- 1Department of Ecology, College of Natural Resources and Environment, South China Agricultural University, Guangzhou, China
- 2Key Laboratory of Agro-Environment in the Tropics, Ministry of Agriculture, Guangzhou, China
- 3Guangdong Engineering Research Center for Modern Eco-Agriculture and Circular Agriculture, Guangzhou, China
- 4School of Geographical Sciences, Guangzhou University, Guangzhou, China
- 5Guangdong Provincial Key Laboratory of Applied Botany, South China Botanical Garden, Chinese Academy of Sciences, Guangzhou, China
- 6College of Resources and Environment, University of Chinese Academy of Sciences, Beijing, China
Atmospheric nitrogen (N) deposition is changing in both load quantity and chemical composition. The load effects have been studied extensively, whereas the composition effects remain poorly understood. We conducted a microcosm experiment to study how N chemistry affected the soil microbial community composition characterized by phospholipid fatty acids (PLFAs) and activity indicated by microbial CO2 release. Surface and subsurface soils collected from an old-growth subtropical forest were supplemented with three N-containing materials (ammonium, nitrate, and urea) at the current regional deposition load (50 kg ha-1 yr-1) and incubated at three temperatures (10, 20, and 30∘C) to detect the interactive effects of N deposition and temperature. The results showed that the additions of N, regardless of form, did not alter the microbial PLFAs at any of the three temperatures. However, the addition of urea significantly stimulated soil CO2 release in the early incubation stage. Compared with the control, N addition consistently reduced the temperature dependency of microbial respiration, implying that N deposition could potentially weaken the positive feedback of the warming-stimulated soil CO2 release to the atmosphere. The consistent N effects for the surface and subsurface soils suggest that the effects of N on soil microbial communities may be independent of soil chemical contents and stoichiometry.
Introduction
Atmospheric nitrogen (N) deposition has been recognized as an important aspect of global changes for decades, with various ecological and economic consequences observed around the world (Galloway et al., 2008; BassiriRad, 2015). N deposits are composed of oxidized/reduced and organic/inorganic N-containing materials and could change a series of ecosystem functions and processes, e.g., acidifying soils (Bobbink et al., 2010; Lu et al., 2015), reducing biodiversity (Bobbink et al., 2010; Lu et al., 2010; Clark et al., 2013), depressing soil respiration and CH4 oxidation but increasing N2O emissions (Mo et al., 2008; Zhang et al., 2008; Janssens et al., 2010), affecting litter decomposition (Mo et al., 2006; Hobbie, 2008; Chen et al., 2015), and increasing soil carbon (C) sequestration (Reay et al., 2008; Liu and Greaver, 2010; Chen et al., 2015). More importantly, N deposition could interact with other global change components, such as elevated CO2 concentration and warming (Hungate et al., 2009; Turner and Henry, 2009), and therefore make it difficult to clearly determine the ecological consequences by using single-factor experiments. With cascading effects on almost all ecosystem components, the effects of N deposition and the underlying mechanisms have been extensively studied in the past few decades (Galloway et al., 2008; BassiriRad, 2015).
In most of the previous studies, researchers have paid the most attention to the effects of N loading (Du et al., 2014; BassiriRad, 2015). However, changes in the chemical compositions of N deposits have been recorded in many places (Rogora et al., 2006; Liu et al., 2013; Hunova et al., 2017) possibly resulting from a combination of many factors, such as economic development policies, motor vehicles, the measures of reducing anthropogenic N emissions and the availability of reliable and cost-effective technologies (Erisman et al., 2001; Liu et al., 2013). The chemical composition of N deposits could vary over season or month in a calendar year (Fang et al., 2008). It is well known that different N-containing materials are involved in different biogeochemical processes (Corre and Lamersdorf, 2004; Du et al., 2014). However, whether change in the composition of N deposits result in diverse ecological consequences remains poorly understood.
Soil is the final receiver of atmospheric deposits, and underground ecological processes are vulnerable to atmospheric N deposition (Janssens et al., 2010; Cusack et al., 2011; Chen et al., 2015). Soil microbial communities play critical roles in these processes since they are the main drivers that are involved in almost all belowground processes (Balser et al., 2006). Previous studies have reported that soil microorganisms could respond rapidly to atmospheric N deposition (Allison et al., 2008; Cusack et al., 2011; Ramirez et al., 2012), and the responses could be ecosystem and N load specific (Treseder, 2008; Cusack et al., 2011; Zhou et al., 2012). The differences in N compositions among studies could partly contribute to these case-specific results because single N-containing chemicals are often used to simulate N deposition. The neglect of the effects of N composition may result in a biased understanding of the ecological consequences of N deposition.
The results of both meta-analyses and case studies suggest that N deposition suppresses soil microbial respiration (Janssens et al., 2010; Ramirez et al., 2010a), especially at highly productive sites with relatively less N limitations (Janssens et al., 2010). Such an effect may be attributed to two mechanisms: one mechanism involves enhanced chemical stabilization of soil organic C (SOC) by forming recalcitrant SOC that is highly resistant to microbial decomposition, and the other mechanism involves shifts in the substrate preference of soil microbes toward labile and energy-rich substrate (Janssens et al., 2010; Ramirez et al., 2012). Indirect effects of N additions (e.g., on soil pH) could not explain the consistent pattern of soil microbial respiration in response to N additions (Ramirez et al., 2010a). Besides, N form also affects the responsive magnitude of microbial respiration (Ramirez et al., 2010a). A recent study in a temperate forest showed that soil C cycling was inhibited by inorganic N, whereas it was promoted by organic N when both were applied at the same load (Du et al., 2014). These observations imply that the effects of N deposition could depend on deposited N chemistry. This dependence may result from different substrate preferences of various soil microbial groups (Treseder, 2008; Baran et al., 2015). For instance, ammonium can decrease fungal abundance, whereas nitrate mainly influences bacterial growth rates (Nunan et al., 2006; Birgander et al., 2014). Therefore, the altered composition of N deposits (e.g., the ratio of ammonium to nitrate) could alter soil microbial communities, although empirical evidence of this phenomenon remains scarce.
The microbial community composition and function in soil can be identified and evaluated by a variety of techniques (Torsvik and Øvreås, 2002; Leckie, 2005; Frostegård et al., 2011). Phospholipid fatty acid (PLFA) analysis is a widely used method for identifying community-level changes in microbial biomass and composition (Frostegård et al., 2011). Soil microbial respiration, i.e., CO2 emitted by soil microbial activities, is a synthesis index that reflects the function of the microbial community at decomposing soil organic matter (SOM) (Hanson et al., 2000; Janssens et al., 2010). In nature, soil microbial communities consist of thousands of microbial species that have different substrate preferences (Fierer et al., 2007; Baran et al., 2015). Diverse microbial groups respond differently to temperature increases, and these differences are accompanied by differing microbial community functions and temperature sensitivities (Wei et al., 2014). The thermal effects are sometimes due to limited substrate availability under warming conditions (Gershenson et al., 2009). As an important soil substrate, the N fertilization effects that are derived from atmospheric N deposition may alleviate such substrate limitations by promoting soil C and N availability, which could, therefore, confound the warming effects (Cusack et al., 2010; Chen et al., 2015).
By incubating surface and sub-surface soils collected from an N-rich subtropical forest (Fang et al., 2008), we conducted this microcosm experiment to understand the effects of N form and the interactions between N and temperature on soil microbial community composition and function. The two environmental factors, i.e., N and temperature, were studied, as atmospheric N deposition and warming have both been recorded for a long time (Erisman et al., 2001; IPCC, 2013; Liu et al., 2013). Both factors can significantly affect ecological processes and have received much attention across multiple ecosystems (Allison et al., 2010; Cusack et al., 2010; BassiriRad, 2015), and interactive effects likely exist between the two (Cusack et al., 2010; Chen et al., 2015; Liu et al., 2017). Microbial respiration could adapt to temperature and therefore have lower temperature dependence at high temperatures (Davidson and Janssens, 2006; Wei et al., 2014). Such a microbial acclimation may result from labile substrate depletion and microbial community adjustments in response to warming (Bradford et al., 2008; Allison et al., 2010; Wei et al., 2014). Atmospheric N deposition could alter soil substrate supply by alleviating soil C limitations and increasing inorganic N availability (Treseder, 2008; Chen et al., 2015), and alter soil microbial communities (Janssens et al., 2010; Ramirez et al., 2010b), consequently affecting microbial responses to temperature changes (Gershenson et al., 2009; Wei et al., 2014). However, inconsistent N and temperature interactions have been reported across N-rich forests (Mo et al., 2008; Cusack et al., 2010; Liu et al., 2017). Furthermore, the effects of N deposition on the temperature sensitivity of soil respiration may be greatly impacted by other factors such as stand age that can determine the direction of ecosystems in response to N addition (Mo et al., 2008; Deng et al., 2010). Great uncertainty still exists regarding the effects of N deposition across ecosystems, and explanation of the contradictions among studies is difficult, mainly due to the underlying mechanisms (including whether such effects depend on N composition) unclear.
In this study, we first aimed to examine the N-induced effects on soil microbial community PLFAs and SOM decomposition. We expected that the soil microbial community composition and function could respond rapidly to the experimental N addition and temperature manipulations. Furthermore, we expected that the microbial responses would depend on the applied N chemicals, because N species (e.g., ammonium vs. nitrate) can influence different microbial groups to different extents (Nunan et al., 2006; Birgander et al., 2014). Moreover, surface and subsurface soils with different physicochemical properties (see Table 1) were used to evaluate the treatment effects across soils. We expected that the surface soil would exhibit a greater response than the subsurface soil, as microbial responses to environmental changes depend greatly on soil properties and substrate availability (Karhu et al., 2010; Eberwein et al., 2015).
