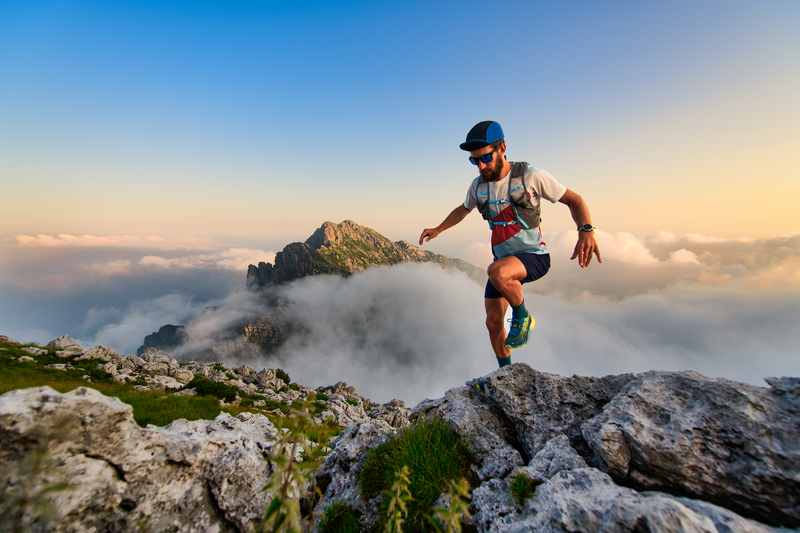
95% of researchers rate our articles as excellent or good
Learn more about the work of our research integrity team to safeguard the quality of each article we publish.
Find out more
ORIGINAL RESEARCH article
Front. Microbiol. , 28 June 2017
Sec. Food Microbiology
Volume 8 - 2017 | https://doi.org/10.3389/fmicb.2017.01137
This article is part of the Research Topic Microorganisms for a Sustainable Viticulture and Winemaking View all 16 articles
Previous studies reported that the use of Metschnikowia pulcherrima in sequential culture fermentation with Saccharomyces cerevisiae mainly induced a reduction of volatile acidity in wine. The impact of the presence of this yeast on the metabolic pathway involved in pyruvate dehydrogenase (PDH) bypass and glycerol production in S. cerevisiae has never been investigated. In this work, we compared acetic acid and glycerol production kinetics between pure S. cerevisiae culture and its sequential culture with M. pulcherrima during alcoholic fermentation. In parallel, the expression levels of the principal genes involved in PDH bypass and glyceropyruvic fermentation in S. cerevisiae were investigated. A sequential culture of M. pulcherrima/S. cerevisiae at an inoculation ratio of 10:1 produced 40% less acetic acid than pure S. cerevisiae culture and led to the enhancement of glycerol content (12% higher). High expression levels of pyruvate decarboxylase PDC1 and PDC5, acetaldehyde dehydrogenase ALD6, alcohol dehydrogenase ADH1 and glycerol-3-phosphate dehydrogenase PDC1 genes during the first 3 days of fermentation in sequential culture conditions are highlighted. Despite the complexity of correlating gene expression levels to acetic acid formation kinetics, we demonstrate that the acetic acid production pathway is altered by sequential culture conditions. Moreover, we show for the first time that the entire acetic acid and glycerol metabolic pathway can be modulated in S. cerevisiae by the presence of M. pulcherrima at the beginning of fermentation.
