- 1Department of Pathology, University of California, San Diego, San Diego, CA, United States
- 2Department of Internal Medicine, University of Texas Medical Branch, Galveston, TX, United States
Pathogenic and commensal microbes induce various levels of inflammation and metabolic disease in the host. Inflammation caused by infection leads to increased production of reactive oxygen species (ROS) and subsequent oxidative DNA damage. These in turn cause further inflammation and exacerbation of DNA damage, and pose a risk for cancer development. Helicobacter pylori-mediated inflammation has been implicated in gastric cancer in many previously established studies, and Fusobacterium nucleatum presence has been observed with greater intensity in colorectal cancer patients. Despite ambiguity in the exact mechanism, infection-mediated inflammation may have a link to cancer development through an accumulation of potentially mutagenic DNA damage in surrounding cells. The multiple DNA repair pathways such as base excision, nucleotide excision, and mismatch repair that are employed by cells are vital in the abatement of accumulated mutations that can lead to carcinogenesis. For this reason, understanding the role of DNA repair as an important cellular mechanism in combatting the development of cancer will be essential to characterizing the effect of infection on DNA repair proteins and to identifying early cancer biomarkers that may be targeted for cancer therapies and treatments.
Introduction
The significance of cancer as a disease that affects a large percentage of the world population is undeniable. It is one of the leading causes of death worldwide and according to World Health Organization, it causes 8–9 million deaths/year. In the United States alone, it is projected that 39.6% of the population will have some type of cancer during their life. Consequently, there are enormous expenditures in the field of cancer care. The expenditures for cancer care in the United States were nearly $125 billion in 2010 and could reach $156 billion in 2020; as mentioned in the website of National Cancer Institute. Infection and infection-associated inflammation is the major threat of cancer. Chronic inflammation from infection causes abnormal immune response, obesity, DNA damage and cancer. The best example is the inflammatory bowel disease where Ulcerative colitis and Crohn's disease develop colon cancer.
In 1863, Rudolf Virchow was the first scientist to link inflammation with cancer. He mentioned that the origin of cancer was at sites of chronic inflammation and a group of irritants with tissue injury causes cell proliferation (Balkwill and Mantovani, 2001). How does inflammation initiate malignancies? The possible answer to this question is the infection associated with chronic inflammation. Approximately 20% of cancer worldwide is caused by infection (Kuper et al., 2000; Parkin, 2006). The last 20 years of research shows that microbial infection is associated with cancer and can induce cancer progression. According to the estimate of the International Agency for Research on Cancer (IARC) approximately 18% of cancer are associated to infectious diseases that are caused by bacteria, viruses, and parasites. The well-known examples are human papilloma viruses (HPV; causing anogenital cancers), Helicobacter pylori (gastric cancers), hepatitis B and C viruses (hepatic cancers), and Fusobacterium (Colon cancer). The detailed list of microbes that have been researched in relation to their effect on certain DNA repair proteins are added in the Table 1. The gaps in knowledge in this field are reflected in the table, where there are many pathogen-associated cancers that have not been studied in relation to DNA repair proteins.
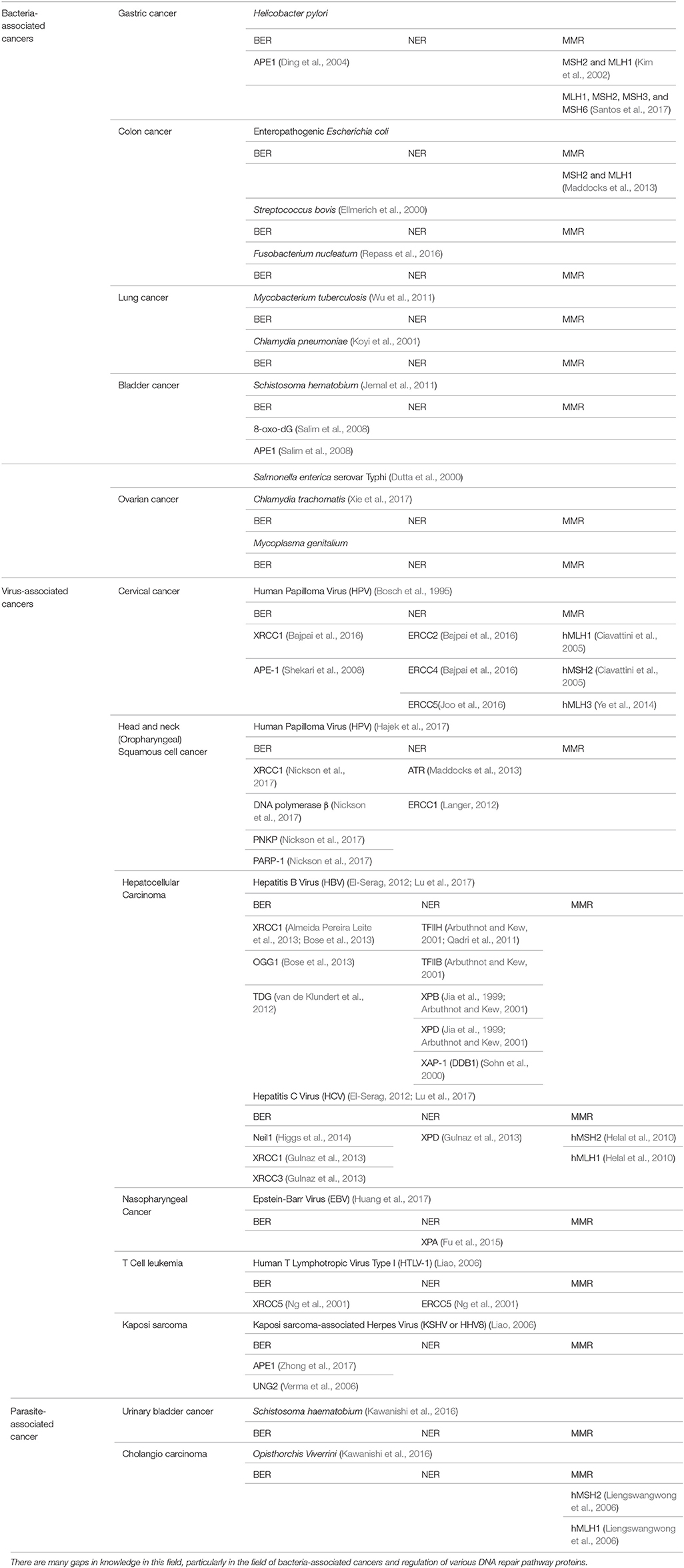
Table 1. A compilation of bacteria, virus, and parasite-associated cancers with some of the available information on their link to BER, NER, and MMR protein expression or mutations.
As cited in the press release of the Nobel Assembly (Marshall and Warren, 1984, 2005): “Many diseases in humans such as Crohn's disease, ulcerative colitis, rheumatoid arthritis, and atherosclerosis are due to chronic inflammation. The discovery that one of the most common diseases of mankind, peptic ulcer disease, has a microbial cause has stimulated the search for microbes as possible causes of other chronic inflammatory conditions. Even though no definite answers are at hand, recent data clearly suggest that a dysfunction in the recognition of microbial products by the human immune system can result in disease development. The discovery of Helicobacter pylori has led to an increased understanding of the connection between chronic infection, inflammation, and cancer.”
Chronic infection generates a milieu of inflammatory cytokines that leads to inflammatory microenvironment, a critical modulator of carcinogenesis. The persistent infection and chronic inflammation changes somatic cells by the influence of associated microbes and epigenetic factors (Fernandes et al., 2015). Hanahan et al. showed that genome instability and inflammation are the emerging hallmarks associated with cancer (Hanahan and Weinberg, 2011). Figure 1 shows the responsive elements that can trigger carcinogenesis. Bacterial infections increase cancer risk through either an extrinsic pathway, linked to induction of chronic inflammatory diseases that can increase cancer risk, or an intrinsic pathway, which is the accrual of genetic mutations that cause inflammation and transformation (Mantovani et al., 2008). Chronic inflammation has been associated with multiple types of cancer to the extent that inflammation period has been linked to increased risk of carcinogenesis (Shacter and Weitzman, 2002). Chronic inflammation is able to adjust the tumor microenvironment with cells such as tumor associated macrophages and various inflammatory agents such as chemokines, to regulate both tumor growth and angiogenesis (Coussens and Werb, 2002). Inflammation is also able to induce growth factors that serve several roles in carcinogenesis and tumorigenesis (Hanahan and Weinberg, 2011). The intrinsic pathway of genome alterations caused by infection is often linked to inflammation-mediated reactive oxygen species (ROS) production, which can increase the rate of genetic mutations that can accumulate to cause cancer (Hanahan and Weinberg, 2011).
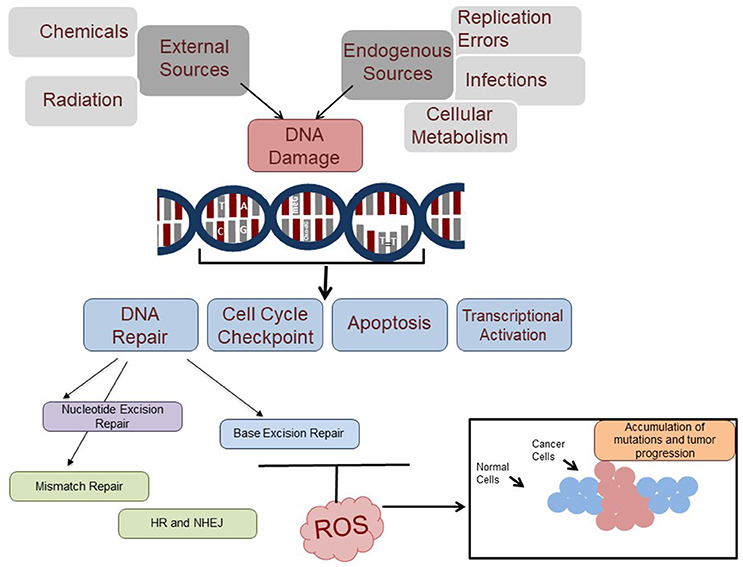
Figure 1. DNA damage response and the potential role of ROS in inhibition of DNA repair. DNA damage is induced by external (various environmental pollutants, chemicals, and radiation), and internal resources (infection, cellular metabolism and replication errors). These DNA damages affect the cell cycle check point, apoptosis, transcriptional activation and cancer. Part of the DNA damages (DNA base adducts, mismatch bases, damaged bases, and double strand breaks) are repaired by the nucleotide excision repair, mismatch repair, base excision repair, and homologous recombination (HR) and non-homologous end joining (NHEJ) pathways, respectively. Many of the DNA damages are ROS-induced and recognized by the BER pathway which excises and repairs the lesions. However, ROS may potentially inhibit repair through down-regulation of certain initial proteins in the BER pathway, which can cause a buildup of carcinogenic mutations and ultimately lead to tumor progression.
Bacterial infection causes inflammatory response and the ROS generated by bacterial infection often results in genomic instability (Chumduri et al., 2016). This implicates a bacterial infection in compromising or at least impeding some of the several cellular mechanisms for maintaining genetic integrity and repairing mutations. As genomic instability is an underlying factor in almost all cancer cells, the link between infection and cancer development and progression is a significant one. This review will focus on the mechanism of inflammation and ROS production post-infection, and then elaborate on the genomic instability induced by infection/inflammation by discussing the effect on various DNA repair pathways. As a major focus, we will bring the link of Helicobacter pylori with gastric cancer and microbial infection associated colorectal cancer under the scope of DNA damage repair following infection.
Microbial Infection-Mediated Inflammation Linked to Cancer
Innate and adaptive immune responses are important to protect self from pathogenic microbial attack. Understanding of the infection process is important as bacterial and viral infection induces the inflammation that increases cancer risk (de Martel and Franceschi, 2009). The innate and adaptive immune responses will be discussed in the next section.
Innate Immune Response
The immune response following recognition and invasion of microbes such as bacteria and viruses split into the innate and adaptive responses. Recognition and the initial precautionary actions are taken by the pattern recognition receptors (PRRs) of innate immune system (Pasare and Medzhitov, 2004; Akira et al., 2006). PRRs are found on the surfaces of epithelial cells and several immune cells to recognize structurally conserved pathogen-associated microbial products (PAMPs). For example, Toll-like receptor 4 (TLR4) is a PRR that binds lipopolysaccharide (LPS) on the outer membrane of gram-negative bacteria; TLR2 binds bacterial lipoproteins and lipoteichoic acids. The cytosolic sensor; NOD-like receptors (NLRs) detect intracellular pathogens. The innate immune response is the immediate mechanism by which the host attempts to clear an infection. Most of the PRRs lead to activation of MYD88-dependent pathways that eventually lead to NF-κB activation. This leads to further production of inflammatory cytokines and chemokines as well as antimicrobial peptides. Like TLRs, NLRs lead to an inflammatory response from both this signaling cascade and through the activation of caspases that act on cytokines that mediate the rest of the inflammatory response (Zarember and Godowski, 2002; Barton and Medzhitov, 2003; Basset et al., 2003; Tsung et al., 2007; Church et al., 2008; Martinon et al., 2009; West et al., 2011b). The PRRs mediated inflammatory response is initiated by various inflammatory cytokines and chemokines, which draw macrophages and mast cells that release inflammatory mediators to recruit neutrophils and plasma proteins. Neutrophils phagocytose the pathogen and surrounding debris. Phagolysosomes form through the fusion of phagosomes with granules consisting of enzymes and ROS that can kill the phagocytosed pathogen. The neutrophils can release these toxic granules and cause collateral damage to the surrounding tissue (Segal, 2005; Medzhitov, 2008; Serhan et al., 2008).