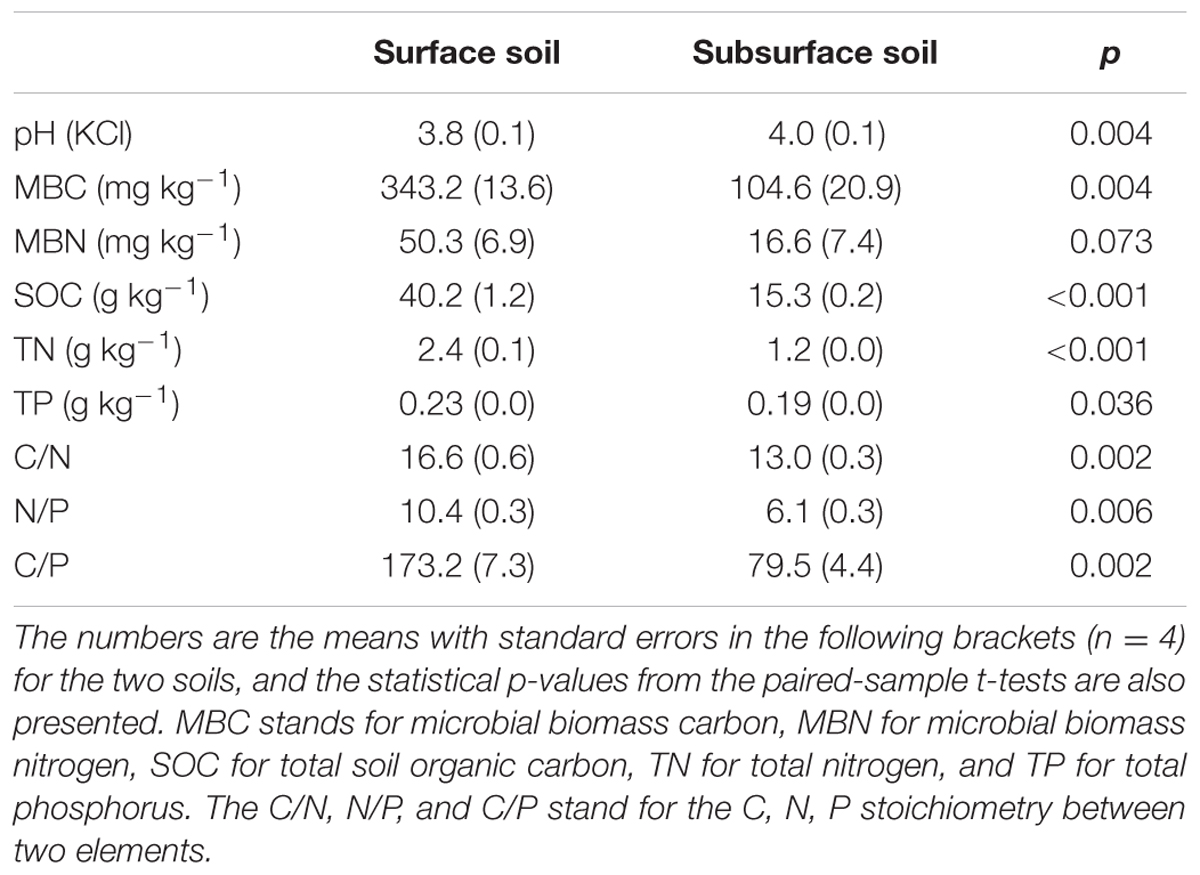
TABLE 1. Initial soil properties of the studied forest soils at the surface (0–10 cm) and subsurface (10–20 cm) soil layers.
Materials and Methods
Site Description
The soils were collected from two soil layers (0–10 and 10–20 cm) of an old-growth monsoon evergreen broadleaved forest, the climax vegetation in the Guangdong Province of southern China (112°30′ – 112°33′E, 23°09′ – 23°11′N). This region experiences a subtropical monsoon climate with the average annual air temperature of 22.3°C and the average precipitation of 1678 mm. The rainy season coincides with high temperature, with 80% of the annual precipitation occurring during the warm season from April to September. The studied forest is a mature forest with a stand age of approximately 400 years. It has naturally developed for hundreds of years under protection by the Buddhist monks in a neighboring temple (Tang et al., 2006). The dominant tree species in this forest include Castanopsis chinensis, Schima superba, Cryptocarya chinensis, Machilus chinensis, and Syzygium rehderianum. The soil in the studied forest is an acidic red soil that is similar to oxisols in USDA soil taxonomy (Tang et al., 2006).
Experimental Design
Three factors, i.e., N form, incubation temperature and soil layer, were included in the present study. These factors included four N treatments [a control with no N addition and the addition of N in three forms (ammonium sulfate, potassium nitrate and urea)], three incubation temperatures (10, 20, and 30°C) and two soil depths (surface soil from 0 to 10 cm and subsurface soil from 10 to 20 cm). A full factorial design was employed in our study; thus, a total of 24 experimental treatments were conducted. For each treatment, four experimental replicates were used from four composite soil samples collected from the old-growth forest.
The three N chemicals were chosen because both inorganic (especially ammonium and nitrate) and organic N chemicals contribute to atmospheric N deposition in various ecosystems (Fang et al., 2008; Chen et al., 2015); however, few N deposition-related studies to date have considered organic N deposition (Cusack et al., 2011; Liu et al., 2013). For example, in the studied forest, organic N was found to contribute approximately 40% of the total N deposition load (18/50 kg N ha-1 yr-1) between 2004 and 2005 (Fang et al., 2008). The amount of N addition in the present study (3.79 mg N 50 g-1 dry soil) was approximately 50 kg N ha-1 for the 10 cm-depth soils, which is a value close to the N deposition load previously measured in this region (Fang et al., 2008).
The three incubation temperatures fall within the temperature range that occurs at the study site within a calendar year (Wei et al., 2015). The soil microbial respiration increased exponentially as the temperature increased within the range in the studied forest (Wei et al., 2015). These previous observations indicated that the soil microbial communities had not been exceedingly stressed by temperature in the present study, and the temperature sensitivity index Q10 could be calculated using the widely used exponential function as described in section “Data Analyses.” The two layers of soil were used to determine the treatment effects across soils, as the initial properties of the two soils were significantly different (see Table 1).
Soil Collection and Incubation
Four quadrats with sizes of 15 m × 15 m and a buffering distance of at least 10 m between quadrats were established for collecting the soil samples. Within each quadrat, four 20 cm × 20 cm sampling spots were randomly located, and the visible plant materials were removed prior to soil sampling. The surface (0–10 cm) and subsurface (10–20 cm) soil samples that were collected from the four spots were composited into two samples – one surface soil sample and one subsurface soil sample. In total, four composite surface soil samples and four subsurface soil samples were taken as experimental replicates from the four quadrats. All samples were transported to the laboratory and passed through a 2-mm sieve to remove the visible stones and plant residues before the following analyses and incubation. Fresh soil samples were used to analyse the initial soil properties, and the results are shown in Table 1. As expected, the surface soil had lower soil pH but higher microbial biomass C (MBC) and N (MBN) and substrate content, including SOC, total N (TN), and total phosphorus (TP), compared to those of the subsurface soil. As a result, the C/N, N/P, and C/P ratios were significantly greater in the surface than the subsurface soil (p < 0.05, Table 1).
In this study, we had a total of 96 fresh soil samples (4 N forms × 3 temperatures × 2 soil layers × 4 replicates) for the incubation. Each sample, equivalent to 50 g of oven-dried soil weight, was placed into a 200 ml triangular glass flask. The soil water content of the incubation samples was adjusted to 50% of the water-holding capacity (WHC), after which the samples were incubated at room temperature (approximately 25°C) for 1 week to stabilize and restore the soil microbial communities. After stabilization, N solutions and deionized water were added to the soil samples (see Experimental Design for the N load determinations) to reach a final water content of 55% WHC, which falls within the optimal range of soil water content for the soil microbial activity in the studied forest soil (Zhou et al., 2014). The 96 soil samples were then incubated for 90 days in three thermostat incubators (RXZ-600B, Southeast Instrument Co., Ltd., Ningbo, China) set to the three temperatures, with each incubator containing 32 samples (4 N forms × 2 soil layers × 4 replicates).
During the incubation, a suitable amount of cotton was placed on the top of each incubation flask to prevent rapid water evaporation but permit gas exchange. Soil water content was checked by weighing the flasks twice per week, and deionized water was added to maintain the moisture level. On incubation days 1, 7, 12, 18, 26, 33, 42, 49, 56, 67, 80, and 90, two headspace gas samples were collected from each incubation flask using injection syringes. A three-way valve was installed on each syringe to maintain gas tightness before the determination of CO2 concentrations. The time interval between the two gas collections was 30 min per flask. To determine the headspace CO2 concentrations, following their collection, these gas samples were immediately analyzed in a gas chromatograph (Agilent 7890A, Agilent Technologies Inc., CA, United States) with a flame ionization detector (FID). The rate of CO2 emission was estimated as the slope of the CO2 concentration increase with time. At the end of the incubation, soil samples were collected for PLFA analysis.
Soil Chemical and PLFA Analyses
The pH of soil suspensions at a ratio of soil to KCl solution of 1:2.5 was tested. The soil MBC and MBN were analyzed using the chloroform and fumigation method (Vance et al., 1987), and the C and N contents in the extracts were determined using a C analyzer (TOC-VCSH, Shimadzu Corp., Kyoto, Japan); the extraction coefficients used were 0.45 for the MBC and 0.54 for the MBN calculations (Brookes et al., 1985; Wu et al., 1990). The SOC was analyzed using the Walkley–Black method, while TN and TP were analyzed using the acid-digestion and colorimetric methods, respectively (Liu, 1996).
The PLFA analysis was conducted following Bossio and Scow (1998). Briefly, 5 g of freeze-dried soils were extracted by a buffering solution with a volume ratio of 1:2:0.8 of chloroform, methanol and citrate for each sample. The phospholipids in the organic phase were then separated and collected using a silica column (500 mg, ANPEL Laboratory Technologies Inc., Shanghai, China). After the samples were methanolysed by adding a mildly alkaline solution, the fatty acids were identified on an Agilent 7890 GC equipped with a Sherlock Microbial Identification System (Version 6.2, MIDI Inc., Newark, DE, United States). The individual PLFA contents (nmol g-1 soil) were determined based on the added internal standard (19:0), and the relative abundance of microbial groups (mol%) was calculated. Gram-positive (G+) bacteria were indicated by the sum of iso- and anteiso-fatty acids, while Gram-negative (G-) bacteria were indicated by the sum of cyclopropane fatty acids, mono-unsaturated fatty acids and hydroxyl fatty acids. Carboxylic acids with methyl functioning on the tenth C were regarded as actinomycetal indicators, and fungi were indicated by the summed abundance of 18:2w6c and 18:1w9c. Finally, the ratios of fungal to bacterial PLFAs and G+ to G- bacterial PLFAs were calculated to indicate the soil microbial community structure.
