- 1Shandong Province Key Laboratory of Applied Mycology, Qingdao International Center on Microbes Utilizing Biogas, School of Life Science, Qingdao Agricultural University, Qingdao, China
- 2Central Laboratory, Qingdao Agricultural University, Qingdao, China
- 3Tianjin Animal Science and Veterinary Research Institute, Tianjin Academy of Agricultural Sciences, Tianjin, China
- 4School of Chemistry and Pharmacy, Qingdao Agricultural University, Qingdao, China
- 5Department of Chemistry, University of Washington, Seattle, WA, United States
- 6Department of Biochemistry and Molecular Biology, School of Basic Medical Sciences, Tianjin Medical University, Tianjin, China
- 7School of Chemical Engineering and Technology, Tianjin University, Tianjin, China
- 8Key Laboratory of Systems Bioengineering, Ministry of Education, Tianjin University, Tianjin, China
The co-culture of Trametes versicolor and Ganoderma applanatum is a model of intense basidiomycete interaction, which induces many newly synthesized or highly produced features. Currently, one of the major challenges is an identification of the origin of induced features during the co-culture. Herein, we report a 13C-dynamic labeling analysis used to determine an association of induced features and corresponding fungus even if the identities of metabolites were not available or almost nothing was known of biochemical aspects. After the co-culture of T. versicolor and G. applanatum for 10 days, the mycelium pellets of T. versicolor and G. applanatum were sterilely harvested and then mono-cultured in the liquid medium containing half fresh medium with 13C-labeled glucose as carbon source and half co-cultured supernatants collected on day 10. 13C-labeled metabolome analyzed by LC-MS revealed that 31 induced features including 3-phenyllactic acid and orsellinic acid were isotopically labeled in the mono-culture after the co-culture stimulation. Twenty features were derived from T. versicolor, 6 from G. applanatum, and 5 features were synthesized by both T. versicolor and G. applanatum. 13C-labeling further suggested that 12 features such as previously identified novel xyloside [N-(4-methoxyphenyl)formamide 2-O-beta-D-xyloside] were likely induced through the direct physical interaction of mycelia. Use of molecular network analysis combined with 13C-labeling provided an insight into the link between the generation of structural analogs and producing fungus. Compound 1 with m/z 309.0757, increased 15.4-fold in the co-culture and observed 13C incorporation in the mono-culture of both T. versicolor and G. applanatum, was purified and identified as a phenyl polyketide, 2,5,6-trihydroxy-4, 6-diphenylcyclohex-4-ene-1,3-dione. The biological activity study indicated that this compound has a potential to inhibit cell viability of leukemic cell line U937. The current work sets an important basis for further investigations including novel metabolites discovery and biosynthetic capacity improvement.
Introduction
Secondary metabolites are an important source of valuable drug leads, of which compounds derived from various fungi, especially medicinal fungi of basidiomycetes, represent an important part. To explore chemical diversity, several approaches such as epigenetic modification or non-targeted metabolic pathway manipulation have recently been developed in the Aspergillus and Streptomyces species (Scherlach and Hertweck, 2009; Chiang et al., 2011). In the case of basidiomycetes, the induction of novel secondary metabolites or enhancement of secreting extracellular enzymes can be achieved by activating cryptic biosynthetic pathways through establishment of a fungi interaction in the co-culture (Peiris et al., 2008; Hiscox et al., 2010). This co-culture strategy mimics natural ecosystem, in which interspecies interaction of basidiomycetes is very common (Hiscox et al., 2015, 2017). Recently, Zheng et al. demonstrated that co-culture of basidiomycetes Inonotus obliquus and Phellinus punctatus resulted in the accumulation of lanostane-type triterpenoids, polyphenols, and melanins, compounds capable of scavenging free radicals and inhibiting tumor cell proliferation (Zheng et al., 2011). The co-culture of Trichoderma Reesei with Coprinus comatus was an important approach for an on-site generation of lignocellulolytic enzyme leading to the increase of lignocellulose degradation rate as described by Ma and Ruan (2015). In our previous work, fifteen wood-decaying basidiomycetes and two straw-decaying basidiomycetes were used to establish 136 pairwise co-cultures on agar plates (Yao et al., 2016). The co-culture system of Trametes versicolor and Ganoderma applanatum showed an interaction zone, in which the accumulation of a series of known carboxylic acids as well as novel xylosides were observed.
The application of co-culture has enhanced the number of discovered novel secondary metabolites but also raises several challenges. One of the major challenges is how to timely and accurately identify the species responsible for the newly induced metabolites during the microorganism interaction. Typically, the structure of compounds and related biochemical information were required to verify the unique secondary metabolite and its particular producer (Riedlinger et al., 2006). Recently, a combined technique of nanospray desorption electrospray ionization and matrix-assisted laser desorption/ionization-time of flight mass spectrometry has been developed as a platform to reveal many unknown metabolites produced by Streptomyces coelicolor when paired with five other actinomycetes (Traxler et al., 2013). However, this approach was limited to associate the novel metabolites with corresponding producer grown on agar plates and was not suitable to the co-culture in the liquid medium. The metabolic incorporation of stable isotopes 13C or 15N is a powerful approach used for quantitative proteome and metabolome studies (Julka and Regnier, 2004; Yang et al., 2010). Moreover, 13C-dynamic labeling analysis has been applied for analyzing the metabolite turnover rates, distinguishing the flux distribution between two pathways starting from the same metabolic point, and interpreting the synthetic process of novel metabolites (Yang et al., 2012; Shlomi et al., 2014; Hammerl et al., 2017). With the advantage of a 13C-labeling approach, the current study reports on the identification of the producer of novel induced metabolites in liquid medium after the two interactive mycelia were sterilely separated and mono-cultured after the induction by the co-culture.
The research of the interspecies crosstalk expands our possibilities to discover novel metabolites, and also increases our understanding of how these metabolites are induced in microbial consortia. A chemical warfare in the fungal-fungal communication is often described as diffusion of harmful and chemically complex metabolites from one partner to the other (Bertrand et al., 2014). For instance, the interaction between the Aspergillus niger and Aspergillus flavus led to the inhibition of aflatoxin B1 produced by A. flavus through signal molecules downregulating expression of major biosynthetic genes (Xing et al., 2017). Under some conditions, co-cultivation appears to trigger production and accumulation of novel metabolites without the involvement of released signaling molecules. For instance, co-cultivation with Corynebacteria glutamicum or Tsukamurella pulmonis was indicated to stimulate a novel pathway in S. endus, contributing to a new heterocyclic chromophore-containing antibiotics alchivemycin A (Onaka et al., 2011). Notably, the mono-culture of S. endus did not generate the same compound with or without the addition of filter sterilized supernatants from bacterial culture. The production of alchivemycin A therefore appeared to require a direct physical interaction between S. endus and the coryneform bacteria cells. For this research, 13C dynamic labeling was further utilized to suggest the potential mechanism of the induction of increased and newly synthesized features.
In this work, we built upon the characteristic of mycelial pellets of basidiomycetes and 13C-labeling analysis, to analyze and catalog a broad range of 13C-labeled features, which were highly accumulated in the co-culture of T. versicolor and G. applanatum. 13C dynamic labeling further suggested two potential mechanism of the induction of these features. Ultimately, compound 1 with M+H+ m/z 309.0757, that displayed 13C incorporation in the mono-culture of both T. versicolor and G. applanatum, was isolated and identified as novel phenyl polyketide, and its biological activity was evaluated.
Materials and Methods
Chemicals
All chemicals including standards (ascorbic acid and phenolic antioxidant 2,6-di-tert-butyl-4-methoxyphenol) were purchased from either Sigma-Aldrich (St. Louis, MO, USA) or TCI (Kita-ku, Tokyo, Japan). Millipore water (Billerica, MA, USA) was used for the preparation of all the media and sample solutions.
Fungi Material and Culture Conditions
G. applanatum (CGMCC No. 5.249) and T. versicolor (CGMCC No. 12241) were deposited at the Shandong Province Key Lab of Applied Mycology in China. The culture medium was supplied at concentrations as follows: 2 g glucose, 0.2 g KH2PO4, 0.1 g MgSO4, 0.4 g peptone, and 4 g agar (only in solid medium) in 200 mL of sterilized water.
Mono-culture of T. versicolor and G. applanatum on Agar Plate
The mono-culture procedure was adapted from our previous publication (Yao et al., 2016), and mono-culture medium was the same as above. Briefly, a 5 mm agar plug of each fungus scraped from agar slant culture-medium was cultured on a Petri dish (9 cm diameter), and were incubated at 28°C for 10 days.
