- 1Małopolska Centre of Biotechnology, Jagiellonian University, Kraków, Poland
- 2Institute of Environmental Sciences, Jagiellonian University, Kraków, Poland
- 3Institute of Biology, Pedagogical University of Kraków, Kraków, Poland
- 4Faculty of Biochemistry, Biophysics and Biotechnology, Jagiellonian University, Kraków, Poland
Over the last years the role of fungal endophytes in plant biology has been extensively studied. A number of species were shown to positively affect plant growth and fitness, thus attempts have been made to utilize these microorganisms in agriculture and phytoremediation. Plant-fungi symbiosis requires multiple metabolic adjustments of both of the interacting organisms. The mechanisms of these adaptations are mostly unknown, however, plant hormones seem to play a central role in this process. The plant hormone strigolactone (SL) was previously shown to activate hyphae branching of mycorrhizal fungi and to negatively affect pathogenic fungi growth. Its role in the plant–endophytic fungi interaction is unknown. The effect of the synthetic SL analog GR24 on the endophytic fungi Mucor sp. growth, respiration, H2O2 production and the activity of antioxidant enzymes was evaluated. We found fungi colony growth rate was decreased in a GR24 concentration dependent manner. Additionally, the fungi accumulated more H2O2 what was accompanied by an altered activity of antioxidant enzymes. Symbiosis with Mucor sp. positively affected Arabidopsis thaliana growth, but SL was necessary for the establishment of the beneficial interaction. A. thaliana biosynthesis mutants max1 and max4, but not the SL signaling mutant max2 did not develop the beneficial phenotype. The negative growth response was correlated with alterations in SA homeostasis and a significant upregulation of genes encoding selected plant defensins. The fungi were also shown to be able to decompose SL in planta and to downregulate the expression of SL biosynthesis genes. Additionally, we have shown that GR24 treatment with a dose of 1 μM activates the production of SA in A. thaliana. The results presented here provide evidence for a role of SL in the plant–endophyte cross-talk during the mutualistic interaction between Arabidopsis thaliana and Mucor sp.
Introduction
A growing number of evidence indicates that endophytic fungi play a significant role in plant biology (Schulz, 2006; Yuan et al., 2009; Johnson et al., 2013). Endophytic fungi facilitate water and nutrient acquisition, resistance to abiotic stress such as drought, salinity and metal stress and provide protection against pathogenic microorganisms and herbivores. Their popularity is growing, for their potential in agriculture and bioremediation (Oelmüller et al., 2009; Li et al., 2012; Johnson et al., 2013). The potential for application of beneficial fungi seems very optimistic, however, understanding the mechanisms of the interactions between plants and endophytic fungi requires extensive research.
Plant adaptation to biotic and abiotic constraints requires several adjustments in plant metabolism, morphology, life cycle etc. These adaptations are often mediated by phytohormones. Recently, the plant hormone strigolactone (SL) has been recognized due to its role in root and shoot architecture determination and plant interactions in the rhizosphere (Rochange, 2010; Foo and Reid, 2013; Koltai and Kapulnik, 2013; Pandya-Kumar et al., 2014; Chen et al., 2015; van Zeijl et al., 2015). SLs are carotenoid derivates synthesized from β-carotene by consecutive action of a β-carotene isomerase, two carotenoid cleavage dioxygenases: CCD7 and CCD8 (MORE AXILARY GROWTH-MAX3 and 4) respectively and an enzyme from the P450 cytochrome family: MAX1 (MORE AXILARY GROWTH1). Downstream, a LATERAL BRANCHING OXYREDUCTASE (LBO) was recently shown to convert a carlactone intermediate in the process of SL biosynthesis (Brewer et al., 2016).
Strigolactones are important in plant responses to nutrient and water deficiency (López-Ráez et al., 2008; Yoneyama et al., 2012; Foo et al., 2013; López-Ráez, 2016; Visentin et al., 2016). SL biosynthesis mutants max3 and max4 and the SL signaling mutant max2 are more sensitive to drought, due to a relationship between SL and ABA (Bu et al., 2014; Ha et al., 2014). Similarly, osmotic stress had a more severe effect on the Lotus japonicus SL biosynthesis mutant: Ljccd7 (Liu et al., 2015). Nitrogen and phosphorus starvation activated SL biosynthesis and exudation and available reports indicate that SL plays a role in plant adaptation to P deficiency (Umehara et al., 2008; Kohlen et al., 2011; Ruyter-Spira et al., 2011; Andreo-Jimenez et al., 2015). Symbiotic microorganisms including endophytic fungi facilitate adaptation to environmental challenges including nutrient deficiencies and drought (reviewed in Bacon and White, 2016).
Strigolactones are signaling molecules involved in plant-soil microorganism interactions (reviewed in López-Ráez, 2016; Waters et al., 2017). The best described is its action in the plant–AMF interaction. In this mutual relationship the fungus provides the plant with necessary nutrients in exchange for reduced carbon. The AMF symbiosis is widespread throughout the plant kingdom; according to available reports, the roots of over 80% of terrestrial plants are colonized by AMF (Smith and Read, 2008; Brundrett, 2009). Only a few plant families, including the Brassicaceae have lost the ability to engage in mutual symbiosis with AMF (reviewed in Venkateshwaran et al., 2013). Nevertheless, recent reports indicate that numerous members of this family harbor a wide variety of fungal symbionts, including beneficial endophytic fungi that may play a similar role in plant physiology as AMF (Barzanti et al., 2007; García et al., 2013; Card et al., 2015; Hong et al., 2015). This also allows to study the mechanisms of symbiosis with well-established plant models such as Arabidopsis thaliana, Thlaspi caerulescens etc.
Strigolactones are secreted from plant roots into the rhizosphere and act as a signal for directional growth of the hyphae, thus SL seems to facilitate the plant-AMF interaction in the pre-symbiotic stage of symbiosis (Akiyama et al., 2005; Besserer et al., 2006; Kretzschmar et al., 2012; Mori et al., 2016). Fungal mycelium treated with synthetic SL analogs exhibits a number of changes such as: hyphal branching and growth, increased respiratory activity and ATP and NADPH production, mitosis, expression of effector genes and spore germination. Additionally, SL treatment activates synthesis and release of short chain chitin oligomers which can activate the symbiotic (SYM) signaling pathway, which in turn trigger symbiotic responses in the plant (Lopez-Raez et al., 2017). According to studies with SL-biosynthesis and SL-exudation mutants of pea, petunia, rice and tomato, SL was not necessary for the establishment of the plant-AMF symbiosis, however, the colonization rate of these mutants was much lower compared to wild type plants (Gomez-Roldan et al., 2008; López-Ráez et al., 2008; Vogel et al., 2009; Koltai et al., 2010; Gutjahr et al., 2012; Kretzschmar et al., 2012). The response of different AMF species differs in respect to various SL molecules, however, doses as low as 10 nM of GR24 were shown to affect the growth pattern of the fungi (Besserer et al., 2008). Even though significant progress has been made in elucidating SL signaling and perception in A. thaliana and rice, the mechanism of SL perception nor signaling are not known in fungi. In A. thaliana the α/β hydrolase D14 was recognized as a SL receptor. SL signaling is mediated by the MAX2 (MORE AXILARY GROWTH2)/SMAXL (SUPRESOR OF MAX2 6, 7, and 8 in particular) signal transduction pathway, but no clear MAX2 or D14 homologs were found in sequenced fungal genomes including Rhizophagus irregularis (Waters et al., 2017).
Strigolactones are also involved in other interactions in the rhizosphere: act as a signal for rhizobacteria, as stimulants of parasitic plant seed (Striga sp. and Orobanche sp.) and pathogenic fungi (Rochange, 2010; Foo and Reid, 2013; Koltai and Kapulnik, 2013). The role of SL in plant–pathogenic fungi interactions is not clear. There are several, contradictory reports. A range of responses to the synthetic SL analog-GR24 on pathogenic fungi growth and branching were reported for the same species (Dor et al., 2011; Torres-Vera et al., 2014; Foo et al., 2016). Recently, Belmondo et al. (2017) has shown that a thioredoxin reductase is necessary for limiting Botrytis cinerea growth by GR24, indicating a relationship between SL and ROS (reactive oxygen species) metabolism.
The role of SL in biotic stress responses may be associated with its interaction with other phytohormones or hormone dependent signaling. In the SL deficient tomato, slccd8, reduced concentration of ABA, SA and JA were shown (Torres-Vera et al., 2014). In response to the parasitic plant Phelipanche ramosa, the expression of SL biosynthetic D27 and CCD8 and SA, JA and ABA marker genes was upregulated (Torres-Vera et al., 2016). The SL signaling mutant max2 was more susceptible to Pectobacterium carotovorum and Pseudomonas syringe, probably due to alterations in ABA metabolism (Piisilä et al., 2015). However, studies performed on SL biosynthesis and signaling garden pea mutants contradict these reports, showing no increased sensitivity to infection by the necrotrophic soilborne oomycete Pythium irregulare (Foo et al., 2016).
Previously, the endophytic fungus Mucor sp. was found to accelerate Arabidopsis arenosa and A. thaliana growth (Rozpądek et al., 2017). The fungus was also shown to improve A. arenosa toxic metal tolerance (Rozpądek et al., 2017). Other members of this genus improved oilseed rape growth in heavily polluted environments (Zhu et al., 2015; Zahoor et al., 2017) As recently suggested by Martin and Plett (2015), the closely related with AMF Mucoromycetes associated with extant, basal land plants, such as liverworts, hornworts and lycopods, in a symbiosis whose mutualistic nature is suspected, making this group of fungi a good model for studying the mechanisms of symbiosis.
In this study, we evaluated the role of SL in the interaction between A. thaliana and its fungal symbiont Mucor sp. It was hypothesized that SL is necessary in the development of mutualism between the two interacting organisms both as a secretory signal adjusting the Mucor sp. metabolism, to the mutualistic mode and a plant, intrinsic regulatory molecule. Its role was assumed to be associated with its connection to SA synthesis or signaling. Additionally, the possibility that the fungi may have an effect on SL metabolism after colonization was tested.