Data Analyses
The accumulated soil CO2 emission (Cm) values were calculated using an integrating method by multiplying the instantaneous CO2 emission rate by the duration of the incubation (Martin Wetterstedt et al., 2009). The entire incubation period was separated into three stages with stage 1 being the first 7 days, stage 2 being days 8–26 and stage 3 being the remaining period, and the accumulated CO2 release within the three stages was calculated. In each stage, the temperature sensitivity, presented as the Q10 value that indicates the Cm increase for every 10°C temperature increase (Arevalo et al., 2012), was also calculated following the commonly used Q10 function (Zhou et al., 2014):
The parameter b was obtained by fitting Cm with the incubation temperature (T) using the first-order exponential function (Arevalo et al., 2012):
Although Q10 can vary with temperature (Lloyd and Taylor, 1994), the apparent temperature sensitivity remains useful for determining implications for soil CO2 emissions in response to temperature changes under existing environmental constraints (Davidson and Janssens, 2006).
Paired-sample t-tests were employed to detect significant differences in the initial properties between the surface and sub-surface soils. A three-way analysis of variances (ANOVA) was conducted on the base-e logarithms of each of the soil CO2 emissions in the three stages, and a repeated measures ANOVA was used to explore the significance of Q10 among N treatments over time. In each stage, one-way ANOVA with Tukey multiple comparisons was further conducted to compare the means among the treatments when normality and homoscedasticity hypotheses were met; otherwise, a Welch test with the Games-Howell method was used. Principal component analysis (PCA) was employed on the PLFA dataset to extract the principal components that were used for the further analyses. The scores of the first two PCs were correlated with total PLFAs, the relative abundances of the different microbial groups and the ratios of G+/G- and F/B to detect which microbial community properties contributed to the observed treatment effects on soil microbial community composition. The significance level was set at p < 0.05. All statistical analyses were finished in IBM SPSS statistics (version 22, IBM Corp., New York, NY, United States), except for Q10, which was estimated in R software (version 3.1.2).
Results
Effects on Soil CO2 Emission
The results of the three-way ANOVA showed that the different N forms did not exhibit significantly different effects on the soil CO2 emissions as expected, except in stage 1 (p < 0.001, Table 2). The incubation temperature and soil layer significantly affected the Cm in the three stages (p < 0.001 for all, Table 2). N form did not show any significant interactive effects in conjunction with incubation temperature (p > 0.05 in all the three stages, Table 2) or soil layer in the first two stages (p > 0.05, Table 2). However, incubation temperature exhibited significant interactive effects with soil layer in the three stages (p < 0.001, Table 2). The interactions among the three factors (i.e., N form, temperature and soil layer) were only significant in stage 3 (p = 0.004, Table 2). In particular, the accumulated CO2 release was significantly higher under urea addition than that under the control at the two lower temperatures (10 and 20°C) in stage 1 (p < 0.05, Figures 1A,B), which was associated with the highest CO2 release at the beginning due to the addition of urea (Supplementary Figures S1, S2) as well as at 10°C during stage 3. N addition with either of the other N forms did not significantly affect the CO2 release (p > 0.05, Figure 1 and Table 2), with the exception of nitrate addition at 10°C in stage 3. During the entire period, nitrate addition tended to cause relatively higher CO2 emissions than did ammonium addition, but the differences were not statistically significant (Figure 1 and Supplementary Figures S1, S2). The surface soil released significantly more CO2 than did the subsurface soil under all the combinations of incubation temperature and N form (p < 0.05, Figure 1 and Table 2), and the soil CO2 emissions significantly increased as the temperature increased (p < 0.001, Figure 1 and Table 2).
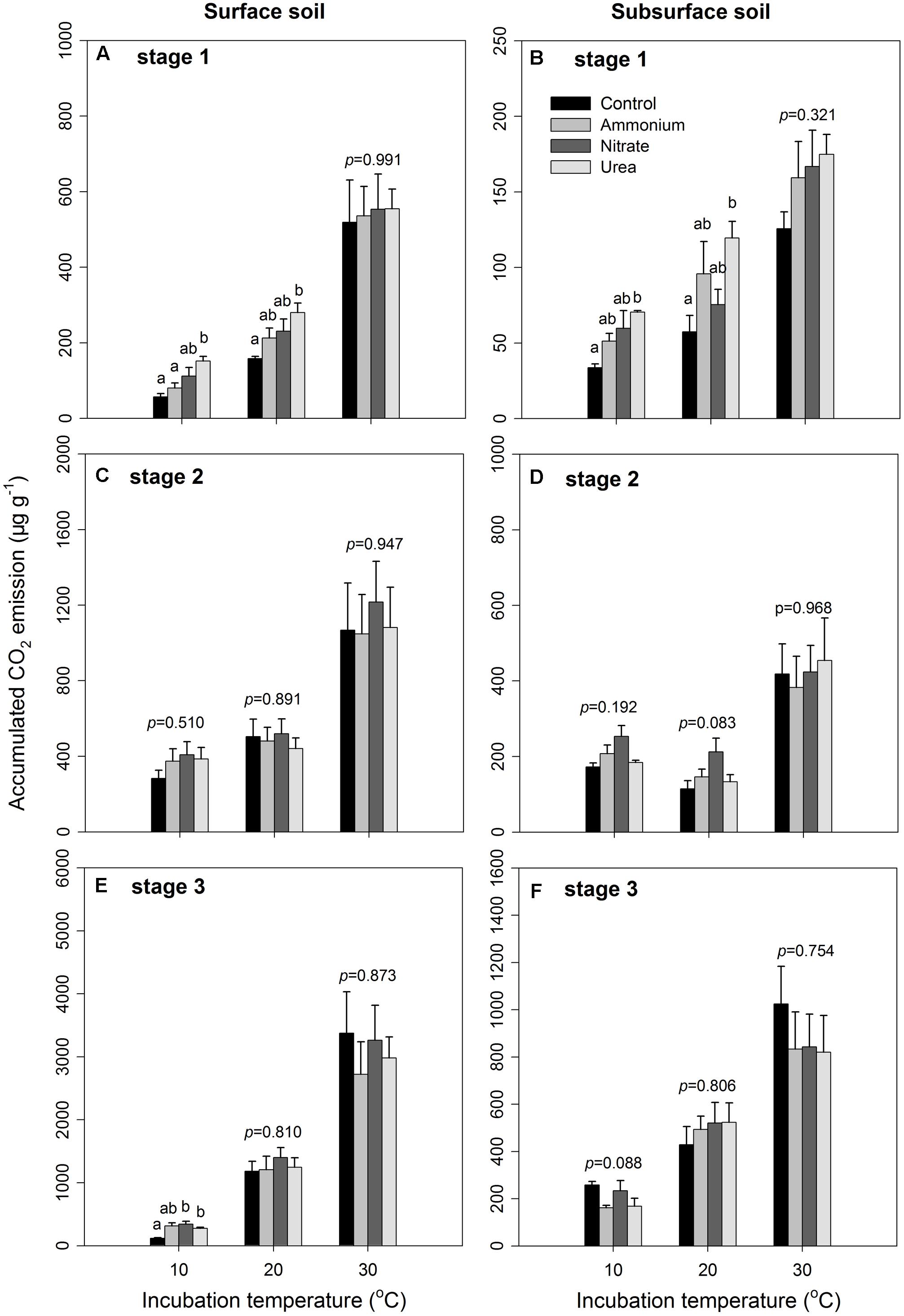
FIGURE 1. Accumulated CO2 emissions under different incubation temperatures and N additions for the surface and subsurface soils. The left three panels (A,C,E) represent the three incubation stages (0–7, 8–26, and 27–90 days) for the surface soil, respectively, while the right three panels (B,D,F) represent the three stages for the subsurface soil. The bars represent the means, and the error bars are the standard errors (n = 4). In each panel, the different lowercase letters above the bars indicate significant differences at p < 0.05 among the N treatments under each of the incubation temperatures. The statistical p-values were given when p > 0.05.
The results of repeated measures ANOVA showed that N form and incubation duration had significant effects on the temperature index Q10 for both of the surface and sub-surface soils, and their interactions were also significant (p < 0.001, Figure 2). Relative to the control, exogenous N addition decreased the temperature sensitivity of soil CO2 emissions (p < 0.05, Figure 2), except that decrease was not significant in stage 2 for the surface soil and for the subsurface soil with urea addition (p > 0.05, Figure 2). Among the three N forms, urea addition induced significantly lower Q10 than did the addition of the other two forms in stage 1 (p < 0.05, Figure 2), while there was no significant difference between ammonium and nitrate addition throughout the investigation period (p > 0.05, Figure 2). However, interactions between N form and temperature were not significant on the specific respiration (Cm values divided by total PLFA amount), indicating that Q10 was not significantly different among N treatments (p > 0.05, Supplementary Table S1). Except for the control and urea treatment in stage 2, the surface soil had significantly greater Q10 values than the subsurface soil (p < 0.05, Figure 2).