Complex interactions between organisms occur when fermentations are conducted with different yeasts (Fleet, 2003; Alexandre et al., 2004; Liu et al., 2015; Albergaria and Arneborg, 2016; Ciani et al., 2016). Considerable differences have been shown in the metabolism of Saccharomyces cerevisiae in single and in co-culture with non-Saccharomyces yeasts. Moreira et al. (2005) reported an increase in the quantity of desirable compounds, such as higher alcohols and esters, when S. cerevisiae was co-fermented with Hanseniaspora uvarum. A previous study (Sadoudi et al., 2012) based on the analysis of 48 volatile compounds belonging to different chemical families, highlighted the existence of different types of interactions independent of biomass production between non-Saccharomyces yeasts co-cultured with S. cerevisiae. More precisely, a positive interaction (synergistic effect) between Metschnikowia pulcherrima and S. cerevisiae resulted in a higher level of aromatic compounds than the sum of the aromatic compounds present in each monoculture. In addition, in a sequential M. pulcherrima/S. cerevisiae culture, acetic acid production was significantly lower compared to that obtained with a S. cerevisiae monoculture. Different studies reported low acetic acid production for certain non-Saccharomyces yeasts (M. pulcherrima, Torulaspora delbrueckii, Starmerella bacillaris) and their capacity in culture with S. cerevisiae to produce lower acetic acid concentrations in comparison to S. cerevisiae monoculture (Bely et al., 2008; Comitini et al., 2011; Milanovic et al., 2012; Rantsiou et al., 2012). These studies suggest that the acetic acid metabolic pathway can be affected by interactions occurring between yeasts, leading to a decrease in the amount of acetic acid. However, little is known as yet of the impact of sequential non-Saccharomyces/S. cerevisiae culture on the genes involved in the acetic acid metabolic pathway of S. cerevisiae.
Acetic acid is the principal volatile acid of wine. It has a negative impact on yeast fermentative performance and affects the quality of some wines when present above a given concentration (Rasmussen et al., 1995). The OIV (2010) states that the maximum acceptable limit for volatile acidity for most wines is 1.2 g l-1 of acetic acid. Unfortunately, higher levels are sometimes produced, depending on the strain (Erasmus et al., 2004; Orlić et al., 2010), on grape or must composition (Delfini and Costa, 1993) and on the winemaking process (Barbosa et al., 2009). Therefore, strains with reduced acetate production would have a high enological value. Studies on the production of volatile acidity by S. cerevisiae in winemaking conditions showed that this acid is mainly formed at the beginning of alcoholic fermentation (Alexandre et al., 2004; Bely et al., 2008). Acetic acid is formed rapidly during the fermentation of the first 50–100 g l-1 of sugar, but part of it is metabolized by S. cerevisiae (Ribéreau-Gayon et al., 2006). This yeast can also assimilate acetic acid added at the beginning of alcoholic fermentation (Vasserot et al., 2010).
Acetic acid is a by-product of alcoholic fermentation produced via the pyruvate dehydrogenase (PDH) bypass (Figure 1). It is produced at the onset of anaerobic growth conditions, as a reducing equivalents regeneration mechanism (NADH and NADPH) essential for maintaining the redox balance (Remize et al., 2000). Enzymes involved in the PDH bypass include pyruvate decarboxylase (Pdc), acetaldehyde dehydrogenase (Ald), and acetyl-CoA synthetase (Acs) (Figure 1). The PDH complex leads to the formation of acetyl-CoA in the mitochondria through the oxidative decarboxylation of pyruvate. However, S. cerevisiae is unable to transport acetyl-CoA out of the mitochondria. Moreover, cytosolic NADP+-dependent Ald is active during alcoholic fermentation, while PDH activity is limited under anaerobic conditions (Remize et al., 2000). Therefore, the PDH bypass is necessary for providing acetyl-CoA in the cytosolic compartment which is used, inter alia, in lipid synthesis (for a review, see Pronk et al., 1996).
FIGURE 1. Enzymes and main genes involved in PDH bypass and in glycerol production (glyceropyruvic fermentation).
Pdc catalyzes the decarboxylation of pyruvate to acetaldehyde and carbon dioxide. In S. cerevisiae, Pdc is encoded by three structural genes, PDC1, PDC5, and PDC6 which encode Pdc1, Pdc5, and Pdc6 isoforms, respectively (Hohmann, 1991; Pronk et al., 1996). Pdc1 and Pdc5 are 88% identical (Hohmann and Cederberg, 1990). Pdc1 is the predominant isoenzyme form, performing 80–90% of the activity in cells. The Pdc6p is an active Pdc (Hohmann, 1991; Zeng et al., 1993; Baburina et al., 1994) but is not apparently involved in glucose fermentation and its role remains unclear (Hohmann, 1991). The regulatory genes PDC2, PDC3, and PCD4 encode probably positive transcriptional regulators required for high-level expression of structural PDC1 and PDC5 genes (Milanovic et al., 2012).
