatment and the control by the value of the respective control. Pearson's correlations were calculated with the CORR procedure of SAS. Only significant correlations are reported and correlation matrices were visualized using the corrplot package in R Studio version 3.4.1 (Wei and Simko, 2016).
Results
Overall Bacterial Community Composition
Across all treatment groups (Figure 1), Firmicutes (86.0%) and Proteobacteria (13.2%) were the most abundant phyla in cecal digesta. At family level, the cecal community was dominated by an unassigned family of the order Clostridiales (38.1%) and Ruminococcaceae (30.6%) followed by Enterobacteriaceae (13.2%), Turicibacteriaceae (8.8%), and Lachnospiraceae (7.0%) (Table 1). Correspondingly, the predominating genera were an unassigned genera of the order Clostridiales (38.1%), Ruminococcaceae (20.7%), and Enterobacteriaceae (12.5%, largely represented by the Escherichia/Shigella OTU2; Table 2, Supplementary Tables S2, S3), Turicibacter (8.8%), Ruminococcus (5.8%), an unassigned genus of the family Lachnospiraceae (3.0%), and Oscillospira (2.4%).
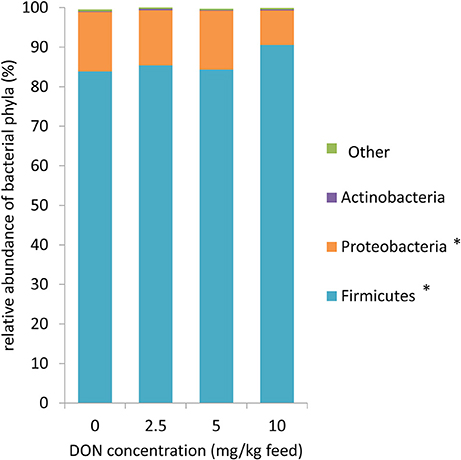
Figure 1. Microbiome composition at phylum level for 16S rRNA sequences in cecal digesta of broiler chickens fed diets with increasing levels of deoxynivalenol (DON; 0, 2.5, 5, or 10 mg DON/ kg diet). Values are presented as least squares means ± standard error of the mean (SEM); n = 20 broilers per feeding group. Presented data include both animals with and without oral lipopolysaccharide challenge (LPS) 1 day prior to slaughter within the respective feeding group. Data were not affected by LPS and DON × LPS (p > 0.10). * Linear contrast: p < 0.10.
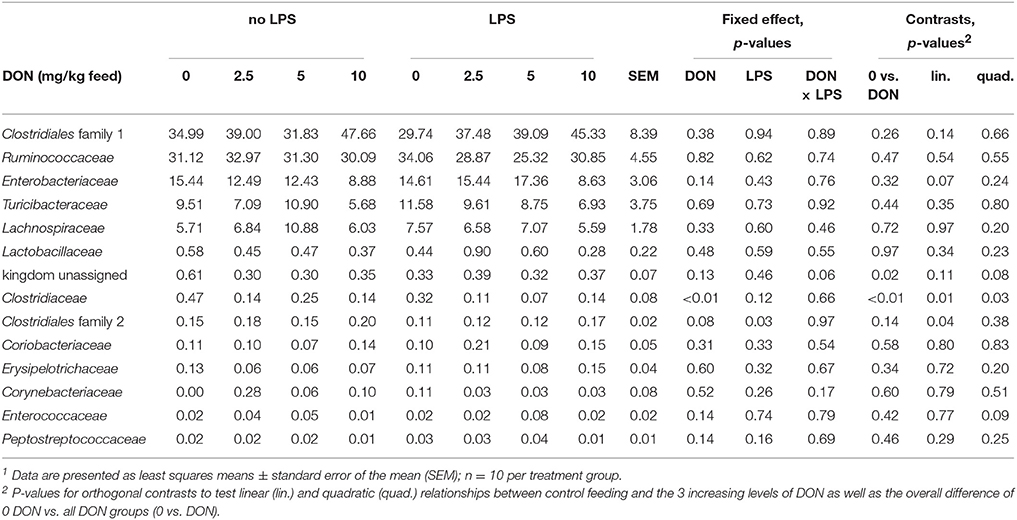
Table 1. Relative abundance (%) of families in cecal digesta of broiler chickens fed diets with increasing levels of deoxynivalenol (DON; 0, 2.5, 5, or 10 mg DON/kg diet) and with or without oral lipopolysaccharide challenge (LPS) 1 day prior slaughter1.
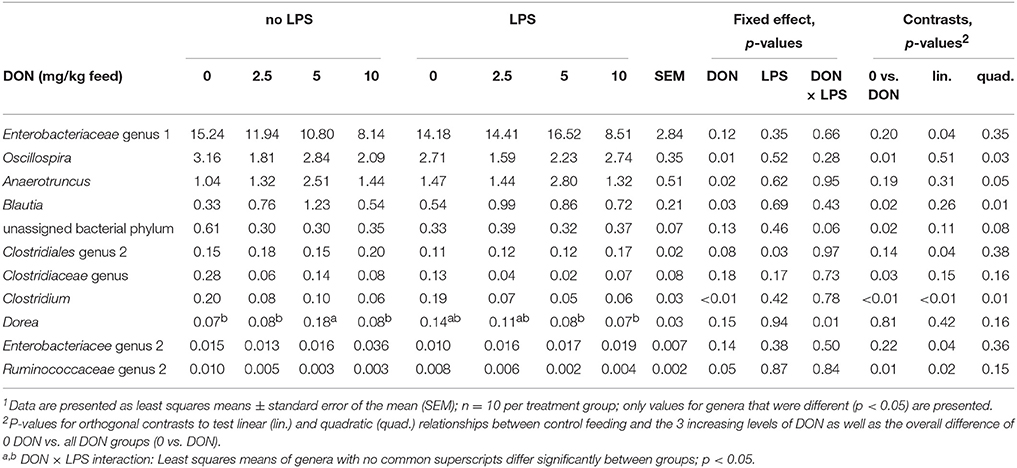
Table 2. Differences in relative abundance (%) of genera in cecal digesta of broiler chickens fed diets with increasing levels of deoxynivalenol (DON; 0, 2.5, 5, or 10 mg DON/kg diet) and with or without oral lipopolysaccharide challenge (LPS) 1 day prior slaughter1.