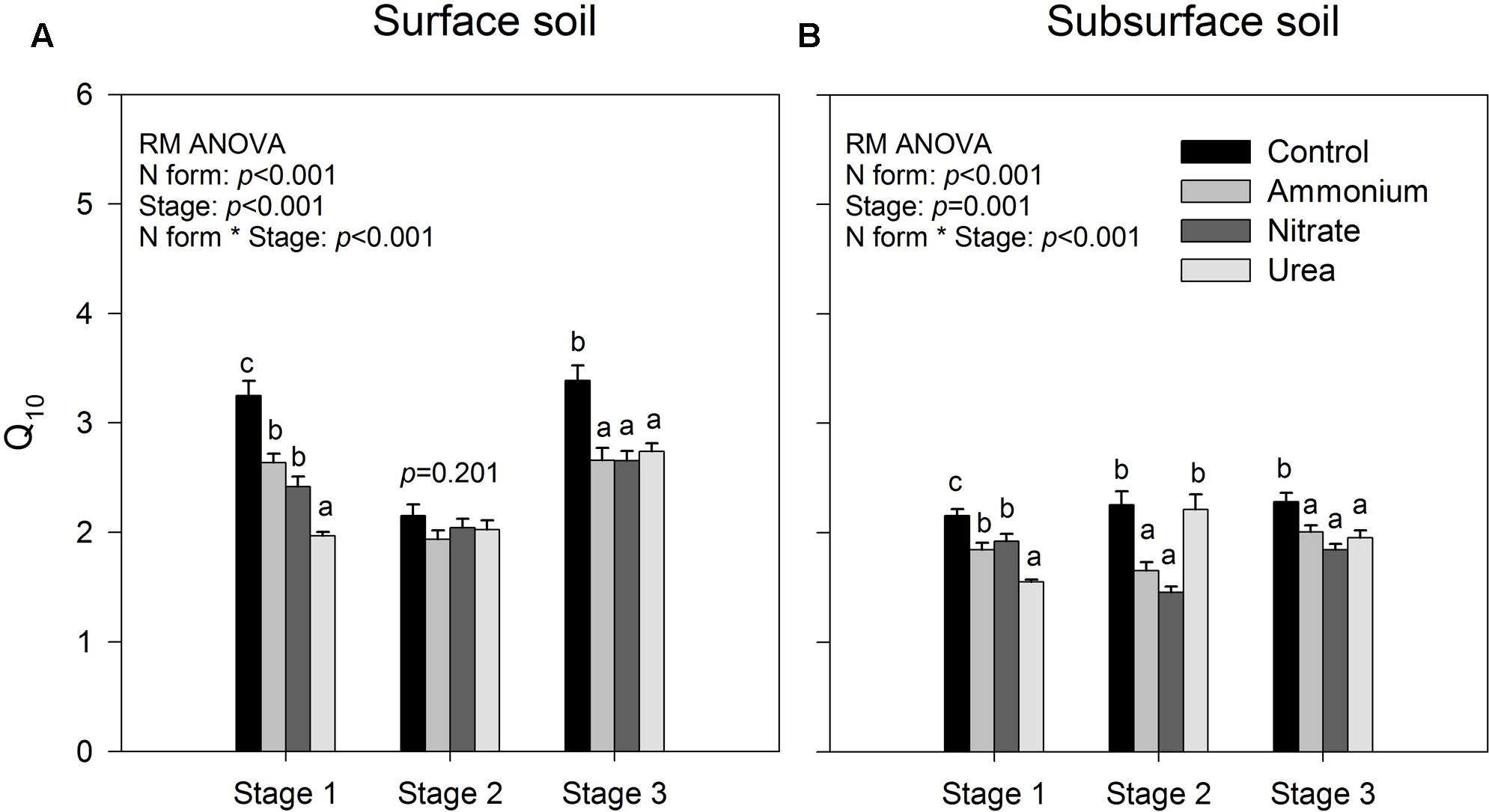
FIGURE 2. Temperature sensitivity (Q10) of accumulated CO2 release for the surface (A) and subsurface (B) soils. Stages 1–3 correspond to days 0–7, 8–26, and 27–90, respectively. The bars represent the means, and the error bars are the standard errors (n = 64). In each panel, the different lowercase letters above the bars indicate significant differences at p < 0.05 among the N treatments at each of the three stages. The statistical p-values were given when p > 0.05.
Effects on Soil Microbial Community Composition
After the 90-day incubation, the PLFA-derived soil microbial community composition consistently shifted downward along the second PC axis as the temperature increased, but the data within the plots did not clearly assemble in accordance with the applied N form to separate from the data in the control plots (Figure 3). Furthermore, ANOVA of the first PCs showed that N treatment had no significant effects on the soil microbial PLFA profiles (p > 0.05). The change was accompanied by alterations in the total PLFAs, relative abundance of microbial groups, and the ratios of fungal to bacterial PLFAs (F/B) and of G+ to G- bacterial PLFAs (G+/G-), as indicated by significant correlations between the first two PCs and these microbial properties (p < 0.01, Table 3 and Supplementary Table S2). In particular, PC1 had significant correlations with the total, actinomycetal, G- and fungal PLFAs, F/B, and G+/G- (p < 0.05), while PC2 was significantly correlated with the G+, G-, actinomycetal and fungal PLFAs, F/B, and G+/G- (p < 0.05, Table 3 and Supplementary Table S2).
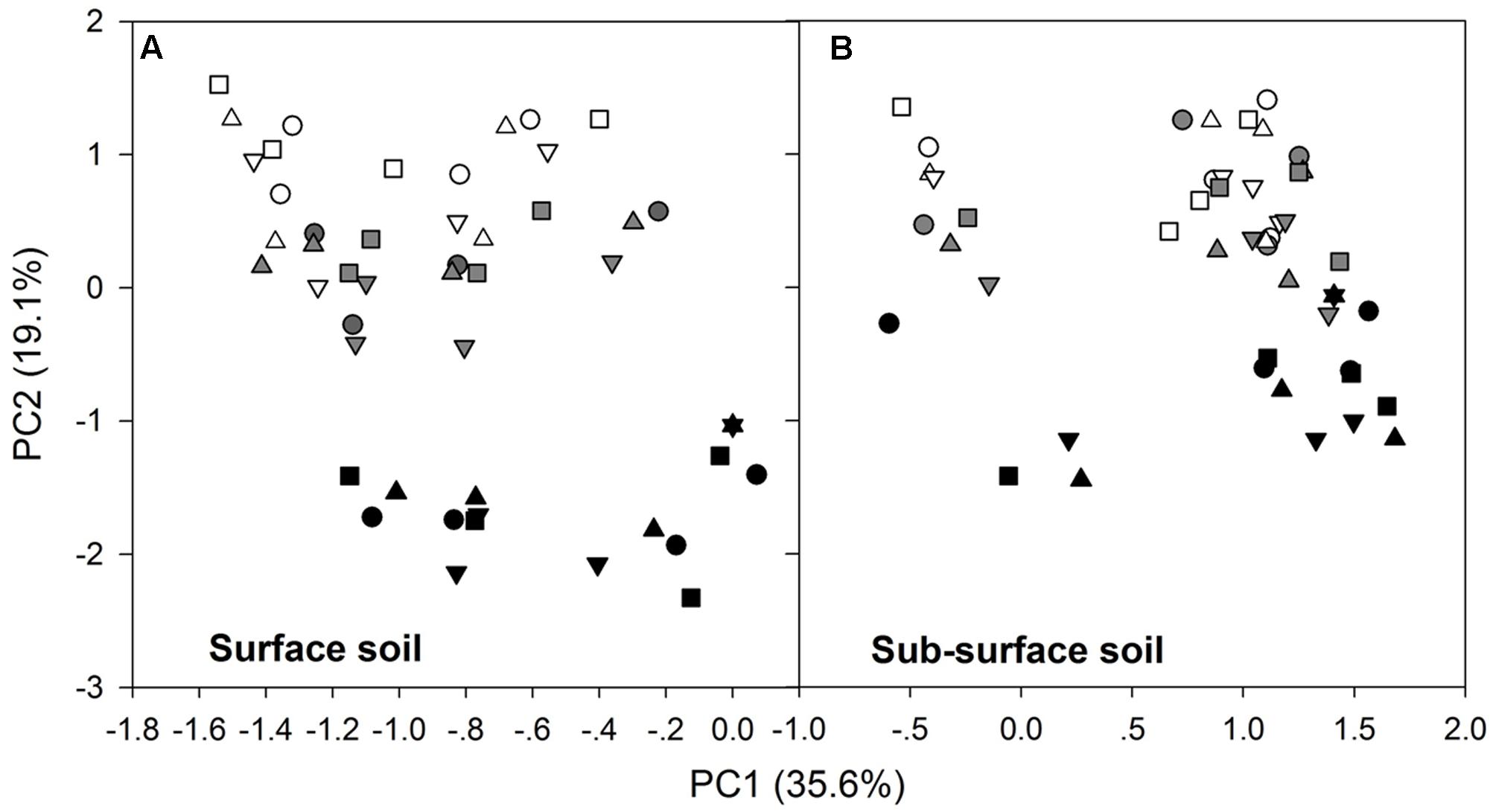
FIGURE 3. Scores of the first two principal components for the surface (A) and subsurface (B) soils. In both panels, the different symbols stand for the different N treatments, i.e., ○ indicates control, △ indicates ammonium addition, ▽ indicates nitrate addition, and □ indicates urea addition. The different colors indicate incubation temperatures, with white used for the 10°C incubation, gray for the 20°C incubation and black for the 30°C incubation.
At the end of the incubation, N addition did not significantly alter the relative abundance of microbial groups in any of the treated soils relative to the control, and N form effect was not significant (p > 0.05, Figures 4, 5). Temperature significantly affected the total PLFAs as well as the relative abundances and ratios of the microbial groups (Figures 4, 5). Briefly, the relative abundance of G+ and the G+/G- PLFA ratio significantly increased with temperature (p < 0.05). The total, G- and fungal PLFAs and F/B were significantly lower under the higher temperature than under the lower temperature for the surface soil (p < 0.05). Likewise, consistent trends were observed for the subsurface soil, but most of the differences were not significant (p > 0.05). Moreover, the subsurface soil had significantly lower microbial biomass, which was indicated by both the fumigation and the PLFA methods (Table 1 and comparing Figures 4A,B), and lower relative abundances of G- and fungal PLFAs (Figure 4). This produces lower F/B but higher G+/G- under all of the experimental combinations of temperature and N form (Figure 5).