Co-culture of T. versicolor and G. applanatum in Liquid Medium and Sample Preparation
Eight 5 mm agar plugs of T. versicolor and G. applanatum were separately pre-cultured in 500 mL shake flask containing 200 mL of culture medium at 28°C for 4 days on orbital shakers at 180 rpm. Then 100 mL culture broth of G. applanatum was transferred into the culture of 100 mL T. versicolor, and co-cultivated up to 18 days. All the co-cultures had three independent biological replicates. At the harvest time, 10 mL of co -culture broth was filtered by using MILLEX-GP PES membrane filters (0.22 μm, 33 mm, Merck Millipore, Germany) and the filtrate was dried in a freeze-dryer ALPHA 1-2LDplus (Christ, Osterode, Germany). Five milliliters of freshly prepared dichloromethane/methanol/water (64:36:8, v/v) solvent mixture was added to the dried samples (Yao et al., 2016). The sample extractions were carried out in a water bath sonicator (KQ-300GVDV, Kunshan, China) at 25°C for 20 min, and were centrifuged at 12,000 rpm for 10 min. Finally, the extracts were dried on a rotational vacuum concentrator (Christ, Osterode, Germany) and stored in a −80°C freezer.
Measurement of the Metabolome
The extracts were dissolved in 200 μL methanol, and then were centrifuged at 12,000 rpm for 15 min. The supernatants were transferred into 250 μL Agilent autosampler vials. The samples were analyzed on an Agilent liquid chromatograph-quadrupole time-of-flight mass spectrometer (LC-QTOF-MS, Agilent 1290 Infinity-6530B, Agilent Technologies, Santa Clara, CA, USA) as previously described (Cui et al., 2016; Hu et al., 2016). Briefly, 10 μL of the samples was separated on an Acquity UPLC BEH C18 column (100 × 2.1 mm, 1.7 μm, Waters, Milford, MA, USA). The mobile phase A was water with 0.1% formic acid. The mobile phase B was pure acetonitrile. The reversed-phase liquid chromatographic elution gradient was optimized in order to maximize the resolution of the induced features. The gradient was the following: 0–3 min, 5% B, 3–12 min, 5–40% B, 12–38 min, 40–95% B, 38–46 min, 95% B, 46–48 min, 95–5% B, 48–55 min, 5% B. This shallow gradient provided reproducible separation for many co-eluting compounds in a time window from 5.0 to 8.0 min. The TOF m/z range was set to 50–1,200 amu in centroid mode with a scan rate of 1.5 spectra/s. All the samples had three independent biological replicates. Each biological replicate had two analytical replicates.
Data Pre-processing and Principal Component Analysis
LC-QTOF-MS data were converted into mzML format using MS Convert software (Holman et al., 2014). Data pre-processing and statistical analysis were performed with MZmine 2 (Version 2.11) (Pluskal et al., 2010) and SIMCA-P 11.5. For the MZmine 2, the peak detection threshold for MS signal intensity was set to 1.0 × 103. The chromatogram building was realized using a minimum time span of 0.01 min, minimum height of 2.5 × 103, and m/z tolerance of 0.005 (or 10 ppm). Chromatograms were deconvoluted with the following settings: search minimum in absolute retention time (RT) range 0.1 min, minimum relative height 10%, minimum absolute height 2.5 × 103 and baseline level 1.2. The chromatogram isotopic peaks grouper algorithm was set as m/z tolerance of 0.005 (or 10 ppm) and absolute RT tolerance of 0.10 min. Chromatograms were peak aligned with m/z tolerance at 0.008 (or 15 ppm) and absolute RT tolerance 1 min. The peak list was eventually gap-filled with m/z tolerance at 0.008 (or 15 ppm), and absolute RT tolerance of 0.20 min. To classify m/z in the peak list, principal component analysis (PCA) was carried out by using SIMCA-P (version 11.5). Two steps were required to perform PCA. The first step was to set the variables m/z and RT as Primary ID and Secondary ID. The secondary step was to do the normalization of the variables with Pareto scaling. This normalized method was embedded in SIMPCA-P, which did not require further parameter tuning. After that, PCA were displayed by a scores plot, mainly observing the overall cluster of the different treatments as well as the presence of outliers. The correlation coefficient loading plot was used to identify the variables responsible for the clustering or separation of the treatments.
Molecular Network Analysis
MS/MS data for molecular network analysis were acquired in targeted MS/MS mode on the same LC-QTOF-MS system (the precursor ions are listed in Table 1). The collision energy and m/z range for different precursor ions were optimized based on their own characteristics as our previous publication (Yao et al., 2016). MS/MS data were converted to mzML format, and then were subjected to the Molecular Networking workflow of Global Natural Products Social Molecular (GNPS at gnps.ucsd.edu) using the Group Mapping feature (Watrous et al., 2012; Wang et al., 2016). The subnetworks were generated with settings of minimum pairs cosine 0.65, parent mass tolerance 1.0 Dalton, ion tolerance 0.5 Dalton, maximum connected components 50, minimum matched peaks 6, minimum cluster size 2. The results were then visualized using Cytoscape (Version 3.1.1) (Su et al., 2014).
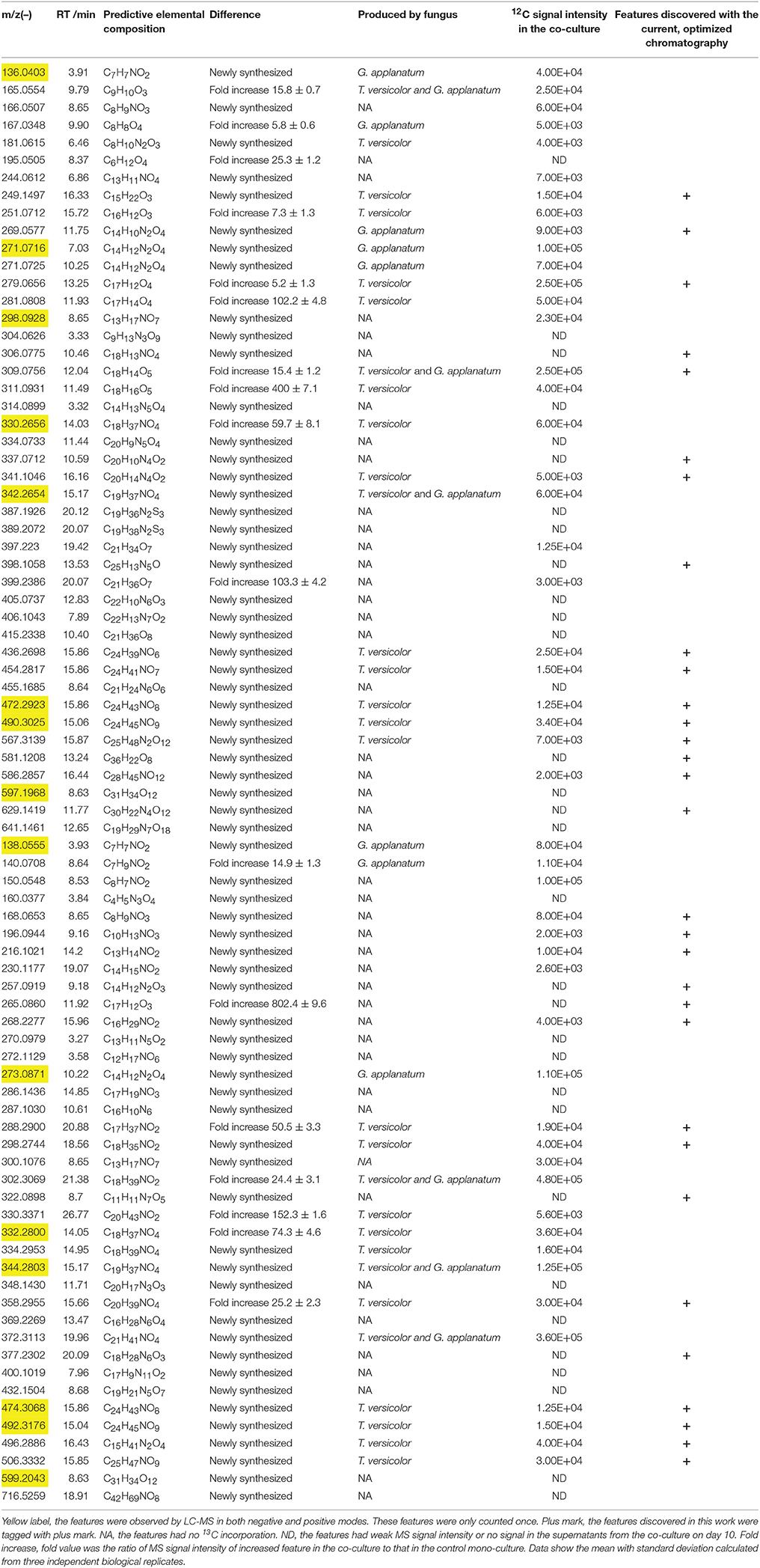
Table 1. List of induced features in the co-culture of T. versicolor and G. applanatum analyzed by LC-MS in the positive/negative mode and corresponding production fungus.