Effects of DON and LPS Treatment on Bacterial Composition
The exposure to increasing levels of DON decreased (p < 0.05) the cecal species richness (Chao1) and evenness (Shannon) by up to 0.2- and 0.1-fold, respectively, compared to 0 DON (Figure 2). Increasing DON levels also affected the bacterial community composition as high as the phylum level. The greatest alterations were observed within the predominant phyla Firmicutes and Proteobacteria which tended (p < 0.10) to linearly increase and decrease by up to 0.1- and 0.4-fold with increasing DON-levels, respectively (Figure 1). Accordingly, the ratio of Firmicutes to Proteobacteria tended to linearly increase (p = 0.08) by up to 0.9-fold with increasing DON-concentrations (Supplementary Table S2). The most pronounced DON-induced changes at family level were a 0.6-fold decrease in the relative abundance of Clostridiaceae in the DON groups (p < 0.01) and a linear increase in an unassigned Clostridiales family 2 by 0.5-fold with increasing DON concentrations (p = 0.04). This was reflected at genus level where Oscillospira, Clostridiaceae genus, Clostridium, and the unassigned Ruminoccaceae genus 2 were decreased by the DON treatment (p < 0.05). Oscillospira and the unassigned Ruminoccaceae genus 2 decreased by 0.2- and 0.5-fold in the DON groups compared to the 0 DON group, respectively, whereas Clostridium linearly decreased by up to 0.7-fold with increasing DON concentrations. DON further quadratically increased the relative abundance of Blautia (p = 0.01) and Anaerotruncus (p = 0.05) by up to 1.4- and 1.1-fold with the highest abundance in the 5 DON group. Moreover, an unassigned Enterobacteriaceae genus 1 linearly decreased (p < 0.05) with increasing DON-concentrations by up to 0.4-fold. Within these genera, the DON treatment affected 38 of the 120 most abundant OTUs (relative abundance > 0.05; Supplementary Figure S1). Among those, 5 OTUs could be assigned to >95% similarity to reference strains (Supplementary Table S3). Increasing DON levels linearly decreased Escherichia/Shigella-OTU2 by up to 0.5-fold and two Salmonella-OTUs (OTU 70 and 73) by up to 0.7- and 0.5-fold, respectively (p < 0.05). Two Anaerotruncus-OTUs (OTU 18 and 22) tended to be quadratically affected by the increasing DON levels, reaching their highest abundance in cecal digesta of chickens in the 5 DON group.
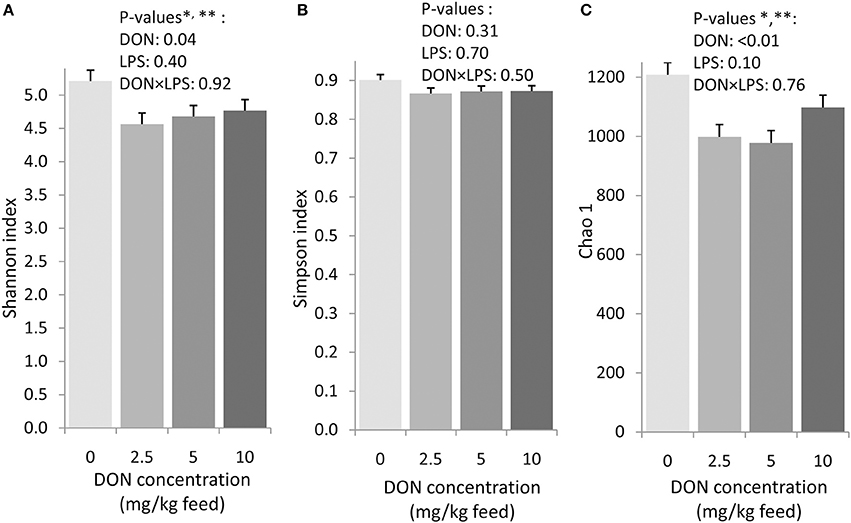
Figure 2. Shannon index (A), Simpson index (B), and Chao1 richness estimate (C) in cecal digesta of chickens fed diets with increasing levels of deoxynivalenol (DON; 0, 2.5, 5, or 10 mg DON/kg diet). Values are presented as least squares means ± standard error of the mean (SEM); n = 20 broilers per feeding group. Presented data include both animals with and without oral lipopolysaccharide challenge (LPS) 1 day prior to slaughter within the respective feeding group. * Contrast comparing the 0 DON with all DON groups (0 vs. DON): p < 0.05. ** Quadratic contrast: p < 0.05.
The oral LPS challenge decreased the relative abundance of an unassigned Clostridiales genus 2 by 0.2-fold compared to the negative control (p = 0.03). At OTU level, the relative abundance of only 4 out of the 120 most abundant OTUs were modified by the LPS treatment.
Moreover, the DON × LPS interaction (p = 0.01) for the genus Dorea indicated that there was a quadratic response of Dorea to the increasing DON concentrations in chickens that did not receive the LPS challenge. In chickens that were additionally challenged with LPS increasing DON levels did not modify the relative abundance of Dorea.
Effects of DON and LPS Treatment on Short-Chain Fatty Acids
Increasing dietary concentrations of DON quadratically affected the cecal total SCFA and butyrate concentration (p < 0.05, Figure 3), with the highest concentration in the 2.5 DON group (Figure 3). The DON × LPS interactions for total SCFA (p = 0.01) and butyric acid (p < 0.01) further indicated that those effects were only observed in chickens that were not challenged with LPS. In chickens that received the LPS, SCFA concentrations linearly decreased with increasing DON concentrations. Furthermore, an opposite quadratic relationship between iso-valerate and increasing DON concentrations was observed (p = 0.01), with the lowest concentration of iso-valerate being found in the 2.5 and 5 DON groups.