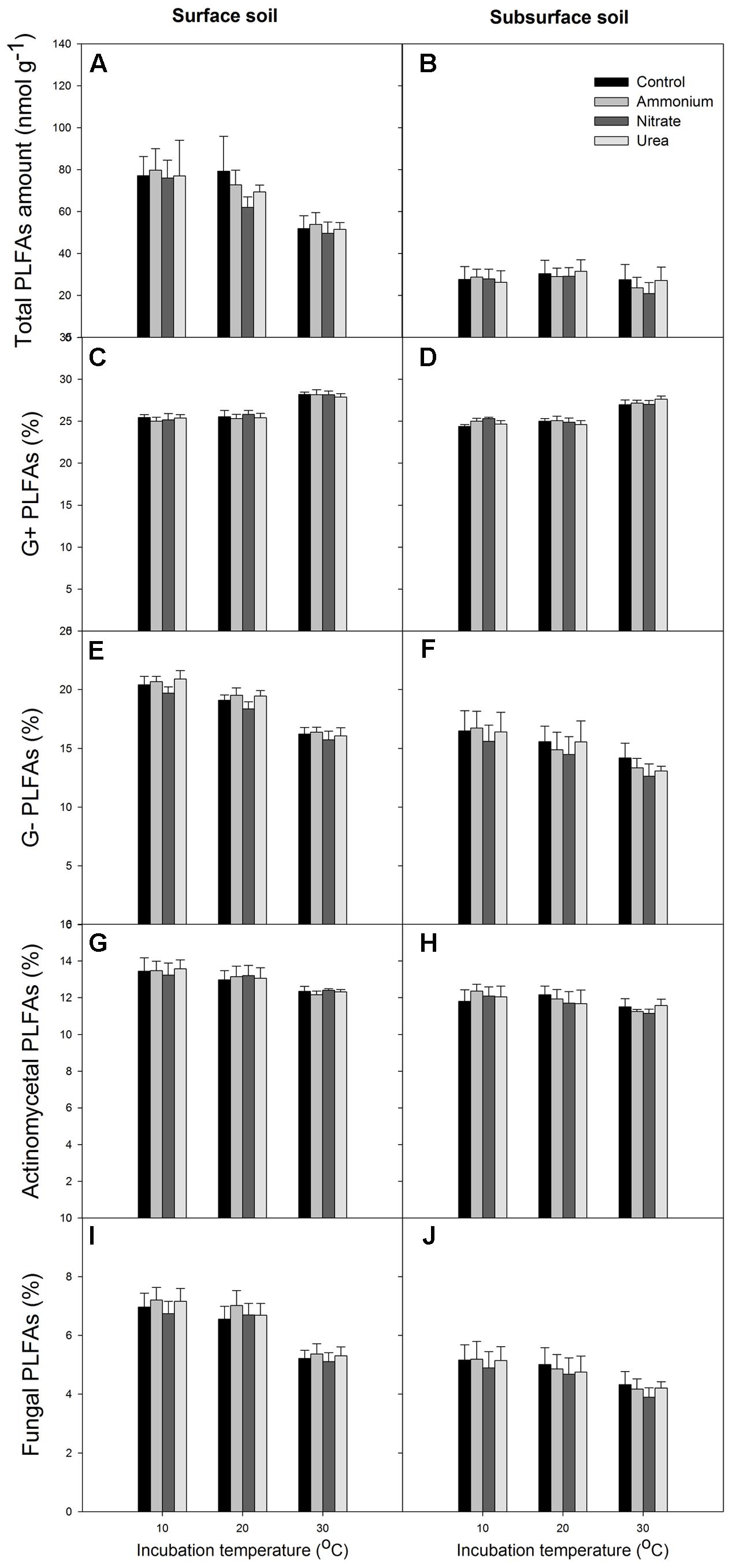
FIGURE 4. Total amount of PLFAs and relative abundance of the different microbial groups for the surface (left column) and subsurface (right column) soils. (A,B) Total PLFAs; (C,D) G+ bacterial PLFAs; (E,F) G- bacterial PLFAs; (G,H) actinomycetal PLFAs; (I,J) fungal PLFAs. In each panel, the bars represent the means, and the error bars indicate the standard errors (n = 4).
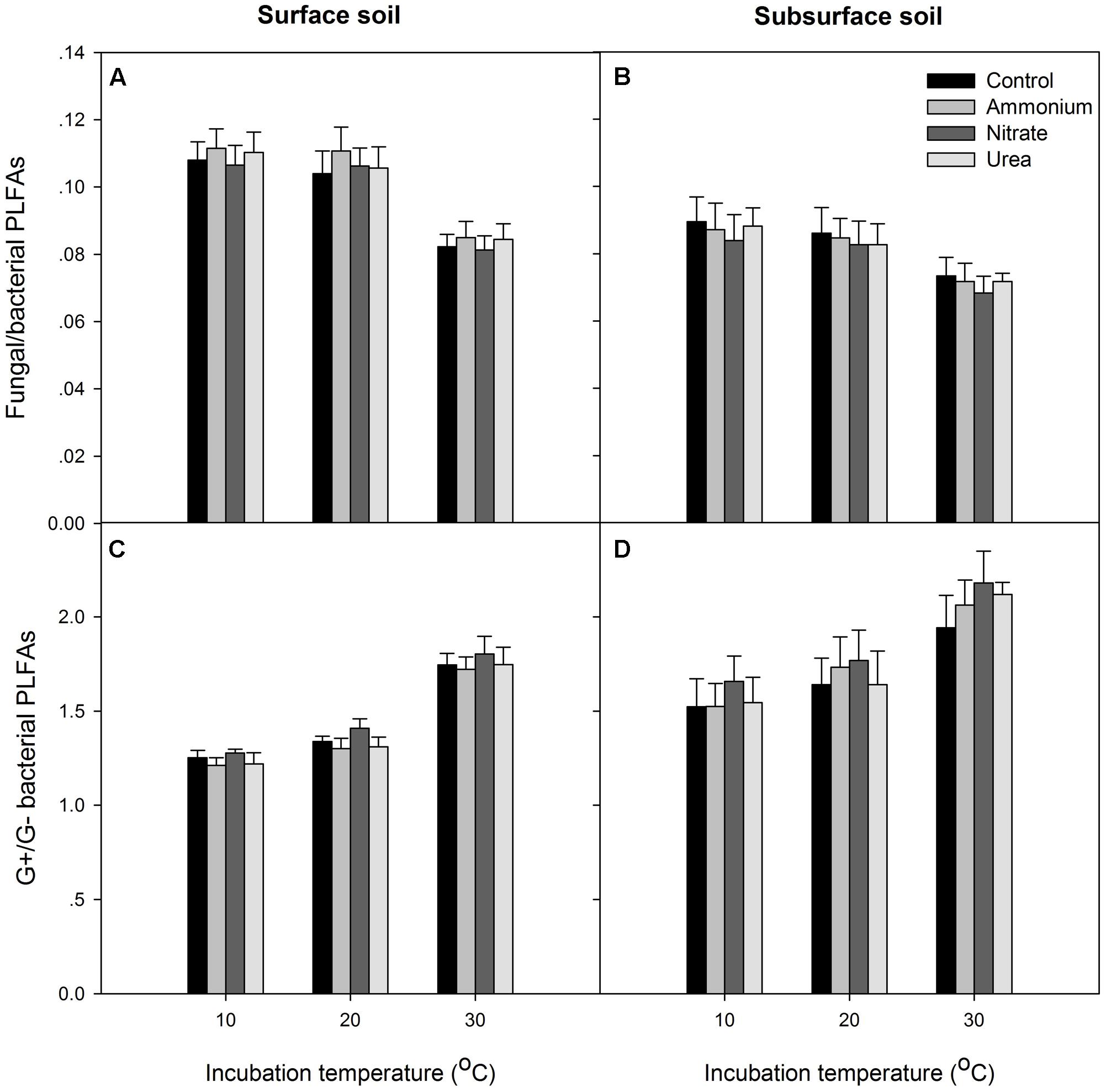
FIGURE 5. The ratios of fungal to bacterial PLFAs and Gram-positive (G+) to Gram-negative (G–) bacterial PLFAs for the two soils. (A,B) The fungal to bacterial ratio; (C,D) the Gram-positive to Gram-negative bacterial ratio. In each panel, the bars represent the means, and the error bars indicate the standard errors (n = 4).
Discussion
N Addition Effects on Soil Microbial Respiration
In this study, we found that only urea addition increased soil CO2 release in the early stage at 10 and 20°C, while ammonium and nitrate additions did not significantly affect soil CO2 emissions in the type of N-rich soils (Figure 1 and Supplementary Figures S1, S2). The significant increase in soil CO2 emissions by urea addition could be derived from the CO2 released via hydrolysis of the added urea in the early stage, as urea is an organic material that can be rapidly hydrolyzed after it is applied to soils. When the first peak points were excluded, the effects of N addition were consistently insignificant under all experimental combinations, suggesting that the current N load may not affect soil C emissions. The neutral N effect could be associated with the comparable soil microbial biomass among the treatments (Figures 4A,B). This observation is consistent with a previous report in which the addition of 50 kg N ha-1 yr-1 (corresponding to the N deposition load at the site) and an even greater N load (100 kg N ha-1 yr-1) did not significantly alter the soil respiration or MBC in the studied forest (Mo et al., 2008), although the plant community diversity and litter decomposition may have been affected (Mo et al., 2006; Lu et al., 2010). Similarly, Deng et al. (2010) reported that a 3-year N addition at a load of 100 kg N ha-1 yr-1 did not significantly affect soil respiration in mimicked young forest ecosystems where the soils were collected from a nearby subtropical evergreen broadleaved forest, although the above- and belowground biomass was higher than that in the control plots. These results, which combine laboratory soil culture and field investigations in young and mature forests, suggest that the soil microbial communities that live in N-rich soils could have been acclimated to the high N conditions and therefore could be highly resistant to a certain amount of extra N input. However, such microbial resistance likely depends on plant growth conditions and substrate needs (e.g., C and N supplies) (Deng et al., 2010).
Previous studies showed that N deposition could depress soil respiration in some ecosystems (Janssens et al., 2010; Chen et al., 2015), while it could stimulate or not affect soil CO2 emissions in other ecosystems (Allison et al., 2008; Zhang et al., 2014). It is likely that the effects of N deposits on soil CO2 release are context-specific and depend greatly on the deposition load and resistance/resilience of the ecosystem components (e.g., tolerance of soil microbial communities) (Knorr et al., 2005; Treseder, 2008; Janssens et al., 2010). In spite of the insignificant N effects observed in this study, it is probable that soil CO2 emissions will be depressed under high N deposition loads as the N deposition increases in this region (Mo et al., 2008; Liu et al., 2013). Soil microbial communities could respond to atmospheric N deposition non-linearly (Zhou et al., 2012; Gao et al., 2016), and there may be a threshold beneath which N deposition will not significantly affect the composition of the soil microbial groups (He et al., 2016). Once the threshold is exceeded, the soil microbial biomass, community activities and diversity will significantly decline (Zhou et al., 2012). However, this threshold varies across different ecosystems and can encompass 2- to 10-fold of the atmospheric N deposition load in studied ecosystems (Mo et al., 2008; Gao et al., 2016; He et al., 2016).
