- Department of Chemical Engineering, Tokyo University of Agriculture and Technology, Koganei, Japan
Nitrous oxide (N2O)-reducing bacteria, which reduce N2O to nitrogen in the absence of oxygen, are phylogenetically spread throughout various taxa and have a potential role as N2O sinks in the environment. However, research on their physiological traits has been limited. In particular, their activities under microaerophilic and aerobic conditions, which severely inhibit N2O reduction, remain poorly understood. We used an O2 and N2O micro-respirometric system to compare the N2O reduction kinetics of four strains, i.e., two strains of an Azospira sp., harboring clade II type nosZ, and Pseudomonas stutzeri and Paracoccus denitrificans, harboring clade I type nosZ, in the presence and absence of oxygen. In the absence of oxygen, the highest N2O-reducing activity, Vm,N2O, was 5.80 ± 1.78 × 10−3 pmol/h/cell of Azospira sp. I13, and the highest and lowest half-saturation constants were 34.8 ± 10.2 μM for Pa. denitirificans and 0.866 ± 0.29 μM for Azospira sp. I09. Only Azospira sp. I09 showed N2O-reducing activity under microaerophilic conditions at oxygen concentrations below 110 μM, although the activity was low (10% of Vm,N2O). This trait is represented by the higher O2 inhibition coefficient than those of the other strains. The activation rates of N2O reductase, which describe the resilience of the N2O reduction activity after O2 exposure, differ for the two strains of Azospira sp. (0.319 ± 0.028 h−1 for strain I09 and 0.397 ± 0.064 h−1 for strain I13) and Ps. stutzeri (0.200 ± 0.013 h−1), suggesting that Azospira sp. has a potential for rapid recovery of N2O reduction and tolerance against O2 inhibition. These physiological characteristics of Azospira sp. can be of promise for mitigation of N2O emission in industrial applications.
Introduction
Nitrous oxide (N2O) is an ozone-depleting and greenhouse gas (Ravishankara et al., 2009), therefore it is important to decrease N2O emissions from natural ecosystems, agriculture, and industrial systems. A large fraction of N2O is emitted from agricultural croplands (IPCC, 2013; Harter et al., 2014). Emissions from industrial systems, mainly wastewater treatment plants, have become more significant as a result of upgrading of biological nitrogen removal processes, i.e., nitrification–denitrification or partial nitrification–anammox processes (Law et al., 2012). N2O is produced via multiple biological and abiotic pathways, e.g., in denitrification as an intermediate (Philippot et al., 2011; Wunderlin et al., 2011; Ishii et al., 2014), in the nitrifier denitrification of ammonia-oxidizing microorganisms (Zhu et al., 2013; Ali et al., 2016), and in chemical oxidation of hydroxylamine (Soler-Jofra et al., 2016; Terada et al., 2017). It is consumed mainly by denitrifying bacteria harboring a N2O reductase system (Nos) (Henry et al., 2006; Zumft and Kroneck, 2006; Jones et al., 2008; Pauleta et al., 2013). In subsequent denitrification steps, N2O reduction is severely affected by environmental factors, i.e., pH, availability of electron donors, and dissolved oxygen (DO) (Law et al., 2012; Pan et al., 2013). Although the physiological traits on N2O reduction by canonical denitrifying species, i.e., the genera Pseudomonas and Paracoccus (Zumft, 1997; Vollack and Zumft, 2001; Philippot, 2002; Read-Daily et al., 2016), have been studied to date, knowledge is still limited. Comprehensive and thorough physiological research on N2O-reducing bacteria under various environmental conditions is therefore warranted.
Recent metagenomic analyses have shown that N2O-reducing bacteria that harbor Nos can be classified into two clade types: clade I and clade II (Sanford et al., 2012; Jones et al., 2013). It has been reported that the abundances of the two clades potentially depend on environmental conditions, e.g., pH, concentration of calcium ion, and C/N ratio, and niche differentiation probably occurs because of their physiological characteristics (Jones et al., 2014; Domeignoz-Horta et al., 2015; Juhanson et al., 2017). Some clade II type N2O-reducing bacteria reportedly have lower half-saturation constants for N2O than do those affiliated to clade I type bacteria, suggesting that a low N2O concentration favors growth of clade II type N2O-reducing bacteria (Yoon et al., 2016). Additionally, most non-denitrifying N2O-reducing bacteria, which are unable to reduce nitrite and nitrate, are clade II type (Sanford et al., 2012; Hallin et al., 2018). Given these traits of clade II type bacteria, reports suggest that they potentially play an important role as N2O sinks (Jones et al., 2014; Orellana et al., 2014).
Studies of the oxygen effects are of fundamental and engineering importance. The DO concentration determines N2O emissions from soils and wastewater treatment plants via mainly nitrifier–denitrification and heterotrophic denitrification (Tallec et al., 2006; Morley et al., 2008; Riya et al., 2012). Studies on gene transcription and enzyme expression have suggested that Nos expression is regulated by the DO concentration (Körner and Zumft, 1989; Bergaust et al., 2012) and, more importantly, that the Nos enzyme is inactivated by oxygen (Pauleta et al., 2013). In addition, the susceptibility of N2O-reducing activity to the oxygen concentration is distinct at species or strain levels, as reported for the genera Thauera and Pseudomonas (Miyahara et al., 2010; Liu et al., 2013; Zheng et al., 2014). However, the influence of O2 on N2O reduction by clade II type N2O-reducing bacteria has not been comprehensively studied, except Gemmatimonas aurantiaca strain T-27 (Park et al., 2017). To enable their use in engineering applications as N2O sinks, the effects of oxygen on the N2O reduction activities of clade I and II type N2O-reducing bacteria need to be compared based on biokinetic analysis.
In this study, we, for the first time, compared the N2O reduction kinetics of clade I and clade II type N2O-reducing bacteria in the presence and absence of oxygen. Pseudomonas stutzeri and Paracoccus denitrificans, harboring nosZ clade I type, and two strains of Azospira sp., isolated from a N2O-fed enrichment device (unpublished data) inoculated with municipal wastewater treatment biomass, were subject to biokinetic comparison.
Materials and Methods
Bacterial Strains and Culture Conditions
Two Azospira sp. strains and two strains of canonical denitrifiers, i.e., Ps. stuzeri strain JCM5965 (ATCC17588) and Pa. denitrificans strain NBRC102528 (ATCC17741), were used in this study. Azospira sp. strains I09 and I13, classified as Betaproteobacteria, were isolated from enrichment devices supplying N2O as an electron acceptor (unpublished data). Ps. stutzeri and Pa. denitrificans were chosen because they are widely used as canonical denitrifiers (Körner et al., 1987; Baumann et al., 1996; Miyahara et al., 2010; Black et al., 2016). For comparison with other biokinetic studies, weight of a bacterial cell was determined as 0.388, 0.604, 0.743, and 0.787 pg-dry weight/cell for Azospira sp. strain I09, I13, Ps. stutzeri and Pa. denitrificans, respectively.