The increase in the pro-inflammatory cytokines will clear the bacteria and the release of pro-inflammatory agents will be halted (Figure 2), when the tissue repair phase will be started. The tissue damage and debris resulting from the neutrophil activity may prevent impedance of inflammatory response and lead to continued chronic inflammation (Nathan, 2002). Various non-degradable components of the eliminated pathogen also contribute to a lasting inflammatory response even after the threat of the invading pathogen has been erased (Medzhitov, 2008).
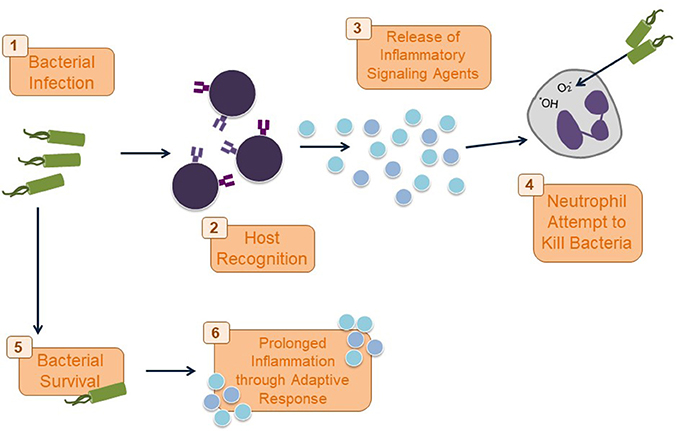
Figure 2. Immune response to bacterial infection. Once a bacterial infection is recognized by PRRs on host cells as part of the innate immune response, inflammatory cytokines and chemokines are released to draw neutrophils to the site of infection. Neutrophils phagocytose and kill the bacteria with ROS. If this initial response does not kill the bacteria, the adaptive immune response kicks in and may result in a chronic inflammatory response.
Adaptive Immune Response
If the innate immune response is not adequate to kill the pathogen, the adaptive immune response is activated by continued inflammation at the site of infection (Zarember and Godowski, 2002; Martinon et al., 2009). The microbes must then be cleared by specialized lymphocyte (B and T-cell)-mediated mechanisms (Medzhitov, 2008). For this reason, the adaptive immune response requires time to be initiated. Prolonged infection can lead to continuous tissue damage and a chronic inflammatory response that results in various diseases. For instance, in the intestinal tract, inflammatory bowel diseases such as Crohn's disease and ulcerative colitis may manifest due to conditions of chronic inflammation that result from excessive immune activation exacerbating the initial inflammatory response to a foreign or commensal microbe (Cong et al., 2002; Macdonald and Monteleone, 2005). Specifically in Crohn's disease, the adaptive immune response plays a role through an extreme CD4 T helper type I response to overexpression of various inflammatory cytokines (Macdonald and Monteleone, 2005).
Mechanisms That Link Inflammation to Cancer Progression
The cytokines and chemokines from innate and adaptive immune cells direct the progression of the tumor microenvironment. Downstream effectors NF-κB, AP-1, STAT control the inflammatory milieu either by affecting tumor survival, growth, and tumor progression by signaling for molecules such as IL-6, IL-23 or by anti-tumor immunity (IFN-γ, IL-12). The inflammatory cytokines have been reported to generate ROS and reactive nitrogen intermediates (RNI) using NADPH oxidase in phagocytic cells and epithelial cells (Yang et al., 2007). These ROS are the major source of damage to nucleic acid, proteins and lipids.
Reactive Oxygen Species (ROS)
ROS refer to various, highly reactive and partially reduced metabolites of oxygen such as H2O2 that are essential signaling molecules in the human immune system (Martindale and Holbrook, 2002; West et al., 2011b). ROS includes oxygen radicals (superoxide, hydroxyl, peroxyl and alkoxyl) and certain nonradicals that are either oxidizing agents and/or are easily converted into radicals, such as HOCl, ozone, peroxynitrite, singlet oxygen and hydrogen peroxide. ROS initiates DNA base oxidation which, if not repaired properly, may lead to induce a mutation.
Inflammatory cytokine signaling also depend on ROS. ROS is also crucial for inflammasome signaling and increased mitochondrial ROS activate the NLRP3 inflammasome (Zhou et al., 2011). Additionally, the post-translational modifications that ROS are associated with are often linked to modifications in protein cysteine residues that are generally associated with either Ca2+ mediated signaling or tyrosine phosphorylation. This implicates ROS in cell motility, mitosis, differentiation, and immune response or regulation (van der Vliet, 2008). The immediate effect of excessive ROS presence in the host is a chronic inflammatory state that exacerbates both inflammation and, as a result, ROS production. Also, cancer cells utilize mROS to constitutively activate proliferation pathways to promote tumor growth (Cairns et al., 2011). Inability of cellular antioxidants to curtail ROS leads to oxidative stress on host cells that can lead to many adverse effects including induction of DNA damage (Ernst, 1999). Therefore, following oxidative stress, the cell must survive by either adapting to the induced stress or by repairing the damage (Martindale and Holbrook, 2002). Inability to repair or adjust to the damage will lead to chronic conditions such as cancer, diabetes, and various neurological or cardiovascular diseases. The specific mechanisms of DNA damage repair that diminish the oxidative damage done by ROS will be discussed later in this review.
ROS in immune cells are important to kill extracellular pathogens by using the NADPH oxidase in phagosomes. Despite the various positive roles of ROS in immunity and other processes, they are associated with conditions such as diabetes, hypertension, cancer, and autoimmune diseases due to their ability to change and damage cellular proteins, lipids, and DNA (Zimmerman and Cerutti, 1984; Guzik et al., 2007). ROS is acquired through both exogenous and endogenous sources. Exogenous sources of ROS include carcinogen induced or generated ROS; for example, xenobiotics, chlorinated compounds, and radiation are associated to oxidative stress. The main endogenous source of ROS in the human body is through mitochondrial respiration or Ox-Phos (Oxidative phosphorylation) system. The electron transport chain uses mitochondrial oxidative phosphorylation complexes that lead to generation of ROS that can potentially harm cellular components such as proteins and nucleic acids through post-translational modifications and oxidation (Molina-Cruz et al., 2008). ROS produced by Ox-Phos pathway participate in immune signaling with TLR and cytosolic RIG-I like receptors (RLRs). West et al. reported that stimulation of cell-surface TLRs (TLR1, TLR2, and TLR4), but not endosomal TLRs (TLR3, TLR7, TLR8, and TLR9), leads to an increase in mROS (Mitochondrial Reactive Oxygen Species) production through TRAF6 and ECSIT signaling (West et al., 2011a). Mitochondrial ROS enhance RLR signaling in autophagy-dependent Atg5-depleted cells that indicate the importance of autophagy in innate antiviral defense (Tal et al., 2009).
The most significant damages caused to DNA due to high concentrations of ROS include double and single strand breaks, oxidized DNA bases, and aberrant DNA cross-linking. Each type of ROS plays a different role in inflicting damage. For example, hydrogen peroxide (H2O2) directly induces DNA damage. The versatility of different ROS is reflected in the wide array of DNA damage that they can cause. In general, the hydroxyl molecule (OH−) is the most damaging form of ROS, but other forms like the oxygen molecule (O2), RO2, and RO are also capable of different types of damage. For instance, while OH− can react with all of the bases and the deoxyribose backbone of DNA, O2 preferentially targets guanine residues (Wiseman and Halliwell, 1996; Valko et al., 2007; Imlay, 2008). In a wider scope, ROS are generally likely to react with and damage DNA through oxidation, methylation, depurination, and deamination. The lesion most often found due to oxidative DNA damage, 8-hydroxyguanine (8-OHdG) or the nucleoside 8-hydroxydeoxyguanosine, is generally considered as marker of oxidative damage incurred by a cell. For instance, many studies examined for increased production of ROS culminating in an increased level of oxidative DNA damage by measuring 8-hydroxyguanine (Dandona et al., 1996; Farinati et al., 1998). In another study, it was observed that P53 acts as a defense against ROS-mediated DNA oxidation in various experimental conditions by detecting the presence of 8-oxodeoxyguanosine in the DNA (Sablina et al., 2005). The 8-hydroxydeoxyguanosine and other oxidized DNA lesions (8-oxo-adenine, thymine glycol, 5-hydroxy-deoxycytidine) have also been observed in many mutated oncogenes and tumor suppressor genes and these lesions are able to induce further neoplastic mutations in the DNA. The presence of high levels of 8-oxoguanine lesions, along with many other oxidative lesions, was shown in the DNA of tumorous tissues from many patients with different types of cancers (Klaunig and Kamendulis, 2004). This indicates a serious implication of oxidative stress and oxidative DNA damage in carcinogenesis (Figure 1).
Involvement of Microbes in ROS-Linked Carcinogenesis
The gut microbiota supplement a significant portion of the human metabolism, and the composition and activity of the microbiota plays a large role in susceptibility to metabolic diseases such as hyperglycemia, hyperlipidemia, insulin resistance, and obesity (Vijay-Kumar et al., 2010; Spencer et al., 2011). Microbes protect themselves from the ROS generated by host using an enzyme called superoxide dismutase (Sod), which is abundant in cells throughout the body. This enzyme attaches (binds) to molecules of copper and zinc to break down toxic, charged oxygen molecules called superoxide radicals. Interestingly, bacteria has protective proteins such as SodA, SodB, SodC, AhpCF, KatG, KatE to detoxify ROS and proteins to counter damage (e.g., SoxRS, OxyRS, and SOS regulons) (Imlay, 2008). On the host side, Nox2, present in the NADPH complex is responsible for the generation of ROS (Lambeth, 2004). The generation of ROS is important for host defense as patients with chronic granulomatous disease (CGD) with deficiencies in NOX2 components are susceptible to infection (Cross et al., 2000).
Transcription factors vital to cellular processes such as inflammation, cell cycle regulation, motility, and growth like NF-κB, p53, HIF-1α, β-catenin/Wnt can be activated by the oxidative stress caused by an imbalance in the presence of oxidative ROS and the countering antioxidants. By mediating activity of genes and proteins related to oxidative stress, ROS are able to affect further cellular properties, such as cell growth, differentiation, and apoptosis, which can induce transformation (Reuter et al., 2010). For instance, ROS produced as a result of exposure of mouse mammary epithelial cells to MMP-3, a stromal enzyme that is linked to inducing epithelial-mesenchymal (EMT) transition and malignant transformation, caused activation of the transcription factor SNAIL, which induced oxidative DNA damage and EMT (Radisky et al., 2005). ROS-induced oxidative stress can also affect gene expression through direct DNA (de)methylation, which is an epigenetic method of silencing and activating certain genes by changing the physical accessibility of certain genes (Klaunig and Kamendulis, 2004). Chromosomal alterations induced by ROS can also lead to cellular damage. The genomic instability and transcriptional changes that accompany ROS and oxidative stress can therefore lead to carcinogenesis.
Post-infection inflammation-mediated mechanisms that assist in tumor formation and progression are an indirect method of bacterial infection leading to cancer. There are also several factors in a bacterial infection that can directly induce DNA damage or alter cell-signaling pathways that can lead to carcinogenesis. For instance, several bacteria such as Escherichia coli and Shigella dysenteriae are able to produce genotoxins (colibactin and shiga toxin, respectively) that inflict damage on the host DNA such as DNA strand breaks that may affect tumor suppressors or oncogenes (Gagnaire et al., 2017).
DNA Repair Mechanisms
Cellular DNA is altered either during replication or by external mutagens. Misincorporation of DNA bases can occur during replication; however, it is combatted through the proofreading activity of DNA polymerases. There may however be errors during DNA replication that are not recognized by the polymerases. There is also a great wealth of mutagens that can cause extensive changes in the sequence of human DNA. Of these, just oxidative DNA damage is estimated to arise about 105 times in 1 day due to ROS-induced damages (Lengauer et al., 1998). Accumulation of all these mutations would severely inhibit the ability of cells to survive and/or maintain proper cellular functions in almost all cases. Fortunately, the cell has several mechanisms that are specialized to recognize and repair different types of DNA damage. Of these, this review will describe the mechanisms that address alterations in DNA sequence and their link to various cancers, including mismatch repair (MMR), nucleotide excision repair (NER), base excision repair (BER), and homologous recombination (HR) and non-homologous end joining (NHEJ). Most importantly, MMR, BER, HR, and NHEJ alterations have been linked to chronic inflammatory states (Figure 3). Therefore, the focus will be on genetic instabilities induced through deregulation of these repair pathways, in some cases due to inflammation, that lead to genetic instabilities that may contribute to cancer formation.