The addition of N decreased the temperature sensitivity of soil CO2 emissions in this study (Figure 2), an observation that is consistent with previous studies (Ramirez et al., 2012; Zhang et al., 2014). However, previous studies in the same region suggest that a certain N load threshold (<150 kg N ha-1 yr-1) could exist, and excess N load over the threshold may reduce the temperature sensitivity of soil respiration in such N-rich forests (Mo et al., 2008), as no significant Q10 decrease was observed under the 100 kg N ha-1 yr-1 N addition treatment in either young or mature forest (Mo et al., 2008; Deng et al., 2010). Our results in which the temperature sensitivity of soil microbial respiration was significantly lower with N treatments than with the control treatment probably indicates that soil microbial communities are more sensitive to atmospheric N deposition than are plants. The significant N effect diminished when the soil CO2 emissions were divided by the total amount of PLFAs accordingly, indicating an underlying association between Q10 and soil microbial biomass (Thiessen et al., 2013). Alternatively, this Q10 reduction could be due to the inhibition of specific microbial species, the adjustment of microbial physiology or shifts in substrate preference under N addition (Treseder, 2008; Allison et al., 2010; Ramirez et al., 2012). Recalcitrant organic matter (OM) is mainly decomposed to obtain N for microbial growth and maintenance by specific microbial groups (Craine et al., 2007; Ramirez et al., 2012). After the easily utilizable N-containing materials were applied, the N inhibition effect and the shift in substrate preference resulted in less decomposition of recalcitrant OM (Carreiro et al., 2000; Treseder, 2008; Du et al., 2014), consequently decreasing the Q10 due to the lower temperature dependency of labile OM than recalcitrant OM (Conant et al., 2008; Xu et al., 2010). This is also evidenced by the observation that the Q10 exhibited the greatest decrease with the addition of urea in the early stage because urea is an easily utilizable organic substrate for soil microorganisms.
Effects of N Form
In contrast to our expectations, we observed that the N effect was independent of N chemistry, as the N type did not cause significantly different soil microbial PLFA compositions (Figures 3–5). The chemistry of the N source added to the soil could affect the microbial activities and respiration (Liu and Greaver, 2010; Du et al., 2014) due to different N substrate preferences (Bardgett et al., 2003), whereas consistent N effects for ammonium, nitrate and urea have also been observed (Ramirez et al., 2010a). It is difficult to completely explain the discrepancy on account of the many underlying factors. In the studied forest, however, the independence could be partly attributed to the continuously high N deposition load and the N-saturated soil conditions (Fang et al., 2008; Chen et al., 2015). Microorganisms that live in N-excess soils could be acclimated to the N-enriched environment. Therefore, exogenous N input at the current deposition rate may not have induced any change in the PLFA-derived soil microbial community composition, regardless of the application of ammonium, nitrate, or urea. However, this result should be interpreted with caution, as the comparable PLFA profiles do not necessarily mean that N form did not significantly affect the soil microbial community composition at other organization levels, e.g., the phylogenetic level. In actuality, the PLFA method is not sensitive enough to detect phylogenetic shifts in soil microbial communities, although it is useful for detecting phenotypic changes (Frostegård et al., 2011). Moreover, our observations do not deny the possibility that a different combination of N load and duration could result in microbial community shifts because soil microbial communities depend greatly on N treatment load and duration (Treseder, 2008; Gao et al., 2016; Wang et al., 2017), consequently changing C cycling (Liu and Greaver, 2010).
Ammonium is the substrate for microbial nitrification, an N transformation process that could potentially enhance the within-soil CO2 uptake (Fleischer and Bouse, 2008). In contrast, nitrate may retard the nitrification process due to it being the product of nitrification, and could therefore increase soil CO2 emissions in accordance with the Le Chatelier principle (Fleischer and Bouse, 2008; Fleischer, 2012). In our study, several PLFAs showed consistent trends in response to N additions, e.g., compared with the control and ammonium addition treatments, nitrate addition reduced the relative abundance of 16:1w7c and 18:1w7c. Such decreases could reduce nitrification rates, as previous studies have suggested that these two PLFAs are dominant fatty acids in N-oxidizing bacteria such as Nitrosomonas and Nitrobacter (Petersen et al., 2004; Börjesson et al., 2011). Consequently, soil CO2 emissions may increase due to the reduced within-soil CO2 uptake (Fleischer and Bouse, 2008; Fleischer, 2012), which is partially supported by the observation that nitrate additions consistently induced higher soil CO2 emissions. This result implies that the decrease in the ammonium/nitrate ratio of atmospheric N deposition (Liu et al., 2013) will tend to cause higher soil CO2 emissions, which increases risk of further accelerating warming by inducing greater positive feedback.
Moreover, although the potential mechanisms remain poorly understood (Sinsabaugh et al., 2005; Cusack, 2013), the ammonium/nitrate ratio positively affects the activities of oxidative enzymes (Cusack, 2013). Such a positive correlation could be due to the inhibition of oxidative enzyme activities by nitrate addition (Saiya-Cork et al., 2002; Sinsabaugh et al., 2005); this phenomenon is often associated with losses of soil microbial biomass (such as white rot basidiomycetes) and reduced microbial investment to produce oxidative enzymes (Sinsabaugh et al., 2005; Sinsabaugh, 2010; Cusack, 2013). Furthermore, reductions in soil oxidative enzyme activity combined with a general increase in the activity of hydrolytic enzymes (e.g., α-glucosidase and cellobiohydrolase) indicate a more rapid turnover and a diminishing stock of labile OM but a slower decomposition of recalcitrant organic compounds (Saiya-Cork et al., 2002; Sinsabaugh et al., 2005). Even though SOM fractions were not investigated in this study, the decreased ammonium/nitrate ratio could be beneficial to the accumulation of recalcitrant OM. This phenomenon further increases the uncertainties of predicting the soil CO2 emissions under the changing N deposition composition in the future.
Comparisons of N Effects between Soil Layers
Within the study period, the CO2 emissions from the surface soil responded more to higher temperatures than the subsurface soil (Figure 2). This is opposite from previous observations in two boreal upland forests in which the subsoil had higher Q10 values than did the surface soil (Karhu et al., 2010). In the present study, this Q10 difference was mainly attributed to the lower soil microbial biomass in the subsurface soils than in the surface soils (Table 1 and Figures 4A,B) because the temperature sensitivity of decomposition greatly depends on soil microbial biomass (Thiessen et al., 2013). This explanation is well supported by our observation that the difference between the surface and subsurface soils became not significant after dividing the CO2 emission by the corresponding total PLFA amounts.
Moreover, the effects of N on the soil microbial communities were consistent between the two layers of soil that exhibited different soil properties. This result does not follow our expectations, albeit soil properties (especially C and N availability) have been suggested to greatly affect the microbial responses to environmental changes (Karhu et al., 2010; Eberwein et al., 2015). This dependence, however, remains inconclusive according to the existing knowledge. Previous studies have also reported consistent N effects on microbial processes and community dynamics across contrasting soils (Ramirez et al., 2010b, 2012), which was also observed in this study. These results suggest that N effects on soil microbial properties could be independent of soil properties in several ecosystems.
Atmospheric N deposition has been reported to decrease Q10 in diverse ecosystems (Mo et al., 2008; Ramirez et al., 2012; Zhang et al., 2014), while positive effects have also been observed (Deng et al., 2010). Such diverse observations among studies are likely due to the relatively low N fertilization rates that occur under the background N deposition and environmental conditions. For example, Mo et al. (2008) reported that low and medium levels of N addition (up to 100 kg N ha-1 yr-1) did not change the temperature sensitivity of soil respiration, but the addition of 150 kg N ha-1 yr-1 significantly decreased the Q10 in the studied forest where atmospheric N deposition was approximately 50 kg N ha-1 yr-1 (Fang et al., 2008). These observations suggest that N deposition could exert consistent effects on microbial activity and community PLFAs across ecosystems, but the consistency may depend greatly on N deposition load.
Conclusion
Exogenous N additions similar to the current N deposition load did not significantly affect soil CO2 emissions or microbial community PLFAs in the two layers of soil collected from a subtropical forest in southern China. The exception was for urea, which stimulated soil CO2 emissions in the early stage. This result implies that soil microbial community PLFAs and activity are resistant to the current deposition loads and the changing composition. However, the addition of N decreased the temperature sensitivity of soil CO2 emissions, indicating that atmospheric N deposition has a negative effect on the microbial response to warming. This further highlights the need to clarify the interactive effects of global changes on ecological processes and functions by conducting multiple-factor experiments. Moreover, similar N effects were observed for the soils that exhibited different soil properties, indicating that there may be a consistent microbial resistance to current N deposition loads and composition changes across those N-rich soils.
Author Contributions
HW and XC carried out the experiment, analyzed the data and wrote the draft manuscript. WS and HW conceived the study. JH and JZ helped with field sampling and laboratory analyses. All authors contributed to manuscript writing and revision.
Funding
This study was financed by the National Natural Science Foundation of China (31500401, 31290222, 31425005, 31130011, and 31400415).
Conflict of Interest Statement
The authors declare that the research was conducted in the absence of any commercial or financial relationships that could be construed as a potential conflict of interest.
Acknowledgments
We thank Dr. Guenet, B. and Professor Janssens, I. for their comments on the initial version of our manuscript. Long, C. Q. and Jia, Y. X. are also acknowledged for their helps on PLFA analysis.
