- 1Department of Microbiology and Biotechnology, Max Rubner-Institut, Kiel, Germany
- 2Department of Microbiology, Faculty of Life Science, University of Benin, Benin City, Nigeria
- 3Department of Safety and Quality of Milk and Fish, Max Rubner-Institut, Kiel, Germany
- 4Laboratory of Microbiology, Ghent University, Ghent, Belgium
Forty-seven Acinetobacter spp. isolates from milk powder obtained from a powdered milk producer in Germany were investigated for their antibiotic resistance susceptibilities, in order to assess whether strains from food harbor multiple antibiotic resistances and whether the food route is important for dissemination of resistance genes. The strains were identified by 16S rRNA and rpoB gene sequencing, as well as by whole genome sequencing of selected isolates and their in silico DNA-DNA hybridization (DDH). Furthermore, they were genotyped by rep-PCR together with reference strains of pan-European groups I, II, and III strains of Acinetobacter baumannii. Of the 47 strains, 42 were identified as A. baumannii, 4 as Acinetobacter Pittii, and 1 as Acinetobacter calcoaceticus based on 16S rRNA gene sequencing. In silico DDH with the genome sequence data of selected strains and rpoB gene sequencing data suggested that the five non-A. baumannii strains all belonged to A. pittii, suggesting that the rpoB gene is more reliable than the 16S rRNA gene for species level identification in this genus. Rep-PCR genotyping of the A. baumannii strains showed that these could be grouped into four groups, and that some strains clustered together with reference strains of pan-European clinical group II and III strains. All strains in this study were intrinsically resistant toward chloramphenicol and oxacillin, but susceptible toward tetracycline, tobramycin, erythromycin, and ciprofloxacin. For cefotaxime, 43 strains (91.5%) were intermediate and 3 strains (6.4%) resistant, while 3 (6.4%) and 21 (44.7%) strains exhibited resistance to cefepime and streptomycin, respectively. Forty-six (97.9%) strains were susceptible to amikacin and ampicillin-sulbactam. Therefore, the strains in this study were generally not resistant to the clinically relevant antibiotics, especially tobramycin, ciprofloxacin, cefepime, and meropenem, suggesting that the food route probably poses only a low risk for multidrug resistant Acinetobacter strains or resistance genes.
Introduction
Species of the genus Acinetobacter (A.) belong to the γ-Proteobacteria, and group in the order Pseudomonadales and family Moraxellaceae (Bouvet and Jeanjean, 1989). Historically, the description of the different species of the genus Acinetobacter has been problematic (Krizova et al., 2015). There are currently 42 validly published species names, which include at least one pair of synonyms (Touchon et al., 2014; Krizova et al., 2015). Previous studies have also proposed several “genomic groups” or “genospecies” (Touchon et al., 2014), which have not yet gained formal recognition in nomenclature (Krizova et al., 2015). The species Acinetobacter baumannii, Acinetobacter Nosocomialis, and Acinetobacter pittii are of clinical importance, as bacteria from these species have been isolated from a number of human infections and hospital outbreaks (Visca et al., 2011). In routine diagnostics, however, it is difficult to differentiate accurately between A. baumannii, A. pittii, A. nosocomialis, and Acinetobacter calcoaceticus strains, as these are highly similar with respect to their phenotypic and biochemical properties. Furthermore, these strains show close relationship in DNA-DNA hybridization (DDH) studies. Therefore, these have commonly been grouped together in the so-called A. calcoaceticus–A. baumannii complex (Sheng et al., 2011).
Acinetobacter baumannii is one of the six most important multi-drug resistant microorganisms in hospitals world-wide (Talbot et al., 2006; Antunes et al., 2014) and in 2005 was estimated to cause 2–10% of all Gram-negative bacterial hospital infections (Antunes et al., 2014). Multidrug-resistant A. baumannii are responsible for severe hospital-acquired infections (bloodstream, skin, soft tissue, wound, urinary tract, pulmonary, ventilator-associated pneumonia, and device-related infections) and are frequently isolated from patients hospitalized in intensive care units (Antunes et al., 2014; Potron et al., 2015). The emergence of A. baumannii as a major nosocomial pathogen has been attributed not only to specific characteristics of the organism such as multidrug resistance and its survival for long periods on surfaces and medical equipment, but also to human factors such as host health status and its association with person-to-person transmission (Medell et al., 2012; Tuon et al., 2015). The increase in antibiotic resistance of A. baumannii has consequently reduced therapeutic options for inhibition of this pathogen (Dijkshoorn et al., 2007). The bacterium is known to survive for extended length in the nosocomial setting and can even cause recurrent outbreaks of e.g., pneumonia, especially in emergency and intensive care units (Peleg et al., 2008; Ahmed et al., 2015). In Germany, a serious nosocomial outbreak with A. baumannii occurred during December 2014/January 2015 in the University Clinic Schleswig Holstein [Universitätsklinikum Schleswig-Holstein (UKSH)] in the city of Kiel, when a multiresistant A. baumannii strain infected 31 patients and resulted in several deaths, leading to a temporary closure of the intensive care unit. This strain was resistant toward penicillins, cephalosporins, carbapenems, and fluoroquinolones (Martins et al., 2014).
Antibiotics are being used for therapeutical purposes in livestock production on a relative large scale, and this has been linked to the emergence and spread of resistant bacteria from animals and animal-derived foods to people (Hamouda et al., 2011). For Acinetobacter, however, a previous study (Hamouda et al., 2011) showed that A. baumannii strains from a Scottish abattoir did not possess epidemiological characteristics that are similar to strains isolated from clinics, and the authors concluded that the A. baumannii isolates from animals investigated in that study did not appear to have evolved to strains causing hospital-acquired infections. Acinetobacter baumannii is also known to occur in animal-derived products such as bulk tank milk (Straley et al., 2006; Gurung et al., 2013; Tamang et al., 2014) and in infant milk formula (Wang et al., 2009; Miled et al., 2010; Araújo et al., 2015; Juma et al., 2016). In a report on a meeting on Cronobacter sakazakii and Salmonella in powdered infant formula, the FAO/WHO classified A. baumanii as an emerging pathogen “Category-B” microorganisms—“causality plausible, but not yet demonstrated” (FAO/WHO, 2006).
Acinetobacter spp. isolates in this study stemmed from a powdered milk production facility, which employs the roller-drying method for milk powder production. The manufacturer performs a permanent microbiological trend analysis (MTA) which primarily aims to monitor the presence of Enterobacteriaceae in the final product. In a joint research project, the Max Rubner-Institut received violet red bile dextrose agar plates (VRBD) with bacterial growth from the producer's MTA samples for further characterization. This study aimed to determine whether foodborne Acinetobacter isolates in dried milk possessed similar antibiotic resistances as the clinical strains, and to assess whether Acinetobacter strains can function as potential reservoirs of antibiotic resistance along the food transmission route and my later become problematic in a hospital setting when hosts are immunocompromised.