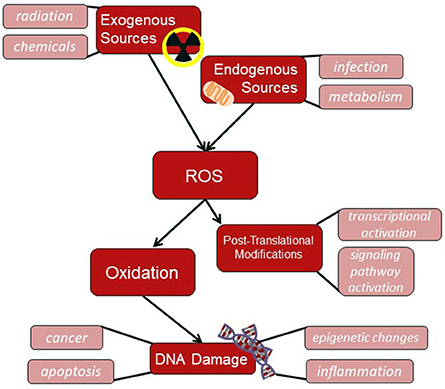
Figure 3. ROS flowchart. ROS produced by either exogenous sources, such as radiation, or endogenous sources, such as through cellular mitochondria, can induce DNA damage through oxidation or cause post-translational modifications on cellular proteins.
DNA repair pathways recognize and correct mismatches present in the DNA, abnormal bases, single-stranded and double-stranded DNA breaks (DSBs). The MMR, BER, and NER pathways respond to specific lesions in DNA residues. DSBs are particularly dangerous lesion and are repaired by two principal pathways: NHEJ pathway functions in all phases of the cell cycle, while the high-fidelity HR pathway requires a template for repair and utilizes available sister chromatids during the S and G2 phases of the cell cycle.
Nucleotide Excision Repair
Nucleotide excision repair, or NER, is a versatile repair pathway that recognizes bulky adducts and general base lesions that cause a distortion of the double-helix structure of DNA. The major sources of these types of DNA damage are ultraviolet radiation and various types of genotoxic chemicals (Hoeijmakers, 2001). These lead to lesions such as pyrimidine dimers, cyclobutane pyrimidine dimers (CPD), and 6–4 photoproducts. NER-mediated pathway employs several different proteins to carry out a multi-step “cut-and-patch”-like pathway (Shuck et al., 2008). The defect in NER generates human genetic disorders and the bulky adducts targeted by NER mechanism can block replication and/or transcription which can lead to apoptosis or necrosis (Sancar et al., 2004).
The NER mechanism is divided into two pathways that have different methods of lesion recognition. These are the global-genome (GG-NER) and transcription-coupled repair (TC-NER) pathways. In GG-NER, DNA damage is removed from the whole genome while TC-NER is primarily involved in repairing the damage on the coding strand of actively transcribed genes (Hoeijmakers, 2001; Jackson and Bartek, 2009). Both pathways differ in the initial recognition steps. In GG-NER, the major proteins involved in the recognition are the XPC/HR23B/CEN2 (XP complementation group C/Rad23 homolog B/Centrin-2) protein complex. TC-NER is important to protect the cells from UV-light-induced apoptosis. In TC-NER, the damage is recognized and RNA Pol II stalls, Cockayne syndrome protein CSB transiently interact with RNA Pol II and the other associated proteins can take care of the damage to repair. In patients with Cockayne syndrome have defective TC-NER (Sancar et al., 2004; Fousteri and Mullenders, 2008; Hanawalt and Spivak, 2008).
Mismatch Repair
Mismatch repair, or MMR, fixes mismatched base pairs and insertion-deletion loops that are generally a product of incorrect genomic DNA replication. MMRs have the ability to inflict serious damage on the cell without killing it since they may go unnoticed and accumulate (Kunkel and Erie, 2005). MMR deficient cells can display a mutator phenotype, characterized by microsatellite instability and an elevated mutation frequency. The germline mutations in MMR genes can lead to a variety of cancers, including the non-polyposis colon cancer/ Lynch syndrome (Peltomäki, 2001). MMR involves three steps: a recognition step for identifying the mispaired bases, an excision step to remove the error-containing and the synthesis step, where the gap is filled-in by DNA polymerases. Therefore, the MMR pathway is very important to prevent cancer.
Several MMR proteins can be regulated upon chronic inflammation through the activation of HIF-1α by inflammatory cytokines and ROS (Colotta et al., 2009). The inability of MMR to repair single base pair or small-scale mutations results in microsatellite instabilities such as poly CA repeats, present in various cancers (Lengauer et al., 1998).
Base Excision Repair
Base-excision repair or BER recognizes a wide variety of damaged bases including those that underwent oxidation, alkylation, methylation, deamination, and hydroxylation (Hoeijmakers, 2001). It also repairs ROS induced strand breaks that consist of sugar fragments or 3′phosphate ends that are non-ligatable (Hegde et al., 2008). Due to its recognition and repair of an extensive range of ROS induced damages, BER is the major defense against accumulation of mutations caused by ROS. Additionally, the lesions targeted by BER are generally small-DNA base adducts. These types of lesions are more likely to be kept within the genome without cell apoptosis and can result in continued mutations in tumor suppressor and oncogenes that can lead to cancer (Hoeijmakers, 2001). Especially through this framework, BER earns greater importance in preventing cellular transformation and cancer.
Single strand break repair pathway (SSBR) is now considered a specialized sub-pathway of BER. They share several common proteins including APE1, Polβ, LIGIIIα, along with the nick sensor poly (ADP-ribose) polymerase 1 (PARP1) and the scaffold protein X-ray cross-complementation group 1 (XRCC1) (Caldecott, 2008). ROS generate 8-oxoguanine (8-oxoG), ring-opened purines (formamidopyrimidines or Fapys), and other oxidized DNA base lesions that are repaired via the DNA BER pathway. BER begins with the recognition of an altered base by a DNA glycosylase. There are two classes of DNA glycosylases, the first group with the enzymes OGG1 and NTH1 utilize an internal Lys residue as the active site nucleophile. The second group comprising of NEIL1, NEIL2, and NEIL3 use N-terminal Pro or Val as the active site (Hazra et al., 2002, 2007; Sancar et al., 2004). The first and second group have distinct structural features and reaction mechanism but have overlapping substrate specificities. The NEIL proteins are able to preferentially target single stranded DNA and also lesions from a DNA bubble while NTH1 and OGG1 only excise lesions from double-stranded DNA, as they use the second strand as a template for repair (Hegde et al., 2008). Therefore, the NEIL proteins can be functional for replication/transcription errors (Banerjee et al., 2011).
These oxidized base-specific mammalian DNA glycosylases are bifunctional. Monofunctional DNA glycosylases excise the altered base in a way that leaves behind an AP site that needs to be processed by an AP endonuclease (3′-OH and 5′dRP are generated by APE1). On the other hand, the mammalian bifunctional DNA glycosylases have an associated AP lyase activity generating 3′dRP (OGG1, NTH1, and NEIL3) or 3′-P (NEIL1 and 2) and 5′-P. The DNA polymerase then fills in the gap using the template DNA strand and finally, DNA ligase seals the nick by completing the repair of the DNA duplex (Hitomi et al., 2007; Hegde et al., 2008; Maynard et al., 2009). The essential component of BER is the DNA glycosylase that recognizes and removes the oxidized base. Inhibition of the BER proteins may lead to an accumulation of oxidized DNA damage induced by ROS and the possible escalation of mutation rate upon an inflammatory response to bacterial infection that can contribute to carcinogenesis (Maynard et al., 2009).
The fact that defects in various components of NER and MMR have been shown to contribute to certain cancers indicates that the mutations that arise from inhibition of these repair mechanisms are certainly an important causative factor to cancer. For this reason, although BER has not been studied as thoroughly as NER and MMR in the context of cancer, it may have a significant role in the perpetuation of bacterial infection and inflammation mediated cancers such as gastric cancer and, to some degree, colorectal cancer (Wallace et al., 2012; Leguisamo et al., 2017). Various human DNA glycosylases have already been implicated in various types of cancer. NEIL2 was shown to protect against the oxidative damage that is induced by secondhand smoke in human lung cells, and lower levels of NEIL2 are associated with development of lung tumors (Sarker et al., 2014). It has also been shown that knock out of NEIL2 increased the accumulation of spontaneous mutations, and a variant of NEIL2 was observed in lung cancer samples (Dey et al., 2012). Furthermore, functional variants of NEIL2 have been linked to greater risk of squamous cell carcinoma in the oral cavity and oropharynx, making it a possible marker for risk to and progression of squamous cell carcinoma in the oral cavity and oropharynx (Zhai et al., 2008). In a study of human non-small cell lung cancer, it was found that carriers who were positive for silencing of the DNA glycosylase OGG1 via methylation had a 2.25-fold higher risk of developing non-small cell lung cancer than carriers who did not exhibit OGG1 methylation (Qin et al., 2017). In another study, an OGG1 variant (Ser326Cys polymorphism) was found to increase lung cancer risk by 24% in an analysis of seven studies totaling over 3,000 cases and controls. Both the mRNA and protein expression of MUTYH, a human DNA glycosylase that repairs the foremost oxidative DNA damage in prostate cancer (8-hydroxyguanine), was down-regulated in about two thirds of prostate cancers compared to the non-cancerous prostate tissue data presented in two separate publicly available databases (Shinmura et al., 2017). The apparent links between DNA glycosylase inhibition or silencing and various cancers indicates that: (1) Oxidative damage is a major contributor to the accumulation of genetic mutations that can lead to carcinogenesis, and (2) BER plays a significant role in repressing the accumulation of ROS-induced mutations and therefore inhibition of certain BER proteins may contribute to carcinogenesis. The roles of various bacterial infections in the development of gastric and colon cancer will be discussed later to explain the relationship of bacterial infection to DNA damage and repair inhibition.
Homologous Recombination and Non-homologous End Joining
HR and NHEJ are mechanisms to repair double strand breaks in DNA (Jackson and Bartek, 2009). These repair processes are important since double strand breaks can be extremely harmful to the genome and are largely considered the most lethal type of DNA lesions since both strands of the DNA are affected (Helleday et al., 2008). Double strand breaks can be induced by X-rays, genotoxic chemicals, during replication of single strand breaks, or by ROS (Hoeijmakers, 2001). Due to the possibility of ROS inducing double strand breaks in the DNA, bacterial infection and the resulting inflammation are implicated in this type of DNA damage. HR is present during DNA replication in the S and G2 phase of the DNA cycle while NHEJ combats direct double strand breaks that can be induced by the other factors listed above, such as X-ray or ROS exposure, and is predominant in the G1 phase of the cell cycle (Hoeijmakers, 2001; Jackson and Bartek, 2009).
In HR, the MRN sensor complex; containing MRE11 (meiotic recombination 11), RAD50, and NBS1 recruits ATM (ataxia telangiectasia mutated), generates DNA breaks followed by phosphorylation of histone H2AX (generating γH2AX) that amplifies the damage signal (Blackwood et al., 2013). Classical non-homologous end-joining (C-NHEJ) is the major pathway for DNA double strand break repair. Depletion of C-NHEJ factors significantly abrogates double strand break repair in transcribed but not in non-transcribed genes (Chakraborty et al., 2016). In NHEJ, the Ku70-Ku80 initiates NHEJ, which are the sensor proteins that recruit DNA–PK (DNA-dependent protein kinase) and end-processing proteins, followed by ligation of the breaks by a complex consisting of DNA ligase IV/XRCC4; all the end-processing enzymes (Jackson and Bartek, 2009). Mutations in proteins that repair double strand breaks are linked to higher risk for different types of cancers, predominantly lymphomas (Hoeijmakers, 2001). Interestingly, HR occurs only in cycling cells while NHEJ occurs in all cells.
Non-ROS Linked Inflammation-Associated Mechanisms for Promoting Carcinogenesis
Cancer related inflammation has been observed in most neoplastic tissues through observations of white blood cell and tumor-associated macrophage infiltration into the tumor microenvironment as well as pro-inflammatory cytokine and chemokine presence. These inflammatory factors have been linked to increased tissue remodeling and angiogenesis and are therefore stated to lead to cancer related inflammation (Balkwill and Mantovani, 2001; Colotta et al., 2009; Ostrand-Rosenberg and Sinha, 2009). Other than the intrinsic effect of cancer stimulating genomic instability induced by chronic inflammation, there are many components of an extrinsic inflammatory pathway that accelerate the further development of cancer. In this case, various pro-inflammatory cytokines, chemokines, and other inflammation-linked factors assist in tissue remodeling and angiogenesis.