Supplementary Material
The Supplementary Material for this article can be found online at: https://www.frontiersin.org/articles/10.3389/fmicb.2017.02382/full#supplementary-material
References
Allison, S. D., Czimczik, C. I., and Treseder, K. K. (2008). Microbial activity and soil respiration under nitrogen addition in Alaskan boreal forest. Glob. Change Biol. 14, 1156–1168. doi: 10.1111/j.1365-2486.2008.01549.x
Allison, S. D., Wallenstein, M. D., and Bradford, M. A. (2010). Soil-carbon response to warming dependent on microbial physiology. Nat. Geosci. 3, 336–340. doi: 10.1038/ngeo846
Arevalo, C. B. M., Chang, S. X., Bhatti, J. S., and Sidders, D. (2012). Mineralization potential and temperature sensitivity of soil organic carbon under different land uses in the parkland region of Alberta, Canada. Soil Sci. Soc. Am. J. 76, 241–251. doi: 10.2136/sssaj2011.0126
Balser, T. C., McMahon, K. D., Bart, D., Bronson, D., Coyle, D. R., Craig, N., et al. (2006). Bridging the gap between micro - and macro-scale perspectives on the role of microbial communities in global change ecology. Plant Soil 289, 59–70. doi: 10.1007/s11104-006-9104-5
Baran, R., Brodie, E. L., Mayberry-Lewis, J., Hummel, E., Da Rocha, U. N., Chakraborty, R., et al. (2015). Exometabolite niche partitioning among sympatric soil bacteria. Nat. Commun. 6:8289. doi: 10.1038/ncomms9289
Bardgett, R. D., Streeter, T. C., and Bol, R. (2003). Soil microbes compete effectively with plants for organic-nitrogen inputs to temperate grasslands. Ecology 84, 1277–1287. doi: 10.1890/0012-9658(2003)084[1277:SMCEWP]2.0.CO;2
BassiriRad, H. (2015). Consequences of atmospheric nitrogen deposition in terrestrial ecosystems: old questions, new perspectives. Oecologia 177, 1–3. doi: 10.1007/s00442-014-3116-2
Birgander, J., Rousk, J., and Olsson, P. A. (2014). Comparison of fertility and seasonal effects on grassland microbial communities. Soil Biol. Biochem. 76, 80–89. doi: 10.1016/j.soilbio.2014.05.007
Bobbink, R., Hicks, K., Galloway, J., Spranger, T., Alkemade, R., Ashmore, M., et al. (2010). Global assessment of nitrogen deposition effects on terrestrial plant diversity: a synthesis. Ecol. Appl. 20, 30–59. doi: 10.1890/08-1140.1
Börjesson, G., Menichetti, L., Kirchmann, H., and Kätterer, T. (2011). Soil microbial community structure affected by 53 years of nitrogen fertilisation and different organic amendments. Biol. Fertil. Soils 48, 245–257. doi: 10.1007/s00374-011-0623-8
Bossio, D. A., and Scow, K. M. (1998). Impacts of carbon and flooding on soil microbial communities: phospholipid fatty acid profiles and substrate utilization patterns. Microb. Ecol. 35, 265–278. doi: 10.1007/s002489900082
Bradford, M. A., Davies, C. A., Frey, S. D., Maddox, T. R., Melillo, J. M., Mohan, J. E., et al. (2008). Thermal adaptation of soil microbial respiration to elevated temperature. Ecol. Lett. 11, 1316–1327. doi: 10.1111/j.1461-0248.2008.01251.x
Brookes, P. C., Landman, A., Pruden, G., and Jenkinson, D. S. (1985). Chloroform fumigation and the release of soil nitrogen: a rapid direct extraction method to measure microbial biomass nitrogen in soil. Soil Biol. Biochem. 17, 837–842. doi: 10.1016/0038-0717(85)90144-0
Carreiro, M. M., Sinsabaugh, R. L., Repert, D. A., and Parkhurst, D. F. (2000). Microbial enzyme shifts explain litter decay responses to simulated nitrogen deposition. Ecology 81, 2359–2365. doi: 10.2307/177459
Chen, H., Li, D., Gurmesa, G. A., Yu, G., Li, L., Zhang, W., et al. (2015). Effects of nitrogen deposition on carbon cycle in terrestrial ecosystems of China: a meta-analysis. Environ. Pollut. 206, 352–360. doi: 10.1016/j.envpol.2015.07.033
Clark, C. M., Morefield, P. E., Gilliam, F. S., and Pardo, L. H. (2013). Estimated losses of plant biodiversity in the United States from historical N deposition (1985-2010). Ecology 94, 1441–1448. doi: 10.1890/12-2016.1
Conant, R. T., Steinweg, J. M., Haddix, M. L., Paul, E. A., Plante, A. F., and Six, J. (2008). Experimental warming shows that decomposition temperature sensitivity increases with soil organic matter recalcitrance. Ecology 89, 2384–2391. doi: 10.1890/08-0137.1
Corre, M. D., and Lamersdorf, N. P. (2004). Reversal of nitrogen saturation after long-term deposition reduction: impact on soil nitrogen cycling. Ecology 85, 3090–3104. doi: 10.1890/03-0423
Craine, J. M., Morrow, C., and Fierer, N. (2007). Microbial nitrogen limitation increases decomposition. Ecology 88, 2105–2113. doi: 10.1890/06-1847.1
Cusack, D. F. (2013). Soil nitrogen levels are linked to decomposition enzyme activities along an urban-remote tropical forest gradient. Soil Biol. Biochem. 57, 192–203. doi: 10.1016/j.soilbio.2012.07.012
Cusack, D. F., Silver, W. L., Torn, M. S., Burton, S. D., and Firestone, M. K. (2011). Changes in microbial community characteristics and soil organic matter with nitrogen additions in two tropical forests. Ecology 92, 621–632. doi: 10.1890/10-0459.1
Cusack, D. F., Torn, M. S., McDowell, W. H., and Silver, W. L. (2010). The response of heterotrophic activity and carbon cycling to nitrogen additions and warming in two tropical soils. Glob. Change Biol. 16, 2555–2572. doi: 10.1111/j.1365-2486.2009.02131.x
Davidson, E. A., and Janssens, I. A. (2006). Temperature sensitivity of soil carbon decomposition and feedbacks to climate change. Nature 440, 165–173. doi: 10.1038/nature04514
Deng, Q., Zhou, G., Liu, J., Liu, S., Duan, H., and Zhang, D. (2010). Responses of soil respiration to elevated carbon dioxide and nitrogen addition in young subtropical forest ecosystems in China. Biogeosciences 7, 315–328. doi: 10.5194/bg-7-315-2010
Du, Y., Guo, P., Liu, J., Wang, C., Yang, N., and Jiao, Z. (2014). Different types of nitrogen deposition show variable effects on the soil carbon cycle process of temperate forests. Glob. Change Biol. 20, 3222–3228. doi: 10.1111/gcb.12555
Eberwein, J. R., Oikawa, P. Y., Allsman, L. A., and Jenerette, G. D. (2015). Carbon availability regulates soil respiration response to nitrogen and temperature. Soil Biol. Biochem. 88, 158–164. doi: 10.1016/j.soilbio.2015.05.014
Erisman, J. W., Grennfelt, P., and Sutton, M. (2001). Nitrogen emission and deposition: the European perspective. Sci. World 1, 879–896. doi: 10.1100/tsw.2001.285
Fang, Y. T., Gundersen, P., Mo, J. M., and Zhu, W. X. (2008). Input and output of dissolved organic and inorganic nitrogen in subtropical forests of South China under high air pollution. Biogeosciences 5, 339–352. doi: 10.5194/bg-5-339-2008
Fierer, N., Bradford, M. A., and Jackson, R. B. (2007). Toward an ecological classification of soil bacteria. Ecology 88, 1354–1364. doi: 10.1890/05-1839
Fleischer, S. (2012). Interaction between N and C in soil has consequences for global carbon cycling. J. Resour. Ecol. 3, 16–19. doi: 10.5814/j.issn.1674-764x.2012.01.003
Fleischer, S., and Bouse, I. (2008). Nitrogen cycling drives a strong within-soil CO2-sink. Tellus Ser. B Chem. Phys. Meteorol. 60, 782–786. doi: 10.1111/j.1600-0889.2008.00374.x
Frostegård,Å., Tunlid, A., and Bååth, E. (2011). Use and misuse of PLFA measurements in soils. Soil Biol. Biochem. 43, 1621–1625. doi: 10.1016/j.soilbio.2010.11.021
Galloway, J. N., Townsend, A. R., Erisman, J. W., Bekunda, M., Cai, Z. C., Freney, J. R., et al. (2008). Transformation of the nitrogen cycle: recent trends, questions, and potential solutions. Science 320, 889–892. doi: 10.1126/science.1136674
Gao, W., Kou, L., Zhang, J., Müller, C., Wang, H., Yang, H., et al. (2016). Enhanced deposition of nitrate alters microbial cycling of N in a subtropical forest soil. Biol. Fertil. Soils 52, 977–986. doi: 10.1007/s00374-016-1134-4
Gershenson, A., Bader, N. E., and Cheng, W. (2009). Effects of substrate availability on the temperature sensitivity of soil organic matter decomposition. Glob. Change Biol. 15, 176–183. doi: 10.1111/j.1365-2486.2008.01827.x
Hanson, P., Edwards, N., Garten, C., and Andrews, J. (2000). Separating root and soil microbial contributions to soil respiration: a review of methods and observations. Biogeochemistry 48, 115–146. doi: 10.1023/A:1006244819642
He, D., Xiang, X., He, J.-S., Wang, C., Cao, G., Adams, J., et al. (2016). Composition of the soil fungal community is more sensitive to phosphorus than nitrogen addition in the alpine meadow on the Qinghai-Tibetan Plateau. Biol. Fertil. Soils 52, 1059–1072. doi: 10.1007/s00374-016-1142-4
Hobbie, S. E. (2008). Nitrogen effects on decomposition: a five-year experiment in eight temperate sites. Ecology 89, 2633–2644. doi: 10.1890/07-1119.1
Hungate, B. A., van Groenigen, K. J., Six, J., Jastrow, J. D., Luo, Y. Q., de Graaff, M. A., et al. (2009). Assessing the effect of elevated carbon dioxide on soil carbon: a comparison of four meta-analyses. Glob. Change Biol. 15, 2020–2034. doi: 10.1111/j.1365-2486.2009.01866.x
Hunova, I., Kurfurst, P., Stranik, V., and Modlik, M. (2017). Nitrogen deposition to forest ecosystems with focus on its different forms. Sci. Total Environ. 575, 791–798. doi: 10.1016/j.scitotenv.2016.09.140
IPCC (2013). Climate Change 2013: The Physical Science Basis. Geneva: Intergovernmental Panel on Climate Change.
Janssens, I. A., Dieleman, W., Luyssaert, S., Subke, J. A., Reichstein, M., Ceulemans, R., et al. (2010). Reduction of forest soil respiration in response to nitrogen deposition. Nat. Geosci. 3, 315–322. doi: 10.1038/ngeo844
Karhu, K., Fritze, H., Tuomi, M., Vanhala, P., Spetz, P., Kitunen, V., et al. (2010). Temperature sensitivity of organic matter decomposition in two boreal forest soil profiles. Soil Biol. Biochem. 42, 72–82. doi: 10.1016/j.soilbio.2009.10.002
Knorr, M., Frey, S. D., and Curtis, P. S. (2005). Nitrogen additions and litter decomposition: a meta-analysis. Ecology 86, 3252–3257. doi: 10.1890/05-0150
Leckie, S. E. (2005). Methods of microbial community profiling and their application to forest soils. For. Ecol. Manag. 220, 88–106. doi: 10.1016/j.foreco.2005.08.007
Liu, G. (1996). Soil Physicochemical Analysis and Description of Soil Profiles. Beijing: China Normative Publishing House.