Materials and Methods
Strain Isolation and Culturing
All Acinetobacter spp. food isolates used in this study originated from dried milk produced via rotating drum drying by a company in Germany. Dried milk samples were taken directly from the end of the production line. Five samples of 10 g each were taken daily at various time points. Microbiological analysis for enterobacteria was done after enrichment in double buffered peptone water [peptone water 20.0 g L−l (Oxoid, Wesel, Germany), 3.5 g L−l Na2HPO4 (Merck), 1.5 g L−l KH2PO4, Merck] by plating out onto VRBD (Becton Dickinson, Heidelberg, Germany) agar medium in the laboratory of the quality control department of the producing company. Plates were surface spread in order to isolate colonies and incubated at 30°C for 24 h aerobically. VRBD plates showing bacterial growth were transferred to the Max Rubner-Institut for further isolation, characterization, and identification of the enterobacteria strains. For this, morphologically different colonies were selected for isolation (random selection). This resulted in 47 isolates from different plates, which were phenotypically characterized by Gram-staining and were presumptively identified as Acinetobacter spp. by API 20E (BioMérieux, Nürtingen, Germany), to occur amongst other enterobacteria. These strains were further characterized by genotypic fingerprinting using rep-PCR fingerprinting. In addition, five clinical A. baumannii strains obtained from the BCCM/LMG Bacteria Collection (Ghent University, Ghent, Belgium), were used as reference strains of the three pan-European genomic subgroups of A. baumannii (I, LMG 10543; II, LMG 22458, LMG 10541; III, LMG 22452, LMG 22863) (Huys et al., 2005a). All Acinetobacter strains were routinely cultured using LB medium (VWR, Darmstadt, Germany) at 37°C.
Strain Genotyping by rep-PCR
For rep-PCR fingerprinting, total genomic DNA was isolated with ZR Fungal/Bacterial DNA MiniPrep™ kit (Zymo Research, Freiburg, Germany) following the manufacturer's instruction, and the final DNA concentration was measured with a Nanodrop (Peqlab, Erlangen, Germany) and adjusted to 10 ng μL−l. The primer used was the (GTG)5 (5′-GTG GTG GTG GTG GTG-3′) primer (Huys et al., 2005a) and amplification conditions were as described previously by Huys et al. (2005a). PCR products were separated by electrophoresis on a 1.8% agarose gel using 1 X TBE (Tris-Borate-EDTA) buffer. Gels were stained in GelRed (Bio Trend, Cologne, Germany) and photographed on an UV transilluminator. Photo-positives were digitized, after which scanned images were normalized and analyzed using the Bionumerics (v. 7.1) software package (Applied Maths, Sint-Martens-Latem, Belgium). Clustering analysis of the rep-PCR fingerprints was performed by means of the Pearson product-moment correlation coefficient (r) and the unweighted pair-group method using arithmetic averages clustering algorithm (UPGMA) using Bionumerics (v. 7.1).
Strain Identification
Strains were identified by sequencing the 16S rRNA and rpoB genes. For this, the DNA of the strains was isolated with ZR Fungal/Bacterial DNA MiniPrep™ kit (Zymo Research) described above. The 16S rRNA and the rpoB genes were amplified as described previously (Kostinek et al., 2005; Higgins et al., 2010b). The PCR products were cleaned using the PCR cleaning kit from Qiagen (Hilden, Germany) and were sequenced bi-directionally at GATC Biotech (Cologne, Germany). Multiple aligned sequences were clustered and similarities were calculated with the UPGMA algorithm in Bionumerics v. 7.1. In addition, 12 strains, representative of different 16S rRNA gene sequence clusters, were selected for whole genome sequencing in order to confirm the species identities.
Antibiotic Resistance Testing
Antibiotic resistance testing was done by the Kirby-Bauer disc diffusion method (CLSI, 2015; EUCAST, 2016). For disc diffusion assays, isolates were incubated in Mueller Hinton broth (Merck, Darmstadt) at 37°C overnight and the turbidity of the fresh culture was adjusted to 0.5 McFarland scale. A 100 μL of adjusted, fresh overnight culture was plated out onto Mueller Hinton agar (Merck). After drying the plates, antibiotic discs (Oxoid, Wesel, Germany) were applied onto the surface using a dispenser. The antibiotic discs used in this study included ampicillin (AMP, 10 μg), chloramphenicol (C, 30 μg), ciprofloxacin (CIP, 5 μg), cefepime (FEP, 30 μg), cefotaxime (CTX, 5 μg), erythromycin (E, 15 μg), meropenem (MEM, 10 μg), oxacillin (OX, 5 μg), streptomycin (S, 10 μg), tetracycline (TET, 30 μg), and tobramycin (TOB, 10 μg). All plates were incubated at 35°C for 18 h as described in the CLSI (CLSI, 2015) and EUCAST (EUCAST, 2016) guidelines. After incubation, the diameter of the inhibition zone was measured and the isolates were grouped into the categories susceptible, intermediate or resistant, based on the diameter of the inhibition zone for the respective antibiotic, according to the CLSI reference (for oxacillin, streptomycin, tetracycline, chloramphenicol, ampicillin, cefotaxime, cefepime, and erythromycin) or EUCAST reference (for ciprofloxacin, meropenem, and tobramycin) for Acinetobacter spp. The inhibition zone diameters used for classifying the microorganism's antibiotic susceptibilities are shown in Table 1. All antibiotic resistance determinations were done in duplicate.
PCR Screening for Antibiotic Resistance Genes
For screening of antibiotic resistance genes, the total genomic DNA of all strains was isolated using the ZR Fungal/Bacterial DNA MiniPrep™ kit. Primers and multiplex PCR conditions for amplification of carbapenem-hydrolyzing, class D β-lactamases (CHDLs), blaOXA-23, blaOXA-24, blaOXA-51, and blaOXA-58, were as described previously (Ma et al., 2015). The primers and amplification conditions used to amplify the cat(I) and tet(A) genes were also previously described (Sianglum et al., 2009; Vilacoba et al., 2013).
Whole Genome Sequencing
The genomic DNA of Acinetobacter strains was isolated using the peqGOLD bacterial DNA kit (Peqlab, Erlangen, Germany). The sequencing library was prepared with an Illumina Nextera XT library prep kit (Illumina, San Diego, USA) and run on the MiSeq platform present in our MRI laboratory with 2 × 300 paired-ends. All obtained paired-end and unpaired-end reads were assembled de novo using SPAdes version 3.10.1 (Bankevich et al., 2012). The assembled contigs were utilized for in silico detection and typing of acquired antibiotic resistance genes, multi locus sequencing typing (MLST), as well as plasmid detection and identification using the ResFinder database (Zankari, 2014), PubMLST (Bartual et al., 2005), and PlasmidFinder (Carattoli et al., 2014) pipelines, respectively. In addition, the contigs related to plasmid sequences were extracted from whole-genome sequence data and were applied to BacMet pipeline (Pal et al., 2014) to identify antibacterial biocide and metal resistance genes. The draft genomes of three isolates clustering together with the A. pittii and A. calcoaceticus type strains in 16S rRNA and rpoB gene sequence analyzes were also used for in silico DDH together with the sequenced A. pittii PHEA-2 and A. calcoaceticus NCTC 7364 reference strains, using the web-based program at http://ggdc.dsmz.de. Whole genome comparisons of the nine sequenced A. baumannii isolates and the A. baumannii AB030 clinical reference strain were done using the Blast Ring Image Generator (BRIG) (Alikhan et al., 2011). The strains were compared especially for the localization of antibiotic resistance genes and selected virulence genes.
Results
Whole Genome Sequencing
The paired-end and unpaired-end sequence reads of the 12 selected Acinetobacter strains (9 A. baumannii and 3 A. pittii strains) were de novo assembled and generated into contigs. The N50 values ranged from 31,249 to 188,943 bp and the genome sizes of these strains were from 3.72 to 4.36 Mbp (Table 2). These genome sizes compared well to the range of sizes of between 3.70 to 4.85 Mbp reported by Wallace et al. (2016). Genes encoding virulence factors such as the genes for siderophore production, the csu operon involved in biofilm production and phospholipase D genes, as well as genes associated with efflux pump mediated antibiotic resistance such as the AcrB family efflux pump were indicated using BRIG (Figure 1). The plasmid sequences from assembled genome sequences, as well as resistance genes to metals and antibacterial biocides were identified by the BacMet web-based program and are shown in Table 3. The presence of specific virulence genes present on the chromosome of the whole genome sequenced isolates are also shown in Table 3.