Ald is responsible for the conversion of acetaldehyde to acetate. The S. cerevisiae Ald family counts five isoenzymes localized in the mitochondria or the cytosol. Ald6 and Ald4 have been shown to be the main cytosolic and mitochondrial Ald, respectively. Cytosolic Ald is encoded by ALD2, ALD3, and ALD6 (occasionally named ALD1) genes and the mitochondrial enzymes are encoded by ALD4 (occasionally named ALD7) and ALD5 genes (Navarro-Aviño et al., 1999). Ald6 uses the NADP+ co-enzyme, activated by Mg2+, and is not glucose-repressed (Dickinson, 1996; Meaden et al., 1997). Ald4 uses both the NAD+ and NADP+ co-enzymes activated by K+ and thiols, and it is highly glucose-repressed (Jacobson and Bernofsky, 1974). Numerous studies stated that cytosolic Ald is responsible for the formation of acetate from glucose and that mitochondrial enzymes are involved during growth on ethanol or glycerol as carbon sources (Saigal et al., 1991; Wang et al., 1998). Remize et al. (2000) showed that a strain deleted in the ALD6 gene led to a considerable decrease in acetate yield. The absence of Ald6p was compensated by mitochondrial isoforms, involving the transcriptional activation of the ALD4 gene (Saint-Prix et al., 2004). More recently, it was demonstrated that the fermentation stress response gene AAF1 regulates acid acetic production under standard laboratory conditions. This gene encodes a probable transcription factor, containing a C2-H2 zinc finger domain at the N-terminus. Indeed, AAF1 regulates the expression of ALD4 and ALD6 (Walkey et al., 2012). The deletion of this gene significantly reduced acetic acid levels without increasing the acetaldehyde concentration in wine (Luo et al., 2013).
Acs catalyzes the formation of acetyl-CoA from acetate. S. cerevisiae contains two structural genes ACS1 and ACS2, each encoding an active Acs (Van den Berg et al., 1996). It has been shown that Acs is an essential enzyme in S. cerevisiae. A disruption of both ACS1 and ACS2 genes is lethal (Van den Berg and Steensma, 1995).
An imbalance of reduction equivalents at the beginning of S. cerevisiae growth in must, due to the initial lack of alcohol dehydrogenase, triggers another mechanism: glycerol production (Gancedo and Serrano, 1989) (Figure 1). Dihydroxyacetone phosphate, the substrate for the glycerol formation pathway, can be provided either by the glycolytic degradation of sugar or by gluconeogenic flux when non-fermentable carbon sources are used (Nevoigt and Stahl, 1997). Dihydroxyacetone phosphate is converted to glycerol-3-phosphate, which is an intermediate for glycerol formation. Two homologous genes GPD1 and GPD2 encode the isoenzymes glycerol-3-phosphate dehydrogenase (Gpd). GPD1 expression is induced by osmotic stress. The repressor/activator Rap1p was demonstrated to be an important determinant of induced transcriptional activities of the GPD1 promoter (Eriksson et al., 2000). Expression of GPD2 is not affected by changes in external osmolarity, but it is stimulated by anoxic conditions (Ansell et al., 1997). A recent study by Pérez-Torrado et al. (2016) showed the induction of GPD1 after the first hour of growth in wine fermentation conditions for different Saccharomyces species. For the GPD2 gene, the time and the level of induction seem to be species- or strain-dependent. Moreover, some strains do not seem to activate this gene which presents very low mRNA levels.
In the present study, we performed sequential fermentations, combining M. pulcherrima and S. cerevisiae strains, in order to evaluate the effect of the presence of M. pulcherrima on the production of acetic acid and glycerol during alcoholic fermentation. Moreover, the impact of this sequential culture on the expression of genes in S. cerevisiae encoding enzymes involved in acetic acid and glycerol pathways during alcoholic fermentation was investigated.