For instance, inflammation activates NF-κB, which in turn activates various other inflammatory cytokines as well as angiogenic factors (Colotta et al., 2009). In the case of an acute inflammatory response of the innate immune system, the activation of NF- κB is not significant to the extent that it will affect angiogenesis and cancer progression. However, in the case of chronic inflammation, as is common in various bacterial infections such as H. pylori infection, the activation of these cytokines and factors will be consistent and significant enough to contribute to cancer development (Mantovani et al., 2008). For instance, the mediators that are downstream of NF-κB will help in neoplasia assisted by inflammation. Therefore, prolonged activation of NF-κB will promote tumor cell survival, initiation and progression of tumor tissue formation (Karin, 2006; Bollrath and Greten, 2009). In addition to NF-κB, various other inflammation-associated molecules such as IL-6 and TNF will aid in cancer progression through various mechanisms (Colotta et al., 2009). IL-6 specifically assists in tumor cell survival and growth. IL-6 is produced by myeloid-derived suppressor cells that, as suggested by the name, suppress T-cell activation. These cells are recruited to areas with chronic inflammation by pro-inflammatory mediators and are extremely influential in promoting cancer survival by allowing them to evade the immune system's attacks via T-cell activation. TNF, on the other hand, mediates inflammation and can promote the growth of a tumor by assisting in angiogenesis, epithelial to mesenchymal transition, and other mechanisms. This pro-inflammatory cytokine is associated with tumor-associated macrophages, which are also found in areas of chronic inflammation. When tumor-associated macrophages secrete TNF, the activation of Wnt/ β-catenin signaling pathway is promoted and this leads to greater tumor development (Colotta et al., 2009). The Wnt/ β-catenin pathway is a significant signaling mechanism that controls transcription for proteins involved in cell proliferation and cell fate determination (MacDonald et al., 2009). Moreover, in both T cells and ECs, an upregulation of the Wnt/β-Catenin pathway occurs upon infection, a pathway usually associated with changes in the cellular turnover rate, tissue regeneration and cellular metabolism (Karin and Clevers, 2016). The activation of microbe-sensing pathways by ECs, associated with similar gene expression changes in both ECs and IELs in immune-response-related and metabolic pathways, pointed to ECs as potential primary microbe-responding cells that could prompt neighboring IELs. Lastly, NF- κB activation leads to the production of proangiogenic factors like vascular endothelial growth factor (VEGF), which allow for the tumor to grow and spread to distal sites to result in metastasis (Ellis and Hicklin, 2008). Of the countless links between inflammation and cancer progression, only a few have been discussed here. Ultimately, however, it is clear that cancer related inflammation is a significant factor in both the initiation of cancer as well as the consecutive growth and metastasis of a tumor.
Bacterial Toxins Can Cause DNA Damage
Bacteria not only generates DNA damage but also interacts with the host DDR pathways so damage cannot be efficiently repaired. Some of the pathogenic strains of bacteria produce toxins such as Cytolethal distending toxin (cdt) and Colibactin. Cdt is present in Campylobacter jejuni, Haemophilus ducreyi, Actinobacillus actinomycetemcomitans, Shigella dysenteriae, Helicobacter cinaedi, Helicobacter hepaticus, Salmonella species. Cdts recruit the MRN complex (MRE11/Rad50/NBS1) and generate DSBs that ultimately progress to gastro-intestinal cancer (Taieb et al., 2016). Another toxin, Colibactin is a polyketide nonribosomal peptide produced by several species of Enterobacteriaceae; for example in some of the E. coli strain with pks, Klebsiella pneumoniae and Enterobacter aerogenes. Colibactin is responsible for alkylation and interstrand crosslinks of DNA followed by generation of DSBs (Nougayrède et al., 2006).
There are multiple mechanisms for bacterial infections to induce cancer, and we focused gastric cancer and colon cancer here. Further studies in this arena will give more in depth insight to the mechanisms of association between specific bacterial infections and cancers, but in the next section we will discuss the well-known and characterized bacterial-infection associated cancers especially through the lens of ROS induced DNA damage and alteration of DNA repair pathways.
Bacterial Infection-Associated With Cancers
The cumulative effect of the various processes that take place after a bacterial infection, either through the direct action of the bacterium or indirectly through bacteria-induced pathways, is linked to carcinogenesis in various tissues. Many infectious agents have previously been linked to cancers, and are implicated in about 20% of human tumors (de Martel et al., 2012). For instance, respiratory tract and lung cancers have been linked to pulmonary infections caused by Chlamydia pneumonia and Mycobacterium tuberculosis (Chaturvedi et al., 2010). Chlamydia trachomatis and Neisseria gonorrhoeae infections have been associated with genitourinary cancers, and so on (Smith et al., 2004). Of the many bacterial species that have been implicated in cancer formation, Helicobacter pylori has been shown to play a significant role in contributing to the global gastric cancer burden and has therefore been studied more thoroughly than many other species linked to cancers. Despite a clear, demonstrated link between H. pylori infection and gastric cancer incidence, the exact mechanism of carcinogenesis has yet to be discovered and characterized in depth. Fusobacterium nucleatum, on the other hand, has been observed in large amounts in the intestinal tissues of colorectal cancer patients, but a link to cancer has not been concretely established as of yet (Gagnaire et al., 2017). Although there are some contributions available on the possible mechanisms with which it may be linked to colorectal cancer, they have not been properly defined either.
Microbial Infection-Associated Mechanisms to Promote Cancer
In mammalian cells, all the above-mentioned repair mechanisms can repair damage using the DNA damage responses (DDRs) and failure in these bring DNA damage/mutation and genomic instability. Microbial infection is one of the major reasons of the failure of DDRs.
Microbes such as bacteria, virus and parasites are able to activate or alter various signaling pathways that may lead to either activation of oncogenes or down-regulation of tumor suppressor genes in a contribution to cancer progression (Francescone et al., 2014; Sheflin et al., 2014). Examples of these modifications and pathways are discussed in this section. They also modulate repair pathways as mentioned in the table that can generate mutations linked to cancer.
Helicobacter pylori infection leads to activation of PI3K-AKT pathway, which ultimately leads to degradation of tumor suppressor p53. It also contributes to cell transformation and growth by both preventing the degradation of and activating β-catenin through various bacterial effectors such as VacA (vacuolating cytotoxin A) (Tabassam et al., 2009). Similarly, a virulence factor of Fusobacterium nucleatum called Fusobacterium adhesin A (FadA) is able to bind E-cadherin to induce greater β-catenin release and ultimately activate WNT signaling, which is oncogenic (Rubinstein et al., 2013). Helicobacter pylori and Salmonella enterica serovar Typhimurium both activate MAPK and AKT signaling pathways upon infection leading to altered mediation of cell growth, proliferation, migration, and other important processes relevant to cellular transformation (Sokolova et al., 2008; Gagnaire et al., 2017).
The bacterial toxins which cells are exposed when infected can alter the cell cycle and ultimately affect some of the processes, which are implicated in carcinogenesis: proliferation, apoptosis, and differentiation (Mager, 2006). Certain bacteria, which are classified as cyclomodulins, are able to change host cell cycle patterns with cell-cycle inhibitors, such as cytolethal distending toxins and cycle inhibiting factor, and cell cycle stimulators such as cytotoxic necrotizing factor (Nougayrède et al., 2005).
Bacteria of the microbiota are also implicated in cancer through their ability to construct biofilm. Biofilms form when bacteria aggregate and secrete a substance that allows them to stick to surfaces that generally have a mucosal lining (Johnson et al., 2016). In addition to being linked to inflammatory bowel conditions, bacterial biofilms have been seen on colorectal cancers, preferentially in proximal colon cancers, which have a higher mortality rate, than distal colon cancers (Dejea et al., 2014).
H. pylori Associated Gastric Cancer
Helicobacter pylori is a gram-negative and spiral shaped bacterium. It has flagella that assist in movement and is able to survive at very low pH, making it a main colonizer of the stomach. Infection with H. pylori is associated with greater susceptibility to further infections, diarrhea, and chronic gastritis (Tomb et al., 1997; Crew and Neugut, 2006). While eradication of H. pylori infection is possible, there is high probability of relapse as well as antimicrobial resistance in many strains of the bacteria. The standard triple antibiotic treatment of H. pylori cures up to 70% of infected patients since there is a growing resistance to clarithromycin. Additionally, a very large portion of the world, about half of the total population, is exposed to or infected by H. pylori (Parsonnet et al., 1994; Malfertheiner et al., 2012). As H. pylori is the greatest risk factor for gastric cancer development, it is very important to study mechanisms of carcinogenesis upon infection in order to develop possible therapeutic and treatment options that address the specific pathways altered by H. pylori. Studies have shown that eradicating H. pylori infection decreases gastric cancer development in patients without premalignant tumors and prevents malignant transformation in patients with premalignant tumors (Wong et al., 2004; Malfertheiner et al., 2012). This further reinforces the link between infection and gastric cancer.
Non-Inflammatory Pathways for Cellular Transformation upon H. pylori Infection
H. pylori has multiple bacterial effectors that are able to alter cellular signaling pathways in favor of carcinogenesis. For instance, vacuolating cytotoxin A (VacA) and outer inflammatory protein A (OipA) are involved in epidermal growth factor receptor activation which leads to PI3K-AKT signaling and ultimately activates β-catenin (Suzuki et al., 2009; Wroblewski et al., 2010). This signaling cascade leads to transcriptional activation for cell growth. If the infection cannot be cleared, this becomes constitutively active and can then cause cellular transformation. This is just one example of how bacterial effectors can contribute to carcinogenesis in a non-inflammatory pathway. However, these effectors such as OipA are also able to induce proinflammatory cytokine expression along with other oncogenic proteins that have a significant effect on cellular transformation. Bacterial oncoproteins such as CagA are additional features of the bacteria that contribute to carcinogenesis (same reference as on line 573). The cag pathogenicity island, present in cag+ strains of H. pylori has genes which encode for type IV bacterial secretion system, commonly known as T4SS, that is able to export bacterial proteins such as CagA upon bacterial attachment to host cells. Once it enters a host epithelial cell, this protein can be activated via phosphorylation to mitigate apoptosis and induce greater cell proliferation to contribute to carcinogenesis (Polk and Peek, 2010). Despite their active role in altering many cancer-associated cellular pathways, bacterial oncoproteins and effectors are only able to increase gastric cancer risk. The ultimate development of gastric cancer also relies on chronic inflammatory response to H. pylori infection, which is accompanied by a wide variety of consequences that contribute to cellular transformation (Lamb and Chen, 2013).
Inflammation-Associated Pathways for Cellular Transformation upon H. pylori Infection
The inflammatory response to H. pylori infection can significantly alter cellular signaling and activity to induce transformation through some of the many pathways already discussed in this review (Figueiredo et al., 2002). The focus here will be on DNA damage induced by H. pylori mediated inflammation as well as obstruction of DNA repair pathways by the bacterium.
H. pylori colonization is characterized by recurring infections even after assumed eradication of the bacteria as well as chronic gastritis, which signifies a continuous inflammatory response that is started as a host response to infection but is continued with the aid of the bacteria by effectors and oncoproteins that alter chemokine and cytokine release in the infected host cells (Miftahussurur et al., 2017). One such oncoprotein is Tipα, a membrane protein secreted by H. pylori that is associated with epithelial to mesenchymal transition by activating IL-6 cytokine-dependent STAT3 signaling and greatly impacts cancer cell invasiveness. Similar to IL-6, many other inflammatory cytokines play a role in extrcellular matrix degradation to promote cell motility and angiogenesis (Chen et al., 2017).
An accumulation of DNA damage caused by chronic inflammation and resulting ROS has the potential to induce cellular transformation. In a normally functioning cell, the multiple DNA repair pathways are able to curb the accumulation of DNA mutations by addressing the damage as it occurs. In a cell infected by H. pylori, it is shown that mismatch repair (MMR), the major pathway that repairs small-scale mutations such as single base pair mismatches, is inhibited (Kim et al., 2002; Santos et al., 2017). This potentially allows for seemingly minute mutations to accumulate in oncogenic or tumor suppressor genes and lead to cancer development. Two vital MMR proteins, MSH2 and MLH1, are directly affected by H. pylori infection. Additional MMR proteins MLH1, MSH3, MSH6, PMS1, and PMS2 have also been shown to be down-regulated upon H. pylori infection of gastric cell lines AGS and BG (Machado et al., 2013; Strickertsson et al., 2014; Santos et al., 2017). The various oxidative damages induced to DNA by H. pylori infection are supposed to be repaired by the BER pathway in a normally functioning cell. In a cell infected with H. pylori, there is decreased expression of vital BER proteins. APE-1, an AP endonuclease, and YB-1, an early-stage repair protein of BER, are down-regulated upon H. pylori infection (Machado et al., 2013). Down-regulation of the DNA glycosylase OGG1, which is very important for recognition and removal of abasic sites induced by bacterial and host ROS, has been observed in gastric epithelial cells upon infection as well. Abasic sites that are targeted by OGG1, such as 8oxodG lesions, are induced at a greater frequency upon H. pylori infection. Reduced expression of OGG1 allows for accumulation of abasic sites that would not be repaired by the other DNA repair pathways and lead to carcinogenic mutation-build up and cellular transformation (Kidane et al., 2014).