Materials and Methods
Plant Cultivation
Arabidopsis thaliana WT (N6000), max1 (N9564), max4-1 (N9568) and max2-2 (N9566) (more axillary branches 1, 4 and 2) mutants (all in Col-0 background) were obtained from NASC (The Nottingham Arabidopsis Stock Centre, United Kingdom). Seeds were surface sterilized with 8% NaOCl, 96% and 75% EtOH and sown to sterile ¼ MS medium in a petri dish and placed in darkness (4°C). After 48 h seeds were transferred to a growth chamber (Panasonic MLR-352H-PE, JP) with a 16 h photoperiod, 21/17°C day/night temperature and 50% humidity. After 10 days seedlings were moved to MSR medium with no sugar (10 plants per petri dish) and inoculated with the fungus. To evaluate the effect of SL on plant biomass yield, MSR was supplemented with 1 μM of the synthetic SL analog: GR24 (StrigoLab, I) in acetone. Inoculation of in vitro cultures was performed by placing 2.1 × 106 Mucor sp. spores 5 mm from the tip of the main root. After 10–12 days of growth plants were harvested, frozen in liquid nitrogen and stored at -80°C. For biomass yield evaluation 3 separate experiments with 25–30 plants were performed. Due to differences in plant growth in between experiments, fresh weight of treated plants (E+, GR24 and E+GR24) was presented in relation to appropriate control. In all GR24 feeding experiments acetone mock control was performed.
Strigolactone Feeding Experiments, Fungi Growth, Respiration
Mucor sp. (KU234656, strain UNIJAG.PL.E50) spores (2.1 × 106) were placed in PDA (potato dextrose agar) medium supplemented with GR24. For colony growth evaluation spores were inoculated onto PDA containing 1, 10, 50, 100, 500, and 1000 nM of GR24 in 9 cm petri dishes. Colony surface area was measured after 48 h of growth in 24°C in darkness. Fungi respiration was measured with a 30 channel Micro-oxymax respirometer (Columbus Instruments, United States) between the 24 and 48 h of growth. A single O2 measurement was performed every 2 h. Spores were placed in PDA supplemented with 50, 500, and 1000 nM of GR24 in 250 ml Duran bottles in the darkness at 24°C (growth chamber of Memmert, IPP400, United States). Fungi respiration was measured as O2 consumption per 2 h for 24 h. The respiration rate was presented in relation to colony diameter. The experiment was run in 5 replicates. For all experiments mock (acetone) treated control was performed.
Enzyme Activity Assays
Protein Extraction and Quantification
Mucor sp. colonies grown in PDA supplemented with 1, 10, 50, 100, and 1000 nM of GR24 were harvested from media after 48 h of growth and grounded with a mortar and pestle in liquid nitrogen. For crude protein extraction, powdered mycelia were homogenized with molybdenum beads in a TissueLyzer LT (Qiagen, DE) in ice cold 100 mM HEPES-NaOH buffer (pH 7.5, 4 mM DTT, 1 mM EDTA) at 35 Hz for 15 min. The homogenizer adapter was precooled in liquid nitrogen to keep samples frozen. After extraction, samples were centrifuged for 10 min at 10,000 g at 4°C. Protein content was quantified according to Bradford (1976) using BSA as a standard. A separate set of fungi mycelium was prepared for each enzyme activity assay. The experiment was run in 5 replicates. For all experiments mock (acetone) treated control was performed.
Catalase Activity
The spectrophotometric measurement was performed according to the modified method described by Aebi (1984). Crude tissue extracts (10 μl) were added to 990 μl of phosphate buffer pH 7.0 containing 3 mM H2O2. CAT activity was determined from the decrease in absorbance at 240 nm due to CAT dependent reduction of H2O2. Enzyme activity was defined as 1 μmol of H2O2 decomposed by 1 mg of total soluble proteins per minute.
Glutathione Reductase Activity
The supernatants were analyzed for GR activity according to the modified method described by Foyer et al. (1995). Enzyme activity was determined from the decrease in absorbance at 340 nm in the reaction mixture containing TRIS-HCl (50 mM, pH 7.5) buffer, EDTA (1 mM) and GSSG (0.5 mM) in a total volume of 1 ml. Reaction was initiated with the addition of 0.15 mM NADPH.
Superoxide Dismutase Activity
Separations of soluble protein fractions were performed using native non-continuous PAGE in the buffer system described by Laemmli (1970) at 4°C and 180 V. SOD bands on 12% polyacrylamide gels were visualized according to the staining procedure described by Beauchamp and Fridovich (1971). The gels were incubated in the staining buffer for 30 min, in darkness, at room temperature and subsequently exposed to white light until SOD activity bands became visible. The gels were scanned using the office scanner Epson V700 Photo, and densitometric analysis was performed with ImageJ (NIH, United States).
Determination of H2O2 Concentration
For H2O2 assay powdered mycelia were homogenized with molybdenum beads in a TissueLyzer LT (Qiagen, DE) in ice cold 50 mM phosphate buffer pH 7.0 and at 40 Hz for 15 min. The homogenizer’s adapter was precooled in liquid nitrogen to keep frozen. After extraction, samples were centrifuged for 15 min at 13,000 g at 4°C. H2O2 was assayed with Amplex® Red Hydrogen Peroxide Kit (Invitrogen) according to the manufacturer’s instructions. The experiment was run in 5 replicates.
Fungi Staining, Confocal Microscopy and Plant Colonization Assessment
Plant colonization by the fungus was assessed according to Domka et al. (under review), by comparing the expression of the fungal TEF1α (Translation elongation factor 1-alpha) with plant ACT7 (Actin-7) with qPCR. To visualize mycelium in planta GFP-expressing strain of Mucor sp. (KU234656, strain UNIJAG.PL.E50) was generated (Domka et al., under review). Visualization was performed with a confocal microscopy (Nikon Eclipse, JP) equipped with GFP filter blocks.
SL Decomposition Assay
The ability of the fungi to decompose SL in planta was verified by transferring 10 day old seedlings from ccc MS medium to MSR supplemented with 1 μM GR24 fluorescent analog: GR24-BODIPY (StrigoLab, I) for 24 h to allow the plant to uptake it. Subsequently, seedlings were transferred to fresh MSR and inoculated with Mucor sp. Plants were harvested after 48 h of growth. Visualization of the fluorescence signal was performed with confocal microscopy (Nikon Eclipse, JP). Fluorescence was excited by 490 nm The fluorescence signal intensity was measured with ImageJ (NIH, United States). Five petri dishes with 10 seedlings for treated and not treated plants were prepared.
Salicylic Acid and Jasmonic Acid Biosynthesis Induction
To test the relationship between SL and SA and JA production, A. thaliana 10 day old seedlings grown in MS medium were transferred to MSR supplemented with 0, 1, 10, 50, 100, 500, 1000 nM of GR24 and harvested after 10 days of vegetation. To evaluate the impact of the fungi on SA production in SL treated plants, A. thaliana seedlings were transferred from MS to MSR supplemented with 1 μM GR24 and simultaneously inoculated with Mucor sp. (as described in “Plant cultivation”). The temporal pattern of SA production dynamics was evaluated with E+ seedlings grown in medium supplemented with GR24 for 1, 2, 5, and 10 days.
Salicylic Acid and Jasmonic Acid Concentration Measurement
Salicylic acid concentration was measured 1, 2, 5, and 10 days after transferring seedlings to MSR supplemented with 1 μM GR24. Inoculation was performed simultaneously with transfer. Unlabeled SA was purchased from Sigma-Aldrich (D). Sample preparation and HPLC analysis were carried out according to Müller et al. (2011) with modifications. Frozen plant roots (about 200 mg Fw – 30 plants per sample) was powdered in liquid nitrogen with a metal pestle in polypropylene tubes and then extracted with methanol:isopropanol:glacial acetic acid (20:79:1; v/v/v) in a 10:1 v/w ratio for 20 min in 4°C. During extraction sonification was applied. Subsequently, samples were centrifuged for 20 min in 15000 g. This procedure was performed 5 times to assure maximum, close to 100% extraction (from the second extraction 1 ml of extraction solution was used).
The HPLC analysis was performed using Shimadzu LCMS-2020 (JP) system equipped with an autosampler. Separation of plant extracts was performed with a Kinetex 2.6u C18 100x2.1 mm column. The total eluent flow was 0.400 ml min-1. Gradient profile described in Müller et al. (2011). The MS analysis was performed using quadrupole mass spectrometer (Shimadzu) negative mode. The following MS parameters were used for analysis: DL temp. 250°C, HB temp. 200°C, detector voltage 0.95 kV, oven temp. 35°C, nebulizing gas flow 15 l min-1. The external standard calibration curve method was used for determination of hormone concentration in plant tissues. Five standard solutions were prepared ranging from 0.05 to 10 ng μl-1. All samples were run in 3 replicates.
Gene Expression Analysis
RNA Preparation
Total RNA was extracted from frozen in liquid nitrogen, ground leaves (from 5 plants per sample) with the Total RNA Mini Kit (Bio-Rad, United States). RNA purity and quantity was determined by Biospec-Nano (SHIMADZU, JP) The integrity of RNA was assessed with the Agilent 2100 Bioanalyzer (United States) and RNA 6000 Nano Kit (Agilent, DE).
qPCR
Reverse transcription was carried out on 1000 ng of total RNA, after digestion with DNase (DNA free kit, Ambion Bioscience, United States), with iScript cDNA synthesis kit (Bio-Rad, United States). For qPCR, probes were labeled with the EvaGreen (SsoFast EvaGreen Supermix, Bio-Rad, United States) fluorescent dye. For a single reaction 10 ng of cDNA and 150 nM of gene specific primers were used. To test amplification specificity a dissociation curve was acquired by heating samples from 60°C to 95°C. As house-keeping reference α-tubulin 5 (At5g19780) and ubiquitin 10 (At4g05320) was used. Reaction efficiency was tested by serial dilutions of cDNAs with gene specific primers (Supplementary Table 1). All samples were run in triplicates. Expression was calculated according to Pfaffl (2001) with WT plants serving as calibrator. For gene expression analysis plants were harvested 10–12 days after inoculation. The experiment was repeated twice. In each experiment 3 samples (10 plants per sample) per variant were collected. Analysis were performed in triplicates.