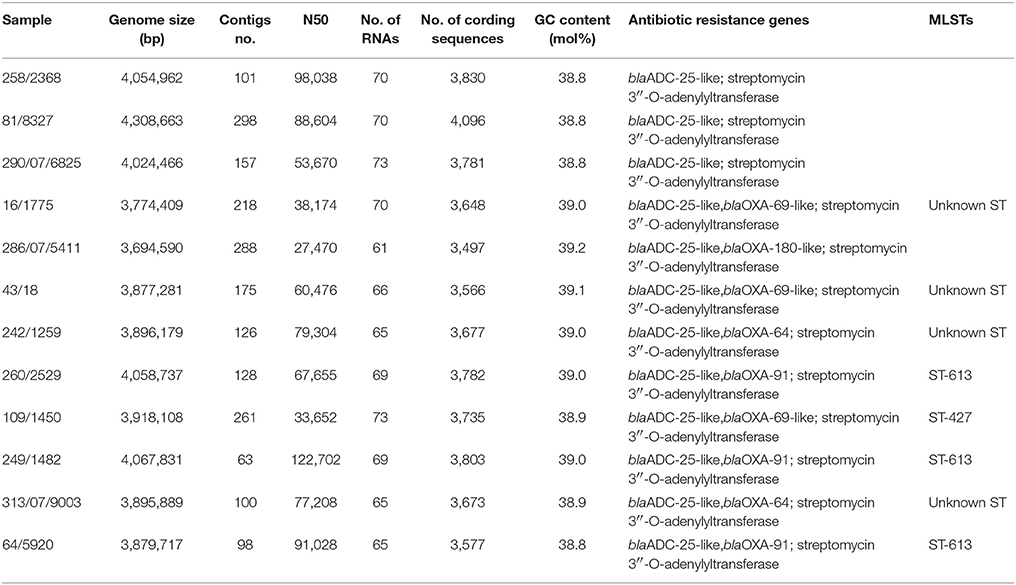
Table 2. Results of de novo genome assembly with SPAdes pipeline and in silico detection of antibiotic resistant genes and MLST of Acinetobacter baumannii/Acinetobacter pittii.
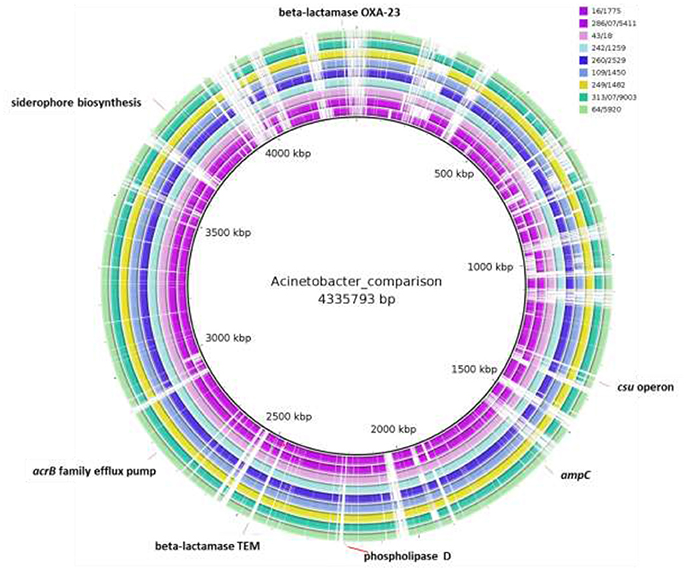
Figure 1. Whole-genome comparisons of food Acinetobacter baumannii isolates considered in this study and the A. baumannii AB030 clinical strain. From outer to inner ring: A. baumannii 64/5920, A. baumannii 43/18, A. baumannii 313/07/9003, A. baumannii 260/2529, A. baumannii 286/07/5411, A. baumannii 249/1482, A. baumannii 242/1259, A. baumannii 16/1775, A. baumannii 109/1450, reference genome: A. baumannii AB030. The loci of selected virulence genes, antibiotic resistance genes are indicated. The gaps shown indicate where the sequence data from isolates differ from the clinical strain and in these gaps the position of antibiotic resistance genes present in the clinical strain but not in the isolates (e.g., TEM-1 and OXA-23) are shown.
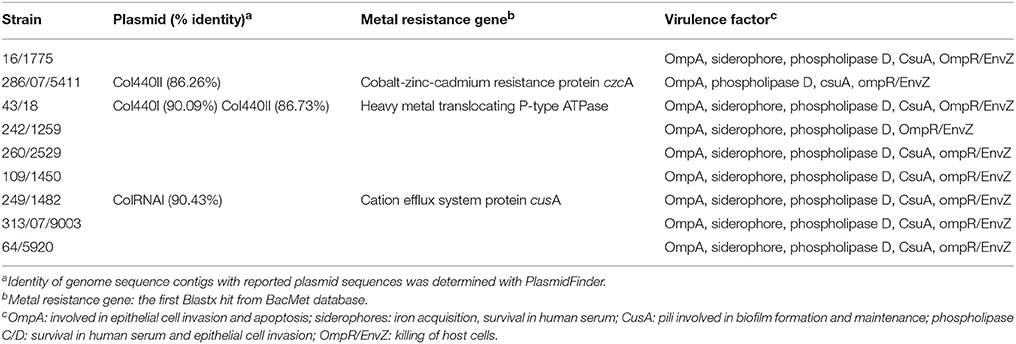
Table 3. Plasmid sequence identity, metal resistance genes, and virulence factors identified in Acinetobacter baumannii strains by genome sequencing in this study.
Strain Typing and Identification
Acinetobacter strains were characterized genotypically by rep-PCR analysis with primer (GTG)5, together with the five A. baumannii reference strains previously shown to belong to one of the three A. baumannii clonal complexes that can be discriminated by (GTG)5-PCR (Huys et al., 2005a). rep-PCR fingerprinting grouped the strains in four groups with a similarity r of ~ 75% or more (Figure 2). Thirteen strains clustered in group I at r = 77.3% and 14 isolates clustered in group II (r = 76.3%) together with the A. baumannii reference strains LMG 10541 and LMG 22458 which belong to pan-European group II (Huys et al., 2005a,b). Group III contained 10 strains which clustered at r = 81.2%, and one strain in group IV clustered together with the pan-European group III reference strains at r = 74.2%. Strain LMG 10543, representative of a pan-European clone group I, clustered apart from the other strains included in this study. The remaining 10 strains (i.e., 12/1258, 132/2279, 229/401, 25/2244, 313/07/9003, 3/700, 8/889, 116/1882, 109/1450, and 133/2516) clustered apart from all other strains.