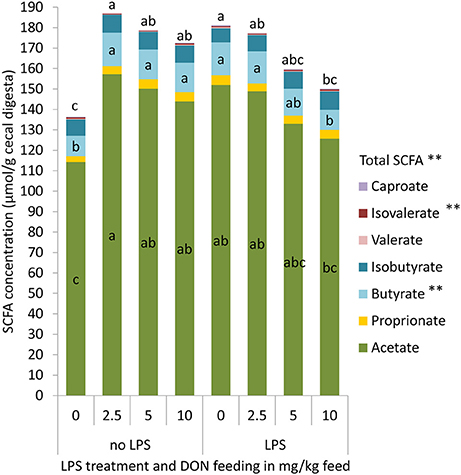
Figure 3. Total short chain fatty acids (SCFA), acetate, proprionate, butyrate, isobutyrate, valerate, isovalerate, and caproate concentrations in cecal digesta of chickens fed diets with increasing levels of deoxynivalenol (DON; 0, 2.5, 5, or 10 mg DON/kg diet) and with or without oral lipopolysaccharide challenge (LPS) 1 day prior to slaughter. Values are presented as least squares means ± standard error of the mean (SEM); n = 10 broilers per treatment; n = 9 in the 5 DON+con and 5 DON+lps groups. a,b,c DON × LPS interaction: Least squares means of total or individual SCFA with no common superscripts differ significantly between groups; p < 0.05. **Quadratic contrast: p < 0.05.
Effect of DON and LPS Treatments on Functional Metagenome Prediction
Functional metagenome prediction was used to assess potential alterations in bacterial metabolism caused by the dietary DON levels (Table 3; Supplementary Table S4). The relative gene abundance of COG pathways for metabolism of co-factors and vitamins, cell motility, and biosynthesis of other secondary metabolites were linearly increased with increasing dietary DON levels (p < 0.05; Table 3). The relative abundance of COG pathway genes for lipid metabolism, genetic information processing and glycan biosynthesis and metabolism, in turn, were linearly decreased by increasing DON levels (p < 0.05). In total, 35 KEGG pathways were less abundant in chickens fed the DON diets compared to the 0 DON diet, including KEGG pathways for amino acid, carbohydrate, and lipid metabolism and cell replication. In contrast, 21 KEGG pathways were more abundant in chickens fed the DON diets compared to those fed the 0 DON diet and were related to bacterial motility, cell metabolism, amino acid, vitamin, and antimicrobial metabolism. The LPS treatment, in turn, did not affect the relative abundance of predicted metabolic pathways.
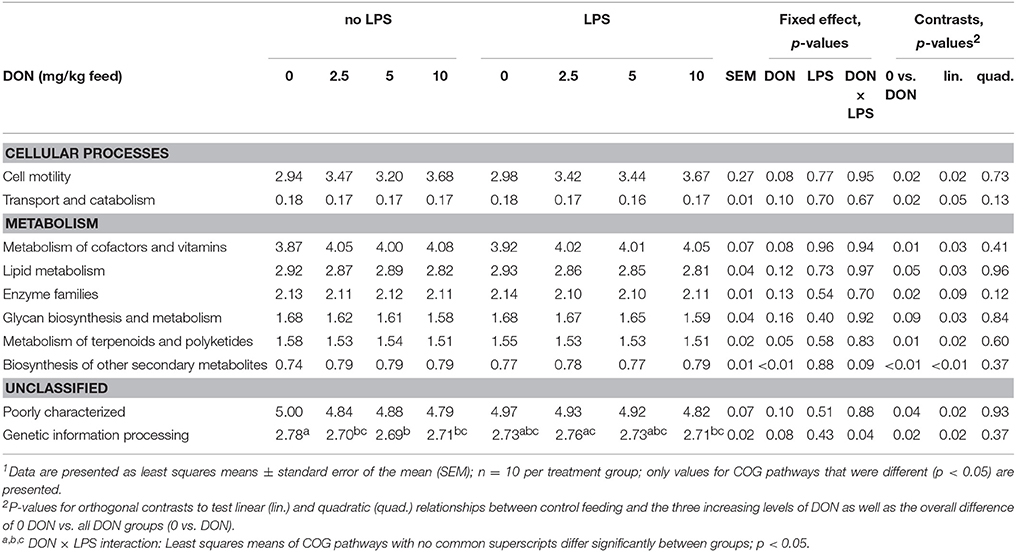
Table 3. Differences in relative abundance (%) of COG pathways (Cluster of orthologous groups of proteins) in cecal digesta of broiler chickens fed diets with increasing levels of deoxynivalenol (DON; 0, 2.5, 5, or 10 mg DON/kg diet) and with or without oral lipopolysaccharide challenge (LPS) 1 day prior slaughter1.
Correlation Analysis
Pearson's correlation analysis was used to characterize associations of bacterial abundances with SCFA concentrations and predicted metabolic functions in cecal digesta. In total, 15 significant correlations between bacterial genera and SCFA could be established (Figure 4). Most correlations were observed within the phylum Firmicutes. Ruminococcaceae genus 2 positively correlated with valerate (r = 0.42). Moreover, several genera positively correlated with caproate including [Ruminococcus] (r = 0.36), Citrobacter (r = 0.42), and Ruminococcaceae genus 2 (r = 0.44). Furthermore, a negative correlation between body weight at the day of sampling (Lucke et al., 2017a) and caproate (r = −0.37) could be established. In addition, slaughter weight positively correlated with Clostridiales genus 1 (r = 0.38). However, correlations of slaughter weight with Blautia (r = −0.36) and Escherichia (r = −0.36) were negative.
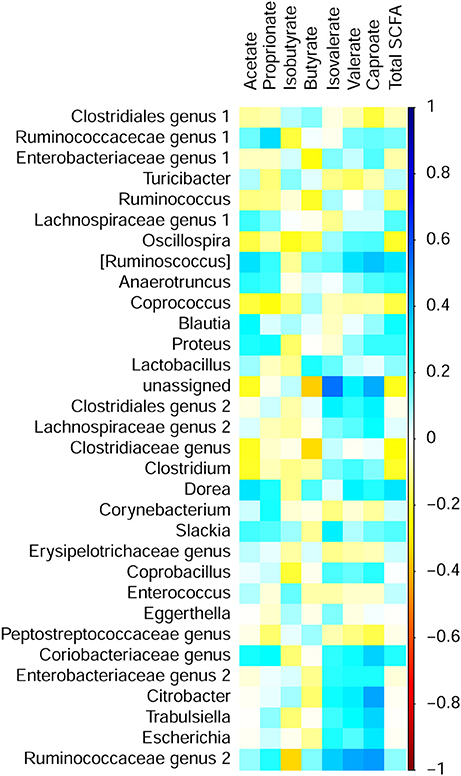
Figure 4. Correlation matrix between short chain fatty acid (SCFA) concentrations and relative abundance of bacterial genera in cecal digesta of chickens fed diets with increasing levels of deoxynivalenol (DON; 0, 2.5, 5, or 10 mg DON/kg diet) and with or without oral lipopolysaccharide challenge (LPS) 1 day prior to slaughter. Colors refer to the degree of correlation.