Statistical Analysis
Data normality and variance homogeneity were evaluated by the Shapiro–Wilk and Levene’s tests, respectively. If necessary, data were normalized with log or Box-Cox transformation. Statistical significance was determined by analysis of variance (ANOVA), followed by Tuckey or Fischer post hoc test (p ≤ 0.05) as indicated in figure captions. Differences between two groups were tested by t-test. Statistical analyses were conducted using Statistica ver. 12.5 (Statsoft).
Results
GR24 Inhibits Mycelium Growth and Increases the Respiration Rate
Supplementation of growth medium with GR24 significantly inhibited the growth of Mucor sp. Colony diameter decreased with growing concentrations of GR24. The lowest concentration which had an inhibitory effect was 50 nM. Treatment with 100 nM inhibited mycelium growth in a similar fashion, whereas treatment with concentrations of 500 and 1000 nM resulted in a gradual decline in colony diameter (Figures 1A,B). Additionally, as shown in Figure 1B treatment with 1000 nM delayed spore formation by the fungi. The respiration rate was significantly decreased only in result of treatment with 1000 nM of GR24. Lower concentrations did not significantly affect it (Figure 1C). Mock treatments did not differ from control.
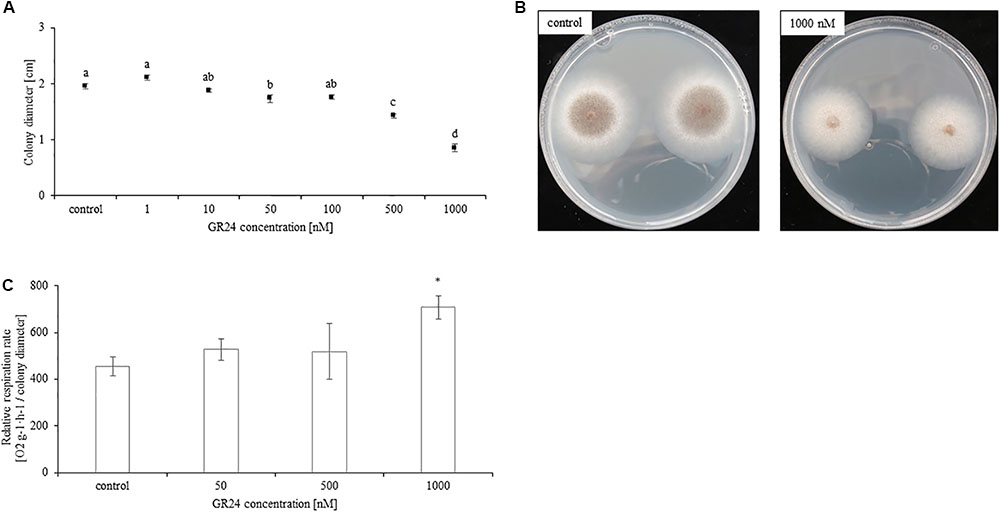
FIGURE 1. Mucor sp. colony diameter measured 48 h after PDA inoculation with 2.1 × 106 fungi spores. The medium was supplemented with 0, 1, 10, 50, 100, 500, and 1000 nM of GR24 (A). Letters above bars indicate statistically significant differences according to one-way ANOVA and Tuckey post hoc test (N = 10, P ≤ 0.05). Respiration rate of Mucor sp. grown in PDA supplemented with 50, 500, and 1000 nM of GR24 and not treated control. Fungi respiration was measured as O2 consumption per 2 h for 24 h. The respiration rate was presented in relation to colony diameter. Stars above bars represent statistically significant differences according to the student’s t-test (N = 5, P ≤ 0.05, mean value ± SE) (B). Photographs illustrating Mucor sp. colony diameter grown for 48 h in PDA supplemented with 1000 nM GR24 (C).
GR24 Activates H2O2 Production and Alters the Activity of Antioxidant Enzymes
GR24 treatment with doses higher than 50 nM induced H2O2 production in the fungi mycelium. There were no difference in H2O2 concentration in mycelium treated with 50, 100, and 1000 nM (Figure 2A) The activity of GR was significantly decreased in all treatments (Figure 2B). The response of CAT and Cu/ZnSOD was dose dependent. Doses of 1 and 10 nM decreased enzyme activity (Figures 2C,D) whereas 10–1000 nM significantly increased Cu/ZnSOD, but not CAT activity (Figures 2C,D). Mock treatments did not differ from control.
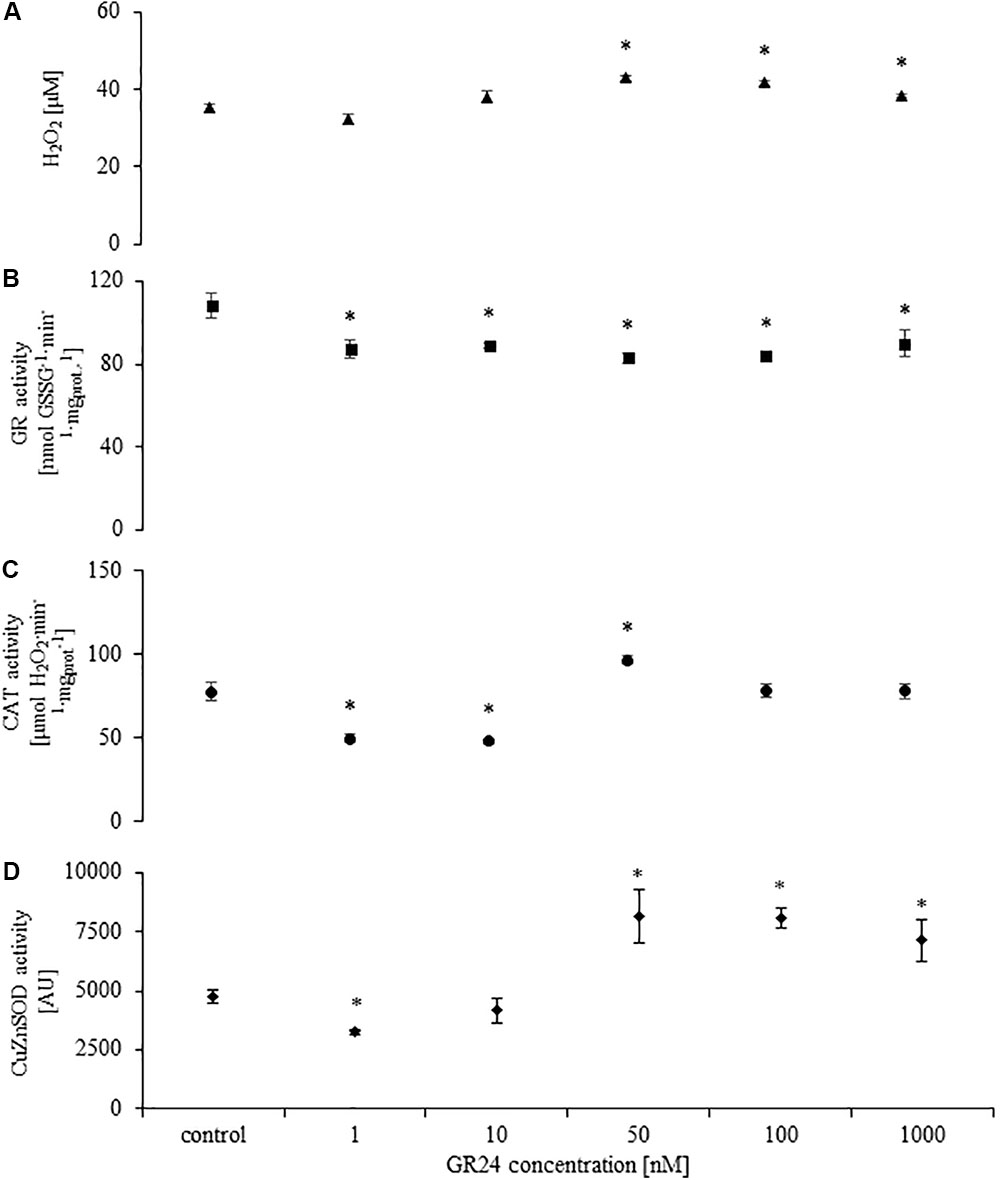
FIGURE 2. H2O2 concentration (A), glutathione reductase (GR; B), catalase (CAT; C), superoxide dismutase (Cu/ZnSOD; D) activities in Mucor sp. treated with 0, 1, 10, 50, 100, and 1000 nM of GR24. Stars above bars represent statistically significant differences according to the student’s t-test N = 5, P ≤ 0.05, mean value ± SE.
Fungi Colonization of SL Biosynthesis Mutants
One day after inoculation fungal hyphae was detected on the surface of the root (Figures 3A,B). Later, the mycelium was present inside root hairs (Figure 3C) and inside other root cells (Figure 3D). Colonization of A. thaliana tissues by Mucor sp. was described in detail by Domka et al. (2017, submitted). No significant changes in colonization rate were observed (Figure 3E).
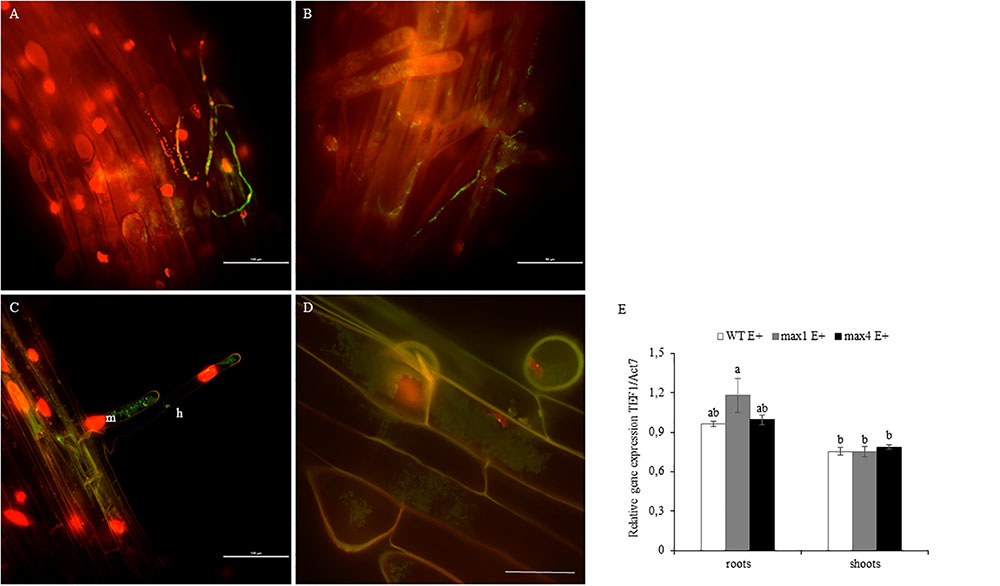
FIGURE 3. Roots of A. thaliana colonized by Mucor sp. tagged with GFP. Fungal hyphae on the root surface, bars 100 and 50 μm respectively (A,B). Mycelium (m) inside root hair (h), bar 100 μm (C). Fungal mycelium (m) inside root cells, bar 50 μm (D). Root colonization rate by mycelium shown as fungal TEF1α gene expression in relation to plant ACT7 gene expression. Letters above bars indicate statistically significant differences according to one-way ANOVA and Tuckey post hoc test (N = 3, P ≤ 0.05, mean value ± SE) (E).