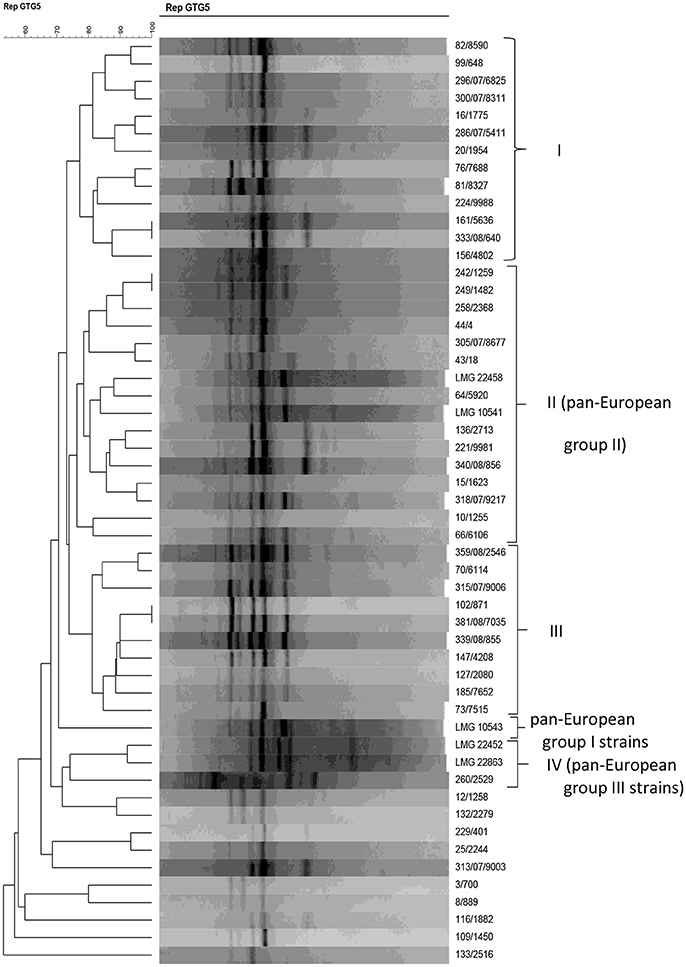
Figure 2. Dendrogram obtained by UPGMA using Pearson correlation coefficient r of digitized (GTG)5-PCR fingerprints of Acinetobacter isolates from powdered milk and reference strains.
All strains investigated with rep-PCR fingerprinting were also identified by 16S rRNA (Figure 3A) and rpoB (Figure 3B) gene sequencing. Forty-two of the 16S rRNA gene sequences of strains clustered together with the corresponding sequence of the A. baumannii type strain DSM 30007T at a high similarity of 99.4% (Figure 3A). Similar to 16S rRNA gene cluster, the rpoB gene sequences of the same 42 A. baumannii strains also clustered together with type strain (A. baumannii CIP 70.34T = A. baumannii DSM 30007T) at r = 98.2% (Figure 3B). Given the consensus between both sequenced genes, these strains were all confirmed as members of A. baumannii. Four of the remaining strains, i.e., 25/2244, 296/07/6825, 300/07/8311, and 81/8327, were characterized as A. pittii strains based on their 16S rRNA gene sequences which clustered at 100% similarity together with the corresponding gene sequence of the A. pittii DSM 25618T type strain. As for the 16S rRNA gene clustering results, these four strains grouped together with the A. pittii type strain (A. pittii CIP 70.29T = A. pittii DSM 25618T) at 99.3% similarity also in the rpoB gene cluster. In 16S rRNA gene analysis, strain 258/2368 clustered closely at r = 99.9% with the A. calcoaceticus type strain NCCB 22016T (Figure 3A), whereas based on rpoB gene sequencing this strain did not cluster with the A. calcoaceticus type strain but with the A. pittii type strain DSM 25168T (= CIP 70.29) (Figure 3B). For this reason, the genome sequences of the three strains 258/2368, 81/8327, and 296/07/6825 were used for in silico DDH together with reference strains A. calcoaceticus NCTC 7364 and A. pittii PHEA-2. The three strains showed DDH-values of ~69% when hybridized with A. pittii PHEA-2, and ~35% when hybridized with A. calcoaceticus NCTC 7364. This showed that the three strains 258/2368, 296/07/6825, and 81/8327, clustering with A. calcoaceticus CIP 81.8T and A. pittii CIP 70.29T type strains in 16S rRNA and rpoB gene sequence analyzes (Figures 3A,B), were in fact all A. pittii strains, as was suggested by the clustering analysis based on the rpoB gene sequences (Figure 3B). As the strains 300/07/8311 and 25/2244 also grouped with these sequenced strains and the A. pittii type strain in both 16S rRNA gene and rpoB gene clustering, it can de deduced that these two strains can also be identified as A. pittii.
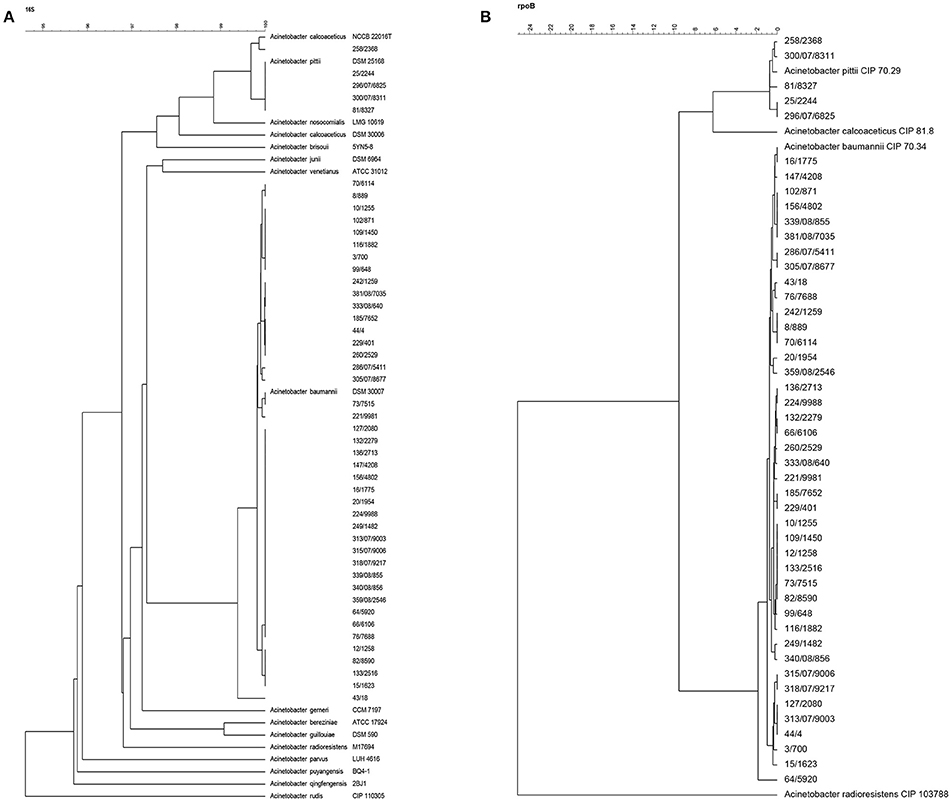
Figure 3. Phylogenetic tree based on multiple aligned sequences of Acinetobacter isolates from powdered milk. (A) 16S rRNA gene sequence analysis; (B) rpoB gene sequence analysis.
Using rep-PCR typing, the A. pittii strains 258/2368 and 81/8327, 296/07/6825, and 300/07/8311 occurred in two clusters (i.e., rep-PCR groups I and II), respectively, together with most of the A. baumannii strains. The A. pittii strain 25/2244 did not cluster into any of the rep-PCR groups. This implies that rep-PCR using the (GTG)5 primer cannot be used for successful species delineation of Acinetobacter isolates from food.