Correlations between the abundances of bacterial genera and COG or KEGG pathways were detected for the five most abundant genera and many low abundant genera (Supplementary Figures S1, S2). Especially the high abundant Clostridiales genus 1 and Enterobacteriaceae genus 1 showed opposite relationships with several COG pathways. Clostridiales genus 1 was positively correlated with COG pathways for translation and transcription (r > 0.70). Enterobacteriaceae genus 1, however, was (r < −0.70) negatively correlated with the aforementioned COG pathways. By contrast, Clostridiales genus 1 negatively correlated (r < −0.70) with COG pathways for cellular processes and signaling, metabolism and glycan biosynthesis and metabolism, whereas Enterobacteriaceae genus 1 positively correlated with these pathways. A comparable pattern was observed for correlations between genera and respective KEGG pathways. Clostridiales genus 1 was further highly negatively correlated with the COG pathway for lipid metabolism (r = −0.94). Moreover, positive correlations were also detected for an Enterobacteriaceae genus and glycan biosynthesis and metabolism (r = 0.92) as well as between Turicibacter and the COG pathway for signaling molecules and interaction (r = 0.91).
Discussion
The present results demonstrated that different levels of dietary DON contamination have the potential to alter the cecal bacterial diversity and composition in chickens, which was especially evident within the two predominant phyla Firmicutes and Proteobacteria, modifying the Firmicutes-to-Proteobacteria ratio toward more Firmicutes bacteria. DON-related changes in predicted bacterial metagenomic functions and cecal SCFA concentrations reflected the alterations in the bacterial community composition. Notably, unclassified members of the order Clostridiales benefited from the increasing dietary DON levels, which may have benefited from the opening intestinal niche due to the DON-related decline in the Enterobacteriaceae population. Nevertheless, the decreased bacterial richness and diversity suggested that certain taxa within the bacterial community may have had growth advantages due to the DON exposure which may have lowered the total number and abundance of species. There is some debate whether a reduced bacterial diversity is detrimental for the host or not. Previously, it was thought that high species diversity may reflect a more stable microbiota by preventing the colonization of pathogens (Han et al., 2017). However, a less diverse, but more specialized bacterial community was proposed to use limiting resources more efficiently and promote the energy acquisition of the host (Lozupone et al., 2012; Siegerstetter et al., 2017). Chickens were fed the contaminated diets from the first day of life. Therefore, it is conceivable that DON may have modified the initial bacterial colonization of chicken's gut. Also, chickens receiving the higher DON contamination levels reduced their feed intake (Lucke et al., 2017a), which may partly explain the quadratic effects of dietary DON on the cecal microbiota composition and α-diversity in the present study. The additional oral challenge with a highly immunogenic E. coli-LPS only little modulated the DON effects on the bacterial community. However, the LPS challenge appeared to enhance microbial activity, as indicated by the cecal SCFA concentrations, but only in chickens receiving the non-contaminated diet. This finding may have been related to LPS effects on mucin secretion as well as on digestion and absorption in the small intestine and consequently intestinal nutrient flow (Smirnova et al., 2003; Amador et al., 2007; Mani et al., 2012; Zhang et al., 2017).
Because DON largely impacts the feed intake (Lucke et al., 2017a) as well as digestive, absorptive and immune functions in the small intestine of chickens (Awad et al., 2011b; Ghareeb et al., 2013; Osselaere et al., 2013), DON-effects on the cecal bacterial microbiota were probably rather indirect effects due to changes in cecal nutrient flow and mucus secretion (Antonissen et al., 2015). In line with that, only about 20% of the dietary DON were reported to reach the chicken ceca (Awad et al., 2011a) due to mucosal absorption, sulfation and bacterial de-epoxidization (Gratz et al., 2013; Schwartz-Zimmermann et al., 2015). Bacterial isolates from the chicken intestine, being able to transform DON to deepoxy-deoxynivalenol (DOM-1), were reported to belong to Clostridiales, Anaerofilum, Collinsella, and Bacillus (Yu et al., 2010). In the present study, the dietary exposure to DON specifically promoted the relative abundance of an unclassified Clostridiales genus. This genus was comprised by OTUs which showed 83.4% sequence similarity to proteolytic species within Clostridium sensu strictu. However, due to the high versatility within the order Clostridiales, the low taxonomic resolution at species level rendered it difficult to deduce specific metabolic abilities; particularly, as many members within Clostridiales have been poorly described and the need to re-annotate many Clostridium species within the 16S rRNA gene tree (Stackebrandt et al., 1999; Biddle et al., 2013). Therefore, we can only speculate that changes in the cecal mucin expression or increased protein flow to the ceca may explain the increased abundance of these taxa. In this context, results of DON-effects on the branched-chain fatty acids were less conclusive to indicate whether more or less substrate was available in the cecal lumen for bacterial protein fermentation. Nevertheless, due to the low similarities to cultured strains and the DON-related decrease in the genus Clostridium, it remains speculative which species were affected and whether this increase was related to DON-degrading capabilities, changes in cecal physiology or competition for intestinal niches with other bacteria. Concurrently, we observed a decrease in the relative abundance of the predominant Enterobacteriaceae genus and within it Escherichia/Shigella-OTU2, which is