13C-Labeling Analysis
T. versicolor and G. applanatum were co-cultivated up to 10 days as described above. Then mycelium pellets of T. versicolor and G. applanatum were respectively harvested using sterilized tweezers based on the difference of diameter size and color. The mycelium pellets of T. versicolor had the size ranging from 6 to 8 mm with the color of faint yellow and those of G. applanatum had the size ranging from 2 to 4 mm with red color. The harvested mycelium pellets were washed with sterile water three times and then mono-cultured in 50 ml shake flask containing 10 mL fresh medium with 13C-labeled glucose at the final concentration of 5 g L−1 and 10 mL of co-cultured supernatants collected on day 10. For the control experiment no co-culture supernatants were added, only 20 mL of fresh medium with 13C-labeled glucose as carbon source. After the mono-culture for 10 or 20 days, 10 mL of T. versicolor and G. applanatum culture broths were harvested for analysis of 13C-labeling. All samples had three independent biological replicates and two analytical replicates. Samples were extracted and analyzed by LC-QTOF-MS as described above. The mass isotopomer distributions were corrected for the contribution from natural isotopes by a matrix-based method (Jennings and Matthews, 2005). The total 13C-incorporation for each feature was obtained by normalizing to its total carbon number as in our previous publication (Yang et al., 2013). Relative isotopic abundance (Mi) for a feature in which i 13C atoms were incorporated was calculated by the Equation (1):
where mi represents the isotopic abundance for a feature in which i 13C atoms were incorporated and n represents the maximum number of 13C atoms incorporated.
Total 13C-incorporation of a feature with N carbon atoms was obtained by normalizing to its total carbon number according to the Equation (2):
The significance of difference of 13C-incorporation between experimental data points was determined by t-tests (Origin 8.0). A P-value <0.05 was considered to be statistically significant.
Isolation and Purification of Compound 1
Twenty liters of the co-cultured supernatant was extracted three times with ethyl acetate (EtOAc). 2.5 g of crude EtOAc extract was concentrated under reduced pressure and purified on a silica gel (200–300 mesh) column and then eluted with petroleum ether/ethyl acetate and chloroform/methanol system to yield ten fractions (Song et al., 2014). Eight hundred and fifty milligrams of fraction 6 and 7 from the chloroform/methanol elution (20:1 and 10:1, v/v) was used to a medium pressure liquid chromatography (Flash CO140080-0, Agela Technologies, China) and eluted with methanol/water (the elution gradient was 10–90% methanol in 65 min) to generate a mixture of 216 mg. This mixture was purified on a silica gel (200–300 mesh, Qingdao Haiyang Chem. Ind. Co. Ltd. China) column by eluting with petroleum ether/ethyl acetate/ methanol (3:3:1, v/v) and then separated on a preparative column (Venusil XBP C18, Agela Technologies, China) by eluting with methanol/water (the elution gradient was 10–50% methanol in 40 min and flow rate was 8 mL/min) to obtain 10 mg of compound 1.
NMR Analysis of Purified Compound 1
1H, 13C, and 2D NMR spectra of the purified compound 1 were all performed by using a Bruker Avance 600 MHz spectrometer (Karlsruhe, Germany) at 25°C. The compound 1 was accurately weighted, and dissolved in 0.5 mL of deuterated methanol as the internal lock. The resulting spectra were manually phased and baseline corrected and calibrated to methanol, using TOPSPIN (Version 2.1, Bruker).
Cell Viability Assay
The human leukemic cell line U937 and lung cancer cell line A549 were purchased from American Type Culture Collection (Manassas, VA, USA). They were cultured in RPMI-1640 (HyClone, USA) with 10% FBS (fetal bovine serum, Gibco). Cells were inoculated in 96-well plates at a density of 3,000 cells per well overnight and then treated to different concentrations of compound 1 for 48 h. The compound 1 was solved in ethanol. At a concentration of 300 μM of compound 1, the ethanol was 0.8% (v/v) in the cell culture medium, showing minimal effect on the viability of both cell lines. The effect of compound 1 on the viability of U937 and A549 cells was evaluated with CellTiter 96®AQueous One Solution (Promega, Madison, WI, USA) (Soman et al., 2009). The absorbance was read at 490 nm with a microplate spectrophotometer (Multiskan FC, Thermo scientific, USA). Three independent experiments were performed and each one had six replicates. Data show the mean with error bar indicating standard deviation calculated from biological replicates by Origin 8.0. IC50 defined as the concentration with the inhibition of 50% cells was calculated by using SPSS (Statistical Package for Social Sciences) package 6, version 15.0.
Antioxidant Activity Assay
The 2,2′-azinobis-(3-ethylbenzothiazoline-6-sulfonic acid) (ABTS) method used is based on the reduction of the ABTS•+ radical action by the antioxidants present in the sample (Rigano et al., 2014). Three biological replicates were performed. A solution of 7.4 mM ABTS•+ (5 mL) mixed with 140 mM K2S2O8 (88 μL) was prepared and stabilized for 12 h at 4°C in the dark. This mixture was then diluted by mixing ABTS•+ solution with ethanol (1:88) to obtain an absorbance of 0.70 ± 0.10 unit at 734 nm using a spectrophotometer (Multiskan FC, Thermo scientific, USA). Compound 1 (100 μL) and the standard controls (ascorbic acid and phenolic antioxidant 2,6-di-tert-butyl-4-methoxyphenol) reacted with 1 mL of diluted ABTS•+ solution for 2.5 min, and then the absorbance was taken at 734 nm against a blank constituted by ABTS•+ solution added with 100 μL of ethanol. ABTS•+ scavenging activity was calculated by the Equation (3):
where Abs blank = 100 μL of ethanol+1 mL of diluted ABTS•+ solution.
Results
Induced Feature Discovery in Fungal Interaction
Unsupervised PCA is well-suited for comparing different biological samples and identifying statistically significant differences (Chen et al., 2007). As shown in Figure 1 (left figure), examination of the scores plot showed that the co-culture treatment is clearly separated from the two mono-cultures. The variable features responsible for discriminating these three groups are shown in the loading plots (Figure 1, right). More than 4,000 features were recorded, out of which 58 features were detected only in the co-culture and 16 features were at least 5-fold more abundant in the co-culture than those in the control mono-culture (Table 1, Figure 2). Compared to our previous work (Yao et al., 2016), 28 additional features were discovered mainly due to the optimized chromatography (Table 1, Figure 2).
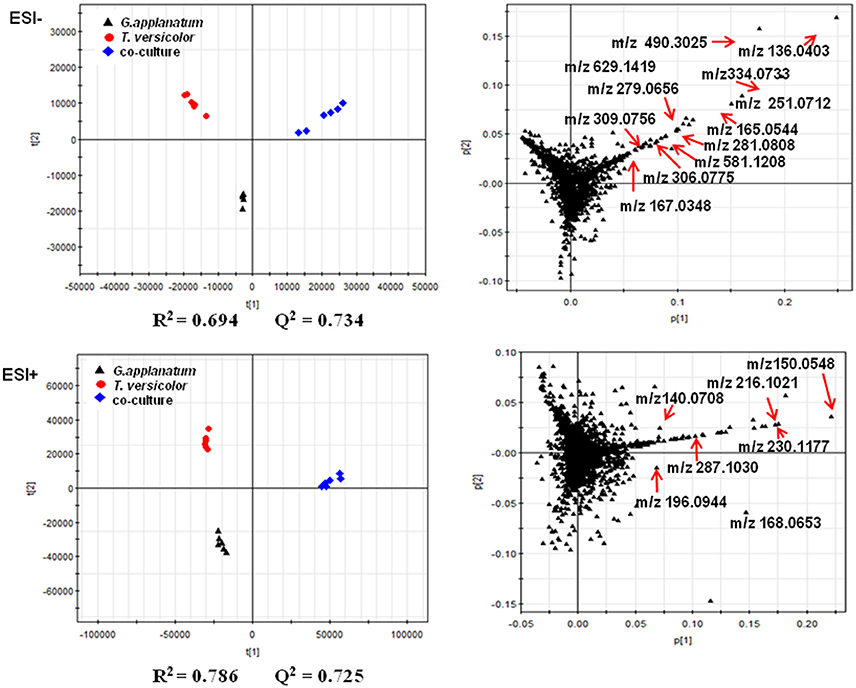
Figure 1. PCA of metabolomics data of co-culture and their corresponding mono-cultures on day 18 is shown. The score and loading plots of the data analyzed by LC-QTOF-MS in the negative mode (2287 features) and positive mode (2076 features), respectively. The parameters (R2 and Q2) of the score plots demonstrated the discriminative ability of this model. The scattered dots labeled with m/z were representative features mentioned in the Results section.