Antibiotic Resistance
All 47 strains were screened for antibiotic resistance using the disc diffusion method. Depending on the presence and size (diameter) of an inhibition zone, strains tested by this method were classified as either being susceptible, intermediate, or resistant toward an antibiotic (Table 1). The antibiotic resistance profiles of the strains from milk powder in this study are shown in Figure 3B and Table 1. All strains were resistant toward chloramphenicol (30 μg) and oxacillin (5 μg) (Table 1), but were susceptible to tetracycline, ciprofloxacin, tobramycin, and erythromycin. Testing against meropenem, amikacin, and ampicillin-sulbactam showed that 46 (97.9%) strains were susceptible to these antibiotics, while only one (2.1%) strain showed an intermediate resistance to each of these antibiotics (Figure 4, Table 1). Forty-three strains (93.8%) were susceptible toward cefepime. About half of the strains were resistant toward streptomycin (44.7%). For cefotaxime, only 3 strains (6.4%) were resistant, while 43 (91.5%) were intermediate and a single strain (2.1%) was susceptible (Figure 4, Table 1).
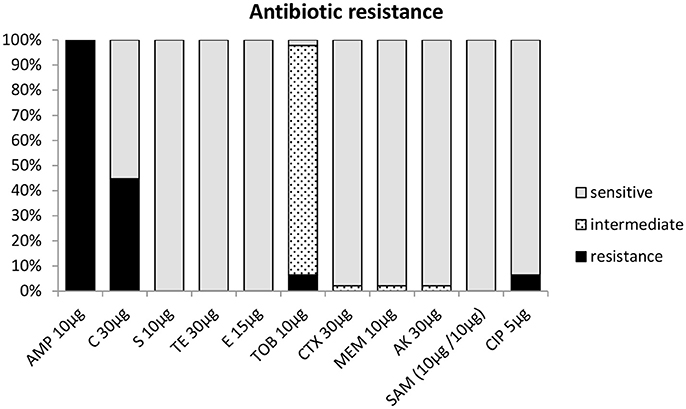
Figure 4. Antibiotic resistance profile of Acinetobacter strains based on inhibition zone diameter (mm) according to CLSI (2015) C, chloramphenicol; S, streptomycin; TET, tetracycline; E, erythromycin; FEP, cefepime; CTX, cefotaxim; AK, amikacin; SAM, ampicillin-sulbactam; and EUCAST (2016) CIP, ciprofloxacin; TOB, tobramycin; MEM, meropenem.
Conventional PCR amplification of selected antibiotic resistance genes such as blaOXA-23, blaOXA-24, blaOXA-51, and blaOXA-58 involved with imipenem and meropenem resistance were carried out. All 42 strains identified as A. baumannii were PCR-positive for blaOXA-51 family genes only. Of these, 34 strains gave a PCR product that was sequenced and showed over 99% identity to blaOXA-51 gene sequences in Genbank (results not shown). Four strains (242/1259, 44/4, 315/07/9006, and 242/1259) yielded larger size PCR products than the expected blaOXA-51 conventional PCR fragment (data not shown). These products were sequenced and they showed over 99% sequence identity with the blaOXA-530 gene, which also belongs to the blaOXA-51 family. The remaining four A. baumannii strains (249/1482, 43/18, 133/2516, and 132/2279) also gave PCR products of larger size when compared to the blaOXA-51 PCR product and were found to correspond to blaOXA-91 and blaOXA-430 genes of the blaOXA-51 family (data not shown). The five A. pittii strains were all negative for blaOXA-51.
Genome sequencing of the nine A. baumannii strains showed that these carried various bla-OXA genes which belong to the blaOXA-51 family, i.e., blaOXA-64, blaOXA 69-like, blaOXA-91, and blaOXA-180-like (Table 2). Furthermore, all strains contained a blaADC-25-like gene, which encodes an intrinsic AmpC beta-lactamase (Boo and Crowley, 2009). Interestingly, genome sequencing of the three strains identified as A. pittii showed that these strains only possessed the AmpC beta-lactamase, while no blaOXA-51-like family genes could be determined. This was in agreement with the PCR results. A BRIG comparison of the nine A. baumannii genomes with a clinical reference strain (A. baumannii ABO30 acc no: CP009257.1) showed that regions in which the important antibiotic resistance genes i.e., blaOXA23 carbapenem-hydrolyzing class D and blaTEM-1 ESBL are located, are absent in the food isolates (Figure 1). A few genome studies have previously identified a common core set of genes, including virulence genes such as phospholipase D and the csu operon (Figure 1, Table 3) and a highly variable accessory genome in A. baumannii, the latter of which includes many of the acquired antibiotic resistance genes (Chan et al., 2015; Li et al., 2015).
Discussion
Acinetobacter spp. including A. baumannii commonly occur in raw bulk tank milk (Straley et al., 2006; Gurung et al., 2013; Tamang et al., 2014), with one investigation reporting up to 7.7% of raw, bulk tank milk samples being positive for species of this genus (Gurung et al., 2013). In the current study, the majority of Acinetobacter isolates (89.4%) were identified as A. baumannii by 16S rRNA and rpoB gene sequencing, while five isolates were identified by 16S rRNA gene, rpoB gene, and in silico DDH as A. pittii. In comparison, the study of Gurung et al. (2013) reported that 57 of 176 (32.4%) Acinetobacter isolates from raw bulk tank milk were identified as A. baumannii.
In this study, most of the A. baumannii strains grouped in one of three (GTG)5—PCR fingerprint groups. Huys et al. (2005a,b) showed the value of rep-PCR with (GTG)5 primer for identifying A. baumannii and for resolving its epidemiologically successful clonal lineages [13]. These included the pan-European clone groups I and II, which were suggested to usually contain either a tet(A) or a tet(B) gene, respectively, and members of the clonal group III, which were suggested to often contain a aphA6 gene conferring resistance to aminoglycosides (Huys et al., 2005a,b). In our study, the clustering analysis of rep-PCR profiles indicated that the Acinetobacter isolates from powdered milk contained only strains representative of pan-European clonal lineages II and III (Figure 2). However, the strains investigated were all tetracycline susceptible and were not clinical isolates, as was the case for the strains described for the pan-European clone group strains in the study of Huys et al. (2005a,b). At the species level, rep-PCR with the (GTG)5 primer appeared less suitable as a taxonomic tool, given that strains identified as A. pittii, A. calcoaceticus, or A. baumannii strains using 16S rRNA and rpoB gene sequencing did not group in separate rep-PCR clusters. These results indicate also that food isolates do not necessarily group into clonal lineages like clinical isolates do and thus rep-PCR with primer GTG5 may not be appropriate for typing of food isolates by this method.
Resistance toward ß-lactam antibiotics is an important part of multidrug resistance in A. baumannii, and all four Ambler classes (A, B, C, and D) of ß-lactamase resistance can be found in this microorganism (Lin and Lan, 2014; Potron et al., 2015). All strains in this study from powdered milk were phenotypically oxacillin resistant, which is a well-known trait for A. baumannii strains. Resistance to this antibiotic is most often the result of class D β-lactamases or oxacillinases which is generally chromosomally encoded. Frequent oxacillinases involved in resistance of A. baumannii strains include OXA-23, OXA-51, and OXA-58 oxacillinases (Sahl et al., 2013), but others such as OXA-21 and OXA-37 type β-lactamases can also occur (Dijkshoorn et al., 2007; Higgins et al., 2010a; Potron et al., 2015). However, none of our isolates were resistant to meropenem. Resistance to carbapenems in Acinetobacter can be based on various mechanisms, including the expression of metallo-β-lactamases (class B-β-lactamases) such as MP-1/2/4/5 or VIM 1/2, or class D-β-lactamases OXA-23-like, OXA-24-like, OXA-51-like, or OXA-58 like carbapenemases (Dijkshoorn et al., 2007). OXA-23 mediated resistance to carbapenems is currently the largest concern posed by A. baumannii (Feng et al., 2016). The most common carrier of blaOXA-23 is ISAba1; this insertion sequence and its transposon vector help insert blaOXA-23 into the chromosome and plasmid (Feng et al., 2016). However, none of the food isolates in our study contained the OXA-23-like carbapenemase.