All the strains were aerobically pre-grown in an autoclaved nutrient medium containing (per liter of distilled water) 5.0 g of Bacto Peptone (BD-Difco, NJ), 3.0 g of Oxoid™ Lab-Lemco meat extract (Thermo Scientific, MA), and 5.0 g of NaCl.
Chemical Analyses
Dissolved organic carbon and dissolved total nitrogen were determined with a TOC analyzer, installing a total nitrogen measurement unit (5000A, Shimadzu, Kyoto, Japan). pH was measured using a pH meter (F-52, HORIBA, Kyoto, Japan). The gaseous N2O concentration was determined using a gas chromatograph with an electron-capture detector (GC-14B, Shimadzu, Kyoto, Japan) instrument. The measurement conditions were described in a previous paper (Terada et al., 2013).
Activity Measurements
The pre-grown bacterial strains were harvested in centrifuge tubes at the early stationary phase. Two strains of Azospira spp. (I09 and I13) were washed twice with 0.05 × phosphate-buffered saline (PBS) by centrifugation at 5,000 rpm for 5 min, and re-suspended in the experimental medium. Our preliminary experiment showed that the N2O-reducing activities of Ps. stutzeri JCM5965 and Pa. denitrificans NBRC102528 were significantly reduced by washing by centrifugation (data not shown). The pre-incubated cell suspensions of these two strains were therefore diluted with the experimental medium instead of washing. The experimental medium was placed in a conical flask, and the flask was sealed with a silicone cap (Shin-Etsu Polymer Co., Tokyo, Japan). The medium was mixed by shaking at 100 rpm at 30°C. The phosphate buffer medium, devoid of electron donors, contained (per liter of distilled water) 100 mg of KH2PO4, 6.6 mg of NaCl, 8.20 mg of MgSO4·7H2O, 13.4 mg of KCl, 115 mg of NH4Cl, 188 mg of NaHCO3, and 1 mL of a trace element solution consisting of (per liter of distilled water) 10 g of FeSO4 7H2O, 10 g of FeCl3 7H2O, 2 g of ZnSO4 7H2O, 4 g of CuSO4 7H2O, 0.5 g of NaMoO4 2H2O, 0.1 g of MnCl2 4H2O, 0.1 g of H3BO4, 0.3 g of Na2SeO3, and 10 g of citric acid (Miyahara et al., 2010). After sterilization, pH of the medium was adjusted to 7.5 with 1 M HCl.
The O2 and N2O consumption behaviors were investigated using an O2 and N2O micro-respiration system with amperometric microsensors (Unisense, Aarhus, Denmark). This system consisted of a Clark-type N2O sensor (N2O-MR), O2 sensor (OX-MR), double-port chamber (10 mL, MR-Ch double port), and stirrers installed in a sensor stand (Figure 1). The temperature during the experiments was controlled at 30 ± 0.2°C by a water bath. The experimental sequences of O2 and N2O consumption are shown in Figure 1B. The chamber was filled with the cell suspension, in which electrodes were inserted. Highly concentrated N2O water (27.05–24.09 mM at 20–25°C and 0% salinity Weiss and Price, 1980) was prepared by pouring 25 mL of deionized water in a 50 mL vial, supplying pure N2O gas to the water for 10 min at a room temperature and subsequently sealing the vial with a butyl rubber stopper. The highly concentrated N2O water with a volume of 15–20 μL was injected into the chamber from the injection port (Figure 1A) using a Hamilton syringe, to replace the bacterial cell suspension and to give an initial dissolved N2O concentration of approximately 50 μM. Then 250 mM sodium acetate solution (25 μL) was added to adjust the initial concentration to 625 μM. To achieve a homogeneous condition immediately, the glass-coated stir bar, was stirred at 600 rpm for 5 min after N2O and carbon source injection and then at 300 rpm during the measurements. O2 and N2O concentrations were continuously monitored using SensorTrace Suite ver.2.8.0 (Unisense, Aarhus, Denmark). After the N2O was completely consumed, highly concentrated N2O water was injected again. The number of N2O injections was changed depending on the distinct trends in the N2O reduction rates observed among the tested strains. After the measurements, a sample of the cell suspension was immediately taken from the chamber and fixed with a 2% glutaraldehyde solution to determine the cell number, as previously described (Lunau et al., 2005). Briefly, the cell-counting procedure was as follows. Ethanol was added to the fixed cell suspension to achieve a final concentration of 10%. The mixture was homogenized for 10 s at 10 W (VP-050, Taitec, Tokyo, Japan), followed by dilution with 0.05 × PBS. The cells were trapped on a 0.2 μm membrane filter (Isopore, Merck Millipore, Germany), and washed twice with 1 mL of sterilized Tris-acetate EDTA buffer. The cells on the filter were stained with Moviol-SYBR Green I (Thermo Fisher Scientific, MA) and enumerated under a fluorescence microscope (BZ-8100, Keyence, Osaka, Japan).
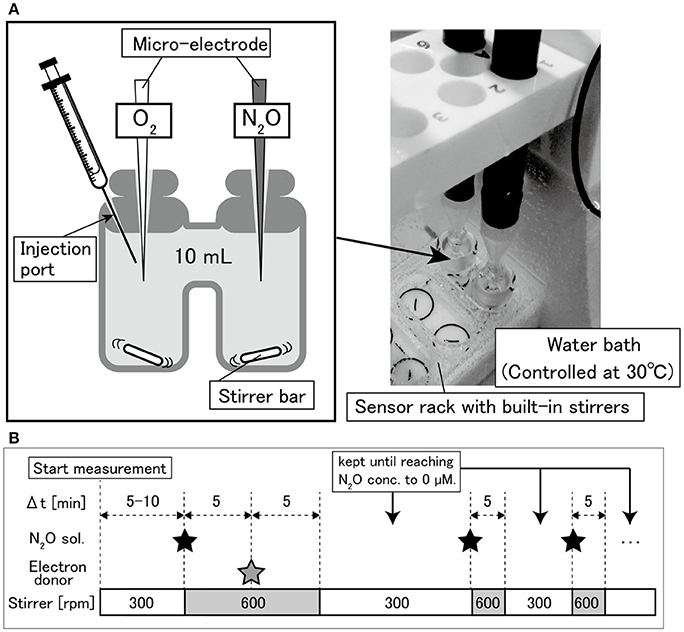
Figure 1. (A) Diagram of double-port chamber and (B) experimental sequence of N2O and O2 respiration measurements.
Kinetic Parameter Estimation
The O2 and N2O concentration profiles were smoothed with the function of Sigma Plot 13.0 (Systat software, CA) to remove high-frequency noise and instantaneous reduction rate (Δ = 3–5 s) was calculated and normalized with cell number (Vs [pmol/h/cell]). The maximum O2 and N2O uptake rates (Vm,O2 and Vm,N2O [pmol/h/cell]) and the half-saturation constants for O2 and N2O (Km,O2 and Km,N2O [μM]) were determined by fitting the profiles to the Michaelis–Menten equation (Equation 1) (Martens-Habbena et al., 2009). The Vm,N2O value was calculated from the last measurement of the N2O profiles.
where S [μM] is the concentration of either O2 or N2O. To compare the N2O reduction activities among the strains, the specific affinity for N2O (a0,N2O [L/cell/h]) was calculated as follows (Equation 2):
Statistical analysis was performed with ANOVA (Tukey HSD) in SPSS Statistics (IBM, NY), and statistical significance was evaluated by p-value below 0.05 as a threshold.