The commercial strain S. cerevisiae PB2023 (SPINDAL-AEB group) was used as control strain. The non-Saccharomyces M. pulcherrima MCR-24 strain (accession number: JX234570) used in this study was previously isolated from Pinot Noir grape must. This strain was selected for its alcoholic fermentation performance (completion of alcoholic fermentation producing around 11% v/v ethanol) and its low acetic acid production (Sadoudi et al., 2012).
Sauvignon Blanc grape must (112 g l-1 glucose, 109 g l-1 fructose, 3.1 g l-1 L-malic acid, 378 mg l-1 total nitrogen, pH 3.35) supplemented with sulfur dioxide (30 mg l-1) was used in the fermentation tests. The must was pasteurized at 100°C for 10 min and the effectiveness of this treatment was verified by plating on YPD solid medium (20 g l-1 glucose, 5 g l-1 yeast extract, 10 g l-1 peptone, 0.2 g l-1 chloramphenicol, agar 20 g l-1). YPD liquid medium was used for yeast pre-cultures before inoculation in musts.
YPD solid medium was used for viable cell counting (non-Saccharomyces or S. cerevisiae yeasts) during mono-culture fermentations and total viable cell counting (both non-Saccharomyces and S. cerevisiae yeasts) during sequential fermentations.
Lysine agar (LA) medium [66 g l-1 Lysine medium (Oxoid), 10 ml 50% potassium lactate, 0.11 ml 90% lactic acid, and 0.2 g l-1 chloramphenicol] was used for viable cell counting of non-Saccharomyces yeast during sequential fermentation. LA medium is a selective medium which limits the growth of S. cerevisiae (Lin, 1975). The number of S. cerevisiae cells was given as the difference between the total plate count using YPD agar and the plate count using LA.
Fermentations were carried out for S. cerevisiae PB2023 in pure culture and M. pulcherrima MCR-24/S. cerevisiae PB2023 in mixed cultures.
Pure cultures were carried out in 500 ml Erlenmeyer flasks containing 350 ml of Sauvignon Blanc grape must and closed with dense cotton plugs. Yeasts were pre-cultured in YPD medium at 30°C for 48 h and then inoculated in musts at a concentration of 106 cells ml-1. Fermentations were carried out in triplicate at 20°C, without shaking. Fermentation progress and yeast growth were monitored throughout the fermentation process by measuring sugar concentration and by viable cells counts.
Sequential fermentations were carried out in 500 ml Erlenmeyer flasks containing 350 ml of the same must as described above. Before must inoculation, S. cerevisiae PB2023 and M. pulcherrima MCR-24 were pre-cultured in YPD medium for 48h. M. pulcherrima MCR 24 and S. cerevisiae PB2023 were then sequentially inoculated at a ratio of 10:1. M. pulcherrima MCR 24 was inoculated at 107 cells ml-1 and after 48 h, S. cerevisiae PB2023 was introduced at 106 cells ml-1. Each experiment was performed in triplicate at 20°C under static conditions. Fermentation progress and yeast growth were monitored throughout the fermentation process by measuring sugar concentration and by viable cell counts, as described previously.
Samples of the fermenting must were taken at different stages of fermentation (-2, -1, 0, 1, 2, 3, 4, 5, 6, and 8 days of fermentation) from each fermentation trial. Day “-2” corresponds to the day of inoculation with M. pulcherrima MCR 24 strain and day “0” corresponds to the day when S. cerevisiae PB2023 was added. One part of each sample was used to determine the cell number. The other part of the sample was centrifuged at 1000 rpm for 5 min at 4°C. Supernatants were stored at -20°C and analyzed later to determine residual sugar, ethanol, glycerol, and acetic acid concentrations. The cell pellet was collected for RNA extraction. The RNA extractions were performed from the day “1” of fermentation until the end of the process.
Glucose, fructose, ethanol, glycerol, and acetic acid were determined using enzymatic kits following the manufacturer’s instructions (Bio-SenTec, France). Total acidity was determined by the potentiometric method. The wine was decarbonated and then titrated by NaOH 0.1 N solution until pH 7. The result was expressed in g l-1 tartaric acid.