adept at utilizing host mucus as substrate (Zhu and Joerger, 2003; Tenaillon et al., 2010; Conway and Cohen, 2015). Lower cecal mucin production as cause for the reduced Enterobacteriaceae abundance with increasing DON levels was supported by the positive correlation between the unclassified Enterobacteriaceae genus and the COG pathway of glycan biosynthesis and metabolism as well as by the lower abundances of metabolic pathways for glycosaminoglycan degradation and amino acid metabolism after DON exposure. As Enterobacteriaceae, such as E. coli, belong to the commensal intestinal microbiota in chickens but also comprise pathobionts (Dozois et al., 2003; Smati et al., 2015), it is difficult to deduce whether this decrease was beneficial for or disturbed the cecal bacterial homeostasis. By contrast, the lower relative abundance of Proteobacteria, in general, and more specifically of Enterobacteriaceae with increasing dietary DON levels may have lowered the cecal mucosal exposure to LPS of high immune reactivity (Gronbach et al., 2014), as indicated by the lower abundance of the KEGG pathway of LPS biosynthesis proteins. This may have reduced the LPS-related mucosal innate immune response (Yang and Jobin, 2014). DON-related alterations within the Ruminococcaceae and Lachnospiraceae may be due to DON-related changes in intestinal nutrient flow and availability in chickens (Awad et al., 2011b). Moreover, correlation analysis with SCFA and the relative abundance of the KEGG pathway of fatty acid biosynthesis indicated that members of the families Ruminococcaceae and Lachnospiraceae (Flint et al., 2015; Polansky et al., 2015) may have been associated with the observed DON-related changes in cecal SCFA concentrations. In this regard, Ruminococcaceae and Lachnospiraceae, including Blautia, Dorea, and Ruminococcus, are known for their capability to degrade cellulose and hemicelluloses (Biddle et al., 2013). DON may have affected the cross-feeding of primary fermentation metabolites, such as lactate or succinate (Flint et al., 2015), or metabolism of gases among microbes in cecal digesta (Rajilić-Stojanović and De Vos, 2014), which may have modified Ruminococcaceae and Lachnospiraceae abundances as well. Corresponding to our findings, Oscillospira, a predominant genus in chicken cecal microbiota (Wang et al., 2016) has been previously found to decrease in inflammatory diseases in humans (Zhu et al., 2013; Walters et al., 2014; Gophna et al., 2017), whereas Anaerotruncus was positively associated with intestinal inflammatory diseases, such as colorectal cancer (Chen et al., 2012).
Due to its lipid A moiety linked to an antigenic O-polysaccharide (Heinrichs et al., 1998), the currently used LPS from E. coli O55:B5 was a highly immunogenic antigen capable to induce an intestinal inflammatory response after repeated oral application of 250 μg/kg BW in chickens (Wu et al., 2013). The related mucosal immune response may have therefore potentiated the effects of increasing DON levels on gut physiology (e.g., mucin expression) and digestive processes with consequences for microbial colonization in the present study. The latter assumption may explain the higher relative abundance of Clostridiales-OTU24 and the decrease in Ruminococcaceae-OTU67 and OTU93 after the LPS administration across all DON levels. However, the strongest effect of LPS was found for Clostridiales-OTU12 and SCFA concentrations, mainly acetate and butyrate, but only in chickens receiving the 0 DON diet. This may indicate that DON and LPS exposure (e.g., nutrient flow and mucin secretion) may have caused similar environmental conditions for bacterial proliferation and activity in cecal digesta.
In conclusion, the present results demonstrated that the different DON contamination levels of chicken feed substantially modulated the cecal bacterial microbiota composition and decreased the bacterial diversity. DON-related alterations in the bacterial community were especially evident by an increase in an unclassified Clostridiales genus and a decrease in the family Enterobacteriaceae. Changes in host digestive physiology and mucin expression as reason for the altered intestinal bacterial abundances were indicated by DON-related alterations in KEGG pathway abundance genes related to glycoprotein and amino acid metabolism and increased cecal SCFA concentrations. Further studies are needed to clarify which DON-related changes in the cecal microbiota and SCFA were caused by bacterial DON degradation and those which were due to DON-induced changes in host physiology. The additional oral challenge with a highly immunogenic E. coli-LPS showed the strongest effect in chickens that received the diet without DON contamination and mainly responded with an increased cecal SCFA concentration.
Author Contributions
JB and QZ conceived and designed the experiments and revised the manuscript; AL performed the experiments; AL and BM-Z analyzed the data, interpreted the data, and drafted the manuscript. All of the authors read and approved the final manuscript.
Funding
The project was funded by the Austrian Research Promotion Agency (FFG; project number 848446) and Biomin GmbH.
Conflict of Interest Statement
The authors declare that the research was conducted in the absence of any commercial or financial relationships that could be construed as a potential conflict of interest.
Acknowledgments
Parts of the results were presented at the ESVCN Congress 2017, Cirencester, UK (Lucke et al., 2017b). The authors would like to thank Arife Sener, Suchitra Sharma, and Melanie Wild for their skillful technical assistance.
Supplementary Material
The Supplementary Material for this article can be found online at: https://www.frontiersin.org/articles/10.3389/fmicb.2018.00804/full#supplementary-material
References
2006/576/EC (2006). Commission recommendation of 17. August 2006 on the presence of deoxynivalenol, zearalenone, ochratoxin A, T-2 and HT-2 and fumonisins in products intended for animal feeding. Off. J. Eur. Union L229,7–9.