SL Biosynthesis Mutants Do Not Develop the Beneficial Growth Phenotype Upon Inoculation
Due to differences in growth tempo between experiments, bars in Figure 4 represent fresh weight treated plants relative to appropriate control: WT, max1, max4 and max2. Inoculation with Mucor sp. improved biomass yield of WT and max2 A. thaliana, whereas SL biosynthesis mutants produced significantly less biomass (Figure 4).
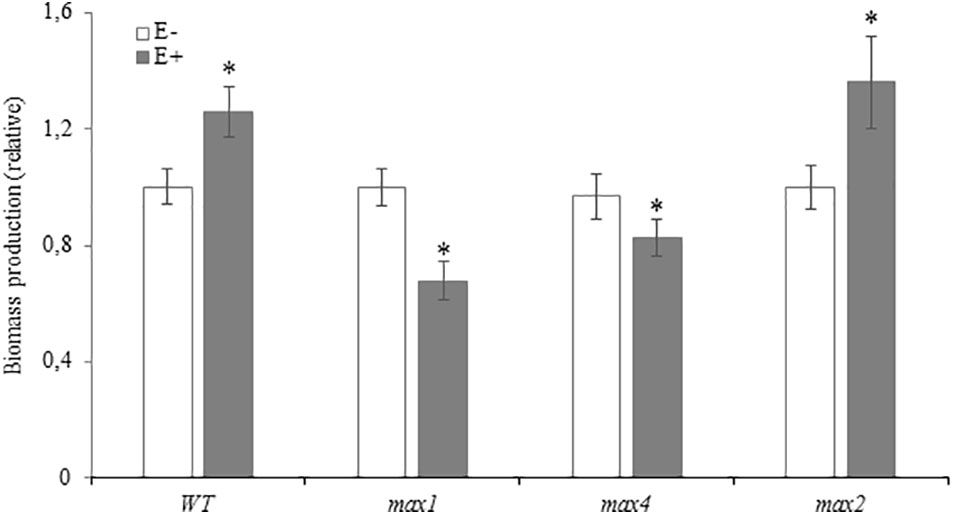
FIGURE 4. Fresh weight of Arabidopsis thaliana WT and max1, max4 and max2 mutants 10 days after inoculation with Mucor sp., GR24 treated and inoculated with Mucor sp. and treated with GR24 (A). Three separate experiments with 25–30 plants per genotype were performed. Due to differences in plant growth in between experiments, fresh weight of treated plants (E+, GR24 and E+GR24) was presented in relation to appropriate control (WT, max1, max4, max2). Stars above bars represent statistically significant differences according to the student’s t-test N = 5, P ≤ 0.05, mean value ± SE.
Mucor sp. Decomposes GR24 in Planta
The fluorescence signal from GR24-BODIPY was significantly lower in E+ plants 48 h after inoculation (Figures 5A,B).
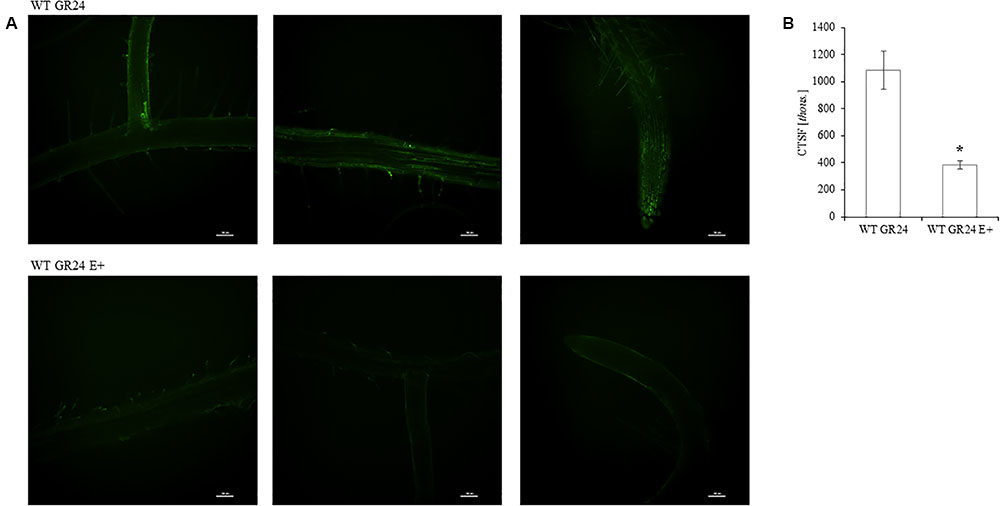
FIGURE 5. Photographs illustrating the decomposition of the fluorescent analog GR24-BODIPY (StrigoLab, I) by Mucor mycelium inside roots of A. thaliana. Plants were fed with 1 μM GR24-BODIPY in MSR for 24 h transferred to GR24 free medium and inoculated with Mucor sp. (A). Visualization was performed with confocal microscope and quantification of the fluorescence signal was performed 48 hpi with ImageJ-NIH. Stars above bars represent statistically significant differences according to the student’s t-test N = 3, P ≤ 0.05, mean value ± SE (B).
High Doses of GR24 Induce SA Synthesis in A. thaliana, But Has No Effect on JA Production
To test the relationship between SL and SA and JA production, A. thaliana 10 day old seedlings grown in medium MS medium were transferred to MSR supplemented with 0, 1, 10, 50, 100, 500, 1000 nM of GR24. Synthetic SL treatment had no effect on JA accumulation in plants (data not shown). SA concentration was significantly increased (close to 30-fold) in plants treated with the highest dose of GR24 (Figure 6A). Lower concentrations did not affect SA synthesis in A. thaliana. No differences in SA concentration were shown 24 hpi (hours past inoculation) (Figure 6B). 48 hpi GR24 treated seedlings accumulated significantly more SA. Twelve days after inoculation GR24 treated plants accumulated 25-fold more SA compared to control. Inoculation with the fungi prevented SA production by the plant (Figure 6B). 48 hpi SA accumulation was slightly lower than in untreated (GR24+) plants, but not significantly higher than in control and E+ seedlings. No differences in SA concentration were shown 12 dpi between control and E+ seedlings. Mock treatments did not differ from control.
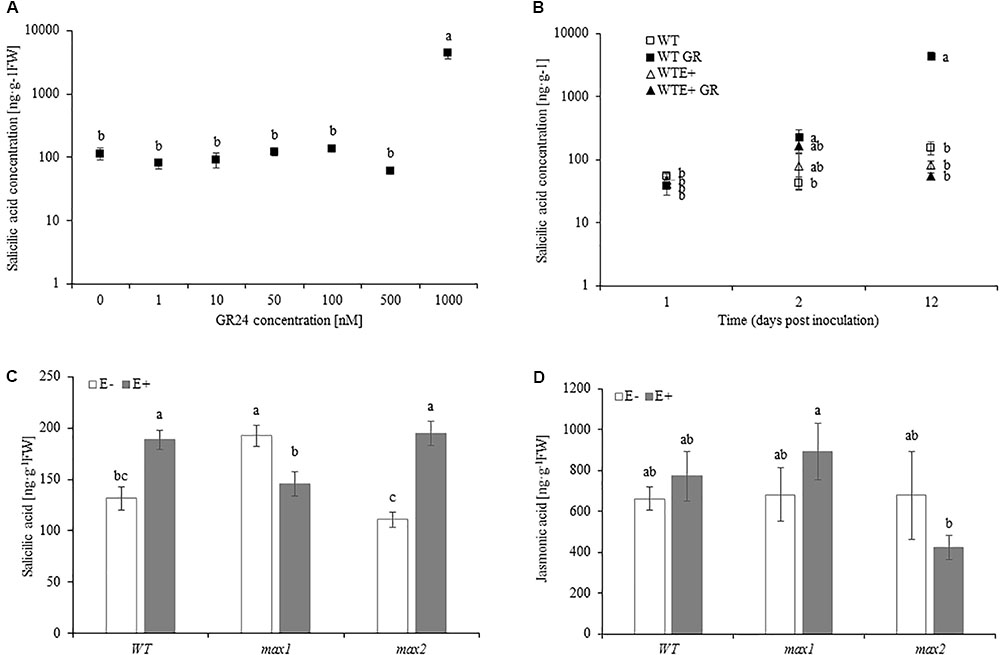
FIGURE 6. SA accumulation in Arabidopsis thaliana WT grown in medium supplemented with 0–1000 nM of GR24 for 10 days (A). SA accumulation in Arabidopsis thaliana WT grown in medium supplemented with 1000 nM of GR24 for 24 h, 48 h and 12 days (B). SA (C) and JA (D) concentration in A. thaliana seedlings inoculated with Mucor sp. 10 days after inoculation. Letters above bars indicate statistically significant differences according to one-way ANOVA and Fischer post hoc test (N = 3, P ≤ 0.05, mean value ± SE).