Bacteria Associated Colon Cancer
The association between bacterial infection and colon cancer has not been elucidated to the extent that H. pylori induced gastric cancer has been. Certain pathogenic species such as enterotoxigenic Bacteroides fragilis and Escherichia coli strain NC101 have been linked to colitis-associated colon cancer (Wu et al., 2009; Arthur et al., 2012), but no bacterial species has been proven to be a major causative agent in colorectal carcinogenesis. Rather, a collection of gram-negative and anaerobic bacteria has been observed in colorectal tumor tissues and may serve as a marker of cancer. The colon is home to commensal bacterial species that play various supporting and valuable roles in processes such as metabolism in the host. These microbiota have also been implicated in carcinogenesis and tumor formation in the colon, potentially through bacterial dysbiosis in the gut (McCoy et al., 2013; Warren et al., 2013).
One area of interest in this field is the presence of Fusobacterium nucleatum in abundance in colorectal tumor tissues (Kostic et al., 2013). F. nucleatum is a gram-negative microbe more often associated with the oral cavity. However, the bacterial species have been identified in the early stages of cancer in colorectal adenomas as well as carcinoma samples (McCoy et al., 2013). Introduction of F. nucleatum to mice was shown to speed up colonic tumorigenesis and induce a pro-inflammatory state through NF-κB signaling (Kostic et al., 2013). F. nucleatum has also been positively correlated with mortality linked to colorectal cancer, meaning that greater abundance of the bacteria more likely resulted in mortality due to the cancer (Mima et al., 2016). There is still much to study concerning whether F. nucleatum is a causative agent in colorectal carcinogenesis, but the preliminary studies have indicated the involvement of bacteria in the induction of cancer (Ray, 2011; Kostic et al., 2013; Gagnaire et al., 2017).
In the oral cavity, F. nucleatum is a very invasive bacterial species due to its ability to adhere well to mucous surfaces (McCoy et al., 2013). In the intestines, F. nucleatum can therefore act similar to H. pylori and adhere to host cell surfaces to alter cellular pathways with its bacterial proteins. Once F. nucleatum adheres to host cells, bacterial FadA adhesin binds E-cadherin to activate β-catenin signaling to increase cell growth and proliferation as well as to regulate inflammatory response of the cell (Rubinstein et al., 2013). F. nucleatum has also been found to activate TLR4 and ultimately lead to NF-κB activation (Yang et al., 2017). Many studies have linked F. nucleatum to a pro-inflammatory state (Kostic et al., 2013; Rubinstein et al., 2013). Inflammatory cytokine gene expression such as IL-10 and TNF- α has a positive association to abundance of F. nucleatum in the colon (Rubinstein et al., 2013). This also poses the possibility of F. nucleatum contributing to carcinogenesis and tumorigenesis by inducing genetic mutations as a result of prolonged inflammation and potentially the down-regulation of various DNA repair pathways, similar to what occurs in H. pylori-mediated inflammation leading to gastric cancer.
Other bacteria associated cancers: Other that H. pylori and Fusobacterium, Salmonella typhi infection has been associated with the development of gallbladder cancer (Mager, 2006; Di Domenico et al., 2017). All other bacteria associated with cancer is mentioned in the Table 1.
Bacterial Infection in Cancer Prevention and Therapy
While a number of bacterial infections have been shown to increase the potential for carcinogenesis, recent developments in the field have provided evidence for a positive role of certain bacteria and toxins in cancer prevention and therapy (Mager, 2006). For example in one case-control study, researchers found that Helicobacter pylori infection correlated to a lower risk for esophageal cancer development (de Martel et al., 2005). Alternatively, the introduction of bacteria or its toxins to treat cancer has become a method of interest for many types of cancer. Dr. William Coley started to treat end stage cancers with a vaccine made of killed Streptococcus pyogenes and Serratia marcescens in the late 1800's to induce an initial fever followed by treatment for many different types of cancers (de Martel et al., 2005). More recently, researchers have shown that a vaccine with live attenuated Salmonella enterica serovar Typhi reduced tumor growth and enhanced survival in mice (Vendrell et al., 2011). The Bacillus Calmette-Guérin vaccine, which has a strain of Mycobacterium bovis, is used clinically for the treatment of high-risk urinary bladder cancer (Kucerova and Cervinkova, 2016). Finally, a number of bacteria species has been tried as anti-tumor agents in experimental models of cancer (Ryan et al., 2006).
Future Research Directions
About 20% of the global cancer burden is linked to infectious agents including, but not limited to, H. pylori, Hepatitis B and C virus, and Human papilloma virus (Mantovani et al., 2008; Gagnaire et al., 2017). By studying how these infectious agents can lead to and exacerbate cancer states, we may be able to prevent certain cancers from forming or advancing and identify cancer markers or therapeutic targets in the treatment of cancer. It is already known that a chronic inflammatory state is able to induce DNA damage through oxidative stress induced by ROS production. As it is a hallmark of cancer, DNA damage must be repaired properly through the multiple machineries present within the cell (Colotta et al., 2009; Hanahan and Weinberg, 2011). However, along with inducing a chronic inflammatory state, bacterial infection may affect function and/or the level of DNA repair proteins leading to a buildup of genetic mutations in potential oncogenic and tumor suppressor genes that are major contributors to carcinogenesis. Studying the effect of infectious agents that are already linked to cancers, such as F. nucleatum and colorectal cancer, on the functionality of the various DNA repair pathways, can lead to novel identification of various markers for early cancer detection as well as more effective therapies and treatments that can combat the loss in DNA repair function in host cells.
Author Contributions
All authors listed, have made substantial, direct and intellectual contribution to the work, and approved it for publication.
Conflict of Interest Statement
The authors declare that the research was conducted in the absence of any commercial or financial relationships that could be construed as a potential conflict of interest.
Acknowledgments
This work was supported, in whole or in part, by National Institute of Health Grants: DK107585 and DK099275 (to SD); R01 NS073976 (to TH).
References
Akira, S., Uematsu, S., and Takeuchi, O. (2006). Pathogen recognition and innate immunity. Cell 124, 783–801. doi: 10.1016/j.cell.2006.02.015
Almeida Pereira Leite, S. T., Marques-Guimarães, N., Silva-Oliveira, J. C., Dutra-Souto, F. J., Alves-dos-Santos, R., and Bassi-Branco, C. L. (2013). The X-ray repair cross complementing protein 1 (XRCC1) rs25487 polymorphism and susceptibility to cirrhosis in Brazilian patients with chronic viral hepatitis. Ann. Hepatol. 12, 733–739.
Arbuthnot, P., and Kew, M. (2001). Hepatitis B virus and hepatocellular carcinoma. Int. J. Exp. Pathol. 82, 77–100. doi: 10.1111/j.1365-2613.2001.iep178.x
Arthur, J. C., Perez-Chanona, E., Mühlbauer, M., Tomkovich, S., Uronis, J. M., Fan, T. J., et al. (2012). Intestinal inflammation targets cancer-inducing activity of the microbiota. Science 338, 120–123. doi: 10.1126/science.1224820
Bajpai, D., Banerjee, A., Pathak, S., Thakur, B., Jain, S. K., and Singh, N. (2016). Single nucleotide polymorphisms in the DNA repair genes in HPV-positive cervical cancer. Eur. J. Cancer Prev. 25, 224–231. doi: 10.1097/CEJ.0000000000000159
Balkwill, F., and Mantovani, A. (2001). Inflammation and cancer: back to Virchow? Lancet 357, 539–545. doi: 10.1016/S0140-6736(00)04046-0
Banerjee, D., Mandal, S. M., Das, A., Hegde, M. L., Das, S., Bhakat, K. K., et al. (2011). Preferential repair of oxidized base damage in the transcribed genes of mammalian cells. J. Biol. Chem. 286, 6006–6016. doi: 10.1074/jbc.M110.198796
Barton, G. M., and Medzhitov, R. (2003). Toll-like receptor signaling pathways. Science 300, 1524–1525. doi: 10.1126/science.1085536
Basset, C., Holton, J., O'Mahony, R., and Roitt, I. (2003). Innate immunity and pathogen-host interaction. Vaccine 21(Suppl. 2), S12–S23. doi: 10.1016/S0264-410X(03)00195-6
Blackwood, J. K., Rzechorzek, N. J., Bray, S. M., Maman, J. D., Pellegrini, L., and Robinson, N. P. (2013). End-resection at DNA double-strand breaks in the three domains of life. Biochem. Soc. Trans. 41, 314–320. doi: 10.1042/BST20120307
Bollrath, J., and Greten, F. R. (2009). IKK/NF-kappaB and STAT3 pathways: central signalling hubs in inflammation-mediated tumour promotion and metastasis. EMBO Rep. 10, 1314–1319. doi: 10.1038/embor.2009.243
Bosch, F. X., Manos, M. M., Munoz, N., Sherman, M., Jansen, A. M., Peto, J., et al. (1995). Prevalence of human papillomavirus in cervical cancer: a worldwide perspective. International biological study on cervical cancer (IBSCC) Study Group. J. Natl. Cancer Inst. 87, 796–802. doi: 10.1097/00006254-199510000-00015
Bose, S., Tripathi, D. M., Sukriti Sakhuja, P., Kazim, S. N., and Sarin, S. K. (2013). Genetic polymorphisms of CYP2E1 and DNA repair genes HOGG1 and XRCC1: association with hepatitis B related advanced liver disease and cancer. Gene 519, 231–237. doi: 10.1016/j.gene.2013.02.025
Cairns, R. A., Harris, I. S., and Mak, T. W. (2011). Regulation of cancer cell metabolism. Nat. Rev. Cancer 11, 85–95. doi: 10.1038/nrc2981
Caldecott, K. W. (2008). Single-strand break repair and genetic disease. Nat. Rev. Genet. 9, 619–631. doi: 10.1038/nrg2380
Chakraborty, A., Tapryal, N., Venkova, T., Horikoshi, N., Pandita, R. K., Sarker, A. H., et al. (2016). Classical non-homologous end-joining pathway utilizes nascent RNA for error-free double-strand break repair of transcribed genes. Nat. Commun. 7:13049. doi: 10.1038/ncomms13049
Chaturvedi, A. K., Gaydos, C. A., Agreda, P., Holden, J. P., Chatterjee, N., Goedert, J. J., et al. (2010). Chlamydia pneumoniae infection and risk for lung cancer. Cancer Epidemiol. Biomarkers Prev. 19, 1498–1505. doi: 10.1158/1055-9965.EPI-09-1261
Chen, G., Tang, N., Wang, C., Xiao, L., Yu, M., Zhao, L., et al. (2017). TNF-alpha-inducing protein of Helicobacter pylori induces epithelial-mesenchymal transition (EMT) in gastric cancer cells through activation of IL-6/STAT3 signaling pathway. Biochem. Biophys. Res. Commun. 484, 311–317. doi: 10.1016/j.bbrc.2017.01.110
Chumduri, C., Gurumurthy, R. K., Zietlow, R., and Meyer, T. F. (2016). Subversion of host genome integrity by bacterial pathogens. Nat. Rev. Mol. Cell Biol. 17, 659–673. doi: 10.1038/nrm.2016.100
Church, L. D., Cook, G. P., and McDermott, M. F. (2008). Primer: inflammasomes and interleukin 1beta in inflammatory disorders. Nat. Clin. Pract. Rheumatol. 4, 34–42. doi: 10.1038/ncprheum0681