Amador, P., García-Herrera, J., Marca, M. C., De La Osada, J., Acín, S., Navarro, M. A., et al. (2007). Intestinal D-galactose transport in an endotoxemia model in the rabbit. J. Membr. Biol. 215, 125–133. doi: 10.1007/s00232-007-9012-5
Andretta, I., Kipper, M., Lehnen, C. R., Hauschild, L., Vale, M. M., and Lovatto, P. A. (2011). Meta-analytical study of productive and nutritional interactions of mycotoxins in broilers. Poult. Sci. 90, 1934–1940. doi: 10.3382/ps.2011-01470
Antonissen, G., van Immerseel, F., Pasmans, F., Ducatelle, R., Janssens, G. P. J., de Baere, S., et al. (2015). Mycotoxins deoxynivalenol and fumonisins alter the extrinsic component of intestinal barrier in broiler chickens. J. Agric. Food Chem. 63, 10846–10855. doi: 10.1021/acs.jafc.5b04119
Awad, W. A., Hess, M., Twaruzek, M., Grajewski, J., Kosicki, R., Böhm, J., et al. (2011a). The impact of the Fusarium mycotoxin deoxynivalenol on the health and performance of broiler chickens. Int. J. Mol. Sci. 12, 7996–8012. doi: 10.3390/ijms12117996
Awad, W. A., Vahjen, W., Aschenbach, J. R., and Zentek, J. (2011b). A diet naturally contaminated with the Fusarium mycotoxin deoxynivalenol (DON) downregulates gene expression of glucose transporters in the intestine of broiler chickens. Livest. Sci. 140, 72–79. doi: 10.1016/j.livsci.2011.02.014
Awad, W., Ghareeb, K., Böhm, J., and Zentek, J. (2013). The toxicological impacts of the Fusarium mycotoxin, deoxynivalenol, in poultry flocks with special reference to immunotoxicity. Toxins 5, 912–925. doi: 10.3390/toxins5050912
Biddle, A., Stewart, L., Blanchard, J., and Leschine, S. (2013). Untangling the genetic basis of fibrolytic specialization by Lachnospiraceae and Ruminococcaceae in diverse gut communities. Diversity 5, 627–640. doi: 10.3390/d5030627
Chen, W., Liu, F., Ling, Z., Tong, X., and Xiang, C. (2012). Human intestinal lumen and mucosa-associated microbiota in patients with colorectal cancer. PLoS ONE 7:e39743. doi: 10.1371/journal.pone.0039743
Conway, T., and Cohen, P. S. (2015). Commensal and pathogenic Escherichia coli metabolism in the gut. Microbiol. Spectr. 3. doi: 10.1128/microbiolspec.MBP-0006-2014
Cornick, S., Tawiah, A., and Chadee, K. (2015). Roles and regulation of the mucus barrier in the gut. Tissue Barriers 3:e982426. doi: 10.4161/21688370.2014.982426
Dohrman, A., Miyata, S., Gallup, M., Li, J. D., Chapelin, C., Coste, A., et al. (1998). Mucin gene (MUC 2 and MUC 5AC) upregulation by gram-positive and gram-negative bacteria. Biochim. Biophys. Acta 1406, 251–259. doi: 10.1016/S0925-4439(98)00010-6
Dozois, C. M., Daigle, F., and Curtiss, R. III (2003). Identification of pathogen-specific and conserved genes expressed in vivo by an avian pathogenic Escherichia coli strain. Proc. Natl. Acad. Sci. U.S.A. 100, 247–252. doi: 10.1073/pnas.232686799
Edgar, R. C. (2010). Search and clustering orders of magnitude faster than BLAST. Bioinformatics 26, 2460–2461. doi: 10.1093/bioinformatics/btq461
Edgar, R. C., Haas, B. J., Clemente, J. C., Quince, C., and Knight, R. (2011). UCHIME improves sensitivity and speed of chimera detection. Bioinformatics 27, 2194–2200. doi: 10.1093/bioinformatics/btr381
Escrivá, L., Font, G., and Manyes, L. (2015). In vivo toxicity studies of Fusarium mycotoxins in the last decade: a review. Food Chem. Toxicol. 78, 185–206. doi: 10.1016/j.fct.2015.02.005
Flint, H. J., Duncan, S. H., Scott, K. P., and Louis, P. (2015). Links between diet, gut microbiota composition and gut metabolism. Proc. Nutr. Soc. 74, 13–22. doi: 10.1017/S0029665114001463
Ganey, P. E., and Roth, R. A. (2001). Concurrent inflammation as a determinant of susceptibility to toxicity from xenobiotic agents. Toxicology 169, 195–208. doi: 10.1016/S0300-483X(01)00523-6
Ge, Y., Ezzel, R. M., and Warren, H. S. (2000). Localization of endotoxin in the rat intestinal epithelium. J. Infect. Dis. 182, 873–881. doi: 10.1086/315784
Ghareeb, K., Awad, W. A., Böhm, J., and Zebeli, Q. (2015). Impacts of the feed contaminant deoxynivalenol on the intestine of monogastric animals: poultry and swine. J. Appl. Toxicol. 35, 327–337. doi: 10.1002/jat.3083
Ghareeb, K., Awad, W. A., Böhm, J., and Zebeli, Q. (2016). Impact of luminal and systemic endotoxin exposure on gut function, immune response and performance of chickens. World's Poult. Sci. J. 72, 367–380. doi: 10.1017/S0043933916000180
Ghareeb, K., Awad, W. A., Soodoi, C., Sasgary, S., Strasser, A., and Böhm, J. (2013). Effects of feed contaminant deoxynivalenol on plasma cytokines and mRNA expression of immune genes in the intestine of broiler chickens. PLoS ONE 8:e71492. doi: 10.1371/journal.pone.0071492
Gophna, U., Konikoff, T., and Nielsen, H. B. (2017). Oscillospira and related bacteria - from metagenomic species to metabolic features. Environ. Microbiol. 19, 835–841. doi: 10.1111/1462-2920.13658
Gratz, S. W., Dinesh, R., Yoshinari, T., Holtrop, G., Richardson, A. J., Duncan, G., et al. (2017). Masked trichothecene and zearalenone mycotoxins withstand digestion and absorption in the upper GI tract but are efficiently hydrolyzed by human gut microbiota in vitro. Mol. Nutr. Food Res. 61:1600680. doi: 10.1002/mnfr.201600680