The effect of O2 on the N2O-reducing activity was investigated by fitting the experimental profiles to a mathematical model. The heterotrophic denitrification model, incorporating the O2 inhibition (Ni et al., 2011), was used to estimate the degree of O2 inhibition to N2O uptake rates. The terms of an electron donor, nitric oxide (NO) and ammonium () were excluded in the model proposed by Ni et al. (2011) (Equation 3) because theoretically NO is not produced and organic carbon and were in excess in the medium (i.e., addition of , organic carbon, and N2O under anoxic conditions).
where X [cells/L] is the concentration of bacterial cells in the chamber, and KI,O2 [μM] is the O2 inhibition coefficient. For Azospira sp. strains I09, I13, and Ps. stutzeri, KI,O2 values were estimated by fitting the 1st-spiked N2O profile to the model based on the least-squares method using the solver function of Microsoft Excel ver. 15.26. For Pa. denitrificans, determination of KI,O2 was not feasible because the trend of N2O consumption is not explainable by the model. The lowest KI,O2 value detected in this study was 0.1 μM due to the detection limit of an O2 microelectrode.
The relative activity of N2O reduction rate E [dimensionless] under an anoxic condition was calculated by Equation (4).
VN2O was attained from an N2O profile with the concentration range from 10 to 40 μM under an anoxic condition. The Nos activation rate (VNos [h−1]) was defined as a degree of activity recovery of N2O reduction after changing an aerobic to anoxic condition. VNos value was acquired by linear approximation of E as a function of elapsed time after DO concentration becomes zero (tanoxic). The analysis was performed with Sigma Plot 13.0.
Quantifying Transcripts of nosZ Gene
Dynamics of nosZ gene transcripts of Azospira sp. strain I09 were quantified by reverse-transcription quantitative PCR (RT-qPCR). A medium, adding (per liter of distilled water) 0.20 g of sodium acetate and 0.050 g of NH4Cl to the synthetic medium for the respirometric test, was autoclaved, followed by pH adjustment at 7.5 with 1 M HCl. Azospira sp. strain I09 was inoculated and aerobically pre-grown at 30°C in a 500 mL bottle until the late-exponential growth phase (OD600 = 0.121 after incubation for 23 h). After the aerobic pre-growth, different gases were supplied via a sterilized filter (HEPA-VENT, GE Healthcare, UK) at three different phases: 1 L/min of air in Phase 1, 0.5 L/min of N2 in Phase 2, and 0.5 L/min of 100 ppm N2O/N2 in Phase 3. The gas flow rate was controlled using a mass flow controller (HORIBA, Kyoto, Japan). DO concentration in the medium was monitored using a DO meter (FDO Multi3410, WTW, Weilheim in Oberbayern, Germany). At each sampling point, cell suspension (10 mL) was transferred to a 15 mL tube and centrifuged at 10,000 rpm for 5 min at 4°C, and the supernatant was subsequently decanted. One milliliter of RNApro solution (FastRNA Pro Blue Kit, MP Biomedicals, CA) as a retardant of RNA degradation was immediately added to completely re-suspend the pellet according to the manufacture's protocol, followed by storage at 4°C until RNA extraction. RNA was extracted with a FastRNA Pro Blue Kit (MP Biomedicals, CA) and quantified by a UV-Vis spectrophotometer (Nanodrop 2000c, Thermo Fisher Scientific, MA). The extracted RNA was reverse-transcribed to complementary DNA with a QuantiTect Reverse Transcription Kit (QIAGEN, Hilden, Germany). The clade II type nosZ and 16S rRNA genes were quantified by real-time qPCR using a CFX96 Real-Time PCR Detection System (BioRad Laboratories, CA). The primer sets for clade II type nosZ and 16S rRNA genes were nosZ-II-F (5′-CTIGGICCIYTKCAYAC-3′)—nosZ-II-R (5′-GCIGARCARAAITCBGTRC-3′) (Jones et al., 2013) and 341f (5′-CCTACGGGAGGCAGCAG-3′)−517r (5′-ATTACCGCGGCTGCTGG-3′) (Muyzer et al., 1993), respectively. PCR for 16S rRNA gene amplification was initiated with initial denaturation at 95°C for 2 min, followed by 40 cycles at 95°C for 30 s, 60°C for 30 s, and 72°C for 30 s. PCR for the clade II type nosZ gene was initiated with denaturation at 95°C for 1 min, followed by 50 cycles at 95°C for 15 s, 54°C for 30 s, 72°C for 30 s, and 80°C for 30 s. The reaction buffer for the 16S rRNA gene consisted of 10 μL of SsoFast™ EvaGreen® Supermix (BioRad Laboratories, Hercules, CA), 1 μL of 10 mM forward and reverse primers, 5 μL of template, and 3 μL of distilled water. The PCR buffer for the clade II type nosZ gene consisted of 10 μL of SYBR Premix Ex Taq II (Tli RNaseH Plus, Takara Bio, Shiga, Japan), 2 μL of 10 mM forward and reverse primers, 1 μL of distilled water, and 5 μL of template. Plasmid DNA (pGEM-T Easy Vector, Promega, WI) containing each gene was transformed into Escherichia coli competent cells (Competent High DH5α, Toyobo, Osaka, Japan) and the plasmids were isolated for each standard solution with a plasmid extraction kit (MagExtractor -Plasmid-, Toyobo, Osaka, Japan). After confirmation of insertion of each functional gene by Sp6 and T7 primers (Huang et al., 2005), the plasmid DNA as a positive control was diluted to obtain standard solutions containing 1.0 × 108 to 1.0 × 102 copies per 5 μL in series. To increase the PCR efficiency, the plasmid containing the nosZ clade II amplicon was linearized with the restriction enzyme EcoRI (Takara bio, Kyoto, Japan) and used as a standard solution. The gene transcripts of nosZ were normalized with the amount of total RNA and gene transcripts of 16S rRNA to trace dynamics of nosZ gene expression. The transcripts of 16S rRNA gene was in the same order of magnitude during the tested three phases (Data not shown).