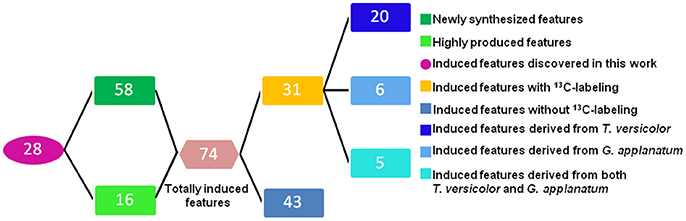
Figure 2. Overview of the number of the induced features in the co-culture of T. versicolor and G. applanatum.
13C-Labeling Analysis to Associate Induced Features with Corresponding Fungus
To associate induced features with corresponding fungus, we initially harvested individual mycelium pellets of T. versicolor and G. applanatum after the co-culture for 10 days and then detected the abundance of in vivo induced features. Many of the induced features were observed for both samples of T. versicolor and G. applanatum (data not shown). This made it impossible to distinguish if individual or both fungi were induced to generate the compounds in the co-culture. To overcome this issue, we designed a 13C-labeling approach in the liquid co-cultivation. The workflow is shown in Figure 3. First, T. versicolor and G. applanatum were co-cultivated for 10 days to activate the cryptic genes. Next, their mycelia were sterilely separated and mono-cultivated in the liquid medium which contained half of fresh medium with 13C-labeled glucose as carbon source and half of co-cultured supernatants collected on day 10. Then, the samples were harvested in the mono-cultures of T. versicolor and G. applanatum on days 10 and 20 and analyzed by LC-QTOF-MS. In the preliminary experiments, the samples were also harvested after 5 days of mono-culture with the addition of the supernatants. However, many of induced features were only slightly labeled. It was likely due to a relatively long lag phase and low growth rate in the mono-culture of T. versicolor and G. applanatum after the stimulation of the co-culture. In addition, for the unlabeled features, 13C-labeling was not detected on day 5 either. Therefore, the samples were harvested later in order to obtain the strong MS signal. As the incorporation of 13C-labeled carbons from glucose increases the molecular weight of metabolites, the mass shift determined from the mass spectra provides then the information about which fungi generated the induced features.
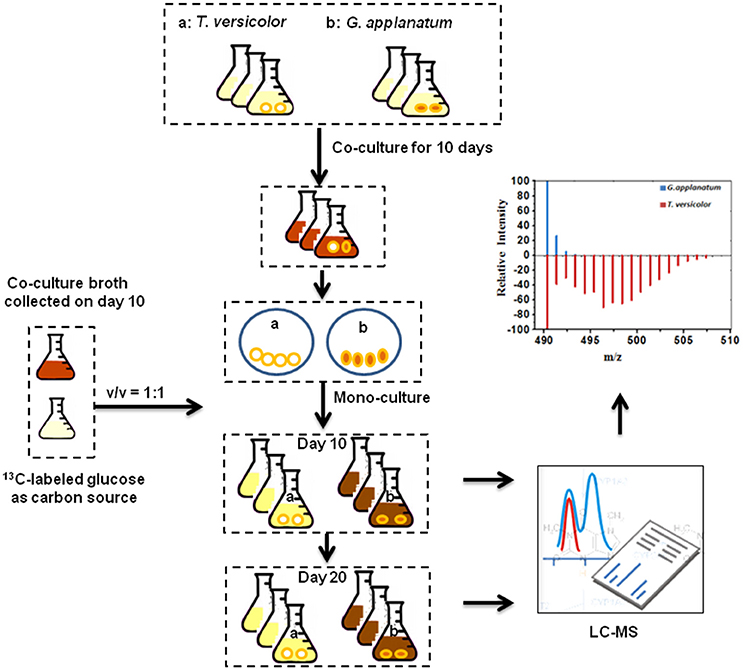
Figure 3. 13C-labeling analysis procedure. T. versicolor and G. applanatum were co-cultivated for 10 days, and then their mycelia were sterilely separated and mono-cultivated in the liquid medium which contained half of fresh medium with 13C-labeled glucose as carbon source and half of co-cultured supernatants. Then samples were harvested in the mono-cultures of T. versicolor and G. applanatum and analyzed on LC-QTOF-MS.
Total 31 induced features were found to have 13C incorporation in the mono-culture, and 43 features did not incorporate any label (Figure 2). Among the labeled features activated by the co-culture, 20 originated from T. versicolor and 6 features were derived from G. applanatum. Five features were induced by both T. versicolor and G. applanatum (Figure 2). Several representative 13C labeling results are shown in Figure 4. In Figure 4A, the m/z 490 (experimental m/z 490.3025, predicted elemental component C24H45NO9, error 0.3 ppm) was a newly synthesized feature in the co-culture. As 13C-labeled carbon was incorporated into the feature m/z 490, intensity of m/z 491 (m/z + 1) up to m/z 506 (m/z + 16) increased. This change was observed only in the mono-culture of T. versicolor and indicates that m/z 490 was particularly induced in this species. The calculation of total 13C incorporation indicated that about 19 and 25% carbon was replaced with 13C on days 10 and 20, respectively (bottom part of Figure 4A). The m/z 167.0348 in Figure 4B was highly produced during the co-culture and identified as orsellinic acid in the previous publication (Yao et al., 2016). In contrast to the above example, the m/z 167 incorporated 13C-labels in the same time frame only in the mono-culture of G. applanatum, resulting in the parent ions shifted from m/z 167 to m/z 174 in the 13C-labeled mass spectra and total 13C incorporation level reached 14% on day 20 (Figure 4B). The m/z 165.0554 was previously identified as 3-phenyllactic acid (Yao et al., 2016), and 13C-labeled mass spectra ranging from m/z 165 to m/z 174 were observed in both mono-cultures of T. versicolor and G. applanatum (Figure 4C). Similar example, feature with m/z 309 (experimental m/z 309.0756, C18H14O5, error 0.37 ppm) increased 15.4-fold during the co-culture in comparison with MS signal in the control mono-culture and 13C-labeling was observed for both fungi (Figure 4D). It is worth mentioning that a contamination from the undesired fungus cannot be absolutely excluded during the transfer from the co-culture to the mono-culture, but that it does not affect the identification of the fungus producing a feature only found in the mono-culture of either T. versicolor or G. applanatum based on the 13C-labeling analysis.