OXA-51-like oxacillinases are chromosomally-encoded, have low level carbapenemase activity and are widely distributed among A. baumannii strains (Wallace et al., 2016). Indeed, these could be detected in all strains from food in this study. Although it is typically a weak carbapenemase, acquiring mutations, or moving from the chromosome to a plasmid and thereby increasing the copy number, has been reported to increase activity in some isolates (Wallace et al., 2016). In addition to the blaOXA-51-like intrinsic resistance, all strains in this study whose genomes were sequenced showed that they also possessed a blaADC-25 type cephalosporinase that can degrade a variety of cephalosporins such as ceftazidime (Feng et al., 2016). Thirty-six of the A. baumannii strains showed resistance to ampicillin by disc diffusion testing. Resistance to aminopenicillins such as ampicillin has been based on blaOXA-21-like and blaOXA-37-like oxacillinase-mediated resistance, as well as narrow spectrum class A ß-lactamases such as TEM-1- or TEM-2-lactamases (Dijkshoorn et al., 2007). However, none of the strains in our study produced TEM-like beta-lactamases. The results thus showed that A. baumannii isolates from powdered milk generally showed resistance to oxacillin of the OXA-51-like type and many also to aminopenicillins, but were mostly susceptible to meropenem. Indeed, genes for carbapenemase involved with meropenem and imipenem resistance such as blaOXA-23, blaOXA-24, and blaOXA-58 could not be detected by PCR. Susceptibility toward carbapenems makes this class of antibiotics the most important to treat clinical infections. Conversely, resistance to these antibiotics dramatically lowers therapeutic options to cure infections (Fishbain and Peleg, 2010).
Most of the strains in this study were intermediate resistant toward the third generation cephalosporin cefotaxime. Cefotaxime resistance is well-known to occur in clinical A. baumannii strains (Potron et al., 2015) and has been attributed to the production of CTX-M-2 extended-spectrum class A β-lactamase. It was interesting to observe that this phenotypic (intermediate) resistance was also present in non-clinical strains which stemmed from a dried milk environment in this study. The investigated strains were all susceptible toward the fourth generation cephalosporin antibiotic cefepime.
All isolates were chloramphenicol resistant. A previous study of Roca et al. (2009) reported that most A. baumannii isolates were intrinsically resistant to chloramphenicol, and that the mechanism responsible for such resistance was identified as a major facilitator superfamily efflux pump. None of the strains were tobramycin resistant, while about half (44.7%) of the strains were streptomycin resistant. Resistance toward streptomycin and tobramycin was reported to rely on the activities of different enzymes. While tobramycin was reported to rely on modification of the antibiotic mostly by acetyltransferases [(AAC(3)-IIa, AAC(6′)-Ib, AAC(6′)-Ih, AAC(6′)-Iad)], streptomycin was reported to be modified by a nucleotidyl transferase [ANT(3″)-Ia] (Dijkshoorn et al., 2007). The 12 strains for which the complete genome was sequenced in this study all contained a streptomycin 3″-O-adenylyltransferase [(E.C. 2.7.7.47) (AAD(3″))], showing this to be a common resistance gene present in the strains investigated. However, of these 12 strains, only 8 were phenotypically resistant to streptomycin, indicating that in the 4 susceptible strains (43/18, 260/2529, 249/1482, and 64/5920) the AAD(3″) genes may either be non-functional, regulated or that other associated mechanisms for streptomycin such as non-specific pumps were present.
The use of antibiotics in livestock production has been linked to the emergence and spread of resistant bacteria from animals or from foods of animal origin to people (Hamouda et al., 2011). In a previous study, Hamouda et al. (2011) showed that A. baumannii strains from a Scottish abattoir showed different epidemiological characteristics to strains isolated from clinics, concluding that the A. baumannii isolates from animals were not the precursors of the strains causing hospital infections. The results from our studies show that some A. baumannii strains group together with pan-European group II and III clones in groups obtained by rep-PCR genotyping, even though these are not tetracycline resistant and do not stem from a clinical source.
Antibiotic-resistant strains of A. baumannii/calcoaceticus complex strains were isolated from infant milk formula in Brazil (Araújo et al., 2015) and were mainly resistant to ampicillin-sulbactam (88.2%) and cefotaxime (82.3%). Similar as in our study, only few strains were resistant to tetracycline (2/17; 11.8%), while 2 and 3 of the 17 strains were resistant to ciprofloxacin (11.8%) and tobramycin (17.6%), respectively (Araújo et al., 2015). These and other studies thus seem to indicate that A. baumannii or A. baumannii/calcoaceticus complex strains from powdered milk may exhibit single or even multiple antibiotic resistances. In contrast to clinical isolates, however, food isolates are still susceptible to clinically important antibiotics such as tobramycin, meropenem, ciprofloxacin, and cefepime. Indeed our genome comparison data showed that all nine A. baumannii food isolates sequenced did not possess chromosomal regions encoding genes for ESBL TEM-1 or high level carbapenemase. One of the main characteristics that accounts for the ability of A. baumannii to persist in the clinical setting was its ability to acquire the DNA for numerous antibiotic resistance mechanisms (Roca et al., 2012). Indeed, the acquisition of a multiply drug resistant phenotype was suggested to be a determinant factor for the success of A. baumannii as a nosocomial pathogen (Imperi et al., 2011; Antunes et al., 2014). The A. baumannii isolates from food, food environments and the associated food route thus seem to hold a relatively lower risk and are thus probably less important in terms of food safety as they are less antibiotic resistant. However, the food route may be a source for contributing new strains or lineages which appear to be inherently able to acquire antibiotic resistance determinants. When such strains enter clinical settings via the food/community route, then could result in the acquisition of new antibiotic resistance genes and the development of new problematic strains.
Author Contributions
All authors listed, have made substantial, direct and intellectual contribution to the work, and approved it for publication.
Conflict of Interest Statement
The authors declare that the research was conducted in the absence of any commercial or financial relationships that could be construed as a potential conflict of interest.