Ciavattini, A., Piccioni, M., Tranquilli, A. L., Filosa, A., Pieramici, T., and Goteri, G. (2005). Immunohistochemical expression of DNA mismatch repair (MMR) system proteins (hMLH1, hMSH2) in cervical preinvasive and invasive lesions. Pathol. Res. Pract. 201, 21–25. doi: 10.1016/j.prp.2004.09.012
Colotta, F., Allavena, P., Sica, A., Garlanda, C., and Mantovani, A. (2009). Cancer-related inflammation, the seventh hallmark of cancer: links to genetic instability. Carcinogenesis 30, 1073–1081. doi: 10.1093/carcin/bgp127
Cong, Y., Weaver, C. T., Lazenby, A., and Elson, C. O. (2002). Bacterial-reactive T regulatory cells inhibit pathogenic immune responses to the enteric flora. J. Immunol. 169, 6112–6119. doi: 10.4049/jimmunol.169.11.6112
Coussens, L. M., and Werb, Z. (2002). Inflammation and cancer. Nature 420, 860–867. doi: 10.1038/nature01322
Crew, K. D., and Neugut, A. I. (2006). Epidemiology of gastric cancer. World J. Gastroenterol. 12, 354–362. doi: 10.3748/wjg.v12.i3.354
Cross, A. R., Noack, D., Rae, J., Curnutte, J. T., and Heyworth, P. G. (2000). Hematologically important mutations: the autosomal recessive forms of chronic granulomatous disease (first update). Blood Cells Mol. Dis. 26, 561–565. doi: 10.1006/bcmd.2000.0333
Dandona, P., Thusu, K., Cook, S., Snyder, B., Makowski, J., Armstrong, D., et al. (1996). Oxidative damage to DNA in diabetes mellitus. Lancet 347, 444–445. doi: 10.1016/S0140-6736(96)90013-6
Dejea, C. M., Wick, E. C., Hechenbleikner, E. M., White, J. R., Mark Welch, J. L., Rossetti, B. J., et al. (2014). Microbiota organization is a distinct feature of proximal colorectal cancers. Proc. Natl. Acad. Sci. U.S.A. 111, 18321–18326. doi: 10.1073/pnas.1406199111
de Martel, C., Ferlay, J., Franceschi, S., Vignat, J., Bray, F., Forman, D., et al. (2012). Global burden of cancers attributable to infections in 2008: a review and synthetic analysis. Lancet Oncol. 13, 607–615. doi: 10.1016/S1470-2045(12)70137-7
de Martel, C., and Franceschi, S. (2009). Infections and cancer: established associations and new hypotheses. Crit. Rev. Oncol. Hematol. 70, 183–194. doi: 10.1016/j.critrevonc.2008.07.021
de Martel, C., Llosa, A. E., Farr, S. M., Friedman, G. D., Vogelman, J. H., Orentreich, N., et al. (2005). Helicobacter pylori infection and the risk of development of esophageal adenocarcinoma. J. Infect. Dis. 191, 761–767. doi: 10.1086/427659
Dey, S., Maiti, A. K., Hegde, M. L., Hegde, P. M., Boldogh, I., Sarkar, P. S., et al. (2012). Increased risk of lung cancer associated with a functionally impaired polymorphic variant of the human DNA glycosylase NEIL2. DNA Repair 11, 570–578. doi: 10.1016/j.dnarep.2012.03.005
Di Domenico, E. G., Cavallo, I., Pontone, M., Toma, L., and Ensoli, F. (2017). Biofilm producing Salmonella typhi: chronic colonization and development of gallbladder cancer. Int. J. Mol. Sci. 18:1887. doi: 10.3390/ijms18091887
Ding, S. Z., O'Hara, A. M., Denning, T. L., Dirden-Kramer, B., Mifflin, R. C., Reyes, V. E., et al. (2004). Helicobacter pylori and H2O2 increase AP endonuclease-1/redox factor-1 expression in human gastric epithelial cells. Gastroenterology 127, 845–858. doi: 10.1053/j.gastro.2004.06.017
Dutta, U., Garg, P. K., Kumar, R., and Tandon, R. K. (2000). Typhoid carriers among patients with gallstones are at increased risk for carcinoma of the gallbladder. Am. J. Gastroenterol. 95, 784–787. doi: 10.1111/j.1572-0241.2000.01860.x
Ellis, L. M., and Hicklin, D. J. (2008). VEGF-targeted therapy: mechanisms of anti-tumour activity. Nat. Rev. Cancer 8, 579–591. doi: 10.1038/nrc2403
Ellmerich, S., Schöller, M., Duranton, B., Gossé, F., Galluser, M., Klein, J. P., et al. (2000). Promotion of intestinal carcinogenesis by Streptococcus bovis. Carcinogenesis 21, 753–756. doi: 10.1093/carcin/21.4.753
El-Serag, H. B. (2012). Epidemiology of viral hepatitis and hepatocellular carcinoma. Gastroenterology 142, 1264.e1–1273.e1. doi: 10.1053/j.gastro.2011.12.061
Ernst, P. (1999). Review article: the role of inflammation in the pathogenesis of gastric cancer. Aliment Pharmacol. Ther. 13(Suppl. 1), 13–18. doi: 10.1046/j.1365-2036.1999.00003.x
Farinati, F., Cardin, R., Degan, P., Rugge, M., Mario, F. D., Bonvicini, P., et al. (1998). Oxidative DNA damage accumulation in gastric carcinogenesis. Gut 42, 351–356. doi: 10.1136/gut.42.3.351
Fernandes, J. V., DE Medeiros Fernandes, T. A., DE Azevedo, J. C., Cobucci, R. N., DE Carvalho, M. G., Andrade, V. S., et al. (2015). Link between chronic inflammation and human papillomavirus-induced carcinogenesis (Review). Oncol. Lett. 9, 1015–1026. doi: 10.3892/ol.2015.2884
Figueiredo, C., Machado, J. C., Pharoah, P., Seruca, R., Sousa, S., Carvalho, R., et al. (2002). Helicobacter pylori and interleukin 1 genotyping: an opportunity to identify high-risk individuals for gastric carcinoma. J. Natl. Cancer Inst. 94, 1680–1687. doi: 10.1093/jnci/94.22.1680
Fousteri, M., and Mullenders, L. H. (2008). Transcription-coupled nucleotide excision repair in mammalian cells: molecular mechanisms and biological effects. Cell Res. 18, 73–84. doi: 10.1038/cr.2008.6
Francescone, R., Hou, V., and Grivennikov, S. I. (2014). Microbiome, inflammation, and cancer. Cancer J. 20, 181–189. doi: 10.1097/PPO.0000000000000048
Fu, X., Hu, J., Han, H. Y., Hua, Y. J., Zhou, L., Shuai, W. D., et al. (2015). High expression of XPA confers poor prognosis for nasopharyngeal carcinoma patients treated with platinum-based chemoradiotherapy. Oncotarget 6, 28478–28490. doi: 10.18632/oncotarget.4424
Gagnaire, A., Nadel, B., Raoult, D., Neefjes, J., and Gorvel, J. P. (2017). Collateral damage: insights into bacterial mechanisms that predispose host cells to cancer. Nat. Rev. Microbiol. 15, 109–128. doi: 10.1038/nrmicro.2016.171
Gulnaz, A., Sayyed, A. H., Amin, F., Khan, A., Aslam, M. A., Shaikh, R. S., et al. (2013). Association of XRCC1, XRCC3, and XPD genetic polymorphism with an increased risk of hepatocellular carcinoma because of the hepatitis B and C virus. Eur. J. Gastroenterol. Hepatol. 25, 166–179. doi: 10.1097/MEG.0b013e328359a775
Guzik, T. J., Hoch, N. E., Brown, K. A., McCann, L. A., Rahman, A., Dikalov, S., et al. (2007). Role of the T cell in the genesis of angiotensin II induced hypertension and vascular dysfunction. J. Exp. Med. 204, 2449–2460. doi: 10.1084/jem.20070657
Hajek, M., Sewell, A., Kaech, S., Burtness, B., Yarbrough, W. G., and Issaeva, N. (2017). TRAF3/CYLD mutations identify a distinct subset of human papillomavirus-associated head and neck squamous cell carcinoma. Cancer 123, 1778–1790. doi: 10.1002/cncr.30570
Hanahan, D., and Weinberg, R. A. (2011). Hallmarks of cancer: the next generation. Cell 144, 646–674. doi: 10.1016/j.cell.2011.02.013
Hanawalt, P. C., and Spivak, G. (2008). Transcription-coupled DNA repair: two decades of progress and surprises. Nat. Rev. Mol. Cell Biol. 9, 958–970. doi: 10.1038/nrm2549
Hazra, T. K., Das, A., Das, S., Choudhury, S., Kow, Y. W., and Roy, R. (2007). Oxidative DNA damage repair in mammalian cells: a new perspective. DNA Repair 6, 470–480. doi: 10.1016/j.dnarep.2006.10.011
Hazra, T. K., Izumi, T., Boldogh, I., Imhoff, B., Kow, Y. W., Jaruga, P., et al. (2002). Identification and characterization of a human DNA glycosylase for repair of modified bases in oxidatively damaged DNA. Proc. Natl. Acad. Sci. U.S.A. 99, 3523–3528. doi: 10.1073/pnas.062053799
Hegde, M. L., Hazra, T. K., and Mitra, S. (2008). Early steps in the DNA base excision/single-strand interruption repair pathway in mammalian cells. Cell Res. 18, 27–47. doi: 10.1038/cr.2008.8
Helal, T. E., Khamis, N. S., El-Sharkawy, T. M., Nada, O. H., and Radwan, N. A. (2010). Immunohistochemical expression of mismatch repair genes (hMSH2 and hMLH1) in hepatocellular carcinoma in Egypt. APMIS 118, 934–940. doi: 10.1111/j.1600-0463.2010.02658.x
Helleday, T., Petermann, E., Lundin, C., Hodgson, B., and Sharma, R. A. (2008). DNA repair pathways as targets for cancer therapy. Nat. Rev. Cancer 8, 193–204. doi: 10.1038/nrc2342
Higgs, M. R., Chouteau, P., and Lerat, H. (2014). “Liver let die”: oxidative DNA damage and hepatotropic viruses. J. Gen. Virol. 95, 991–1004. doi: 10.1099/vir.0.059485-0
Hitomi, K., Iwai, S., and Tainer, J. A. (2007). The intricate structural chemistry of base excision repair machinery: implications for DNA damage recognition, removal, and repair. DNA Repair 6, 410–428. doi: 10.1016/j.dnarep.2006.10.004
Hoeijmakers, J. H. (2001). Genome maintenance mechanisms for preventing cancer. Nature 411, 366–374. doi: 10.1038/35077232
Huang, J., Fogg, M., Wirth, L. J., Daley, H., Ritz, J., Posner, M. R., et al. (2017). Epstein-Barr virus-specific adoptive immunotherapy for recurrent, metastatic nasopharyngeal carcinoma. Cancer 123, 2642–2650. doi: 10.1002/cncr.30541
Imlay, J. A. (2008). Cellular defenses against superoxide and hydrogen peroxide. Annu. Rev. Biochem. 77, 755–776. doi: 10.1146/annurev.biochem.77.061606.161055
Jackson, S. P., and Bartek, J. (2009). The DNA-damage response in human biology and disease. Nature 461, 1071–1078. doi: 10.1038/nature08467
Jemal, A., Bray, F., Center, M. M., Ferlay, J., Ward, E., and Forman, D. (2011). Global cancer statistics. CA Cancer J. Clin. 61, 69–90. doi: 10.3322/caac.20107
Jia, L., Wang, X. W., and Harris, C. C. (1999). Hepatitis B virus X protein inhibits nucleotide excision repair. Int. J. Cancer 80, 875–879. doi: 10.1002/(SICI)1097-0215(19990315)80:6<875::AID-IJC13>3.0.CO;2-Z
Johnson, C. H., Spilker, M. E., Goetz, L., Peterson, S. N., and Siuzdak, G. (2016). Metabolite and microbiome interplay in cancer immunotherapy. Cancer Res. 76, 6146–6152. doi: 10.1158/0008-5472.CAN-16-0309
Joo, J., Yoon, K. A., Hayashi, T., Kong, S. Y., Shin, H. J., Park, B., et al. (2016). Nucleotide excision repair gene ERCC2 and ERCC5 variants increase risk of uterine cervical cancer. Cancer Res. Treat. 48, 708–714. doi: 10.4143/crt.2015.098
Karin, M. (2006). Nuclear factor-kappaB in cancer development and progression. Nature 441, 431–436. doi: 10.1038/nature04870
Karin, M., and Clevers, H. (2016). Reparative inflammation takes charge of tissue regeneration. Nature 529, 307–315. doi: 10.1038/nature17039
Kawanishi, S., Ohnishi, S., Ma, N., Hiraku, Y., Oikawa, S., and Murata, M. (2016). Nitrative and oxidative DNA damage in infection-related carcinogenesis in relation to cancer stem cells. Genes Environ. 38:26. doi: 10.1186/s41021-016-0055-7
Kidane, D., Murphy, D. L., and Sweasy, J. B. (2014). Accumulation of abasic sites induces genomic instability in normal human gastric epithelial cells during Helicobacter pylori infection. Oncogenesis 3:e128. doi: 10.1038/oncsis.2014.42
Kim, J. J., Tao, H., Carloni, E., Leung, W. K., Graham, D. Y., and Sepulveda, A. R. (2002). Helicobacter pylori impairs DNA mismatch repair in gastric epithelial cells. Gastroenterology 123, 542–553. doi: 10.1053/gast.2002.34751
Klaunig, J. E., and Kamendulis, L. M. (2004). The role of oxidative stress in carcinogenesis. Annu. Rev. Pharmacol. Toxicol. 44, 239–267. doi: 10.1146/annurev.pharmtox.44.101802.121851