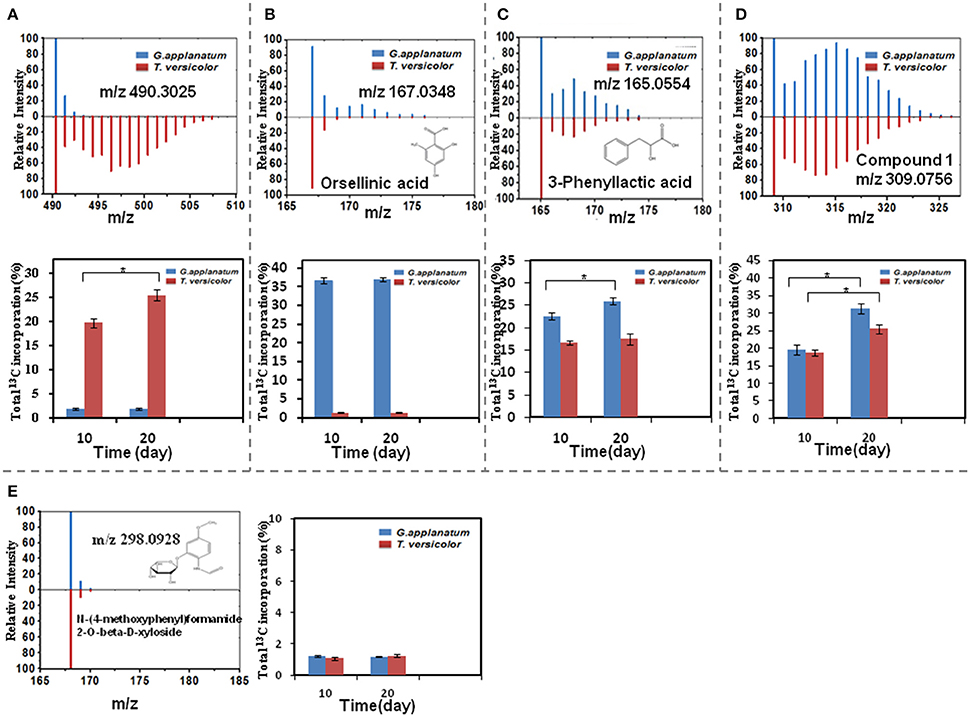
Figure 4. A comparison of relative MS intensity suggests that the induced features have 13C incorporation with time. (A–D) Upper figures: representative mass spectral data for 10 day time point showing that mass intensity caused by 13C incorporation in the mono-culture of T. versicolor or G. applanatum, Lower figures: comparison of total 13C incorporation shows that induced feature was produced by either T. versicolor or G. applanatum, or both. Data show the mean with error bars indicating standard deviation calculated from three independent replicates (*P ≤ 0.05, t-test). (E) There was no 13C incorporation for N-(4-methoxyphenyl)formamide 2-O-beta-D-xyloside on day 10 and 20.
13C incorporation could be due to the induction of features which have been released into the medium during the co-culture. To confirm whether diffusible features were involved in triggering the silent gene expression in this study, we treated T. versicolor and G. applanatum with fresh medium containing 13C labeled glucose as carbon source but without the addition of the supernatant of co-culture on day 10. As shown in Figure 5 as an example, 13C labeling of m/z 490.3025 and 136.0403 was not observed up to 20 days but when the supernatant was added the features were significantly labeled. Notably, for 12 induced features with high signal intensities, including newly synthesized xyloside [N-(4-methoxyphenyl)formamide 2-O-beta-D-xyloside; Yao et al., 2016], we did not detected any 13C incorporation in the mono-culture of either fungus (Table 1, Figure 4E, Supplementary Figure 1D). Therefore, it was not possible to assign their origin to a specific fungus.
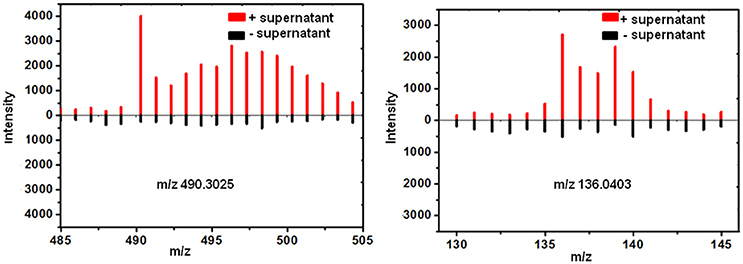
Figure 5. 13C incorporation was not observed for the induced features on day 20 in the mono-culture of T. versicolor (Left) or G. applanatum (Right) without the addition of the supernatant of co-culture.
Dereplication of Newly Discovered Features by Molecular Network Analysis
To investigate the structural similarities occurring for 28 newly discovered features in this study, MS/MS fragmentation spectra of the induced features in Table 1 were processed and organized as molecular network with the previously identified features. Figure 6 (upper part) shows a constructed subnetwork to dereplicate m/z 196.0944 and 216.1021. These two new features likely possessed similar backbone structure with the previously identified N-(2-hydroxy-4-methoxyphenyl) formamide (m/z 168.0653) and its analogous features (m/z 140.0708, 150.0548, 230.1177, 287.1030) due to their close fragmentation patterns (Supplementary Figure 2). This data was also in agreement with 13C-labeling analysis, in which most of features involved in this subnetwork were not labeled either. In another example, five newly identified features and three previous features (m/z 251.0712, 281.0808, and 334.0733) were clustered together with high scores (Figure 6, bottom part). Comparison of the fragment ions of m/z 306.0775, 334.0733, 581.1208, 629.1419, and 279.0656 showed some common ions of m/z 77.04, 117.03, 235.08 which were likely derived from fragmentation of m/z 279.0656, suggesting that these features probably had the same backbone structure and belonged to a series of structural derivatives (Supplementary Figure 2). Moreover, 13C incorporation of m/z 279.0656, 251.0712, and 281.0808 were clearly detected in the mono-culture of T. versicolor with the addition of the supernatant of co-culture (Supplementary Figure 1), suggesting that the derivatives of m/z 279.0656 (i.e., m/z 306.0775, 334.0733, 581.1208, and 629.1419, which did not show 13C incorporation due to the weak signals in the mono-culture) were also likely biosynthesized by T. versicolor under the co-cultured condition.
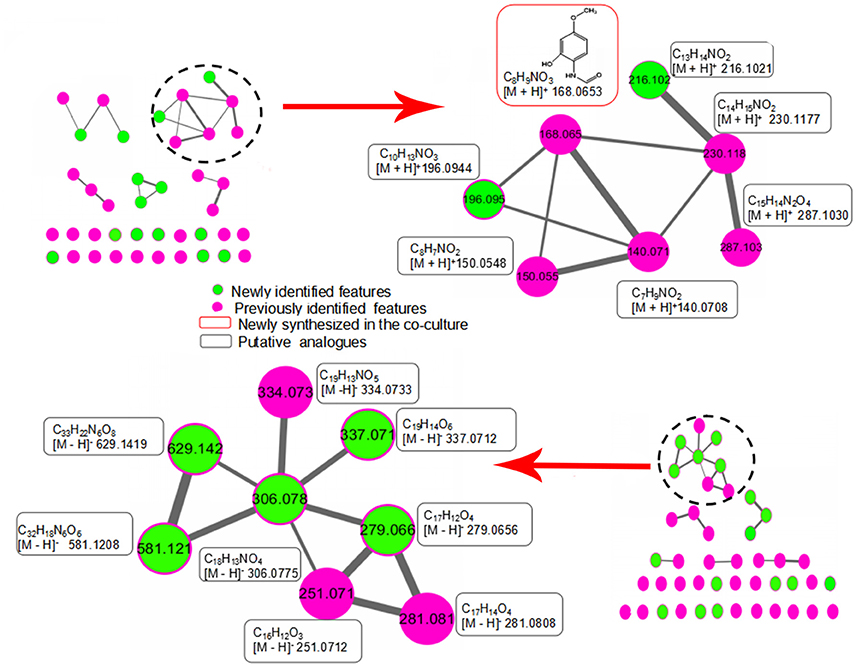
Figure 6. Molecular network analysis of newly discovered features in the co-culture. The green nodes represent parent ions of newly identified features and purple nodes are the previous features, and the thickness of the edges between nodes indicates the degree of similarity between their respective MS/MS spectra. Upper part shows a constructed subnetwork to dereplicate m/z 196.0944 and 216.1021. Lower part shows five newly identified features (m/z 279.0656, 306.0775, 337.0712, 581.1208, 629.1419) and three previous features (m/z 251.0712, 281.0808 and 334.0733) that clustered together.