Results
Activity Measurements and Biokinetic Comparison
Activity measurement of each strain was conducted in triplicate. The representative O2 and N2O concentrations profiles are shown in Figure 2 and the two other replicates in SI (Figures S1–S4). All the bacterial strains showed facultative N2O-reducing activities, consuming O2 prior to N2O. However, the O2 concentration at which N2O-reducing activity was initiated was not consistent for each strain. Except in the case of Pa. denitrificans, the first-spiked N2O was completely consumed, followed by injection of N2O-concentrated liquid. For Azospira sp. strains I09 and I13, the maximum N2O reduction rate reached a plateau at the second or third additional N2O injection. The maximum N2O reduction rate of Azospira sp. I09 after the second injection was normalized to 114 ± 8% (n = 3) higher than that after the first injection. The analogous trend was attained for Azospira sp. I13, displaying that the maximum N2O reduction rates after the second and third injections were 132 ± 14% (n = 3) and 132 ± 8% (n = 2) of the rate after the first one, respectively. For Ps. stutzeri, the maximum N2O reduction rates after the additional injections further increased: 224 ± 69% (n = 3) for the second, 267 ± 62% (n = 3) for the third, 280 ± 40% (n = 2) for the fourth, and 294 ± 29% (n = 2) for the fifth, higher than the rate after the first injection. Pa. denitrificans was spiked with N2O once because the reduction rate slowed down below 50 μM N2O, entailing 10 h to consume the first-spiked N2O (Figure S4). The initial N2O concentration hampered accurate measurement for the N2O reduction activity of Pa. denitrificans; therefore, the volume of injected N2O solution was adjusted to ensure a higher N2O concentration (100–150 μM) (Figure 2D and Figure S4). The maximum N2O uptake rate (Vm,N2O) and half saturation constant for N2O (Km,N2O) were estimated by fitting the Michaelis–Menten equation to the N2O profile at the final injection in each run (Table 1). Azospira sp. I13 showed the highest Vm,N2O, i.e., 5.80 ± 1.78 × 10−3 pmol/h/cell, among all the strains, followed by Ps. stutzeri (1.64 ± 0.34 × 10−3 pmol/h/cell), Azospira sp. I09 (6.34 ± 0.8 × 10−4 pmol/h/cell), and Pa. denitrificans (5.01 ± 1.0 × 10−4 pmol/h/cell). The highest and lowest Km,N2O values were 34.8 ± 10.2 μM for Pa. denitirificans and 0.866 ± 0.29 μM for Azospira sp. I09, respectively. The other two strains displayed comparable Km,N2O values of about 4 μM. The N2O affinities (a0,N2O) of Azospira sp. strains I13 and I09 were higher than those of Ps. stutzeri and Pa. denitirificans because of the inherently higher Vm,N2O (I13) or lower Km,N2O (I09).
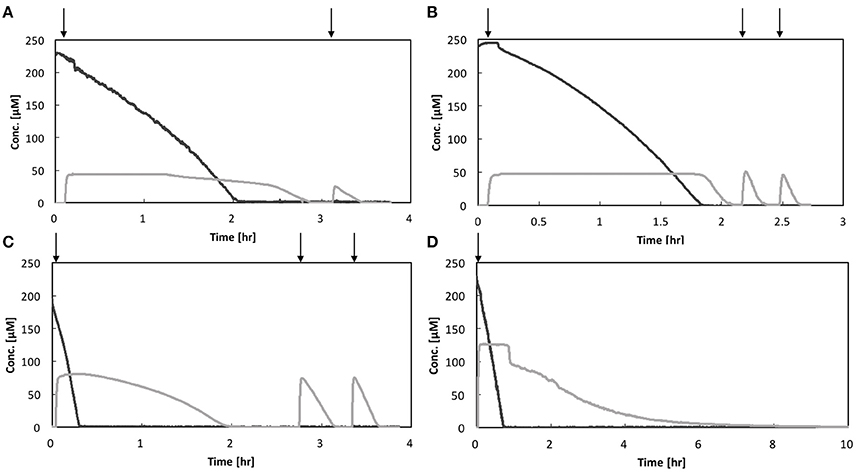
Figure 2. N2O and O2 respiration profiles of isolates: (A) Azospira sp. strain I09, (B) Azospira sp. strain I13, (C) Pseudomonas stutzeri JCM5965, and (D) Paracoccus denitrificans strain NBRC102528. Black line: O2, gray line: N2O. Vertical arrows indicate N2O spikes.
Effects of O2 on N2O Reduction
The effects of O2 on N2O reduction were compared on the basis of the relative activities of N2O reduction, E (Equation 4). E as a function of O2 concentration is shown in Figure 3. The effect of the DO concentration on E differed among the tested strains. Although the activity was lower (10% of Vm,N2O) than that in the absence of oxygen, Azospira sp. I09 showed N2O consumption activity under microaerophilic conditions at DO concentrations of 100–110 μM. In contrast, the DO concentration needed to initiate N2O consumption by Azospira sp. I13 and Pa. denitrificans was much lower (25 μM O2). The N2O consumptions of these three strains recovered significantly after complete consumption of O2. Ps. stutzeri did not consume N2O until the O2 was completely depleted.
The time series for the N2O relative reduction rates under anoxic conditions are shown in Figure 4. Pa. denitrificans was excluded from the analysis because the inherently low N2O affinity of Pa. denitrificans hampered accurate measurement of the N2O reduction rate (Table 1). Relative activity of N2O reduction rate increased linearly in the three strains. The two Azospira sp. strains showed the same trend in E-values, and regained 0.8 of the initial value in 1.93 h (I09) and 1.38 h (I13), respectively. In contrast, Ps. stutzeri required 4.32 h for 80% recovery of E (Figure 4). The Nos activation rates (VNos), i.e., the slopes in Figure 4, were estimated to be 0.319 ± 0.028, 0.397 ± 0.064, and 0.200 ± 0.013 h−1 for Azospira sp. strain I09, I13, and Ps. stutzeri, respectively, indicating the highest VNos value for Azospira sp. strain I13.
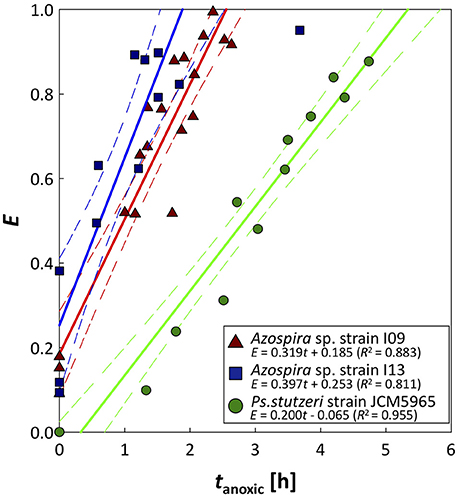
Figure 4. Recovery of N2O reduction activity after O2 exposure. Plots are derived from N2O consumption tests, performed in duplicate or triplicate: tanoxic = 0 is elapsed time after DO is completely depleted. Solid and broken lines represent approximated activity recovery for N2O reduction in each strain and the 95% confidence interval of each approximated line, respectively. For the approximation, triplicate and duplicate data were used for the two Azospira sp. strains and Ps. stutzeri, respectively.
The effect of O2 on the N2O reduction activity was quantitatively compared by determination of biokinetic parameters (Figure S5). KI,O2 was estimated by the model fitting for N2O profiles (Figure S5). Azospira sp. strain I09 showed the highest KI,O2 value of 2.33 ± 1.7 μM while those of Azospira sp. strain I13 and Ps. stutzeri were below 0.5 μM as summarized in Table 1.
nosZ Gene Transcription of Azospira sp. Strain I09
Dynamics of nosZ transcription of Azospira sp. strain I09, displaying N2O uptake even at above 100 μM O2, was monitored under different redox conditions (Figure 5). During Phase 1 for aerobic pre-incubation by air bubbling, nosZ transcription level slightly increased. Switching to the anoxic condition by pure N2 bubbling decreased the nosZ transcription level after 40 min by 74%. Subsequently, mixing N2O with N2 gas to keep N2O concentration of 100 ppmv (equivalent dissolved N2O of 2.16 μM at 30°C) in Phase 3 stimulated nosZ transcription, reaching the comparable level in Phase 1.