Salicylic Acid and Jasmonic Acid Accumulation in E+ WT and max1 and 2 Mutants
SA accumulation in max1 mutants was significantly higher, whereas max2 accumulated significantly less SA than in WT plants. Upon inoculation SA concentration increased in WT and max2. max1 mutants responded to inoculation with decreased SA accumulation, which was significantly lower than in WT and max2 (Figure 6C). No differences in JA accumulation were shown between WT, max1 and max2 mutants, nor did inoculation affect it (Figure 6D).
The Expression SL Biosynthesis Was Downregulated in E+ Arabidopsis thaliana
The expression of genes encoding proteins involved in SL biosynthesis: CYP711A (cytochrome P450, family 711, subfamily A), D27 (beta-carotene isomerase D27-like protein) and CCD8 (carotenoid cleavage dioxygenase 8) were significantly downregulated in inoculated WT plants, whereas genes encoding proteins involved in SL signaling were either unaffected by inoculation: D14 (strigolactone esterase D14), BRC1 (branched 1) (Figure 7C).
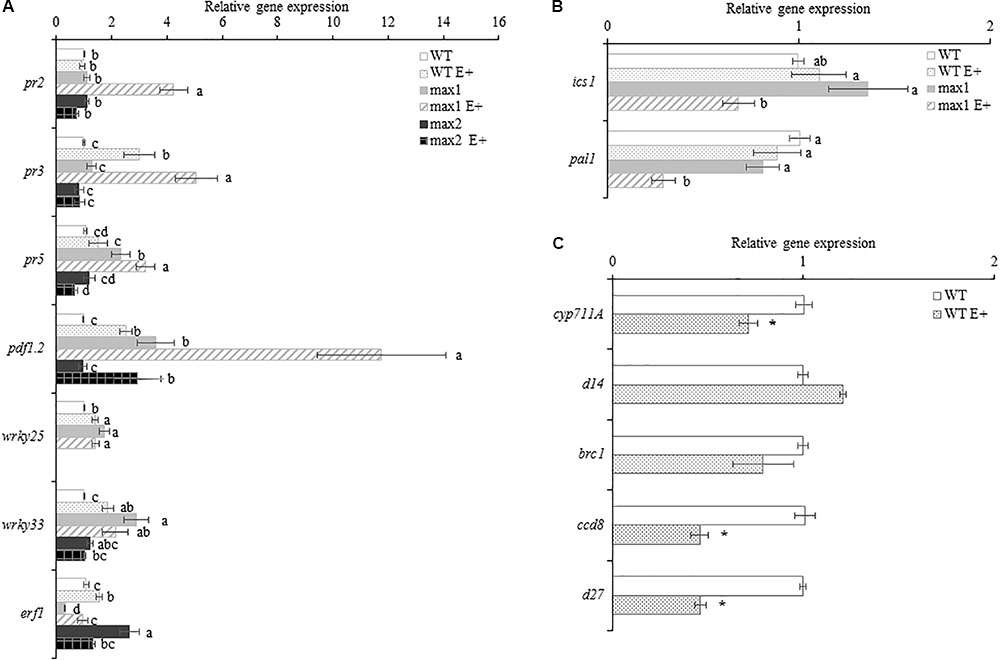
FIGURE 7. The expression of A. thaliana WT, max1 and max2 inoculated with Mucor sp. (E+) defense related genes (A), salicylic acid biosynthesis genes (B) and strigolactone biosynthesis and signaling genes (C) Letters above bars indicate statistically significant differences according to one-way ANOVA and Fischer post hoc test (N = 6, P ≤ 0.05, mean value ± SE).
The Expression Profiles of Plant Defense Related Genes Were Altered in max1 and max2 Mutants During the Interaction With Mucor sp.
In order to evaluate the role of SL in adjusting A. thaliana’s defense to inoculation with Mucor sp. the expression of selected plant defensins was quantified (Figure 7A). Upon inoculation, the abundance of PR2 mRNA was not changed in WT and max2. max1 exhibited a significant upregulation of the expression of this gene. The expression of PR3 was upregulated in E+WT and E+max1 (in max1 more significantly then in WT), whereas in max2 no differences in PR3 expression after inoculation were shown. No differences in the expression of PR5 between E+ and appropriate controls were shown, however, in the SL biosynthesis mutant PR5 transcript abundance was significantly higher compared to WT and max2 (in both control and E+). Interestingly, PR5 expression in E+max4 was significantly higher (Supplementary Figure 1).
Inoculation with Mucor sp. resulted in a significant upregulation in the expression of PDF1.2 in relation to relevant controls in all plants examined. Expression levels in WT and max2 were similar, whereas PDF1.2 transcript abundance in max1 was significantly higher (in both control and E+ plants). The expression of two defense related transcription factors WRKY25 and WRKY33 was upregulated in max1 compared to WT plants. After inoculation their abundance rose in WT, whereas in max1 and max2 no differences were found. The response of SA biosynthesis genes was also altered in SL biosynthesis mutants. Genes encoding ICS1 and PAL1 were downregulated upon inoculation in max1 E+ in relation to respective control, whereas no differences in their expression were found in WT (Figure 7B). The expression of the examined genes in max4 closely resembled that in max1 (Supplementary Figure 1).
Disscusion
GR24 strongly inhibited the growth of a number of phyto-pathogenic fungi, suggesting a role in plant defense (Dor et al., 2011). Additionally, hyphal branching was shown to be activated upon treatment with the SL analog. In this study GR24 treatment had a similar effect on the endophytes growth, but it did not affect branching (data not shown). GR24 doses used in this study were, however significantly lower compared to the concentrations used by other authors. Previously, micromolar concentrations of GR24 were shown to limit growth of mycelium (Dor et al., 2011; Belmondo et al., 2017), whereas here, 50 nM of GR24 was sufficient enough to limit colony expansion. This suggests that SL in the mutualistic interaction, imposes its effects in concentrations relatively lower compared to plant-pathogen interactions. Higher pathogen resistance to SL may have evolved due to selective pressure of this group of fungi. This, however, requires more detailed research.
Mycelium growth inhibition was accompanied by increased production/accumulation of H2O2 and inhibited the activity of GR which plays an important role in H2O2 scavenging. Lower concentrations of GR24, below 50 nM, reduced the activity of antioxidant enzymes examined, indicating that SL activates ROS production in the mycelium either directly by inducing an oxidative burst or indirectly, by reducing the activity of ROS scavenging enzymes. ROS were suggested to be necessary in limiting B. cinerea radial growth by GR24 (Belmondo et al., 2017). Previously, Tanaka et al. (2006) showed that by knocking out the NOXA gene encoding the plasmalemma bound, generating NADPH oxidase, the perennial ryegrass endophyte Epichloë festucae spreads throughout the plant causing disease symptoms. This indicated that by inducing ROS production the host plant may control its symbiotic partner. GR24 concentrations of 50 nM and above induced the production of H2O2 in Mucor sp. and activated the H2O2 producing/
scavenging Cu/Zn SOD, suggesting that SL plays a role in activating ROS production during plant–fungi symbiosis.
Strigolactone are perceived by D14, a non-canonical α/β hydrolase receptor. Upon binding D14 was proposed to hydrolyse SL (Koltai and Kapulnik, 2013). The mechanism of SL perception by fungi is unknown, however, SL decomposition is not a unique plant feature. Recently it was shown that soil borne fungi from the Trichoderma and Fusarium genus were able to degrade 4 different natural and synthetic SLs including GR24. This ability is considered to be used in prevention of parasitic plant seed germination, but the biological relevance of this phenomenon is not known (Boari et al., 2016). In this study, we showed that Mucor sp. can also decompose GR24, both ex planta and more importantly in planta, thus it cannot be excluded that the fungi can modulate SL metabolism during the colonization process. Additionally, the results presented here show that upon inoculation, the expression of SL biosynthesis genes was downregulated in A. thaliana. This provides further evidence for the modulatory role of the endophytic Mucor sp. on SL metabolism during symbiosis establishment.
To evaluate the relevance of SL in the interaction between A. thaliana and Mucor sp. SL mutants were inoculated with the fungi. Biosynthesis mutants max1 and max4 responded negatively, in terms of growth to inoculation. The phenotype of max2 (which synthesizes, but does not respond to SL) after inoculation with Mucor sp. resembled WT, suggesting that SL may had induced changes in Mucor sp. metabolism by switching it from “beneficial to parasitic mode” and MAX2 dependent signaling was not necessary for the development of the beneficial phenotype. What’s interesting is that the colonization rate of max1 and 4 mutants did not differ from WT, indicating that in the absence of SL the plants did not lose ability to control colonization.
Plants control mycelium spread inside their tissues by activating a specific immune response. Selected defense mechanisms controlled by plant hormones JA, SA and ET are upregulated and others are downregulated (Jacobs et al., 2011; Lahrmann et al., 2015). A relationship between SL and these phytohormones has also been suggested (Marzec, 2016). According to the only report connecting SL to plant defense (Torres-Vera et al., 2014), SL deficiency alters defense related hormone profiles: the Slccd8 tomato RNAi line accumulated less JA and SA. According to results presented here JA accumulation was not affected by neither SL deficiency, lack of MAX2 dependent SL signaling nor inoculation. To verify weather SL acts in controlling plant defense (possibly in the suppression of plant defense) we quantified the accumulation of SA in WT and max mutants inoculated with the fungus. The results indicate that, SL may act in suppressing SA production: A. thaliana max1 and max4 (see Supplement) accumulated significantly more SA than WT plants. At the same time, SA concentration in WT E+ and max2 E+ increased what was coincident with deactivation of SL biosynthesis genes, suggesting that there may be a relationship between these two processes. SA biosynthesis gene expression was not changed in WT plants what indicates that other routes of SA accumulation regulation (SA catabolism for instance) had to control this process (Lahrmann et al., 2015). Exogenously applied synthetic SL activated SA synthesis in A. thaliana but only in concentrations of 1 μM, lower concentrations did not affect SA production in seedlings. This undermines the hypothesis of the downregulating role of SL in SA production. The physiological relevance of SL activated SA production is controversial. There are no reports indicating that plants can be exposed to such high concentrations in nature, nM and pM concentrations are usually found in planta (Seto et al., 2014), but 1 μM of GR24 is preferentially used in most studies using this SL analog (Marquez-Garcia et al., 2014; Passaia et al., 2014; Ito et al., 2015). In literature, there is only one report indicating that micromolar doses of GR24 can be toxic to the plant (Ito et al., 2015). No reports consider the fact that A. thaliana seedlings treated with GR24 can be exposed to stress (SA biosynthesis is stress responsive), nor consider the fact of SA synthesis activation upon GR24 treatment. Nevertheless, the results of SA accumulation seem to confirm that SL serves in inhibition of SA accumulation. SA accumulated in max1 mutants, co-cultivation of A. thaliana with Mucor sp. resulted in downregulation of SL biosynthesis genes and an increase in accumulation of SA (probably via a max2 independent pathway). Additionally, we have shown that the fungus has the ability to degrade SL in planta what can be another routes of regulating SL content by the fungus. Several reports indicate that mycorrhizal plants prevent further root colonization by AMF possibly by altering SL production (reviewed in Steinkellner et al., 2007). The results presented here provide indirect evidence confirming that, indeed the endophytic fungus Mucor sp. possesses the ability to modulate SL metabolism/abundance in planta. Verifying the ecological significance of this phenomenon requires, however, further research. The relationship and the role of SL and SA in the establishment of the plant–fungi symbiosis is much more complicated than the picture presented above, the accumulation of SA in max1 E+ and max4 E+ mutants, as well as SA biosynthesis gene downregulation in E+ mutants indicates that other factors are involved in establishing the post infection equilibrium between SA and SL in plant–endophytic fungi interactions.