Identification of Induced Compound 1 and Analysis of Its Biological Activity
Since compound 1 was derived from both T. versicolor and G. applanatum (Figure 4D), and 15.4-fold more abundant in the co-culture, we isolated and purified sufficient amount for detailed characterization. Compound 1 was a pale yellow powder with the molecular formula of C18H14O5. The 1H, 13C-NMR and HSQC spectrum showed the presence of two carbonyl carbon, one oxygen connected CH, one oxygen connected to quaternary carbon, two single benzene rings and two olefinic carbon atoms (one with oxygen attached to it) (Supplementary Table 1 and Supplementary Figure 3). All the information suggested that the basic skeleton of the compound 1 is a terphenyl derivative with two mono-substituted benzene rings. The COSY spectrum showed the 1H–1H spin systems of H-2′/H-3′/H-4′/H-5′/H-6′ and H-2″/H-3″/H-4″/H-5″/H-6″, assigned two mono-substituted benzene rings (A and B). The HMBC correlations from H-2 (δH 4.49) to C1 (δC 203.4), C3 (δC 197.4), C4 (δC 113.5), and C6 (δC 90.9), and from OH-6 (δH 5.44, dimethylsulfoxide (DMSO-d6) to C1 (δC 202.45), C5 (δC 191.28) and C6 (δC 90.9) established the ring C. The fragment ring A was linked to C-6 of ring C supported by the HMBC correlations from H-6' (δH 7.98) to the carbonyl C1 (δC 203.4). Likewise, the linkage of the ring B to the ring C at C-4 was confirmed by HMBC correlations from H-2″ (δH 7.88) to C4 (δC 113.5) and C-1″(δC 135.4). Therefore, the structure of compound 1 was identified as a phenyl polyketide, 2,5,6-trihydroxy-4,6-diphenylcyclohex-4 -ene-1,3-dione (Figure 7).
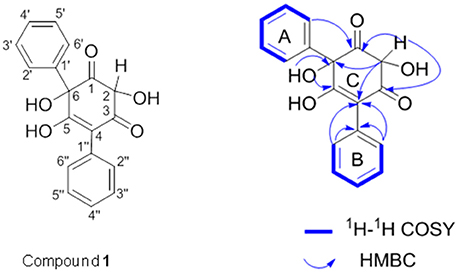
Figure 7. 1H−1H COSY (blue bold lines) and key HMBC (blue arrows) correlations of compound 1. COSY is two-dimensional spectrum which is used to identify spins coupled to each other, and HMBC is two-dimensional inverse H,C correlation technique that allow for the determination of carbon to hydrogen connectivity.
We further tested biological activity of compound 1. The human lung cancer cell lines A549 and leukemic cell lines U937 were treated with compound 1 at various concentrations for 48 h. As shown in Figure 8A, no visible changes in cell viability were detected for human lung cancer cell line A549 when the concentrations were increased to 300 μM. In contrast, compound 1 inhibited the viability of leukemic cells in a dose-dependent manner. The IC50 at 48 h was determined to be 276 ± 5 μM (equal to 85 mg/L). In addition, based on the structural characteristics of compound 1, we also studied whether compound 1 had antioxidant properties using ABTS assay (Figure 8B). The highest percentage of antioxidant capacities (82.65 ± 1.25%) was observed for compound 1 at the concentration of 200 μg/mL. This is comparable with the report of crude extracts from berries (Abu-Bakar et al., 2016). The comparison of the antioxidant activity with ascorbic acid (VC) and phenolic antioxidant 2,6-di-tert-butyl-4-methoxyphenol (BHT) revealed that ascorbic acid> compound 1 > BHT.
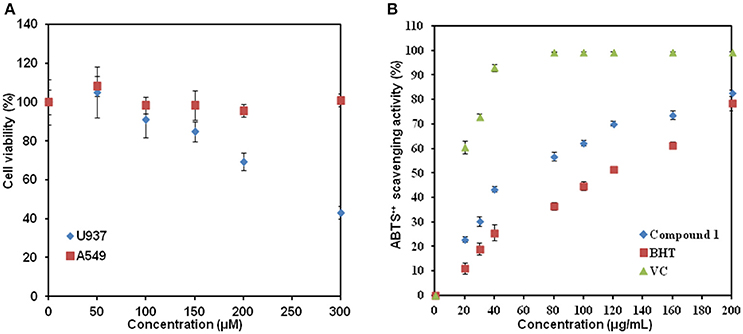
Figure 8. The biological activity study of compound 1. (A) Addition of compound 1 affected the cell viability of U937 but not of A549. (B) Graph of ABTS•+ scavenging activity vs. concentration. Data show the mean with error bars indicating standard deviation calculated from three independent biological replicates. VC, ascorbic acid; BHT, 2,6-di-tert-butyl-4-methoxyphenol.
Discussion
Determining the origin of induced features in the co-culture remains a large challenge, because it generally requires the chemical structures with their biological information. Previously Bertrand et al. discovered five de novo induced compounds from the co-culture of Trichophyton rubrum and Bionectria ochroleuca, and elucidated the origin of one of them based on its non-sulfated form detected in the mono-culture of B. ochroleuca (Bertrand et al., 2013). However, other four compounds could not be associated with corresponding fungi due to the lack of their structures. In another study Ola et al. isolated nine compounds and speculated that four of them detected only in the co-culture originated from Fusarium tricinctum in terms of structural analogies with the known fungal products from the Xylariaceae family, but the producer of remaining five compounds was still unclear because of insufficient biochemical evidences (Ola et al., 2013). In this work, among 74 induced features, 31 features were shown to be produced specifically by either T. versicolor or G. applanatum, or by both fungi using a 13C-based labeling analysis. This methodology was able to distinguish the origin even if the identities of compounds were not available or almost nothing was known about biochemical aspects. In more detail, the total 13C incorporation in the same fungal culture varied noticeably among features. Isotopic steady state is the state that 13C-labeling signatures in metabolites become time invariant (Antoniewicz et al., 2007). In current work, 13C incorporation into 20 features (e.g., orsellinic acid) had the similar abundance levels between days 10 and 20 and these features had the similar 13C-labeling pattern (data not shown), indicating that they likely reached isotopic steady state around 10 days (Figure 4B, Supplementary Figure 1). In contrast, 13C incorporation level of 11 features including novel phenyl polyketide (compound 1) increased from day 10 to day 20 (Figures 4A,C,D, Supplementary Figure 1), suggesting either the fluxes via their synthetic pathways were low or their metabolite pools were relatively large (Zamboni et al., 2009).
By comparison of 13C-labeling patterns between with and without the addition of the supernatant of co-culture, it was demonstrated that the induction of gene expression and synthesis of corresponding metabolites during the co-culture of T. versicolor and G. applanatum depended indeed on the signaling molecules released into the medium. Forty-three induced features in the supernatant of co-culture on day 10 were detected with signal intensity ranging from 103 to 105 (Table 1), but in the current work we were not able to determine which features were responsible for signaling to activate gene expression in T. versicolor or G. applanatum. Additional studies will be needed to confirm this link as well as to elucidate the structure and function of signaling molecules. Notably, the previous report also demonstrated that an intimate physical interaction of the actinomycete S. hygroscopicus and fungal mycelia of Aspergillus nidulans was required to induce specific stimulation of the silent polyketide synthases and non-ribosomal peptide synthetases gene clusters (Schroeckh et al., 2009). In our research, we found 12 highly produced features that did not incorporate 13C labeling in the mono-culture. One potential explanation is that these features were also produced in the co-culture via mycelium physical interaction to elicit the specific response. In addition, compound 1 had over 15-fold higher MS signal intensity in the co-culture than in the control mono-culture of T. versicolor where the mycelia were not pre-induced by the co-culture. It was not detected in the control mono-culture of G. applanatum, yet 13C incorporation in G. applanatum was 1.3-fold higher than that in T. versicolor on day 20 (Figure 4D). This finding suggests that the co-culture could significantly activate the encoding genes or gene clusters related to the synthesis of Compound 1 in G. applanatum. This provides an important insight into possible manipulation of G. applanatum to enhance biosynthesis of novel metabolites.
Comparing MS fragmentation similarity including common losses, molecular network analysis is able to obtain a simultaneous visual investigation of identical molecules, analogs, or metabolite families, thereby assisting the structural analysis (Winnikoff et al., 2014; Cabral et al., 2016). In our previous research, this method was utilized to show that the common neutral loss of 132 Dalton resulted from the deglycosylation reaction, which helped to find a series of novel xylosides generated during the co-culture (Yao et al., 2016). Here, combined molecular network analysis and 13C-labeling analysis suggested further that some newly discovered features were not only structurally analogous but also had similar induction mechanism and were likely produced by the same fungus. Thus, combination of network analysis and 13C labeling shows promise to accelerate the elucidation of biosynthetic pathways of novel metabolites.