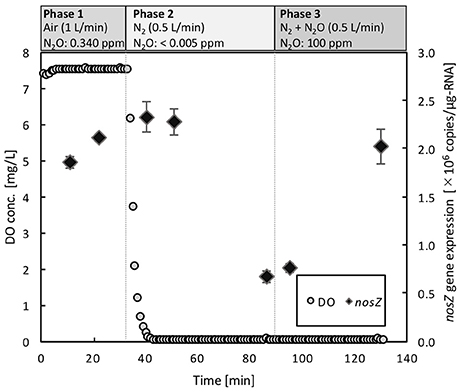
Figure 5. Dynamics of nosZ gene expression in Azospira sp. I09 under three different redox conditions. The gaseous compositions were ambient air (N2O: 0.34 ppm) in Phase 1, N2 (N2O: <0.005 ppm) in Phase 2, and N2 + N2O (N2O: 100 ppm) in Phase 3, respectively. The gas flow rate was consistently 0.5 L/min.
Discussion
This study, for the first time, showed the effects of oxygen on the N2O reduction activities of nosZ clade I and II type N2O-reducing bacteria in a comparative manner. Monitoring N2O consumption dynamics has always been challenging because of the difficulty in setting up conditions under which the N2O and O2 concentrations are always transitioning. A common batch experiment, measuring the headspace gas in a vial (Bueno et al., 2015), tackled the difficulty of monitoring N2O and O2 concentrations within short intervals. The micro-respirometric system using DO and N2O microsensors used in this study enabled real-time monitoring of these dissolved gases at a much higher resolution (second level) than in conventional methods. The highly resolved profiles detect N2O-reducing activity even under aerobic conditions. We found that physiological characteristics do not clearly distinguish clade I and II type N2O-reducing bacteria.
There has been open discussion on whether clade II type N2O-reducing bacteria have inherently high N2O affinities compared with those of their clade I type counterparts (Yoon et al., 2016; Hallin et al., 2018). Current research suggested the existence of unique electron transport module in clade II type microorganisms, i.e., Wolinella succinogenes (Hein et al., 2017). The feature is advantageous for low-energy translocation pathways and effective energy conversion within the N2O respiration compared to nosZ clade I type bacteria. Our biokinetic analyses of the clade II type N2O-reducing bacterium, i.e., two Azospira sp. strains, show that their half-saturation constants (Km,N2O) are lower than those of the two clade I type denitrifying bacteria (Table 1). This is in agreement with previous studies, in which clade II type strains, namely Dechloromonas aromatica strain RCB and Anaeromyxobacter dehalogenans strain 2CP-C, gave lower Km,N2O and higher biomass yields than did clade I type strains (Yoon et al., 2016). Further comparison, with the substrate affinity a0,N2O as an indicator of N2O-reducing activity, shows that clade II type Azospira sp. strain I13 is a promising N2O sink (Table 1). However, even for the same Azospira sp., the two isolates had distinct a0,N2O values. Furthermore, the recent report demonstrated that a chemostat system, supplying N2O as a sole electron acceptor, enriched clade I type N2O reducing bacteria with high a0,N2O (Conthe et al., 2018). Given that taxonomic proximity is not necessarily linked to physiology, N2O-reducing bacteria having very high affinity to N2O could be broadly distributed irrespective nosZ clade type. Comprehensive biokinetic analysis focusing on not only clade II type but also clade I type is needed.
The N2O reduction activities in the presence of O2 differed among the four bacterial strains, irrespective of their nosZ clade types. Azospira sp. strain I09 exhibited N2O-reducing activity at a DO concentration of 110 μM (3.52 mg/L) and higher O2 inhibition coefficient (2.33 μM), confirming the participation of aerobic N2O reduction. Previous physiological studies using isolates or mixed cultures implied that some bacteria are capable of denitrifying and respiring N2O under aerobic or microaerobic conditions, but the level of DO concentration needed for detectable N2O-reducing activity depends on the bacterial strain (Liu et al., 2013). Thauera sp. strain 63 expresses nosZ mRNA under microaerobic conditions (<10 μM), although T. terpenica strain DSM12139 does so under obligate anaerobic conditions (Liu et al., 2013). The Nos of Ps. stutzeri strain ATCC14405 is expressed at a DO concentration of 119 μM (Körner and Zumft, 1989) and Ps. stutzeri strain TR2 showed N2O-reducing activity at a DO concentration of 35 μM (Miyahara et al., 2010). In a mixed culture, reduction activity by microorganisms in soils appears at a DO concentration of 80 μM (Morley et al., 2008), and denitrification gene transcription is continuously detected under O2-remaining conditions in coastal sediments (Marchant et al., 2017). On the basis of previous reports, the DO level for the emergence of N2O consumption by Azospira sp. strain I09 (110 μM) is higher than those of Thauera sp. strain 63 and Ps. stutzeri strain TR2, and comparable to that of Ps. stutzeri strain ATCC14405. Thorough, holistic investigations are needed, but our results suggest that Azospira sp. strain I09 has promise as a N2O consumer under microaerophilic conditions.
The activity tolerance of O2 inhibition was reasonably described applying the Nos activation rate, VNos (Figure 4). The higher values of VNos for the Azospira sp. strains (0.319 ± 0.028h−1 for I09 and 0.397 ± 0.064 h−1 for I13) than that for Ps. stutzeri (0.200 ± 0.013 h−1) indicate that the recovery of N2O reduction activity by the Azospira sp. strains is faster than that by Ps. stutzeri. In addition, E-values for the Azospira sp. strains higher than 0 at tanoxic = 0 suggests their superior N2O uptake resilience to O2 exposure, which was not observed for Ps. stutzeri (Figure 4). In previous reports, two mechanisms of O2 inhibition to N2O reduction activity have been hypothesized, namely regulation of the RNA transcription level (Arai, 2011; Bergaust et al., 2012) and Nos inactivation (Otte et al., 1996; Miyahara et al., 2010). The quantification of nosZ clade II type mRNA transcripts for Azospira sp. strain I09 under different redox conditions likely provided Nos reactivation as a limiting factor to determine N2O uptake rate. The application of RT-qPCR demonstrated that expression of the nosZ mRNA by Azospira sp. strain I09 was suppressed in the absence of O2 and N2O but not in the presence of N2O and O2 (Phase 1 and Phase 3 in Figure 5). This agrees with the trend that nosZ gene is expressed even in the presence of O2 in coastal sediments (Marchant et al., 2017). The consistent trend corroborates that Nos is probably synthesized even under aerobic or microaerobic conditions. Studies on extracted and purified Nos support Nos recovery after switching from aerobic to anoxic conditions (Ghosh et al., 2003; Chan et al., 2004). On the basis of these observations, it is likely that recovery of N2O consumption depends on the rate of Nos reactivation.