Kostic, A. D., Chun, E., Robertson, L., Glickman, J. N., Gallini, C. A., Michaud, M., et al. (2013). Fusobacterium nucleatum potentiates intestinal tumorigenesis and modulates the tumor-immune microenvironment. Cell Host Microbe 14, 207–215. doi: 10.1016/j.chom.2013.07.007
Koyi, H., Brandén, E., Gnarpe, J., Gnarpe, H., and Steen, B. (2001). An association between chronic infection with Chlamydia pneumoniae and lung cancer. A prospective 2-year study. APMIS 109, 572–580. doi: 10.1034/j.1600-0463.2001.d01-177.x
Kucerova, P., and Cervinkova, M. (2016). Spontaneous regression of tumour and the role of microbial infection–possibilities for cancer treatment. Anticancer. Drugs 27, 269–277. doi: 10.1097/CAD.0000000000000337
Kunkel, T. A., and Erie, D. A. (2005). DNA mismatch repair. Annu. Rev. Biochem. 74, 681–710. doi: 10.1146/annurev.biochem.74.082803.133243
Kuper, H., Adami, H. O., and Trichopoulos, D. (2000). Infections as a major preventable cause of human cancer. J. Intern. Med. 248, 171–183. doi: 10.1046/j.1365-2796.2000.00742.x
Lamb, A., and Chen, L. F. (2013). Role of the Helicobacter pylori-induced inflammatory response in the development of gastric cancer. J. Cell. Biochem. 114, 491–497. doi: 10.1002/jcb.24389
Lambeth, J. D. (2004). NOX enzymes and the biology of reactive oxygen. Nat. Rev. Immunol. 4, 181–189. doi: 10.1038/nri1312
Langer, C. J. (2012). Exploring biomarkers in head and neck cancer. Cancer 118, 3882–3892. doi: 10.1002/cncr.26718
Leguisamo, N. M., Gloria, H. C., Kalil, A. N., Martins, T. V., Azambuja, D. B., Meira, L. B., et al. (2017). Base excision repair imbalance in colorectal cancer has prognostic value and modulates response to chemotherapy. Oncotarget 8, 54199–54214. doi: 10.18632/oncotarget.14909
Lengauer, C., Kinzler, K. W., and Vogelstein, B. (1998). Genetic instabilities in human cancers. Nature 396, 643–649. doi: 10.1038/25292
Liengswangwong, U., Karalak, A., Morishita, Y., Noguchi, M., Khuhaprema, T., Srivatanakul, P., et al. (2006). Immunohistochemical expression of mismatch repair genes: a screening tool for predicting mutator phenotype in liver fluke infection-associated intrahepatic cholangiocarcinoma. World J. Gastroenterol. 12, 3740–3745. doi: 10.3748/wjg.v12.i23.3740
Lu, R., Zhang, Y. G., and Sun, J. (2017). STAT3 activation in infection and infection-associated cancer. Mol. Cell. Endocrinol. 451, 80–87. doi: 10.1016/j.mce.2017.02.023
MacDonald, B. T., Tamai, K., and He, X. (2009). Wnt/beta-catenin signaling: components, mechanisms, and diseases. Dev. Cell 17, 9–26. doi: 10.1016/j.devcel.2009.06.016
Macdonald, T. T., and Monteleone, G. (2005). Immunity, inflammation, and allergy in the gut. Science 307, 1920–1925. doi: 10.1126/science.1106442
Machado, A. M., Desler, C., Bøggild, S., Strickertsson, J. A., Friis-Hansen, L., Figueiredo, C., et al. (2013). Helicobacter pylori infection affects mitochondrial function and DNA repair, thus, mediating genetic instability in gastric cells. Mech. Ageing Dev. 134, 460–466. doi: 10.1016/j.mad.2013.08.004
Maddocks, O. D., Scanlon, K. M., and Donnenberg, M. S. (2013). An Escherichia coli effector protein promotes host mutation via depletion of DNA mismatch repair proteins. MBio 4:e00152-13. doi: 10.1128/mBio.00152-13
Mager, D. L. (2006). Bacteria and cancer: cause, coincidence or cure? A review. J. Transl. Med. 4:14. doi: 10.1186/1479-5876-4-14
Malfertheiner, P., Megraud, F., O'Morain, C. A., Atherton, J., Axon, A. T., Bazzoli, F., et al. (2012). Management of Helicobacter pylori infection–the Maastricht IV/ Florence consensus report. Gut 61, 646–664. doi: 10.1136/gutjnl-2012-302084
Mantovani, A., Allavena, P., Sica, A., and Balkwill, F. (2008). Cancer-related inflammation. Nature 454, 436–444. doi: 10.1038/nature07205
Marshall, B. J., and Warren, J. R. (1984). Unidentified curved bacilli in the stomach of patients with gastritis and peptic ulceration. Lancet 1, 1311–1315. doi: 10.1016/S0140-6736(84)91816-6
Marshall, B. J., and Warren, J. R. (2005). The Bacterium Helicobacter pylori and Its Role in Gastritis and Peptic ulcer Disease. The Nobel Prize in Physiology or Medicine, Press Release.
Martindale, J. L., and Holbrook, N. J. (2002). Cellular response to oxidative stress: signaling for suicide and survival. J. Cell. Physiol. 192, 1–15. doi: 10.1002/jcp.10119
Martinon, F., Mayor, A., and Tschopp, J. (2009). The inflammasomes: guardians of the body. Annu. Rev. Immunol. 27, 229–265. doi: 10.1146/annurev.immunol.021908.132715
Maynard, S., Schurman, S. H., Harboe, C., de Souza-Pinto, N. C., and Bohr, V. A. (2009). Base excision repair of oxidative DNA damage and association with cancer and aging. Carcinogenesis 30, 2–10. doi: 10.1093/carcin/bgn250
McCoy, A. N., Araujo-Perez, F., Azcarate-Peril, A., Yeh, J. J., Sandler, R. S., and Keku, T. O. (2013). Fusobacterium is associated with colorectal adenomas. PLoS ONE 8:e53653. doi: 10.1371/journal.pone.0053653
Medzhitov, R. (2008). Origin and physiological roles of inflammation. Nature 454, 428–435. doi: 10.1038/nature07201
Miftahussurur, M., Yamaoka, Y., and Graham, D. Y. (2017). Helicobacter pylori as an oncogenic pathogen, revisited. Expert Rev. Mol. Med. 19:e4. doi: 10.1017/erm.2017.4
Mima, K., Nishihara, R., Qian, Z. R., Cao, Y., Sukawa, Y., Nowak, J. A., et al. (2016). Fusobacterium nucleatum in colorectal carcinoma tissue and patient prognosis. Gut 65, 1973–1980. doi: 10.1136/gutjnl-2015-310101
Molina-Cruz, A., DeJong, R. J., Charles, B., Gupta, L., Kumar, S., Jaramillo-Gutierrez, G., et al. (2008). Reactive oxygen species modulate Anopheles gambiae immunity against bacteria and Plasmodium. J. Biol. Chem. 283, 3217–3223. doi: 10.1074/jbc.M705873200
Ng, P. W., Iha, H., Iwanaga, Y., Bittner, M., Chen, Y., Jiang, Y., et al. (2001). Genome-wide expression changes induced by HTLV-1 Tax: evidence for MLK-3 mixed lineage kinase involvement in tax-mediated NF-kappaB activation. Oncogene 20, 4484–4496. doi: 10.1038/sj.onc.1204513
Nickson, C. M., Moori, P., Carter, R. J., Rubbi, C. P., and Parsons, J. L. (2017). Misregulation of DNA damage repair pathways in HPV-positive head and neck squamous cell carcinoma contributes to cellular radiosensitivity. Oncotarget 8, 29963–29975. doi: 10.18632/oncotarget.16265
Nougayrède, J. P., Homburg, S., Taieb, F., Boury, M., Brzuszkiewicz, E., Gottschalk, G., et al. (2006). Escherichia coli induces DNA double-strand breaks in eukaryotic cells. Science 313, 848–851. doi: 10.1126/science.1127059
Nougayrède, J. P., Taieb, F., De Rycke, J., and Oswald, E. (2005). Cyclomodulins: bacterial effectors that modulate the eukaryotic cell cycle. Trends Microbiol. 13, 103–110. doi: 10.1016/j.tim.2005.01.002
Ostrand-Rosenberg, S., and Sinha, P. (2009). Myeloid-derived suppressor cells: linking inflammation and cancer. J. Immunol. 182, 4499–4506. doi: 10.4049/jimmunol.0802740
Parkin, D. M. (2006). The global health burden of infection-associated cancers in the year 2002. Int. J. Cancer 118, 3030–3044. doi: 10.1002/ijc.21731
Parsonnet, J., Hansen, S., Rodriguez, L., Gelb, A. B., Warnke, R. A., Jellum, E., et al. (1994). Helicobacter pylori infection and gastric lymphoma. N. Engl. J. Med. 330, 1267–1271. doi: 10.1056/NEJM199405053301803
Pasare, C., and Medzhitov, R. (2004). Toll-like receptors and acquired immunity. Semin. Immunol. 16, 23–26. doi: 10.1016/j.smim.2003.10.006
Peltomäki, P. (2001). Deficient DNA mismatch repair: a common etiologic factor for colon cancer. Hum. Mol. Genet. 10, 735–740. doi: 10.1093/hmg/10.7.735
Polk, D. B., and Peek, R. M. Jr. (2010). Helicobacter pylori: gastric cancer and beyond. Nat. Rev. Cancer 10, 403–414. doi: 10.1038/nrc2857
Qadri, I., Fatima, K., and Abdel Hafiz, H. (2011). Hepatitis B virus X protein impedes the DNA repair via its association with transcription factor, TFIIH. BMC Microbiol. 11:48. doi: 10.1186/1471-2180-11-48
Qin, H., Zhu, J., Zeng, Y., Du, W., Shen, D., Lei, Z., et al. (2017). Aberrant promoter methylation of hOGG1 may be associated with increased risk of non-small cell lung cancer. Oncotarget 8, 8330–8341. doi: 10.18632/oncotarget.14177
Radisky, D. C., Levy, D. D., Littlepage, L. E., Liu, H., Nelson, C. M., Fata, J. E., et al. (2005). Rac1b and reactive oxygen species mediate MMP-3-induced EMT and genomic instability. Nature 436, 123–127. doi: 10.1038/nature03688
Ray, K. (2011). Colorectal cancer: Fusobacterium nucleatum found in colon cancer tissue–could an infection cause colorectal cancer? Nat. Rev. Gastroenterol. Hepatol. 8:662. doi: 10.1038/nrgastro.2011.208
Repass, J., Maherali, N., Owen, K., and Reproducibility Project: Cancer Biology. (2016). Registered report: Fusobacterium nucleatum infection is prevalent in human colorectal carcinoma. Elife 5:e10012. doi: 10.7554/eLife.10012
Reuter, S., Gupta, S. C., Chaturvedi, M. M., and Aggarwal, B. B. (2010). Oxidative stress, inflammation, and cancer: how are they linked? Free Radic. Biol. Med. 49, 1603–1616. doi: 10.1016/j.freeradbiomed.2010.09.006
Rubinstein, M. R., Wang, X., Liu, W., Hao, Y., Cai, G., and Han, Y. W. (2013). Fusobacterium nucleatum promotes colorectal carcinogenesis by modulating E-cadherin/beta-catenin signaling via its FadA adhesin. Cell Host Microbe 14, 195–206. doi: 10.1016/j.chom.2013.07.012
Ryan, R. M., Green, J., and Lewis, C. E. (2006). Use of bacteria in anti-cancer therapies. Bioessays 28, 84–94. doi: 10.1002/bies.20336
Sablina, A. A., Budanov, A. V., Ilyinskaya, G. V., Agapova, L. S., Kravchenko, J. E., and Chumakov, P. M. (2005). The antioxidant function of the p53 tumor suppressor. Nat. Med. 11, 1306–1313. doi: 10.1038/nm1320
Salim, E. I., Morimura, K., Menesi, A., El-Lity, M., Fukushima, S., and Wanibuchi, H. (2008). Elevated oxidative stress and DNA damage and repair levels in urinary bladder carcinomas associated with schistosomiasis. Int. J. Cancer 123, 601–608. doi: 10.1002/ijc.23547
Sancar, A., Lindsey-Boltz, L. A., Unsal-Kaçmaz, K., and Linn, S. (2004). Molecular mechanisms of mammalian DNA repair and the DNA damage checkpoints. Annu. Rev. Biochem. 73, 39–85. doi: 10.1146/annurev.biochem.73.011303.073723
Santos, J. C., Brianti, M. T., Almeida, V. R., Ortega, M. M., Fischer, W., Haas, R., et al. (2017). Helicobacter pylori infection modulates the expression of miRNAs associated with DNA mismatch repair pathway. Mol. Carcinog. 56, 1372–1379. doi: 10.1002/mc.22590
Sarker, A. H., Chatterjee, A., Williams, M., Lin, S., Havel, C., Jacob, P. 3rd., et al. (2014). NEIL2 protects against oxidative DNA damage induced by sidestream smoke in human cells. PLoS ONE 9:e90261. doi: 10.1371/journal.pone.0090261
Segal, A. W. (2005). How neutrophils kill microbes. Annu. Rev. Immunol. 23, 197–223. doi: 10.1146/annurev.immunol.23.021704.115653
Serhan, C. N., Chiang, N., and van Dyke, T. E. (2008). Resolving inflammation: dual anti-inflammatory and pro-resolution lipid mediators. Nat. Rev. Immunol. 8, 349–361. doi: 10.1038/nri2294
Shacter, E., and Weitzman, S. A. (2002). Chronic inflammation and cancer. Oncology 16, 217–226, 229. discussion: 230–232.