Several type II polyketides have been reported to be high-value medicals (Zhang W. et al., 2012). In antioxidant assay, compound 1 had better activity than BHT. More interestingly, compound 1 at micromolar concentration was able to inhibit the viability of leukemic cells. For comparison, Zhang et al. reported that matrine extracted from Sophora flavescens inhibited the proliferation of acute myeloid leukemia cell lines U937 in a dose- and time-dependent manner with the IC50 of 590 mg/L at 24 h, and resulted in the maximal apoptosis rates with 37.2% for 24 h (Zhang S. et al., 2012). Wang et al. also demonstrated that 100 μg/mL of Ganoderma lucidum extracts could greatly suppress leukemic cell growth with the inhibition rate of 75% (Wang et al., 1997). In our case, the IC50 of compound 1 was at the same level with matrine and crude extracts from G. lucidum. Thus, it can become a starting point for development of lead compounds to cure leukemia or other cancers.
Conclusion
The application of 13C-labeling analysis produced valuable insight into the role of individual partners in the co-culture in production of known or unknown induced metabolites. Moreover, 13C-labeling approach combined with molecular network analysis can reveal whether certain structural analogs were produced by the same fungus or through the similar activation mechanism. The current 13C-labeling information sets an important foundation for further studies in the basidiomycetes, including but not limited to novel metabolites discovery and biosynthetic capacity improvement.
Author Contributions
X-YX, BQ, and SY: conceived and designed the project; X-YX, X-TS, X-JY, Y-MZ, HF, and JY: performed the experiments; X-YX, X-TS, L-PZ, F-YD, MS, and SY: interpreted the data. All authors contributed to the preparation of the manuscript, read and approved the final manuscript.
Funding
This work was supported by a grant from the Petrochemical Joint Fund of National Natural Science Foundation of China (No. U1462109), a grant from Shandong Province Natural Science Foundation (No. ZR2013CM024), a grant from National Natural Science Foundation of China (No. 31600028), a grant from Qingdao Applied Basic Research Program (No. 16-5-1-76-jch), a grant from Key Laboratory for Industrial Biocatalysis (Tsinghua University) Ministry of Education (No. 2015102) and a grant from Shandong provincial key research and development plan (Grant No. 2016GSF121010).
Conflict of Interest Statement
The authors declare that the research was conducted in the absence of any commercial or financial relationships that could be construed as a potential conflict of interest.
The reviewer MT and handling Editor declared their shared affiliation.
Acknowledgments
We thank Dr. Yan Li at the University of Texas Health Science Center for his assistance with NMR analysis.
Supplementary Material
The Supplementary Material for this article can be found online at: https://www.frontiersin.org/articles/10.3389/fmicb.2017.02647/full#supplementary-material
References
Abu-Bakar, M. F., Ismail, N. A., Isha, A., and Mei-Ling, A. L. (2016). Phytochemical composition and biological activities of selected wild berries (Rubus moluccanus L., R. fraxinifolius Poir., and R. alpestris Blume). Evid. Based Complement. Alternat. Med. 2016:2482930. doi: 10.1155/2016/2482930
Antoniewicz, M. R., Kelleher, J. K., and Stephanopoulos, G. (2007). Elementary Metabolite Units (EMU): a novel framework for modeling isotopic distributions. Metab. Eng. 9, 68–86. doi: 10.1016/j.ymben.2006.09.001
Bertrand, S., Bohni, N., Schnee, S., Schumpp, O., Gindro, K., and Wolfender, J. L. (2014). Metabolite induction via microorganism co-culture: a potential way to enhance chemical diversity for drug discovery. Biotechnol. Adv. 32, 1180–1204. doi: 10.1016/j.biotechadv.2014.03.001
Bertrand, S., Schumpp, O., Bohni, N., Monod, M., Gindro, K., and Wolfender, J. L. (2013). De novo production of metabolites by fungal co-culture of Trichophyton rubrum and Bionectria ochroleuca. J. Nat. Prod. 76, 1157–1165. doi: 10.1021/np400258f
Cabral, R. S., Allard, P. M., Marcourt, L., Young, M. C., Queiroz, E. F., and Wolfender, J. L. (2016). Targeted isolation of indolopyridoquinazoline alkaloids from Conchocarpus fontanesianus based on molecular networks. J. Nat. Prod. 79, 2270–2278. doi: 10.1021/acs.jnatprod.6b00379
Chen, C., Gonzalez, F. J., and Idle, J. R. (2007). LC-MS-based metabolomics in drug metabolism. Drug Metab. Rev. 39, 581–597. doi: 10.1080/03602530701497804
Chiang, Y. M., Chang, S. L., Oakley, B. R., and Wang, C. C. (2011). Recent advances in awakening silent biosynthetic gene clusters and linking orphan clusters to natural products in microorganisms. Curr. Opin. Chem. Boil. 15, 137–143. doi: 10.1016/j.cbpa.2010.10.011
Cui, J., Good, N. M., Hu, B., Yang, J., Wang, Q., Sadilek, M., et al. (2016). Metabolomics revealed an association of metabolite changes and defective growth in Methylobacterium extorquens AM1 overexpressing ecm during growth on methanol. PLoS ONE 11:e0154043. doi: 10.1371/journal.pone.0154043
Hammerl, R., Frank, O., and Hofmann, T. (2017). Differential off-line LC-NMR (DOLC-NMR) metabolomics to monitor tyrosine-induced metabolome alterations in Saccharomyces cerevisiae. J. Agric. Food Chem. 65, 3230–3241. doi: 10.1021/acs.jafc.7b00975
Hiscox, J., Baldrian, P., Rogers, H. J., and Boddy, L. (2010). Changes in oxidative enzyme activity during interspecific mycelial interactions involving the white-rot fungus Trametes versicolor. Fungal Genet. Biol. 47, 562–571. doi: 10.1016/j.fgb.2010.03.007
Hiscox, J., Savoury, M., Müller, C. T., Lindahl, B. D., Rogers, H. J., and Boddy, L. (2015). Priority effects during fungal community establishment in beech wood. ISME J. 9, 2246–2260. doi: 10.1038/ismej.2015.38
Hiscox, J., Savoury, M., Toledo, S., Kingscott-Edmunds, J., Bettridge, A., Waili, N. A., et al. (2017). Threesomes destabilise certain relationships: multispecies interactions between wood decay fungi in natural resources. FEMS Microbiol. Ecol. 93:fix 014. doi: 10.1093/femsec/fix014
Holman, J. D., Tabb, D. L., and Mallick, P. (2014). Employing ProteoWizard to convert raw mass spectrometry data. Curr. Protoc. Bioinformatics 46, 1–9. doi: 10.1002/0471250953.bi1324s46
Hu, B., Yang, Y. M., Beck, D. A., Wang, Q. W., Chen, W. J., Yang, J., et al. (2016). Comprehensive molecular characterization of Methylobacterium extorquens AM1 adapted for 1-butanol tolerance. Biotechnol. Biofuels 9:84. doi: 10.1186/s13068-016-0497-y
Jennings, M. E., and Matthews, D. E. (2005). Determination of complex isotopomer patterns in isotopically labeled compounds by mass spectrometry. Anal. Chem. 77, 6435–6444. doi: 10.1021/ac0509354
Julka, S., and Regnier, F. (2004). Quantification in proteomics through stable isotope coding: a review. J. Proteome Res. 3, 350–363. doi: 10.1021/pr0340734
Ma, K., and Ruan, Z. (2015). Production of a lignocellulolytic enzyme system for simultaneous bio-delignification and saccharification of corn stover employing co-culture of fungi. Bioresour. Technol. 175, 586–593. doi: 10.1016/j.biortech.2014.10.161
Ola, A. R., Thomy, D., Lai, D., Brötz-Oesterhelt, H., and Proksch, P. (2013). Inducing secondary metabolite production by the endophytic fungus Fusarium tricinctum through coculture with Bacillus subtilis. J. Nat. Prod. 76, 2094–2099. doi: 10.1021/np400589h
Onaka, H., Mori, Y., Igarashi, Y., and Furumai, T. (2011). Mycolic acid-containing bacteria induce natural-product biosynthesis in Streptomyces species. Appl. Environ. Microbiol. 77, 400–406. doi: 10.1128/AEM.01337-10