To further explore the relationship between SL and plant defense during the interaction between A. thaliana and Mucor sp. the expression of selected defense related genes in A. thaliana SL was measured. The expression of plant defensins is SA and JA inducible (Thomma et al., 1998, 1999). The expression of the tested defensins (PR2, PR3, PR5, PDF1.2) was significantly upregulated in inoculated plants under SL deficiency. Additionally, PDF1.2 and PR5 expression was significantly higher in the absence of the fungal stimulus providing further evidence for a link between SL and plant defense. The expression of the two defense related TFs examined also differed in WT and max1. WRKY25 and WRKY33 transcript abundance was higher in max1 and did not respond to inoculation, whereas WT exhibited an activation of their expression. Surprisingly, the response of the SL signaling mutant max2 differed from both WT and max1. Constitutive expression of the genes tested resembled WT, but, barely any of the genes responded to the fungi. Out of the tested genes only PDF1.2 exhibited a response similar to that found in WT. Nevertheless, inhibition of max2 dependent SL signaling resulted in almost complete non-responsiveness of the tested genes. The max2 mutant produces SL and developed the beneficial phenotype upon inoculation, what indicates that SL serves both as a signal influencing the fungi and a signaling molecule controlling plant defense in response to the endophyte. It seems as though, SL MAX2 dependent signaling and activation of defense that takes place in the WT is not a necessary condition in the development of a mutual symbiosis between A. thaliana and Mucor sp. However, defense related gene expression in max1 and max4 mutants indicates that SL plays a role in suppressing the expression of the tested genes. The MAX2 dependent SL signaling pathway is intact in these mutants, suggesting that other factors compensate for SL under SL deficiency. Additionally, MAX2 signaling is not a unique feature of SL signal transduction. Another group of plant signaling molecules: karrikins, derived from cellulose combustion and involved in fire follower plant seed germination (among other functions) utilize MAX2 dependent signaling (Waters et al., 2014). MAX2 also targets several other proteins including the brassinosteroid target protein BRI1-EMS SUPPRESSOR1 (BES1) and the proteins from the DELLA family of GRAS transcriptional regulators involved in gibberellin signaling (Wang et al., 2013). Thus, caution needs to be taken when interpreting the response of the MAX2 mutant.
During the A. thaliana–Mucor sp. interaction an accumulation of defense compounds took place in WT (PDF1.2 and PR3), but in significantly less quantities when compared to max1 and max4. Previously, Jacobs et al. (2011) have shown that some basal defense is necessary for the establishment of the beneficial interaction between an endophytic fungus and its host plant. The results presented here seem to confirm this statement, however, there are variations in the response of A. thaliana to Mucor sp. and other fungi species in terms of activation specific defense related genes.
Upregulated defense mechanisms may not necessarily indicate a direct link between SL and defense. We can also imagine that since SL affects the fungi limiting its growth, it can also affect some unknown recognition patterns rendering the fungi recognizable as an endophyte and the upregulation of plant defense results from not recognizing the fungi as potentially beneficial. Additionally, the negative growth response of SL biosynthesis mutants suggests that in the absence of SL, a shift in resource allocation from growth to defense necessary to restrain fungi spread may take place. This, however, requires further investigations.
Conclusion
Recently, intense efforts are made to define the elements that allow plants to distinguish between potential symbionts and pathogens and to elucidate the plant-fungi cross-talk. Studying signals exchanged in the rhizosphere by plants and fungi is thus significantly important (Bonfante and Genre, 2010; Antolín-Llovera et al., 2014; Hayashi and Parniske, 2014). The role of SL in this process has been proposed previously. The data presented in this study indicates that SL plays a dual role in the interaction between plants and endophytic fungal symbionts. The synthetic SL analog GR24 was shown to affect the metabolism of Mucor sp. by limiting its growth and inducing ROS production. On the other hand, the fungus was shown to be able to decompose SL in planta and reduce SL biosynthesis gene expression. At the same time SL deficient A. thaliana mutants, but not the max2 signaling mutant were not able to benefit from its fungal symbiont. The expression of a number of defense related genes was upregulated in SL deficient plants, suggesting a role of SL in regulating the plants immune response. However, it seems that the postulated link between SL and JA, SA is not as unequivocal as previously thought. Nevertheless, we present several lines of evidence of a direct and/or indirect relation of SL and plant defense. Additionally, we have shown that high doses of GR24 activate SA production in A. thaliana and that by inoculating the plant with Mucor sp. we were able to prevent SA accumulation most probably by limiting root exposition to GR24 (the fungi decomposed GR24). This model represents a good illustration of the role that fungi play in natural environments. SL decomposition and possible downregulation of SL biosynthesis in the host plant limits SL availability in the soil, what in turn may have a limiting effect on parasitic plant seed germination.
Author Contributions
PR designed the study, participated in experiments, analyzed the data and prepared the text of the manuscript. AD performed the qPCR, microscopic analyses. RW performed the qPCR and statistical analysis of data. RJ performed the HPLC determination of hormones. MN performed enzyme activity assays. KT was involved in designing the study and interpretation of the results. All authors were involved in the manuscript preparation and approved the manuscript prior to the submission.
Funding
This work was supported by NSC, Maestro Project, DEC – 2011/02/A/NZ9/00137, COST action FA1206, and Jagiellonian University funds (DS 758).
Conflict of Interest Statement
The authors declare that the research was conducted in the absence of any commercial or financial relationships that could be construed as a potential conflict of interest.
Acknowledgments
The authors would like to acknowledge Teresa Anielska, Martyna Janicka, Weronika Janas (Institute of Environmental Sciences, Jagiellonian University, Kraków, Poland) and Agnieszka Bednarska (Institute of Nature Conservation, Polish Academy of Sciences, Kraków, Poland) for technical support.