Sheflin, A. M., Whitney, A. K., and Weir, T. L. (2014). Cancer-promoting effects of microbial dysbiosis. Curr. Oncol. Rep. 16:406. doi: 10.1007/s11912-014-0406-0
Shekari, M., Sobti, R. C., Tamandani, D. M., Malekzadeh, K., Kaur, P., and Suri, V. (2008). Association of genetic polymorphism of the DNA base excision repair gene (APE-1 Asp/148 Glu) and HPV type (16/18) with the risk of cervix cancer in north Indian population. Cancer Biomark. 4, 63–71. doi: 10.3233/CBM-2008-4202
Shinmura, K., Kato, H., Kawanishi, Y., Yoshimura, K., Igarashi, H., Goto, M., et al. (2017). Reduced expression of the DNA glycosylase gene MUTYH is associated with an increased number of somatic mutations via a reduction in the DNA repair capacity in prostate adenocarcinoma. Mol. Carcinog. 56, 781–788. doi: 10.1002/mc.22509
Shuck, S. C., Short, E. A., and Turchi, J. J. (2008). Eukaryotic nucleotide excision repair: from understanding mechanisms to influencing biology. Cell Res. 18, 64–72. doi: 10.1038/cr.2008.2
Smith, J. S., Bosetti, C., Muñoz, N., Herrero, R., Bosch, F. X., Eluf-Neto, J., et al. (2004). Chlamydia trachomatis and invasive cervical cancer: a pooled analysis of the IARC multicentric case-control study. Int. J. Cancer 111, 431–439. doi: 10.1002/ijc.20257
Sohn, S., Jaitovitch-Groisman, I., Benlimame, N., Galipeau, J., Batist, G., and Alaoui-Jamali, M. A. (2000). Retroviral expression of the hepatitis B virus x gene promotes liver cell susceptibility to carcinogen-induced site specific mutagenesis. Mutat. Res. 460, 17–28. doi: 10.1016/S0921-8777(00)00010-0
Sokolova, O., Bozko, P. M., and Naumann, M. (2008). Helicobacter pylori suppresses glycogen synthase kinase 3beta to promote beta-catenin activity. J. Biol. Chem. 283, 29367–29374. doi: 10.1074/jbc.M801818200
Spencer, M. D., Hamp, T. J., Reid, R. W., Fischer, L. M., Zeisel, S. H., and Fodor, A. A. (2011). Association between composition of the human gastrointestinal microbiome and development of fatty liver with choline deficiency. Gastroenterology 140, 976–986. doi: 10.1053/j.gastro.2010.11.049
Strickertsson, J. A., Desler, C., and Rasmussen, L. J. (2014). Impact of bacterial infections on aging and cancer: impairment of DNA repair and mitochondrial function of host cells. Exp. Gerontol. 56, 164–174. doi: 10.1016/j.exger.2014.03.024
Suzuki, M., Mimuro, H., Kiga, K., Fukumatsu, M., Ishijima, N., Morikawa, H., et al. (2009). Helicobacter pylori CagA phosphorylation-independent function in epithelial proliferation and inflammation. Cell Host Microbe 5, 23–34. doi: 10.1016/j.chom.2008.11.010
Tabassam, F. H., Graham, D. Y., and Yamaoka, Y. (2009). Helicobacter pylori activate epidermal growth factor receptor- and phosphatidylinositol 3-OH kinase-dependent Akt and glycogen synthase kinase 3beta phosphorylation. Cell. Microbiol. 11, 70–82. doi: 10.1111/j.1462-5822.2008.01237.x
Taieb, F., Petit, C., Nougayrède, J. P., and Oswald, E. (2016). The enterobacterial genotoxins: cytolethal distending toxin and colibactin. EcoSal Plus 7, 1–21. doi: 10.1128/ecosalplus.ESP-0008-2016
Tal, M. C., Sasai, M., Lee, H. K., Yordy, B., Shadel, G. S., and Iwasaki, A. (2009). Absence of autophagy results in reactive oxygen species-dependent amplification of RLR signaling. Proc. Natl. Acad. Sci. U.S.A. 106, 2770–2775. doi: 10.1073/pnas.0807694106
Tomb, J. F., White, O., Kerlavage, A. R., Clayton, R. A., Sutton, G. G., Fleischmann, R. D., et al. (1997). The complete genome sequence of the gastric pathogen Helicobacter pylori. Nature 388, 539–547. doi: 10.1038/41483
Tsung, A., Klune, J. R., Zhang, X., Jeyabalan, G., Cao, Z., Peng, X., et al. (2007). HMGB1 release induced by liver ischemia involves Toll-like receptor 4 dependent reactive oxygen species production and calcium-mediated signaling. J. Exp. Med. 204, 2913–2923. doi: 10.1084/jem.20070247
Valko, M., Leibfritz, D., Moncol, J., Cronin, M. T., Mazur, M., and Telser, J. (2007). Free radicals and antioxidants in normal physiological functions and human disease. Int. J. Biochem. Cell Biol. 39, 44–84. doi: 10.1016/j.biocel.2006.07.001
van de Klundert, M. A., van Hemert, F. J., Zaaijer, H. L., and Kootstra, N. A. (2012). The hepatitis B virus x protein inhibits thymine DNA glycosylase initiated base excision repair. PLoS ONE 7:e48940. doi: 10.1371/journal.pone.0048940
van der Vliet, A. (2008). NADPH oxidases in lung biology and pathology: host defense enzymes, and more. Free Radic. Biol. Med. 44, 938–955. doi: 10.1016/j.freeradbiomed.2007.11.016
Vendrell, A., Gravisaco, M. J., Pasetti, M. F., Croci, M., Colombo, L., Rodriguez, C., et al. (2011). A novel Salmonella typhi-based immunotherapy promotes tumor killing via an antitumor Th1-type cellular immune response and neutrophil activation in a mouse model of breast cancer. Vaccine 29, 728–736. doi: 10.1016/j.vaccine.2010.11.017
Verma, S. C., Bajaj, B. G., Cai, Q., Si, H., Seelhammer, T., and Robertson, E. S. (2006). Latency-associated nuclear antigen of Kaposi's sarcoma-associated herpesvirus recruits uracil DNA glycosylase 2 at the terminal repeats and is important for latent persistence of the virus. J. Virol. 80, 11178–11190. doi: 10.1128/JVI.01334-06
Vijay-Kumar, M., Aitken, J. D., Carvalho, F. A., Cullender, T. C., Mwangi, S., Srinivasan, S., et al. (2010). Metabolic syndrome and altered gut microbiota in mice lacking Toll-like receptor 5. Science 328, 228–231. doi: 10.1126/science.1179721
Wallace, S. S., Murphy, D. L., and Sweasy, J. B. (2012). Base excision repair and cancer. Cancer Lett. 327, 73–89. doi: 10.1016/j.canlet.2011.12.038
Warren, R. L., Freeman, D. J., Pleasance, S., Watson, P., Moore, R. A., Cochrane, K., et al. (2013). Co-occurrence of anaerobic bacteria in colorectal carcinomas. Microbiome 1:16. doi: 10.1186/2049-2618-1-16
West, A. P., Brodsky, I. E., Rahner, C., Woo, D. K., Erdjument-Bromage, H., Tempst, P., et al. (2011a). TLR signalling augments macrophage bactericidal activity through mitochondrial ROS. Nature 472, 476–480. doi: 10.1038/nature09973
West, A. P., Shadel, G. S., and Ghosh, S. (2011b). Mitochondria in innate immune responses. Nat. Rev. Immunol. 11, 389–402. doi: 10.1038/nri2975
Wiseman, H., and Halliwell, B. (1996). Damage to DNA by reactive oxygen and nitrogen species: role in inflammatory disease and progression to cancer. Biochem. J. 313 (Pt 1), 17–29. doi: 10.1042/bj3130017
Wong, B. C., Lam, S. K., Wong, W. M., Chen, J. S., Zheng, T. T., Feng, R. E., et al. (2004). Helicobacter pylori eradication to prevent gastric cancer in a high-risk region of China: a randomized controlled trial. JAMA 291, 187–194. doi: 10.1001/jama.291.2.187
Wroblewski, L. E., Peek, R. M. Jr., and Wilson, K. T. (2010). Helicobacter pylori and gastric cancer: factors that modulate disease risk. Clin. Microbiol. Rev. 23, 713–739. doi: 10.1128/CMR.00011-10
Wu, C. Y., Hu, H. Y., Pu, C. Y., Huang, N., Shen, H. C., Li, C. P., et al. (2011). Pulmonary tuberculosis increases the risk of lung cancer: a population-based cohort study. Cancer 117, 618–624. doi: 10.1002/cncr.25616
Wu, S., Rhee, K. J., Albesiano, E., Rabizadeh, S., Wu, X., Yen, H. R., et al. (2009). A human colonic commensal promotes colon tumorigenesis via activation of T helper type 17 T cell responses. Nat. Med. 15, 1016–1022. doi: 10.1038/nm.2015
Xie, X., Yang, M., Ding, Y., and Chen, J. (2017). Microbial infection, inflammation and epithelial ovarian cancer. Oncol. Lett. 14, 1911–1919. doi: 10.3892/ol.2017.6388
Yang, D., Elner, S. G., Bian, Z. M., Till, G. O., Petty, H. R., and Elner, V. M. (2007). Pro-inflammatory cytokines increase reactive oxygen species through mitochondria and NADPH oxidase in cultured RPE cells. Exp. Eye Res. 85, 462–472. doi: 10.1016/j.exer.2007.06.013
Yang, Y., Weng, W., Peng, J., Hong, L., Yang, L., Toiyama, Y., et al. (2017). Fusobacterium nucleatum increases proliferation of colorectal cancer cells and tumor development in mice by activating toll-like receptor 4 signaling to nuclear factor-kappaB, and up-regulating expression of microRNA-21. Gastroenterology 152, 851.e24–866.e24. doi: 10.1053/j.gastro.2016.11.018
Ye, F., Cheng, Q., Shen, J., Zhou, C., and Chen, H. (2014). Mismatch repair gene MLH3 Pro844Leu and Thr942Ile polymorphisms and the susceptibility to cervical carcinoma and HPV infection: a case-control study in a Chinese population. PLoS ONE 9:e96224. doi: 10.1371/journal.pone.0096224
Zarember, K. A., and Godowski, P. J. (2002). Tissue expression of human Toll-like receptors and differential regulation of Toll-like receptor mRNAs in leukocytes in response to microbes, their products, and cytokines. J. Immunol. 168, 554–561. doi: 10.4049/jimmunol.168.2.554
Zhai, X., Zhao, H., Liu, Z., Wang, L. E., El-Naggar, A. K., Sturgis, E. M., et al. (2008). Functional variants of the NEIL1 and NEIL2 genes and risk and progression of squamous cell carcinoma of the oral cavity and oropharynx. Clin. Cancer Res. 14, 4345–4352. doi: 10.1158/1078-0432.CCR-07-5282
Zhong, C., Xu, M., Wang, Y., Xu, J., and Yuan, Y. (2017). An APE1 inhibitor reveals critical roles of the redox function of APE1 in KSHV replication and pathogenic phenotypes. PLoS Pathog. 13:e1006289. doi: 10.1371/journal.ppat.1006289
Zhou, R., Yazdi, A. S., Menu, P., and Tschopp, J. (2011). A role for mitochondria in NLRP3 inflammasome activation. Nature 469, 221–225. doi: 10.1038/nature09663
Keywords: bacterial infection, commensal bacteria, DNA damage, inflammation and cancer, Fusobacterium nucleatum, Helicobacter pylori
Citation: Sahan AZ, Hazra TK and Das S (2018) The Pivotal Role of DNA Repair in Infection Mediated-Inflammation and Cancer. Front. Microbiol. 9:663. doi: 10.3389/fmicb.2018.00663
Received: 03 November 2017; Accepted: 21 March 2018;
Published: 11 April 2018.
Edited by:
Chew Chieng Yeo, Sultan Zainal Abidin University, MalaysiaReviewed by:
M. Gabriela Kramer, University of the Republic, UruguayArijit Dutta, Yale University, United States
Copyright © 2018 Sahan, Hazra and Das. This is an open-access article distributed under the terms of the Creative Commons Attribution License (CC BY). The use, distribution or reproduction in other forums is permitted, provided the original author(s) and the copyright owner are credited and that the original publication in this journal is cited, in accordance with accepted academic practice. No use, distribution or reproduction is permitted which does not comply with these terms.
*Correspondence: Soumita Das, c29kYXNAdWNzZC5lZHU=