Peiris, D., Dunn, W. B., Brown, M., Kell, D. B., Roy, I., and Hedger, J. N. (2008). Metabolite profiles of interacting mycelial fronts differ for pairings of the wood decay basidiomycete fungus, Stereum hirsutum with its competitors Coprinus micaceus and Coprinus disseminatus. Metabolomics 4, 52–62. doi: 10.1007/s11306-007-0100-4
Pluskal, T., Castillo, S., Villar-Briones, A., and Oresic, M. (2010). MZmine 2: modular framework for processing, visualizing, and analyzing mass spectrometry-based molecular profile data. BMC Bioinformatics 11:395. doi: 10.1186/1471-2105-11-395
Riedlinger, J., Schrey, S. D., Tarkka, M. T., Hampp, R., Kapur, M., and Fiedler, H. P. (2006). Auxofuran, a novel metabolite that stimulates the growth of fly agaric, is produced by the mycorrhiza helper bacterium Streptomyces strain AcH 505. Appl. Environ. Microbiol. 72, 3550–3557. doi: 10.1128/AEM.72.5.3550-3557.2006
Rigano, M. M., Raiola, A., Tenore, G. C., Monti, D. M., Del-Giudice, G. R., Frusciante, L., et al. (2014). Quantitative trait loci pyramiding can improve the nutritional potential of tomato (Solanum lycopersicum) fruits. J. Agri. Food Chem. 62, 11519–11527. doi: 10.1021/jf502573n
Scherlach, K., and Hertweck, C. (2009). Triggering cryptic natural product biosynthesis in microorganisms. Organ. Biomol. Chem. 7, 1753–1760. doi: 10.1039/b821578b
Schroeckh, V., Scherlach, K., Nützmann, H. W., Shelest, E., Schmidt-Heck, W., Schuemann, J., et al. (2009). Intimate bacterial–fungal interaction triggers biosynthesis of archetypal polyketides in Aspergillus nidulans. Proc. Natl. Acad. Sci. U.S.A. 106, 14558–14563. doi: 10.1073/pnas.0901870106
Shlomi, T., Fan, J., Tang, B., Kruger, W. D., and Rabinowitz, J. D. (2014). Quantitation of cellular metabolic fluxes of methionine. Anal. Chem. 86, 1583–1591. doi: 10.1021/ac4032093
Soman, G., Yang, X., Jiang, H., Giardina, S., Vyas, V., Mitra, G., et al. (2009). MTS dye based colorimetric CTLL-2 cell proliferation assay for product release and stability monitoring of interleukin-15: assay qualification, standardization and statistical analysis. J. Immunol. Methods 348, 83–94. doi: 10.1016/j.jim.2009.07.010
Song, A. R., Sun, X. L., Kong, C., Zhao, C., Qin, D., Huang, F., et al. (2014). Discovery of a new sesquiterpenoid from Phellinus ignarius with antiviral activity against influenza virus. Arch. Virol. 159, 753–760. doi: 10.1007/s00705-013-1857-6
Su, G., Morris, J. H., Demchak, B., and Bader, G. D. (2014). Biological network exploration with cytoscape 3. Curr. Protoc. Bioinformatics 47, 1–24. doi: 10.1002/0471250953.bi0813s47
Traxler, M. F., Watrous, J. D., Alexandrov, T., Dorrestein, P. C., and Kolter, R. (2013). Interspecies interactions stimulate diversification of the Streptomyces coelicolor secreted metabolome. MBio 4:e00459–e00413. doi: 10.1128/mBio.00459-13
Wang, M., Carver, J. J., and Phelan, V. V. (2016). Sharing and community curation of mass spectrometry data with global natural products social molecular networking. Nat. Biotechnol. 34, 828–837. doi: 10.1038/nbt.3597
Wang, S. Y., Hsu, M. L., Hsu, H. C., Tzeng, C. H., Lee, S. S., Shiao, M. S., et al. (1997). The antitumor effect of Ganoderma lucidum is mediated by cytokines released from activated macrophages and T lymphocytes. Int. J. Cancer 70, 699–705. doi: 10.1002/(SICI)1097-0215(19970317)70:6<699::AID-IJC12>3.0.CO;2-5
Watrous, J., Roach, P., Alexandrov, T., Heath, B. S., Yang, J. Y., Kersten, R. D., et al. (2012). Mass spectral molecular networking of living microbial colonies. Proc. Natl. Acad. Sci. U.S.A. 109, 1743–1752. doi: 10.1073/pnas.1203689109
Winnikoff, J. R., Glukhov, E., Watrous, J., Dorrestein, P. C., and Gerwick, W. H. (2014). Quantitative molecular networking to profile marine cyanobacterial metabolomes. J. Antibiot. 67, 105–112. doi: 10.1038/ja.2013.120
Xing, F., Wang, L., Liu, X., Selvaraj, J. N., Wang, Y., Zhao, Y., et al. (2017). Aflatoxin B1 inhibition in Aspergillus flavus by Aspergillus niger through down-regulating expression of major biosynthetic genes and AFB1 degradation by atoxigenic A. flavus. Int. Food Microbiol. 256, 1–10. doi: 10.1016/j.ijfoodmicro.2017.05.013
Yang, S., Matsen, J. B., Konopka, M., Green-Saxena, A., Clubb, J., Sadilek, M., et al. (2013). Global molecular analyses of methane metabolism in methanotrophic Alphaproteobacterium, Methylosinus trichosporium OB3b. Part II. metabolomics and 13C-labeling study. Front. Microbial. 4:70. doi: 10.3389/fmicb.2013.00070
Yang, S., Nadeau, J. S., Humston-Fulmer, E. M., Hoggard, J. C., Lidstrom, M. E., and Synovec, R. E. (2012). Gas chromatography-mass spectrometry with chemometric analysis for determining 12C and 13C labeled contributions in metabolomics and 13C flux analysis. J. Chromatogr. A. 1240, 156–164. doi: 10.1016/j.chroma.2012.03.072
Yang, S., Sadilek, M., and Lidstrom, M. E. (2010). Streamlined pentafluorophenylpropyl column liquid chromatography-tandem quadrupole mass spectrometry and global 13C-labeled internal standards improve performance for quantitative metabolomics in bacteria. J. Chromatogr. A 1217, 7401–7410. doi: 10.1016/j.chroma.2010.09.055
Yao, L., Zhu, L. P., Xu, X. Y., Tan, L. L., Sadilek, M., Fan, H., et al. (2016). Discovery of novel xylosides in co-culture of basidiomycetes Trametes versicolor and Ganoderma applanatum by integrated metabolomics and bioinformatics. Sci. Rep. 6:33237. doi: 10.1038/srep33237
Zamboni, N., Fendt, S. M., Rühl, M., and Sauer, U. (2009). 13C-based metabolic flux analysis. Nat. Protoc. 4, 878–892. doi: 10.1038/nprot.2009.58
Zhang, S., Zhang, Y., Zhuang, Y., Wang, J., Ye, J., Zhang, S., et al. (2012). Matrine induces apoptosis in human acute myeloid leukemia cells via the mitochondrial pathway and Akt inactivation. PLoS ONE 7:e46853. doi: 10.1371/journal.pone.0046853
Zhang, W., Wang, L., Kong, L., Wang, T., Chu, Y., Deng, Z., et al. (2012). Unveiling the post-PKS redox tailoring steps in biosynthesis of the type II polyketide antitumor antibiotic xantholipin. Chem. Biol. 19, 422–432. doi: 10.1016/j.chembiol.2012.01.016
Keywords: basidiomycete, interaction, 13C-labeling, origin of induced features, phenyl polyketide, metabolite identification, LC-MS
Citation: Xu X-Y, Shen X-T, Yuan X-J, Zhou Y-M, Fan H, Zhu L-P, Du F-Y, Sadilek M, Yang J, Qiao B and Yang S (2018) Metabolomics Investigation of an Association of Induced Features and Corresponding Fungus during the Co-culture of Trametes versicolor and Ganoderma applanatum. Front. Microbiol. 8:2647. doi: 10.3389/fmicb.2017.02647
Received: 03 September 2017; Accepted: 19 December 2017;
Published: 09 January 2018.
Edited by:
Erika Kothe, Friedrich Schiller Universität Jena, GermanyReviewed by:
Nico Jehmlich, Helmholtz-Zentrum für Umweltforschung (UFZ), GermanyMartin Taubert, Friedrich Schiller Universität Jena, Germany
Copyright © 2018 Xu, Shen, Yuan, Zhou, Fan, Zhu, Du, Sadilek, Yang, Qiao and Yang. This is an open-access article distributed under the terms of the Creative Commons Attribution License (CC BY). The use, distribution or reproduction in other forums is permitted, provided the original author(s) or licensor are credited and that the original publication in this journal is cited, in accordance with accepted academic practice. No use, distribution or reproduction is permitted which does not comply with these terms.
*Correspondence: Song Yang, eWFuZ3NvbmcxMjA5QDE2My5jb20=
†These authors have contributed equally to this work.