Toward N2O mitigation in engineered systems, the degree of Nos reactivation after O2 exposure should be quantitatively estimated. Combination of a respirometric analysis with a mechanistic modeling potentially accelerates selection of highly efficient N2O-reducing bacteria able to respire N2O under microaerophilic conditions. The heterotrophic denitrification model, incorporating the O2 inhibition (Equation 3) (Ni et al., 2011), likely necessitates the extension due to some discrepancies with the experimental results (Figure S5). Validation and verification of the extended models, e.g., an enzyme-explicit denitrification model, where a ratio of inactive Nos to total Nos and its recovery rate determine N2O uptake rate (Zheng and Doskey, 2015) and an integrated model incorporating the O2 inhibition and Nos recovery, warrant future intensive studies.
This study shows that strains of the genus Azospira are promising N2O reducers because of their O2 inhibition tolerance and high affinity for N2O. Use of the proposed kinetic parameters, VNos and KI,O2, provides, for the first time, quantitative information on Nos recovery from O2 inhibition. Application of respirometric biokinetic analysis will be useful in developing N2O mitigation strategies using N2O reducers in engineered systems. Comprehensive screening of N2O-reducing bacteria displaying high N2O affinities (a0,N2O) and high resilience against O2 exposure (VNos, KI,O2) is required.
Conclusion
This study investigated the effects of O2 on the N2O consumption biokinetics of bacterial isolates affiliated to nosZ clade I and II types. Respirometric assays showed that the N2O affinities of two Azospira sp. strains of nosZ clade II type were higher than those of Pa. denitrificans and Ps. stutzeri of nosZ clade I type. However, the clade type does not completely explain the different N2O affinities, and this suggests that the physiological traits of N2O-reducing bacteria differ at the species and strain levels. The N2O consumption activities are also significantly affected by the O2 levels but the degree of O2 inhibition differs depending on the species or strain rather than the nosZ type. Azospira sp. strain I09 showed aerobic N2O-reducing activity and nosZ gene transcription at a DO concentration of 110 μM. Recoveries of the N2O-reducing activities of Azospira sp. strains I09 and I13 after O2 exposure are faster than that of Ps. stutzeri of nosZ clade I type. This underscores that the Nos of Azospira spp. is reactivated after switching from aerobic to anoxic conditions. Our results provide new information on the physiological characteristics of N2O-reducing bacteria, and also a valuable investigative parameter involving a respirometric analysis. This comprehensive and thorough analyses based on a respirometric approach will accelerate the discovery of novel N2O reducers and contribute to N2O mitigation in engineering applications.
Author Contributions
AT: designed and led the study. TS: performed the biokinetic estimation and oxygen effect on nitrous oxide reduction of the isolates. TS: wrote the paper with major edits and inputs from SR, MH, and AT.
Funding
We would like to thank the Japan Society for the Promotion of Science (JSPS) for supporting this research with Grants-in-Aid for Challenging Exploratory Research (26630420, 16K12616) and JSPS fellows (16J08601).
Conflict of Interest Statement
The authors declare that the research was conducted in the absence of any commercial or financial relationships that could be construed as a potential conflict of interest.
Acknowledgments
We thank Dr. Barth F. Smets (Technical University of Denmark, Kongens Lyngby, Denmark) and Dr. Tomoyuki Hori (National Institute of Advanced Industrial Science and Technology, Ibaraki, Japan) for discussing this research and Ms. Kanako Mori for supporting molecular biology experiments. The microorganism cultures were provided by the RIKEN Biological Resource Center (JCM, Ibaraki, Japan) and NITE Biological Resource Center (NBRC, Chiba, Japan). We thank Helen McPherson, Ph.D., from Edanz Group (www.edanzediting.com/ac) for editing a draft of this manuscript.
Supplementary Material
The Supplementary Material for this article can be found online at: https://www.frontiersin.org/articles/10.3389/fmicb.2018.00697/full#supplementary-material
References
Ali, M., Rathnayake, R. M. L. D., Zhang, L., Ishii, S., Kindaichi, T., Satoh, H., et al. (2016). Source identification of nitrous oxide emission pathways from a single-stage nitritation-anammox granular reactor. Water Res. 102, 147–157. doi: 10.1016/j.watres.2016.06.034
Arai, H. (2011). Regulation and function of versatile aerobic and anaerobic respiratory metabolism in Pseudomonas aeruginosa. Front. Microbiol. 2:103. doi: 10.3389/fmicb.2011.00103
Baumann, B., Snozzi, M., Zehnder, A. J., and Van Der Meer, J. R. (1996). Dynamics of denitrification activity of Paracoccus denitrificans in continuous culture during aerobic-anaerobic changes. J. Bacteriol. 178, 4367–4374. doi: 10.1128/jb.178.15.4367-4374.1996
Bergaust, L., van Spanning, R. J., Frostegård, Å., and Bakken, L. R. (2012). Expression of nitrous oxide reductase in Paracoccus denitrificans is regulated by oxygen and nitric oxide through FnrP and NNR. Microbiology 158, 826–834. doi: 10.1099/mic.0.054148-0
Black, A., Hsu, P. C. L., Hamonts, K. E., Clough, T. J., and Condron, L. M. (2016). Influence of copper on expression of nirS, norB and nosZ and the transcription and activity of NIR, NOR and N2OR in the denitrifying soil bacteria Pseudomonas stutzeri. Microb. Biotechnol. 9, 381–388. doi: 10.1111/1751-7915.12352
Bueno, E., Mania, D., Frostegard, Å., Bedmar, E. J., Bakken, L. R., and Delgado, M. J. (2015). Anoxic growth of Ensifer meliloti 1021 by N2O-reduction, a potential mitigation strategy. Front. Microbiol. 6:537. doi: 10.3389/fmicb.2015.00537
Chan, J. M., Bollinger, J. A., Grewell, C. L., and Dooley, D. M. (2004). Reductively activated nitrous oxide reductase reacts directly with substrate. J. Am. Chem. Soc. 126, 3030–3031. doi: 10.1021/ja0398868
Conthe, M., Wittorf, L., Kuenen, J. G., Kleerebezem, R., van Loosdrecht, M. C. M., and Hallin, S. (2018). Life on N2O: deciphering the ecophysiology of N2O respiring bacterial communities in a continuous culture. ISME J. 12, 1142–1153. doi: 10.1038/s41396-018-0063-7
Domeignoz-Horta, L. A., Spor, A., Bru, D., Breuil, M. C., Bizouard, F., Léonard, J., et al. (2015). The diversity of the N2O reducers matters for the N2O:N2 denitrification end-product ratio across an annual and a perennial cropping system. Front. Microbiol. 6:971. doi: 10.3389/fmicb.2015.00971
Ghosh, S., Gorelsky, S. I., Chen, P., Cabrito, I., MouraMoura, I., and Solomon, E. I. (2003). Activation of N2O reduction by the fully reduced μ4-sulfide bridged tetranuclear CuZ cluster in nitrous oxide reductase. J. Am. Chem. Soc. 125, 15708–15709. doi: 10.1021/ja038344n
Hallin, S., Philippot, L., Sanford, R. A., and Jones, C. M. (2018). Genomics and ecology of novel N2O-reducing microorganisms. Trends Microbiol. 26, 43–55. doi: 10.1016/j.tim.2017.07.003
Harter, J., Krause, H.-M., Schuettler, S., Ruser, R., Fromme, M., Scholten, T., et al. (2014). Linking N2O emissions from biochar-amended soil to the structure and function of the N-cycling microbial community. ISME J. 8, 660–674. doi: 10.1038/ismej.2013.160
Hein, S., Witt, S., and Simon, J. (2017). Clade II nitrous oxide respiration of Wolinella succinogenes depends on the NosG, -C1, -C2, -H electron transport module, NosB and a Rieske/cytochrome bc complex. Environ. Microbiol. 19, 4913–4925. doi: 10.1111/1462-2920.13935
Henry, S., Bru, D., Stres, B., Hallet, S., and Philippot, L. (2006). Quantitative detection of the nosZ gene, encoding nitrous oxide reductase, and comparison of the abundances of 16S rRNA, narG, nirK, and nosZ genes in soils. Appl. Environ. Microbiol. 72, 5181–5189. doi: 10.1128/AEM.00231-06
Huang, G. Z., Dong, R. H., Allen, R., Davis, E. L., Baum, T. J., and Hussey, R. S. (2005). Developmental expression and molecular analysis of two Meloidogyne incognita pectate lyase genes. Int. J. Parasitol. 35, 685–692. doi: 10.1016/j.ijpara.2005.01.006
IPCC. (2013). Climate Change 2013: The Physical Science Basis. Contribution of Working Group I to the Fifth Assessment Report of the Intergovernmental Panel on Climate Change, IPCC.