References
Ahmed, N. H., Hussain, T., and Biswal, I. (2015). Antimicrobial resistance of bacterial isolates from respiratory secretions of ventilated patients in a multi-specialty hospital. Avicenna J. Med. 5, 74–78. doi: 10.4103/2231-0770.160233
Alikhan, N. F., Petty, N. K., Ben Zakour, N. L., and Beatson, S. A. (2011). BLAST Ring Image Generator (BRIG): simple prokaryote genome comparisons. BMC Genomics 12:402. doi: 10.1186/1471-2164-12-402
Antunes, L. C., Visca, P., and Towner, K. J. (2014). Acinetobacter baumannii: evolution of a global pathogen. Pathog. Dis. 71, 292–301. doi: 10.1111/2049-632X.12125
Araújo, B. C., Moraes, M. S., Costa, L. E., and Nascimento, J. S. (2015). Short communication: multidrug-resistant Acinetobacter baumannii-calcoaceticus complex isolated from infant milk formula and utensils in a nursery in Rio de Janeiro, Brazil. J. Dairy Sci. 98, 2303–2306. doi: 10.3168/jds.2014-8825
Bankevich, A., Nurk, S., Antipov, D., Gurevich, A. A., Dvorkin, M., Kulikov, A. S., et al. (2012). SPAdes: a new genome assembly algorithm and its applications to single-cell sequencing. J. Comput. Biol. 19, 455–477. doi: 10.1089/cmb.2012.0021
Bartual, S. G., Seifert, H., Hippler, C., Luzon, M. A., Wisplinghoff, H., and Rodríguez-Valera, F. (2005). Development of a multilocus sequence typing scheme for characterization of clinical isolates of Acinetobacter baumannii. J. Clin. Microbiol. 43, 4382–4390. doi: 10.1128/JCM.43.9.4382-4390.2005
Boo, T. W., and Crowley, B. (2009). Detection of blaOXA-58 and blaOXA-23-like genes in carbapenem-susceptible Acinetobacter clinical isolates: should we be concerned? J. Med. Microbiol. 58(Pt 6), 839–841. doi: 10.1099/jmm.0.008904-0
Bouvet, P. J., and Jeanjean, S. (1989). Delineation of new proteolytic genomic species in the genus Acinetobacter. Res. Microbiol. 140, 291–299. doi: 10.1016/0923-2508(89)90021-1
Carattoli, A., Zankari, E., García-Fernández, A., Voldby Larsen, M., Lund, O., Villa, L., et al. (2014). In silico detection and typing of plasmids using PlasmidFinder and plasmid multilocus sequence typing. Antimicrob. Agents Chemother. 58, 3895–3903. doi: 10.1128/AAC.02412-14
Chan, A. P., Sutton, G., DePew, J., Krishnakumar, R., Choi, Y., Huang, X. Z., et al. (2015). A novel method of consensus pan-chromosome assembly and large-scale comparative analysis reveal the highly flexible pan-genome of Acinetobacter baumannii. Genome Biol. 16:143. doi: 10.1186/s13059-015-0701-6
CLSI (2015). M100-S25 Performance Standards for Antimicrobial Susceptibility Testing. Twenty-fifth Informational Supplement. Clinical and Laboratory Standards Institute.
Dijkshoorn, L., Nemec, A., and Seifert, H. (2007). An increasing threat in hospitals: multidrug-resistant Acinetobacter baumannii. Nat. Rev. Microbiol. 5, 939–951. doi: 10.1038/nrmicro1789
EUCAST (2016). European Committee on Antimicrobial Susceptibility Testing Breakpoint Tables for Interpretation of MICs and Zone Diameters, Version 6, valid from 2016-01-01.
FAO/WHO (2006). Microbiological Risk Assessment Series No. 10: Enterobacter sakazakii and Salmonella in Powdered Infant Formula: Meeting Report. Geneva: WHO Press.
Feng, Y., Ruan, Z., Shu, J., Chen, C. L., and Chiu, C. H. (2016). A glimpse into evolution and dissemination of multidrug-resistant Acinetobacter baumannii isolates in East Asia: a comparative genomics study. Sci. Rep. 6:24342. doi: 10.1038/srep24342
Fishbain, J., and Peleg, A. Y. (2010). Treatment of Acinetobacter infections. Clin. Infect. Dis. 51, 79–84. doi: 10.1086/653120
Gurung, M., Nam, H. M., Tamang, M. D., Chae, M. H., Jang, G. C., Jung, S. C., et al. (2013). Prevalence and antimicrobial susceptibility of Acinetobacter from raw bulk tank milk in Korea. J. Dairy Sci. 96, 1997–2002. doi: 10.3168/jds.2012-5965
Hamouda, A., Findlay, J., Al Hassan, L., and Amyes, S. G. (2011). Epidemiology of Acinetobacter baumannii of animal origin. Int. J. Antimicrob. Agents 38, 314–318. doi: 10.1016/j.ijantimicag.2011.06.007
Higgins, P. G., Dammhayn, C., Hackel, M., and Seifert, H. (2010a). Global spread of carbapenem-resistant Acinetobacter baumannii. J. Antimicrob. Chemother. 65, 233–238. doi: 10.1093/jac/dkp428
Higgins, P. G., Lehmann, M., Wisplinghoff, H., and Seifert, H. (2010b). gyrB multiplex PCR to differentiate between Acinetobacter calcoaceticus and Acinetobacter genomic species 3. J. Clin. Microbiol. 48, 4592–4594. doi: 10.1128/JCM.01765-10
Huys, G., Cnockaert, M., Nemec, A., Dijkshoorn, L., Brisse, S., Vaneechoutte, M., et al. (2005a). Repetitive-DNA-element PCR fingerprinting and antibiotic resistance of pan-European multi-resistant Acinetobacter baumannii clone III strains. J. Med. Microbiol. 54(Pt 9), 851–856. doi: 10.1099/jmm.0.45986-0
Huys, G., Cnockaert, M., Vaneechoutte, M., Woodford, N., Nemec, A., Dijkshoorn, L., et al. (2005b). Distribution of tetracycline resistance genes in genotypically related and unrelated multiresistant Acinetobacter baumannii strains from different European hospitals. Res. Microbiol. 156, 348–355. doi: 10.1016/j.resmic.2004.10.008
Imperi, F., Antunes, L. C., Blom, J., Villa, L., Iacono, M., Visca, P., et al. (2011). The genomics of Acinetobacter baumannii: insights into genome plasticity, antimicrobial resistance and pathogenicity. IUBMB Life 63, 1068–1074. doi: 10.1002/iub.531
Juma, N. A., Manning, G., and Forsythe, S. J. (2016). Desiccation survival of Acinetobacter spp. in infant formula. Food Control 68, 162–166. doi: 10.1016/j.foodcont.2016.03.043
Kostinek, M., Specht, I., Edward, V. A., Schillinger, U., Hertel, C., Holzapfel, W. H., et al. (2005). Diversity and technological properties of predominant lactic acid bacteria from fermented cassava used for the preparation of gari, a traditional African food. Syst. Appl. Microbiol. 28, 527–540. doi: 10.1016/j.syapm.2005.03.001
Krizova, L., McGinnis, J., Maixnerova, M., Nemec, M., Poirel, L., Mingle, L., et al. (2015). Acinetobacter variabilis sp. nov. (formerly DNA group 15 sensu Tjernberg & Ursing), isolated from humans and animals. Int. J. Syst. Evol. Microbiol. 65(Pt 4):1395. doi: 10.1099/ijs.0.000208
Li, H., Liu, F., Zhang, Y., Wang, X., Zhao, C., Chen, H., et al. (2015). Evolution of carbapenem-resistant Acinetobacter baumannii revealed through whole-genome sequencing and comparative genomic analysis. Antimicrob. Agents Chemother. 59, 1168–1176. doi: 10.1128/AAC.04609-14
Lin, M. F., and Lan, C. Y. (2014). Antimicrobial resistance in Acinetobacter baumannii: from bench to bedside. World J. Clin. Cases 2, 787–814. doi: 10.12998/wjcc.v2.i12.787