Gratz, S. W., Duncan, G., and Richardson, A. J. (2013). The human fecal microbiota metabolizes deoxynivalenol and deoxynivalenol-3-glucoside and may be responsible for urinary deepoxy-deoxynivalenol. Appl. Environ. Microbiol. 79, 1821–1825. doi: 10.1128/AEM.02987-12
Gronbach, K., Flade, I., Holst, O., Lindner, B., Ruscheweyh, H. J., Wittmann, A., et al. (2014). Endotoxicity of lipopolysaccharide as a determinant of T-cell-mediated colitis induction in mice. Gastroenterology 146, 765–775. doi: 10.1053/j.gastro.2013.11.033
Han, Z., Willer, T., Li, L., Pielsticker, C., Rychlik, I., Velge, P., et al. (2017). Influence of the gut microbiota composition on Campylobacter jejuni colonization in chickens. Infect. Immun. 85, e00380–e00317. doi: 10.1128/IAI.00380-17
Heinrichs, D. E., Yethon, J. A., and Whitfield, C. (1998). Molecular basis for structural diversity in the core regions of the lipopolysaccharides of Escherichia coli and Salmonella enterica. Mol. Microbiol. 30, 221–232. doi: 10.1046/j.1365-2958.1998.01063.x
Islam, Z., and Pestka, J. J. (2006). LPS priming potentiates and prolongs proinflammatory cytokine response to the trichothecene deoxynivalenol in the mouse. Toxicol. Appl. Pharmacol. 211, 53–63. doi: 10.1016/j.taap.2005.04.031
Kogut, M. H., and Arsenault, R. J. (2016). Editorial: gut health: the new paradigm in food animal production. Front. Vet. Sci. 3:71. doi: 10.3389/fvets.2016.00071
Langille, M. G., Zaneveld, J., Caporaso, J. G., Mcdonald, D., Knights, D., Reyes, J. A., et al. (2013). Predictive functional profiling of microbial communities using 16S rRNA marker gene sequences. Nat. Biotechnol. 31, 814–821. doi: 10.1038/nbt.2676
Lozupone, C. A., Stombaugh, J. I., Gordon, J. I., Jansson, J. K., and Knight, R. (2012). Diversity, stability and resilience of the human gut microbiota. Nature 489, 220–230. doi: 10.1038/nature11550
Lucke, A., Doupovec, B., Paulsen, P., Zebeli, Q., and Böhm, J. (2017a). Effects of low to moderate levels of deoxynivalenol on feed and water intake, weight gain, and slaughtering traits of broiler chickens. Mycotoxin Res. doi: 10.1007/s12550-017-0284-z
Lucke, A., Metzler-Zebeli, B. U., Zebeli, Q., and Böhm, J. (2017b). “Effects of feeding graded levels of deoxynivalenol and oral administration of lipopolysaccharide on the cecal microbiota of broiler chickens,” in ESVCN Congress (Cirencester).
Magoč, T., and Salzberg, S. L. (2011). FLASH: fast length adjustment of short reads to improve genome assemblies. Bioinformatics 27, 2957–2963. doi: 10.1093/bioinformatics/btr507
Mani, V., Weber, T. E., Baumgard, L. H., and Gabler, N. K. (2012). Growth and development symposium: endotoxin, inflammation, and intestinal function in livestock. J. Anim. Sci. 90, 1452–1465. doi: 10.2527/jas.2011-4627
Martin, M. (2011). Cutadapt removes adaptor sequences from high-throughput sequencing reads. EMBnet J. 17, 10–12. doi: 10.14806/ej.17.1.200
Maslowski, K. M., and Mackay, C. R. (2011). Diet, gut microbiota and immune responses. Nat. Immunol. 12, 5–9. doi: 10.1038/ni0111-5
Metzler-Zebeli, B. U., Lawlor, P. G., Magowan, E., and Zebeli, Q. (2016). Effect of freezing conditions on fecal bacterial composition in pigs. Animals 6. doi: 10.3390/ani6030018
Metzler-Zebeli, B. U., Schmitz-Esser, S., Mann, E., Grull, D., Molnar, T., and Zebeli, Q. (2015). Adaptation of the cecal bacterial microbiome of growing pigs in response to resistant starch type 4. Appl. Environ. Microbiol. 81, 8489–8499. doi: 10.1128/AEM.02756-15
Oakley, B. B., Lillehoj, H. S., Kogut, M. H., Kim, W. K., Maurer, J. J., Pedroso, A., et al. (2014). The chicken gastrointestinal microbiome. FEMS Microbiol. Lett. 360, 100–112. doi: 10.1111/1574-6968.12608
Osselaere, A., Santos, R., Hautekiet, V., De Backer, P., Chiers, K., Ducatelle, R., et al. (2013). Deoxynivalenol impairs hepatic and intestinal gene expression of selected oxidative stress, tight junction and inflammation proteins in broiler chickens, but addition of an adsorbing agent shifts the effects to the distal parts of the small intestine. PLoS ONE 8:e69014. doi: 10.1371/journal.pone.0069014
Piotrowska, M., Slizewska, K., Nowak, A., Zielonka, L., Zakowska, Z., Gajecka, M., et al. (2014). The effect of experimental fusarium mycotoxicosis on microbiota diversity in porcine ascending colon contents. Toxins 6, 2064–2081. doi: 10.3390/toxins6072064
Polansky, O., Sekelova, Z., Faldynova, M., Sebkova, A., Sisak, F., and Rychlik, I. (2015). Important metabolic pathways and biological processes expressed by chicken cecal microbiota. Appl. Environ. Microbiol. 82, 1569–1576. doi: 10.1128/AEM.03473-15
Rajilić-Stojanović, M., and De Vos, W. M. (2014). The first 1000 cultured species of the human gastrointestinal microbiota. FEMS Microbiol. Rev. 38, 996–1047. doi: 10.1111/1574-6976.12075
Robert, H., Payros, D., Pinton, P., Théodorou, V., Mercier-Bonin, M., and Oswald, I. P. (2017). Impact of mycotoxins on the intestine: are mucus and microbiota new targets? J. Toxicol. Environ. Health B 20, 249–275. doi: 10.1080/10937404.2017.1326071
Saadia, R., Schein, M., Macfarlane, C., and Boffard, K. D. (1990). Gut barrier function and the surgeon. Br. J. Surg. 77, 487–492. doi: 10.1002/bjs.1800770505
Saint-Cyr, M. J., Perrin-Guyomard, A., Houée, P., Rolland, J. G., and Laurentie, M. (2013). Evaluation of an oral subchronic exposure of deoxynivalenol on the composition of human gut microbiota in a model of human microbiota-associated rats. PLoS ONE 8:e80578. doi: 10.1371/journal.pone.0080578