Ishii, S., Song, Y., Rathnayake, L., Tumendelger, A., Satoh, H., Toyoda, S., et al. (2014). Identification of key nitrous oxide production pathways in aerobic partial nitrifying granules. Environ. Microbiol. 16, 3168–3180. doi: 10.1111/1462-2920.12458
Jones, C. M., Graf, D. R., Bru, D., Philippot, L., and Hallin, S. (2013). The unaccounted yet abundant nitrous oxide-reducing microbial community: a potential nitrous oxide sink. ISME J. 7, 417–426. doi: 10.1038/ismej.2012.125
Jones, C. M., Spor, A., Brennan, F. P., Breuil, M. C., Bru, D., Lemanceau, P., et al. (2014). Recently identified microbial guild mediates soil N2O sink capacity. Nat. Clim. Chang. 4, 801–805. doi: 10.1038/nclimate2301
Jones, C. M., Stres, B., Rosenquist, M., and Hallin, S. (2008). Phylogenetic analysis of nitrite, nitric oxide, and nitrous oxide respiratory enzymes reveal a complex evolutionary history for denitrification. Mol. Biol. Evol. 25, 1955–1966. doi: 10.1093/molbev/msn146
Juhanson, J., Hallin, S., Söderström, M., Stenberg, M., and Jones, C. M. (2017). Spatial and phyloecological analyses of nosZ genes underscore niche differentiation amongst terrestrial N2O reducing communities. Soil Biol. Biochem. 115, 82–91. doi: 10.1016/j.soilbio.2017.08.013
Körner, H., Frunzke, K., Döhler, K., and Zumft, W. G. (1987). Immunochemical patterns of distribution of nitrous oxide reductase and nitrite reductase (cytochrome cd1) among denitrifying pseudomonads. Arch. Microbiol. 148, 20–24. doi: 10.1007/BF00429641
Körner, H., and Zumft, W. G. (1989). Expression of denitrification enzymes in response to the dissolved oxygen levels and respiratory substrate in continuous culture of Pseudomonas stutzeri. Appl. Environ. Microbiol. 55, 1670–1676.
Law, Y., Ye, L., Pan, Y., and Yuan, Z. (2012). Nitrous oxide emissions from wastewater treatment processes. Philos. Trans. R. Soc. Lond. B Biol. Sci. 367, 1265–1277. doi: 10.1098/rstb.2011.0317
Liu, B., Mao, Y., Bergaust, L., Bakken, L. R., and Frostegard, A. (2013). Strains in the genus Thauera exhibit remarkably different denitrification regulatory phenotypes. Environ. Microbiol. 15, 2816–2828. doi: 10.1111/1462-2920.12142
Lunau, M., Lemke, A., Walther, K., Martens-Habbena, W., and Simon, M. (2005). An improved method for counting bacteria from sediments and turbid environments by epifluorescence microscopy. Environ. Microbiol. 7, 961–968. doi: 10.1111/j.1462-2920.2005.00767.x
Marchant, H. K., Ahmerkamp, S., Lavik, G., Tegetmeyer, H. E., Graf, J., Klatt, J. M., et al. (2017). Denitrifying community in coastal sediments performs aerobic and anaerobic respiration simultaneously. ISME J. 11, 1799–1812. doi: 10.1038/ismej.2017.51
Martens-Habbena, W., Berube, P. M., Urakawa, H., de la Torre, J. R., Stahl, D. A., Torre, J., et al. (2009). Ammonia oxidation kinetics determine niche separation of nitrifying Archaea and Bacteria. Nature 461, 976–979. doi: 10.1038/nature08465
Miyahara, M., Kim, S. W., Fushinobu, S., Takaki, K., Yamada, T., Watanabe, A., et al. (2010). Potential of aerobic denitrification by Pseudomonas stutzeri TR2 to reduce nitrous oxide emissions from wastewater treatment plants. Appl. Environ. Microbiol. 76, 4619–4625. doi: 10.1128/AEM.01983-09
Morley, N., Baggs, E. M., Dörsch, P., and Bakken, L. (2008). Production of NO, N2O and N2 by extracted soil bacteria, regulation by and O2 concentrations. FEMS Microbiol. Ecol. 65, 102–112. doi: 10.1111/j.1574-6941.2008.00495.x
Muyzer, G., Dewaal, E. C., and Uitterlinden, A. G. (1993). Profiling of complex microbial populations by denaturing gradient gel electrophoresis analysis of polymerase chain reaction-amplified genes coding for 16S rRNA. Appl. Environ. Microbiol. 59, 695–700.
Ni, B.-J., Ruscalleda, M., Pellicer-Nacher, C., and Smets, B. F. (2011). Modeling nitrous oxide production during biological nitrogen removal via nitrification and denitrification: extensions to the general ASM models. Environ. Sci. Technol. 45, 7768–7776. doi: 10.1021/es201489n
Orellana, L. H., Rodriguez-R, L. M., Higgins, S., Chee-Sanford, J. C., Sanford, R. A., Ritalahti, K. M., et al. (2014). Detecting nitrous oxide reductase (nosZ) genes in soil metagenomes: method development and implications for the nitrogen cycle. MBio 5:e01193-14. doi: 10.1128/mBio.01193-14
Otte, S., Grobben, N. G., Robertson, L. A., Jetten, M. S., and Kuenen, J. G. (1996). Nitrous oxide production by Alcaligenes faecalis under transient and dynamic aerobic and anaerobic conditions. Appl. Environ. Microbiol. 62, 2421–2426.