Ma, Z., Zhou, L., Wang, H., and Luo, L. (2015). Investigation on the genomic diversity of OXA from isolated Acinetobacter baumannii. Int. J. Clin. Exp. Med. 8, 4429–4432. doi: 10.4238/2015.November.18.36
Martins, H. S., Bomfim, M. R., França, R. O., Farias, L. M., Carvalho, M. A., Serufo, J. C., et al. (2014). Resistance markers and genetic diversity in Acinetobacter baumannii strains recovered from nosocomial bloodstream infections. Int. J. Environ. Res. Public Health 11, 1465–1478. doi: 10.3390/ijerph110201465
Medell, M., Hart, M., Marrero, O., Espinosa, F., Montes de Oca, Z., and Valdés, R. (2012). Clinical and microbiological characterization of pneumonia in mechanically ventilated patients. Braz. J. Infect. Dis. 16, 442–447. doi: 10.1016/j.bjid.2012.08.005
Miled, R. B., Neves, S., Baudouin, N., Lombard, B., Deperrois, V., Colin, P., et al. (2010). Impact of pooling powdered infant formula samples on bacterial evolution and Cronobacter detection. Int. J. Food Microbiol. 138, 250–259. doi: 10.1016/j.ijfoodmicro.2010.01.014
Pal, C., Bengtsson-Palme, J., Rensing, C., Kristiansson, E., and Larsson, D. G. (2014). BacMet: antibacterial biocide and metal resistance genes database. Nucleic Acids Res. 42(Database issue), D737–D743. doi: 10.1093/nar/gkt1252
Peleg, A. Y., Seifert, H., and Paterson, D. L. (2008). Acinetobacter baumannii: emergence of a successful pathogen. Clin. Microbiol. Rev. 21, 538–582. doi: 10.1128/CMR.00058-07
Potron, A., Poirel, L., and Nordmann, P. (2015). Emerging broad-spectrum resistance in Pseudomonas aeruginosa and Acinetobacter baumannii: mechanisms and epidemiology. Int. J. Antimicrob. Agents 45, 568–585. doi: 10.1016/j.ijantimicag.2015.03.001
Roca, I., Espinal, P., Vila-Farrés, X., and Vila, J. (2012). The Acinetobacter baumannii oxymoron: commensal hospital dweller turned pan-drug-resistant menace. Front. Microbiol. 3:148. doi: 10.3389/fmicb.2012.00148
Roca, I., Marti, S., Espinal, P., Martínez, P., Gibert, I., and Vila, J. (2009). CraA, a major facilitator superfamily efflux pump associated with chloramphenicol resistance in Acinetobacter baumannii. Antimicrob. Agents Chemother. 53, 4013–4014. doi: 10.1128/AAC.00584-09
Sahl, J. W., Gillece, J. D., Schupp, J. M., Waddell, V. G., Driebe, E. M., Engelthaler, D. M., et al. (2013). Evolution of a pathogen: a comparative genomics analysis identifies a genetic pathway to pathogenesis in Acinetobacter. PLoS ONE 8:e54287. doi: 10.1371/journal.pone.0054287
Sheng, W. H., Wang, J. T., Li, S. Y., Lin, Y. C., Cheng, A., Chen, Y. C., et al. (2011). Comparative in vitro antimicrobial susceptibilities and synergistic activities of antimicrobial combinations against carbapenem-resistant Acinetobacter species: Acinetobacter baumannii versus Acinetobacter genospecies 3 and 13TU. Diagn. Microbiol. Infect. Dis. 70, 380–386. doi: 10.1016/j.diagmicrobio.2011.03.003
Sianglum, W., Kittiniyom, K., Srimanote, P., and Wonglumsom, W. (2009). Development of multiplex PCR assays for detection of antimicrobial resistance genes in Escherichia coli and Enterococci. J. Rapid Meth. Aut. Mic. 17, 117–134. doi: 10.1111/j.1745-4581.2009.00161.x
Straley, B. A., Donaldson, S. C., Hedge, N. V., Sawant, A. A., Srinivasan, V., Oliver, S. P., et al. (2006). Public health significance of antimicrobial-resistant gram-negative bacteria in raw bulk tank milk. Foodborne Pathog. Dis. 3, 222–233. doi: 10.1089/fpd.2006.3.222
Talbot, G. H., Bradley, J., Edwards, J. E. Jr., Gilbert, D., Scheld, M., Bartlett, J. G., et al. (2006). Bad bugs need drugs: an update on the development pipeline from the antimicrobial availability task force of the infectious diseases society of America. Clin. Infect. Dis. 42, 657–668. doi: 10.1086/499819
Tamang, M. D., Gurung, M., Nam, H. M., Kim, S. R., Jang, G. C., Jung, S. C., et al. (2014). Short communication: genetic characterization of antimicrobial resistance in Acinetobacter isolates recovered from bulk tank milk. J. Dairy Sci. 97, 704–709. doi: 10.3168/jds.2013-7403
Touchon, M., Cury, J., Yoon, E. J., Krizova, L., Cerqueira, G. C., Murphy, C., et al. (2014). The genomic diversification of the whole Acinetobacter genus: origins, mechanisms, and consequences. Genome Biol. Evol. 6, 2866–2882. doi: 10.1093/gbe/evu225
Tuon, F. F., Rocha, J. L., and Merlini, A. B. (2015). Combined therapy for multi-drug-resistant Acinetobacter baumannii infection–is there evidence outside the laboratory? J. Med. Microbiol. 64, 951–959. doi: 10.1099/jmm.0.000144
Vilacoba, E., Almuzara, M., Gulone, L., Traglia, G. M., Figueroa, S. A., Sly, G., et al. (2013). Emergence and spread of plasmid-borne tet(B)::ISCR2 in minocycline-resistant Acinetobacter baumannii isolates. Antimicrob. Agents Chemother. 57, 651–654. doi: 10.1128/AAC.01751-12
Visca, P., Seifert, H., and Towner, K. J. (2011). Acinetobacter infection–an emerging threat to human health. IUBMB Life 63, 1048–1054. doi: 10.1002/iub.534
Wallace, L., Daugherty, S. C., Nagaraj, S., Johnson, J. K., Harris, A. D., and Rasko, D. A. (2016). Use of comparative genomics to characterize the diversity of Acinetobacter baumannii surveillance isolates in a health care institution. Antimicrob. Agents Chemother. 60, 5933–5941. doi: 10.1128/AAC.00477-16
Wang, M., Cao, B., Gao, Q., Sun, Y., Liu, P., Feng, L., et al. (2009). Detection of Enterobacter sakazakii and other pathogens associated with infant formula powder by use of a DNA microarray. J. Clin. Microbiol. 47, 3178–3184. doi: 10.1128/JCM.00366-09
Keywords: Acinetobacter, antibiotic resistance, food, transmission, powdered milk
Citation: Cho G-S, Li B, Rostalsky A, Fiedler G, Rösch N, Igbinosa E, Kabisch J, Bockelmann W, Hammer P, Huys G and Franz CMAP (2018) Diversity and Antibiotic Susceptibility of Acinetobacter Strains From Milk Powder Produced in Germany. Front. Microbiol. 9:536. doi: 10.3389/fmicb.2018.00536
Received: 11 January 2018; Accepted: 08 March 2018;
Published: 27 March 2018.
Edited by:
David Rodriguez-Lazaro, University of Burgos, SpainReviewed by:
Stephen Forsythe, foodmicrobe.com, United KingdomSéamus Fanning, University College Dublin, Ireland
Copyright © 2018 Cho, Li, Rostalsky, Fiedler, Rösch, Igbinosa, Kabisch, Bockelmann, Hammer, Huys and Franz. This is an open-access article distributed under the terms of the Creative Commons Attribution License (CC BY). The use, distribution or reproduction in other forums is permitted, provided the original author(s) and the copyright owner are credited and that the original publication in this journal is cited, in accordance with accepted academic practice. No use, distribution or reproduction is permitted which does not comply with these terms.
*Correspondence: Charles M. A. P. Franz, Y2hhcmxlcy5mcmFuekBtcmkuYnVuZC5kZQ==