Schokker, D., Zhang, J., Vastenhouw, S. A., Heilig, H. G., Smidt, H., Rebel, J. M., et al. (2015). Long-lasting effects of early-life antibiotic treatment and routine animal handling on gut microbiota composition and immune system in pigs. PLoS ONE 10:e0116523. doi: 10.1371/journal.pone.0116523
Schwartz-Zimmermann, H. E., Fruhmann, P., Dänicke, S., Wiesenberger, G., Caha, S., Weber, J., et al. (2015). Metabolism of deoxynivalenol and deepoxy-deoxynivalenol in broiler chickens, pullets, roosters and turkeys. Toxins 7, 4706–4729. doi: 10.3390/toxins7114706
Siegerstetter, S.-C., Schmitz-Esser, S., Magowan, E., Wetzels, S. U., Zebeli, Q., Lawlor, P. G., et al. (2017). Intestinal microbiota profiles associated with low and high residual feed intake in chickens across two geographical locations. PLoS ONE 12:e0187766. doi: 10.1371/journal.pone.0187766
Smati, M., Clermont, O., Bleibtreu, A., Fourreau, F., David, A., Daubie, A. S., et al. (2015). Quantitative analysis of commensal Escherichia coli populations reveals host-specific enterotypes at the intra-species level. MicrobiologyOpen 4, 604–615. doi: 10.1002/mbo3.266
Smirnova, M. G., Guo, L., Birchall, J. P., and Pearson, J. P. (2003). LPS up-regulates mucin and cytokine mRNA expression and stimulates mucin and cytokine secretion in goblet cells. Cell. Immunol. 221, 42–49. doi: 10.1016/S0008-8749(03)00059-5
Stackebrandt, E., Kramer, I., Swiderski, J., and Hippe, H. (1999). Phylogenetic basis for a taxonomic dissection of the genus Clostridium. FEMS Immunol. Med. Microbiol. 24, 253–258. doi: 10.1111/j.1574-695X.1999.tb01291.x
Tenaillon, O., Skurnik, D., Picard, B., and Denamur, E. (2010). The population genetics of commensal Escherichia coli. Nat. Rev. Microbiol. 8, 207–217. doi: 10.1038/nrmicro2298
Waché, Y. J., Valat, C., Postollec, G., Bougeard, S., Burel, C., Oswald, I. P., et al. (2009). Impact of deoxynivalenol on the intestinal microflora of pigs. Int. J. Mol. Sci. 10, 1–17. doi: 10.3390/ijms10010001
Walters, W. A., Xu, Z., and Knight, R. (2014). Meta-analyses of human gut microbes associated with obesity and IBD. FEBS Lett. 588, 4223–4233. doi: 10.1016/j.febslet.2014.09.039
Wang, L., Lilburn, M., and Yu, Z. (2016). Intestinal microbiota of broiler chickens as affected by litter management regimens. Front. Microbiol. 7:593. doi: 10.3389/fmicb.2016.00593
Wei, T., and Simko, V. (2016). Corrplot: Visualization of a Correlation Matrix. R package version 0.77. Avaiable online at: https://CRAN.R-project.org/package=corrplot
Wu, Q. J., Zhou, Y. M., Wu, Y. N., Zhang, L. L., and Wang, T. (2013). The effects of natural and modified clinoptilolite on intestinal barrier function and immune response to LPS in broiler chickens. Vet. Immunol. Immunopathol. 153, 70–76. doi: 10.1016/j.vetimm.2013.02.006
Yang, Y., and Jobin, C. (2014). Microbial imbalance and intestinal pathologies: connections and contributions. Dis. Model. Mech. 7, 1131–1142. doi: 10.1242/dmm.016428
Yu, H., Zhou, T., Gong, J., Young, C., Su, X., Li, X. Z., et al. (2010). Isolation of deoxynivalenol-transforming bacteria from the chicken intestines using the approach of PCR-DGGE guided microbial selection. BMC Microbiol. 10:182. doi: 10.1186/1471-2180-10-182
Zhang, Q., Eicher, S. D., Ajuwon, K. M., and Applegate, T. J. (2017). Development of a chicken ileal explant culture model for measurement of gut inflammation induced by lipopolysaccharide. Poult. Sci. 96, 3096–3103. doi: 10.3382/ps/pex160
Zhou, H. R., Harkema, J. R., Hotchkiss, J. A., Yan, D., Roth, R. A., and Pestka, J. J. (2000). Lipopolysaccharide and the trichothecene vomitoxin (deoxynivalenol) synergistically induce apoptosis in murine lymphoid organs. Toxicol. Sci. 53, 253–263. doi: 10.1093/toxsci/53.2.253
Zhu, L., Baker, S. S., Gill, C., Liu, W., Alkhouri, R., Baker, R. D., et al. (2013). Characterization of gut microbiomes in nonalcoholic steatohepatitis (NASH) patients: a connection between endogenous alcohol and NASH. Hepatology 57, 601–609. doi: 10.1002/hep.26093
Keywords: broiler, 16S RNA sequencing, cecum, deoxynivalenol, lipopolysaccharide, microbiota
Citation: Lucke A, Böhm J, Zebeli Q and Metzler-Zebeli BU (2018) Dietary Deoxynivalenol Contamination and Oral Lipopolysaccharide Challenge Alters the Cecal Microbiota of Broiler Chickens. Front. Microbiol. 9:804. doi: 10.3389/fmicb.2018.00804
Received: 29 November 2017; Accepted: 10 April 2018;
Published: 25 April 2018.
Edited by:
Emilio M. Ungerfeld, Instituto de Investigaciones Agropecuarias (INIA), ChileReviewed by:
Robert J. Moore, RMIT University, AustraliaKyung-Woo Lee, Konkuk University, South Korea
Copyright © 2018 Lucke, Böhm, Zebeli and Metzler-Zebeli. This is an open-access article distributed under the terms of the Creative Commons Attribution License (CC BY). The use, distribution or reproduction in other forums is permitted, provided the original author(s) and the copyright owner are credited and that the original publication in this journal is cited, in accordance with accepted academic practice. No use, distribution or reproduction is permitted which does not comply with these terms.
*Correspondence: Barbara U. Metzler-Zebeli, QmFyYmFyYS5NZXR6bGVyQHZldG1lZHVuaS5hYy5hdA==