Supplementary Material
The Supplementary Material for this article can be found online at: https://www.frontiersin.org/articles/10.3389/fmicb.2018.00441/full#supplementary-material
References
Aebi, H. (1984). Catalase in vitro. Methods Enzymol. 105, 121–126. doi: 10.1016/S0076-6879(84)05016-3
Akiyama, K., Matsuzaki, K., and Hayashi, H. (2005). Plant sesquiterpenes induce hyphal branching in arbuscular mycorrhizal fungi. Nature 435, 824–827. doi: 10.1038/nature03608
Andreo-Jimenez, B., Ruyter-Spira, C., Bouwmeester, H. J., and Lopez-Raez, J. A. (2015). Ecological relevance of strigolactones in nutrient uptake and other abiotic stresses, and in plant-microbe interactions below-ground. Plant Soil 394, 1–19. doi: 10.1007/s11104-015-2544-z
Antolín-Llovera, M., Ried, M. K., and Parniske, M. (2014). Cleavage of the symbiosis receptor-like kinase ectodomain promotes complex formation with nod factor receptor 5. Curr. Biol. 24, 422–427. doi: 10.1016/j.cub.2013.12.053
Bacon, C. W., and White, J. F. (2016). Functions, mechanisms and regulation of endophytic and epiphytic microbial communities of plants. Symbiosis 68, 87–98. doi: 10.1007/s13199-015-0350-2
Barzanti, R., Ozino, F., Bazzicalupo, M., Gabbrielli, R., Galardi, F., Gonnelli, C., et al. (2007). Isolation and characterization of endophytic bacteria from the nickel hyperaccumulator plant Alyssum bertolonii. Microb. Ecol. 53, 306–316. doi: 10.1007/s00248-006-9164-3
Beauchamp, C., and Fridovich, I. (1971). Superoxide dismutase: improved assays and an assay applicable to acrylamide gels. Anal. Biochem. 44, 276–287. doi: 10.1016/0003-2697(71)90370-8
Belmondo, S., Marschall, R., Tudzynski, P., López Ráez, J. A., Artuso, E., Prandi, C., et al. (2017). Identification of genes involved in fungal responses to strigolactones using mutants from fungal pathogens. Curr. Genet. 63, 201–213. doi: 10.1007/s00294-016-0626-y
Besserer, A., Becard, G., Jauneau, A., Roux, C., and Sejalon-Delmas, N. (2008). GR24, a synthetic analog of strigolactones, stimulates the mitosis and growth of the Arbuscular Mycorrhizal Fungus Gigaspora rosea by boosting its energy metabolism. Plant Physiol. 148, 402–413. doi: 10.1104/pp.108.121400
Besserer, A., Puech-Pagès, V., Kiefer, P., Gomez-Roldan, V., Jauneau, A., Roy, S., et al. (2006). Strigolactones stimulate arbuscular mycorrhizal fungi by activating mitochondria. PLoS Biol. 4:e226. doi: 10.1371/journal.pbio.0040226
Boari, A., Ciasca, B., Pineda-Martos, R., Lattanzio, V. M. T., Yoneyama, K., and Vurro, M. (2016). Parasitic weed management by using strigolactone-degrading fungi. Pest Manag. Sci. 72, 2043–2047. doi: 10.1002/ps.4226
Bonfante, P., and Genre, A. (2010). Mechanisms underlying beneficial plant–fungus interactions in mycorrhizal symbiosis. Nat. Commun. 1, 1–11. doi: 10.1038/ncomms1046
Bradford, M. M. (1976). A rapid and sensitive method for the quantitation of microgram quantities of protein utilizing the principle of protein-dye binding. Anal. Biochem. 72, 248–254. doi: 10.1101/pdb.prodprot15
Brewer, P. B., Yoneyama, K., Filardo, F., Meyers, E., Scaffidi, A., Frickey, T., et al. (2016). LATERAL BRANCHING OXIDOREDUCTASE acts in the final stages of strigolactone biosynthesis in Arabidopsis. Proc. Natl. Acad. Sci. U.S.A. 113, 6301–6306. doi: 10.1073/pnas.1601729113
Brundrett, M. C. (2009). Mycorrhizal associations and other means of nutrition of vascular plants: understanding the global diversity of host plants by resolving conflicting information and developing reliable means of diagnosis. Plant Soil 320, 37–77. doi: 10.1007/s11104-008-9877-9
Bu, Q., Lv, T., Shen, H., Luong, P., Wang, J., Wang, Z., et al. (2014). Regulation of drought tolerance by the F-Box protein MAX2 in Arabidopsis. Plant Physiol. 164, 424–439. doi: 10.1104/pp.113.226837
Card, S. D., Hume, D. E., Roodi, D., McGill, C. R., Millner, J. P., and Johnson, R. D. (2015). Beneficial endophytic microorganisms of Brassica - A review. Biol. Control 90, 102–112. doi: 10.1016/j.biocontrol.2015.06.001
Chen, Z., Gao, X., Zhang, J., Bonfante, P., Genre, A., Al-Babili, S., et al. (2015). Strigolactones affect lateral root formation and root-hair elongation in Arabidopsis. Planta 6, 209–216. doi: 10.1007/s00425-010-1310-y
Dor, E., Joel, D. M., Kapulnik, Y., Koltai, H., and Hershenhorn, J. (2011). The synthetic strigolactone GR24 influences the growth pattern of phytopathogenic fungi. Planta 234, 419–427. doi: 10.1007/s00425-011-1452-6
Foo, E., Blake, S. N., Fisher, B. J., Smith, J. A., and Reid, J. B. (2016). The role of strigolactones during plant interactions with the pathogenic fungus Fusarium oxysporum. Planta 243, 1387–1396. doi: 10.1007/s00425-015-2449-3
Foo, E., and Reid, J. B. (2013). Strigolactones: new physiological roles for an ancient signal. J. Plant Growth Regul. 32, 429–442. doi: 10.1007/s00344-012-9304-6
Foo, E., Yoneyama, K., Hugill, C. J., Quittenden, L. J., and Reid, J. B. (2013). Strigolactones and the regulation of pea symbioses in response to nitrate and phosphate deficiency. Mol. Plant 6, 76–87. doi: 10.1093/mp/sss115
Foyer, C. H., Souriau, N., Perret, S., Lelandais, M., Kunert, K. J., Pruvost, C., et al. (1995). Overexpression of glutathione reductase but not glutathione synthetase leads to increases in antioxidant capacity and resistance to photoinhibition in poplar trees. Plant Physiol. 109, 1047–1057. doi: 10.1104/pp.109.3.1047
García, E., Alonso,Á., Platas, G., and Sacristán, S. (2013). The endophytic mycobiota of Arabidopsis thaliana. Fungal Divers. 60, 71–89. doi: 10.1007/s13225-012-0219-0
Gomez-Roldan, V., Fermas, S., Brewer, P. B., Puech-Pagès, V., Dun, E. A., Pillot, J.-P., et al. (2008). Strigolactone inhibition of shoot branching. Nature 455, 189–194. doi: 10.1038/nature07271
Gutjahr, C., Radovanovic, D., Geoffroy, J., Zhang, Q., Siegler, H., Chiapello, M., et al. (2012). The half-size ABC transporters STR1 and STR2 are indispensable for mycorrhizal arbuscule formation in rice. Plant J. 69, 906–920. doi: 10.1111/j.1365-313X.2011.04842.x
Ha, C. V., Leyva-Gonzalez, M. A., Osakabe, Y., Tran, U. T., Nishiyama, R., Watanabe, Y., et al. (2014). Positive regulatory role of strigolactone in plant responses to drought and salt stress. Proc. Natl. Acad. Sci. U.S.A. 111, 851–856. doi: 10.1073/pnas.1322135111
Hayashi, M., and Parniske, M. (2014). Symbiosis and pathogenesis: what determines the difference? Curr. Opin. Plant Biol. 20, v–vi. doi: 10.1016/j.pbi.2014.07.008
Hong, C. E., Jo, S. H., Moon, J. Y., Lee, J. S., Kwon, S. Y., and Park, J. M. (2015). Isolation of novel leaf-inhabiting endophytic bacteria in Arabidopsis thaliana and their antagonistic effects on phytophathogens. Plant Biotechnol. Rep. 9, 451–458. doi: 10.1007/s11816-015-0372-5
Ito, S., Nozoye, T., Sasaki, E., Imai, M., Shiwa, Y., Shibata-Hatta, M., et al. (2015). Strigolactone regulates anthocyanin accumulation, acid phosphatases production and plant growth under low phosphate condition in Arabidopsis. PLoS One 10:e0119724. doi: 10.1371/journal.pone.0119724
Jacobs, S., Zechmann, B., Molitor, A., Trujillo, M., Petutschnig, E., Lipka, V., et al. (2011). Broad-spectrum suppression of innate immunity is required for colonization of Arabidopsis roots by the fungus Piriformospora indica. Plant Physiol. 156, 726–740. doi: 10.1104/pp.111.176446
Johnson, L. J., De Bonth, A. C. M., Briggs, L. R., Caradus, J. R., Finch, S. C., Fleetwood, D. J., et al. (2013). The exploitation of epichloae endophytes for agricultural benefit. Fungal Divers. 60, 171–188. doi: 10.1007/s13225-013-0239-4
Kohlen, W., Charnikhova, T., Liu, Q., Bours, R., Domagalska, M. A., Beguerie, S., et al. (2011). Strigolactones are transported through the xylem and play a key role in shoot architectural response to phosphate deficiency in nonarbuscular mycorrhizal host Arabidopsis. Plant Physiol. 155, 974–987. doi: 10.1104/pp.110.164640
Koltai, H., and Kapulnik, Y. (2013). Unveiling signaling events in root responses to strigolactone. Mol. Plant 6, 589–591. doi: 10.1093/mp/sst057
Koltai, H., LekKala, S. P., Bhattacharya, C., Mayzlish-Gati, E., Resnick, N., Wininger, S., et al. (2010). A tomato strigolactone-impaired mutant displays aberrant shoot morphology and plant interactions. J. Exp. Bot. 61, 1739–1749. doi: 10.1093/jxb/erq041
Kretzschmar, T., Kohlen, W., Sasse, J., Borghi, L., Schlegel, M., Bachelier, J. B., et al. (2012). A petunia ABC protein controls strigolactone-dependent symbiotic signalling and branching. Nature 483, 341–344. doi: 10.1038/nature10873
Laemmli, U. K. (1970). Cleavage of structural proteins during the assembly of the head of bacteriophage T4. Nature 227, 680–685. doi: 10.1038/227680a0
Lahrmann, U., Strehmel, N., Langen, G., Frerigmann, H., Leson, L., Ding, Y., et al. (2015). Mutualistic root endophytism is not associated with the reduction of saprotrophic traits and requires a noncompromised plant innate immunity. New Phytol. 207, 841–857. doi: 10.1111/nph.13411
Li, H. Y., Wei, D. Q., Shen, M., and Zhou, Z. P. (2012). Endophytes and their role in phytoremediation. Fungal Divers. 54, 11–18. doi: 10.1007/s13225-012-0165-x
Liu, J., He, H., Vitali, M., Visentin, I., Charnikhova, T., Haider, I., et al. (2015). Osmotic stress represses strigolactone biosynthesis in Lotus japonicus roots: exploring the interaction between strigolactones and ABA under abiotic stress. Planta 241, 1435–1451. doi: 10.1007/s00425-015-2266-8
López-Ráez, J. A. (2016). How drought and salinity affect arbuscular mycorrhizal symbiosis and strigolactone biosynthesis? Planta 243, 1375–1385. doi: 10.1007/s00425-015-2435-9
López-Ráez, J. A., Charnikhova, T., Gómez-Roldán, V., Matusova, R., Kohlen, W., De Vos, R., et al. (2008). Tomato strigolactones are derived from carotenoids and their biosynthesis is promoted by phosphate starvation. New Phytol. 178, 863–874. doi: 10.1111/j.1469-8137.2008.02406.x
Lopez-Raez, J. A., Shirasu, K., and Foo, E. (2017). Strigolactones in plant interactions with beneficial and detrimental organisms: the Yin and Yang. Trends Plant Sci. 22, 527–537. doi: 10.1016/j.tplants.2017.03.011
Marquez-Garcia, B., Njo, M., Beeckman, T., Goormachtig, S., and Foyer, C. H. (2014). A new role for glutathione in the regulation of root architecture linked to strigolactones. Plant Cell Environ. 37, 488–498. doi: 10.1111/pce.12172
Martin, F., and Plett, J. M. (2015). Reconsidering mutualistic plant–fungal interactions through the lens of effector biology. Curr. Opin. Plant Biol. 26, 45–50. doi: 10.1016/j.pbi.2015.06.001
Marzec, M. (2016). Strigolactones as part of the plant defence system. Trends Plant Sci. 21, 900–903. doi: 10.1016/j.tplants.2016.08.010
Mori, N., Nishiuma, K., Sugiyama, T., Hayashi, H., and Akiyama, K. (2016). Carlactone-type strigolactones and their synthetic analogues as inducers of hyphal branching in arbuscular mycorrhizal fungi. Phytochemistry 130, 90–98. doi: 10.1016/j.phytochem.2016.05.012
Müller, M., Munné-Bosch, S., Pan, X., Welti, R., Wang, X., Dun, E., et al. (2011). Rapid and sensitive hormonal profiling of complex plant samples by liquid chromatography coupled to electrospray ionization tandem mass spectrometry. Plant Methods 7:37. doi: 10.1186/1746-4811-7-37
Oelmüller, R., Sherameti, I., Tripathi, S., and Varma, A. (2009). Piriformospora indica, a cultivable root endophyte with multiple biotechnological applications. Symbiosis 49, 1–17. doi: 10.1007/s13199-009-0009-y
Pandya-Kumar, N., Shema, R., Kumar, M., Mayzlish-Gati, E., Levy, D., Zemach, H., et al. (2014). Strigolactone analog GR24 triggers changes in PIN2 polarity, vesicle trafficking and actin filament architecture. New Phytol. 202, 1184–1196. doi: 10.1111/nph.12744
Passaia, G., Queval, G., Bai, J., Margis-Pinheiro, M., and Foyer, C. H. (2014). The effects of redox controls mediated by glutathione peroxidases on root architecture in Arabidopsis. J. Exp. Bot. 65, 1403–1413. doi: 10.1093/jxb/ert486
Pfaffl, M. (2001). A new mathematical model for relative quantification in real-time RT–PCR. Nucleic Acids Res. 29, 2003–2009. doi: 10.1093/nar/29.9.e45
Piisilä, M., Keceli, M. A., Brader, G., Jakobson, L., Jõesaar, I., Sipari, N., et al. (2015). The F-box protein MAX2 contributes to resistance to bacterial phytopathogens in Arabidopsis thaliana. BMC Plant Biol. 15:53. doi: 10.1186/s12870-015-0434-4
Rochange, S. (2010). “Strigolactones and their role in arbuscular mycorrhizal symbiosis,” in Arbuscular Mycorrhizas: Physiology and Function, ed. Y. Kapulnik (Dordrecht: Springer), 73–90.