Liu, L., and Greaver, T. L. (2010). A global perspective on belowground carbon dynamics under nitrogen enrichment. Ecol. Lett. 13, 819–828. doi: 10.1111/j.1461-0248.2010.01482.x
Liu, X., Yang, Z., Lin, C., Giardina, C. P., Xiong, D., Lin, W., et al. (2017). Will nitrogen deposition mitigate warming-increased soil respiration in a young subtropical plantation? Agric. For. Meteorol. 246, 78–85. doi: 10.1016/j.agrformet.2017.06.010
Liu, X., Zhang, Y., Han, W., Tang, A., Shen, J., Cui, Z., et al. (2013). Enhanced nitrogen deposition over China. Nature 494, 459–462. doi: 10.1038/nature11917
Lloyd, J., and Taylor, J. A. (1994). On the temperature dependence of soil respiration. Funct. Ecol. 8, 315–323. doi: 10.2307/2389824
Lu, X., Mao, Q., Mo, J., Gilliam, F. S., Zhou, G., Luo, Y., et al. (2015). Divergent responses of soil buffering capacity to long-term N deposition in three typical tropical forests with different land-use history. Environ. Sci. Technol. 49, 4072–4080. doi: 10.1021/es5047233
Lu, X. K., Mo, J. M., Gilliam, F. S., Zhou, G. Y., and Fang, Y. T. (2010). Effects of experimental nitrogen additions on plant diversity in an old-growth tropical forest. Glob. Change Biol. 16, 2688–2700. doi: 10.1111/j.1365-2486.2010.02174.x
Martin Wetterstedt, J. Å., Persson, T., and Ågren, G. I. (2009). Temperature sensitivity and substrate quality in soil organic matter decomposition: results of an incubation study with three substrates. Glob. Change Biol. 16, 1806–1819. doi: 10.1111/j.1365-2486.2009.02112.x
Mo, J., Zhang, W., Zhu, W., Gundersen, P., Fang, Y., Li, D., et al. (2008). Nitrogen addition reduces soil respiration in a mature tropical forest in southern China. Glob. Change Biol. 14, 403–412. doi: 10.1111/j.1365-2486.2007.01503.x
Mo, J. M., Brown, S., Xue, J., Fang, Y., and Li, Z. (2006). Response of litter decomposition to simulated N deposition in disturbed, rehabilitated and mature forests in subtropical China. Plant Soil 282, 135–151. doi: 10.1007/s11104-005-5446-7
Nunan, N., Singh, B., Reid, E., Ord, B., Papert, A., Squires, J., et al. (2006). Sheep-urine-induced changes in soil microbial community structure. FEMS Microbiol. Ecol. 56, 310–320. doi: 10.1111/j.1574-6941.2006.00072.x
Petersen, S. O., Roslev, P., and Bol, R. (2004). Dynamics of a pasture soil microbial community after deposition of cattle urine amended with [13C] urea. Appl. Environ. Microbiol. 70, 6363–6369. doi: 10.1128/AEM.70.11.6363-6369.2004
Ramirez, K. S., Craine, J. M., and Fierer, N. (2010a). Nitrogen fertilization inhibits soil microbial respiration regardless of the form of nitrogen applied. Soil Biol. Biochem. 42, 2336–2338. doi: 10.1016/j.soilbio.2010.08.032
Ramirez, K. S., Lauber, C. L., Knight, R., Bradford, M. A., and Fierer, N. (2010b). Consistent effects of nitrogen fertilization on soil bacterial communities in contrasting systems. Ecology 91, 3463–3470. doi: 10.1890/10-0426.1
Ramirez, K. S., Craine, J. M., and Fierer, N. (2012). Consistent effects of nitrogen amendments on soil microbial communities and processes across biomes. Glob. Change Biol. 18, 1918–1927. doi: 10.1111/j.1365-2486.2012.02639.x
Reay, D. S., Dentener, F., Smith, P., Grace, J., and Feely, R. A. (2008). Global nitrogen deposition and carbon sinks. Nat. Geosci. 1, 430–437. doi: 10.1038/ngeo230
Rogora, M., Mosello, R., Arisci, S., Brizzio, M., Barbieri, A., Balestrini, R., et al. (2006). An overview of atmospheric deposition chemistry over the Alps: present status and long-term trends. Hydrobiologia 562, 17–40. doi: 10.1007/s10750-005-1803-z
Saiya-Cork, K. R., Sinsabaugh, R. L., and Zak, D. R. (2002). The effects of long term nitrogen deposition on extracellular enzyme activity in an Acer saccharum forest soil. Soil Biol. Biochem. 34, 1309–1315. doi: 10.1016/S0038-0717(02)00074-3
Sinsabaugh, R. L. (2010). Phenol oxidase, peroxidase and organic matter dynamics of soil. Soil Biol. Biochem. 42, 391–404. doi: 10.1016/j.soilbio.2009.10.014
Sinsabaugh, R. L., Gallo, M. E., Lauber, C., Waldrop, M. P., and Zak, D. R. (2005). Extracellular enzyme activities and soil organic matter dynamics for northern hardwood forests receiving simulated nitrogen deposition. Biogeochemistry 75, 201–215. doi: 10.1007/s10533-004-7112-1
Tang, X., Liu, S., Zhou, G., Zhang, D., and Zhou, C. (2006). Soil-atmospheric exchange of CO2, CH4, and N2O in three subtropical forest ecosystems in southern China. Glob. Change Biol. 12, 546–560. doi: 10.1111/j.1365-2486.2006.01109.x
Thiessen, S., Gleixner, G., Wutzler, T., and Reichstein, M. (2013). Both priming and temperature sensitivity of soil organic matter decomposition depend on microbial biomass – An incubation study. Soil Biol. Biochem. 57, 739–748. doi: 10.1016/j.soilbio.2012.10.029
Torsvik, V., and Øvreås, L. (2002). Microbial diversity and function in soil: from genes to ecosystems. Curr. Opin. Microbiol. 5, 240–245. doi: 10.1016/S1369-5274(02)00324-7
Treseder, K. K. (2008). Nitrogen additions and microbial biomass: a meta-analysis of ecosystem studies. Ecol. Lett. 11, 1111–1120. doi: 10.1111/j.1461-0248.2008.01230.x
Turner, M. M., and Henry, H. A. L. (2009). Interactive effects of warming and increased nitrogen deposition on 15N tracer retention in a temperate old field: seasonal trends. Glob. Change Biol. 15, 2885–2893. doi: 10.1111/j.1365-2486.2009.01881.x
Vance, E. D., Brookes, P. C., and Jenkinson, D. (1987). An extraction method for measuring soil microbial biomass C. Soil Biol. Biochem. 19, 703–707. doi: 10.1016/0038-0717(87)90052-6
Wang, R., Dorodnikov, M., Dijkstra, F. A., Yang, S., Xu, Z., Li, H., et al. (2017). Sensitivities to nitrogen and water addition vary among microbial groups within soil aggregates in a semiarid grassland. Biol. Fertil. Soils 53, 129–140. doi: 10.1007/s00374-016-1165-x
Wei, H., Chen, X. M., Xiao, G. L., Guenet, B., Vicca, S., and Shen, W. J. (2015). Are variations in heterotrophic soil respiration related to changes in substrate availability and microbial biomass carbon in the subtropical forests? Sci. Rep. 5:18370. doi: 10.1038/srep18370
Wei, H., Guenet, B., Vicca, S., Nunan, N., AbdElgawad, H., Pouteau, V., et al. (2014). Thermal acclimation of organic matter decomposition in an artificial forest soil is related to shifts in microbial community structure. Soil Biol. Biochem. 71, 1–12. doi: 10.1016/j.soilbio.2014.01.003
Wu, J., Joergensen, R. G., Pommerening, B., Chaussod, R., and Brookes, P. C. (1990). Measurement of soil microbial biomass C by fumigation-extraction-an automated procedure. Soil Biol. Biochem. 22, 1167–1169. doi: 10.1016/j.talanta.2010.01.026
Xu, X., Zhou, Y., Ruan, H., Luo, Y., and Wang, J. (2010). Temperature sensitivity increases with soil organic carbon recalcitrance along an elevational gradient in the Wuyi Mountains, China. Soil Biol. Biochem. 42, 1811–1815. doi: 10.1016/j.soilbio.2010.06.021
Zhang, C., Niu, D., Hall, S. J., Wen, H., Li, X., Fu, H., et al. (2014). Effects of simulated nitrogen deposition on soil respiration components and their temperature sensitivities in a semiarid grassland. Soil Biol. Biochem. 75, 113–123. doi: 10.1016/j.soilbio.2014.04.013
Zhang, W., Mo, J., Fang, Y., Lu, X., and Wang, H. (2008). Effects of nitrogen deposition on the greenhouse gas fluxes from forest soils. Acta Ecol. Sin. 28, 2309–2319. doi: 10.1016/s1872-2032(08)60047-5
Zhou, W., Hui, D., and Shen, W. (2014). Effects of soil moisture on the temperature sensitivity of soil heterotrophic respiration: a laboratory incubation study. PLOS ONE 9:e92531. doi: 10.1371/journal.pone.0092531
Keywords: microbial communities, N deposition, greenhouse gas, SOM decomposition, Q10 index, global change
Citation: Wei H, Chen X, He J, Zhang J and Shen W (2017) Exogenous Nitrogen Addition Reduced the Temperature Sensitivity of Microbial Respiration without Altering the Microbial Community Composition. Front. Microbiol. 8:2382. doi: 10.3389/fmicb.2017.02382
Received: 29 August 2017; Accepted: 17 November 2017;
Published: 01 December 2017.
Edited by:
Per Bengtson, Lund University, SwedenReviewed by:
Minna Männistö, Natural Resources Institute Finland, FinlandChristopher Blackwood, Kent State University, United States
Copyright © 2017 Wei, Chen, He, Zhang and Shen. This is an open-access article distributed under the terms of the Creative Commons Attribution License (CC BY). The use, distribution or reproduction in other forums is permitted, provided the original author(s) or licensor are credited and that the original publication in this journal is cited, in accordance with accepted academic practice. No use, distribution or reproduction is permitted which does not comply with these terms.
*Correspondence: Weijun Shen, c2hlbndlaWpAc2NiZy5hYy5jbg==
†These authors have contributed equally to this work.