Pan, Y., Ni, B. J., Bond, P. L., Ye, L., and Yuan, Z. (2013). Electron competition among nitrogen oxides reduction during methanol-utilizing denitrification in wastewater treatment. Water Res. 47, 3273–3281. doi: 10.1016/j.watres.2013.02.054
Park, D., Kim, H., and Yoon, S. (2017). Nitrous oxide reduction by an obligate aerobic bacterium Gemmatimonas aurantiaca strain T-27. Appl. Environ. Microbiol. 83:e00502-17. doi: 10.1128/AEM.00502-17
Pauleta, S. R., Dell'Acqua, S., and Moura, I. (2013). Nitrous oxide reductase. Coord. Chem. Rev. 257, 332–349. doi: 10.1016/j.ccr.2012.05.026
Philippot, L. (2002). Denitrifying genes in bacterial and Archaeal genomes. Biochim. Biophys. Acta 1577, 355–376. doi: 10.1016/S0167-4781(02)00420-7
Philippot, L., Andert, J., Jones, C. M., Bru, D., and Hallin, S. (2011). Importance of denitrifiers lacking the genes encoding the nitrous oxide reductase for N2O emissions from soil. Glob. Chang. Biol. 17, 1497–1504. doi: 10.1111/j.1365-2486.2010.02334.x
Ravishankara, A. R., Daniel, J. S., and Portmann, R. W. (2009). Nitrous oxide (N2O): The dominant ozone-depleting substance emitted in the 21st century. Science 326, 123–125. doi: 10.1126/science.1176985
Read-Daily, B. L., Sabba, F., Pavissich, J. P., and Nerenberg, R. (2016). Kinetics of nitrous oxide (N2O) formation and reduction by Paracoccus pantotrophus. AMB Express 6:85. doi: 10.1186/s13568-016-0258-0
Riya, S., Zhou, S., Watanabe, Y., Sagehashi, M., Terada, A., and Hosomi, M. (2012). CH4 and N2O emissions from different varieties of forage rice (Oryza sativa L.) treating liquid cattle waste. Sci. Total Environ. 419, 178–186. doi: 10.1016/j.scitotenv.2012.01.014
Sanford, R. A., Wagner, D. D., Wu, Q., Chee-Sanford, J. C., Thomas, S. H., Cruz-García, C., et al. (2012). Unexpected nondenitrifier nitrous oxide reductase gene diversity and abundance in soils. Proc. Natl. Acad. Sci. U.S.A. 109, 19709–19714. doi: 10.1073/pnas.1211238109
Soler-Jofra, A., Stevens, B., Hoekstra, M., Picioreanu, C., Sorokin, D., van Loosdrecht, M. C. M., et al. (2016). Importance of abiotic hydroxylamine conversion on nitrous oxide emissions during nitritation of reject water. Chem. Eng. J. 287, 720–726. doi: 10.1016/j.cej.2015.11.073
Tallec, G., Garnier, J., Billen, G., and Gousailles, M. (2006). Nitrous oxide emissions from secondary activated sludge in nitrifying conditions of urban wastewater treatment plants: effect of oxygenation level. Water Res. 40, 2972–2980. doi: 10.1016/j.watres.2006.05.037
Terada, A., Sugawara, S., Hojo, K., Takeuchi, Y., Riya, S., Harper, W. F., et al. (2017). Hybrid nitrous oxide production from a partial nitrifying bioreactor: hydroxylamine interactions with nitrite. Environ. Sci. Technol. 51, 2748–2756. doi: 10.1021/acs.est.6b05521
Terada, A., Sugawara, S., Yamamoto, T., Zhou, S., Koba, K., and Hosomi, M. (2013). Physiological characteristics of predominant ammonia-oxidizing bacteria enriched from bioreactors with different influent supply regimes. Biochem. Eng. J. 79, 153–161. doi: 10.1016/j.bej.2013.07.012
Vollack, K. U., and Zumft, W. G. (2001). Nitric oxide signaling and transcriptional control of denitrification genes in Pseudomonas stutzeri. J. Bacteriol. 183, 2516–2526. doi: 10.1128/JB.183.8.2516-2526.2001
Weiss, R. F., and Price, B. A. (1980). Nitrous-oxide solubility in water and seawater. Mar. Chem. 8, 347–359. doi: 10.1016/0304-4203(80)90024-9
Wunderlin, P., Mohn, J., Joss, A., and Emmenegger, L. (2011). Mechanisms of N2O production in biological wastewater treatment under nitrifying and denitrifying conditions. Water Res. 46, 1027–1037. doi: 10.1016/j.watres.2011.11.080
Yoon, S., Nissen, S., Park, D., Sanford, R. A., and Löffler, F. E. (2016). Nitrous oxide reduction kinetics distinguish bacteria harboring clade I versus clade II NosZ. Appl. Environ. Microbiol. 82, 3793–3800. doi: 10.1128/AEM.00409-16
Zheng, J., and Doskey, P. V. (2015). Modeling nitrous oxide production and reduction in soil through explicit representation of denitrification enzyme kinetics. Environ. Sci. Technol. 49, 2132–2139. doi: 10.1021/es504513v
Zheng, M., He, D., Ma, T., Chen, Q., Liu, S., Ahmad, M., et al. (2014). Reducing NO and N2O emission during aerobic denitrification by newly isolated Pseudomonas stutzeri PCN-1. Bioresour. Technol. 162, 80–88. doi: 10.1016/j.biortech.2014.03.125
Zhu, X., Burger, M., Doane, T. A., and Horwath, W. R. (2013). Ammonia oxidation pathways and nitrifier denitrification are significant sources of N2O and NO under low oxygen availability. Proc. Natl. Acad. Sci. U.S.A. 110, 6328–6333. doi: 10.1073/pnas.1219993110
Zumft, W. G. (1997). Cell biology and molecular basis of denitrification. Microbiol. Mol. Biol. Rev. 61, 533–616.
Keywords: nitrous oxide reduction, O2 inhibition, heterotrophic denitrification, biokinetic analysis, microsensor
Citation: Suenaga T, Riya S, Hosomi M and Terada A (2018) Biokinetic Characterization and Activities of N2O-Reducing Bacteria in Response to Various Oxygen Levels. Front. Microbiol. 9:697. doi: 10.3389/fmicb.2018.00697
Received: 05 December 2017; Accepted: 26 March 2018;
Published: 10 April 2018.
Edited by:
Frank Schreiber, Bundesanstalt für Materialforschung und Prüfung (BAM), GermanyReviewed by:
Sukhwan Yoon, Korea Advanced Institute of Science & Technology (KAIST), South KoreaPaul V. Doskey, Michigan Technological University, United States
Copyright © 2018 Suenaga, Riya, Hosomi and Terada. This is an open-access article distributed under the terms of the Creative Commons Attribution License (CC BY). The use, distribution or reproduction in other forums is permitted, provided the original author(s) and the copyright owner are credited and that the original publication in this journal is cited, in accordance with accepted academic practice. No use, distribution or reproduction is permitted which does not comply with these terms.
*Correspondence: Akihiko Terada, YWt0ZUBjYy50dWF0LmFjLmpw