Rozpądek, P., Domka, A., Ważny, R., Nosek, M., Jędrzejczyk, R., Tokarz, K., et al. (2017). How does the endophytic fungus Mucor sp. improve Arabidopsis arenosa vegetation in the degraded environment of a mine dump? Environ. Exp. Bot. 147, 31–42. doi: 10.1016/j.envexpbot.2017.11.009
Ruyter-Spira, C., Kohlen, W., Charnikhova, T., van Zeijl, A., van Bezouwen, L., de Ruijter, N., et al. (2011). Physiological effects of the synthetic strigolactone analog GR24 on root system architecture in Arabidopsis: another belowground role for strigolactones? Plant Physiol. 155, 721–734. doi: 10.1104/pp.110.166645
Schulz, B. (2006). “Mutualistic interactions with fungal root endophytes,” in Microbial Root Endophytes, eds B. Schulz, C. Boyale, and T. Sieber (Berlin: Springer), 261–279. doi: 10.1007/3-540-33526-9_15
Seto, Y., Sado, A., Asami, K., Hanada, A., Umehara, M., Akiyama, K., et al. (2014). Carlactone is an endogenous biosynthetic precursor for strigolactones. Proc. Natl. Acad. Sci. U.S.A. 111, 1640–1645. doi: 10.1073/pnas.1314805111
Steinkellner, S., Lendzemo, V., Langer, I., Schweiger, P., Khaosaad, T., Toussaint, J. P., et al. (2007). Flavonoids and strigolactones in root exudates as signals in symbiotic and pathogenic plant-fungus interactions. Molecules 12, 1290–1306. doi: 10.3390/12071290
Tanaka, A., Christensen, M. J., Takemoto, D., Park, P., and Scott, B. (2006). Reactive oxygen species play a role in regulating a fungus-perennial ryegrass mutualistic interaction. Plant Cell 18, 1052–1066. doi: 10.1105/tpc.105.039263
Thomma, B. P., Eggermont, K., Tierens, K. F., and Broekaert, W. F. (1999). Requirement of functional ethylene-insensitive 2 gene for efficient resistance of Arabidopsis to infection by Botrytis cinerea. Plant Physiol. 121, 1093–1102. doi: 10.1104/pp.121.4.1093
Thomma, B. P. H. J., Eggermont, K., Penninckx, I. A. M. A., Mauch-Mani, B., Vogelsang, R., Cammue, B. P. A., et al. (1998). Separate jasmonate-dependent and salicylate-dependent defense-response pathways in Arabidopsis are essential for resistance to distinct microbial pathogens. Proc. Natl. Acad. Sci. U.S.A. 95, 15107–15111. doi: 10.1073/pnas.95.25.15107
Torres-Vera, R., García, J. M., Pozo, M. J., and Juan, A. L. (2016). Physiological and molecular plant pathology expression of molecular markers associated to defense signaling pathways and strigolactone biosynthesis during the early interaction tomato- Phelipanche ramosa. Physiol. Mol. Plant Pathol. 94, 100–107. doi: 10.1016/j.pmpp.2016.05.007
Torres-Vera, R., García, J. M., Pozo, M. J., and López-Ráez, J. A. (2014). Do strigolactones contribute to plant defence? Mol. Plant Pathol. 15, 211–216. doi: 10.1111/mpp.12074
Umehara, M., Hanada, A., Yoshida, S., Akiyama, K., Arite, T., Takeda-Kamiya, N., et al. (2008). Inhibition of shoot branching by new terpenoid plant hormones. Nature 455, 195–200. doi: 10.1038/nature07272
van Zeijl, A., Liu, W., Xiao, T. T., Kohlen, W., Yang, W., Bisseling, T., et al. (2015). The strigolactone biosynthesis gene DWARF27 is co-opted in rhizobium symbiosis. BMC Plant Biol. 15:260. doi: 10.1186/s12870-015-0651-x
Venkateshwaran, M., Volkening, J. D., Sussman, M. R., and Ané, J. M. (2013). Symbiosis and the social network of higher plants. Curr. Opin. Plant Biol. 16, 118–127. doi: 10.1016/j.pbi.2012.11.007
Visentin, I., Vitali, M., Ferrero, M., Zhang, Y., Ruyter-Spira, C., Novák, O., et al. (2016). Low levels of strigolactones in roots as a component of the systemic signal of drought stress in tomato. New Phytol. 212, 954–963. doi: 10.1111/nph.14190
Vogel, J. T., Walter, M. H., Giavalisco, P., Lytovchenko, A., Kohlen, W., Charnikhova, T., et al. (2009). SlCCD7 controls strigolactone biosynthesis, shoot branching and mycorrhiza-induced apocarotenoid formation in tomato. Plant J. 61, 300–311. doi: 10.1111/j.1365-313X.2009.04056.x
Wang, Y., Sun, S., Zhu, W., Jia, K., Yang, H., and Wang, X. (2013). Strigolactone/MAX2-induced degradation of brassinosteroid transcriptional effector BES1 regulates shoot branching. Dev. Cell 27, 681–688. doi: 10.1016/j.devcel.2013.11.010
Waters, M. T., Gutjahr, C., Bennett, T., and Nelson, D. C. (2017). Strigolactone signaling and evolution. Annu. Rev. Plant Biol. 68, 291–322. doi: 10.1146/annurev-arplant-042916-040925
Waters, M. T., Scaffidi, A., Sun, Y. K., Flematti, G. R., and Smith, S. M. (2014). The karrikin response system of Arabidopsis. Plant J. 79, 623–631. doi: 10.1111/tpj.12430
Yoneyama, K., Xie, X., Kim, H., Kisugi, T., Nomura, T., Sekimoto, H., et al. (2012). How do nitrogen and phosphorus deficiencies affect strigolactone production and exudation? Planta 235, 1197–1207. doi: 10.1007/s00425-011-1568-8
Yuan, Z., Zhang, C., and Lin, F. (2009). Role of diverse non-systemic fungal endophytes in plant performance and response to stress: progress and approaches. J. Plant Growth Regul. 29, 116–126. doi: 10.1007/s00344-009-9112-9
Zahoor, M., Irshad, M., Rahman, H., Qasim, M., Afridi, S. G., Qadir, M., et al. (2017). Alleviation of heavy metal toxicity and phytostimulation of Brassica campestris L. by endophytic Mucor sp. MHR-7. Ecotoxicol. Environ. Saf. 142, 139–149. doi: 10.1016/j.ecoenv.2017.04.005
Zhu, S., Tang, J., Zeng, X., Wei, B., Yang, S., and Huang, B. (2015). Isolation of Mucor circinelloides Z4 and Mucor racemosus Z8 from heavy metal-contaminated soil and their potential in promoting phytoextraction with Guizhou oilseed rap. J. Cent. South Univ. 22, 88–94. doi: 10.1007/s11771-015-2498-6
Keywords: strigolactone, fungal endophytes, Arabidopsis thaliana, salicylic acid, symbiosis, jasmonic acid
Citation: Rozpądek P, Domka AM, Nosek M, Ważny R, Jędrzejczyk RJ, Wiciarz M and Turnau K (2018) The Role of Strigolactone in the Cross-Talk Between Arabidopsis thaliana and the Endophytic Fungus Mucor sp. Front. Microbiol. 9:441. doi: 10.3389/fmicb.2018.00441
Received: 31 August 2017; Accepted: 26 February 2018;
Published: 19 March 2018.
Edited by:
Mohamed Hijri, Université de Montréal, CanadaReviewed by:
George Newcombe, University of Idaho, United StatesMika Tapio Tarkka, Helmholtz-Zentrum für Umweltforschung (UFZ), Germany
Copyright © 2018 Rozpądek, Domka, Nosek, Ważny, Jędrzejczyk, Wiciarz and Turnau. This is an open-access article distributed under the terms of the Creative Commons Attribution License (CC BY). The use, distribution or reproduction in other forums is permitted, provided the original author(s) and the copyright owner are credited and that the original publication in this journal is cited, in accordance with accepted academic practice. No use, distribution or reproduction is permitted which does not comply with these terms.
*Correspondence: Piotr Rozpądek, cGlvdHIucm96cGFkZWtAdWouZWR1LnBs