Total RNAs extraction was performed using a commercial RNeasy kit (Qiagen) with slight modifications. After centrifugation, cells were added to the extraction buffer together with 600 μl of sterile glass beads (0.5 mm in diameter). The cells were then disrupted using the Precellys instrument (Bertin Technologies, France) at 6500 g for 30 s followed by chilling on ice for 30 s. This step was repeated six times. The extraction was then continued according to the manufacturer’s instructions (Qiagen).
The extracted RNA was quantified by measuring absorbance at 260 nm using a bio-photometer (Eppendorf). The RNAs (2 μg of total RNA) were treated with 5 U of DNase (Fermentas/Thermo Fisher Scientific, France) following the protocol described by the manufacturer. As a quality control assay, the absence of contaminant genomic DNA in RNA preparations was checked before cDNA synthesis using RNA as a template in real-time PCR assays (RNA not reverse-transcribed to cDNA). cDNA was then synthesized from 1 μg of total RNA in 20 μl reaction mixture using the iScript cDNA synthesis kit (Bio-Rad, France). Each RNA extraction was performed in triplicate.
The primers for RT-PCR (target and housekeeping reference genes) given in Table 1 were designed using the free online Primer3 0.4.0 software1. The primers were designed to have length about 18–22 bp, a G/C content of over 50%, and a Tm of about 60°C. The PCR product sizes ranged from 90 to 120 bp. Secondary structures and dimers formation were controlled with the Oligo Analyzer 1.0.3.0 software. Primer specificity and PCR product size were obtained in silico from the entire genome of the S288C strain2.
PGK1 and TDH2 genes (Table 1) were used as housekeeping reference genes because they were shown to be two genes whose expression remained stable and independent of growth conditions, as highlighted by (Vaudano et al., 2011).
Primers were purchased from Eurogentec, Belgium. In order to confirm the specificity of the primers only for S. cerevisiae genomic DNA in sequential culture samples, each couple of primers was tested in RT-qPCR using the genomic DNA of S. cerevisiae or M. pulcherrima as a template. No amplification was detected in the M. pulcherrima genomic DNA template (data not shown).
Real time PCR was performed in 96-well plates on a CFX-96TM Real Time system (Bio-Rad) using SYBR Green as fluorophore. Reactions were carried out in 25 μl of mix containing 12.5 μl of PCR master mix (Promega), 2.0 μl of primer mix (7 pM final concentration), 5.5 μl of DNase and RNase free H2O, and 5 μl of cDNA. Positive (S. cerevisiae genomic DNA as template) and negative (water as template) controls were also incorporated in each assay. The thermocycling program consisted of one hold at 95°C for 3 min; 40 cycles of 10 s at 95°C, 30 s at 60°C and 30 s at 72°C and a final extension at 72°C for 5 min. After the completion of the thermocycling program, melting curve data were then collected to verify PCR specificity, contamination and the absence of primer dimers. The melting curve was obtained by increasing the temperature from 60 to 95°C at 0.5°C/10 s.
The PCR efficiency of each primer pair (E) was evaluated by running a standard curve with serial dilution of cDNA. When E = 100%, the amount of PCR product can double in each cycle. Efficiencies and threshold cycle (CT) values were obtained by using the automated system software setting. The threshold cycle value was defined as the number of cycles required to reach a point in which the first fluorescent signal is recorded as statistically significant above background. In this study, the threshold fluorescence baseline was set manually at 100 relative fluorescence units (RFU).
The relative expression of a given gene was calculated using the 2-ΔΔCT method (Livak and Schmittgen, 2001). The gene expression levels were given as a differential of the expression levels in S. cerevisiae in mixed culture conditions versus expression levels of S. cerevisiae in pure culture. The results were normalized by using two reference genes PGK1 and TDH2 (Table 1). The data were analyzed using the comparative critical threshold (ΔΔCT) in which the amount of sample target RNA was adjusted to a control target RNA, where:
- Control: target RNA of S. cerevisiae from pure culture conditions
- Sample: target RNA of S. cerevisiae from mixed culture conditions
ΔCT = CT gene of interest -CT reference gene
ΔΔCT = ΔCT of sample - ΔCT of control
Relative expression level = 2-ΔΔCT
We considered that genes were significantly down- or over-expressed if their relative expression level was found to be at least twofolds lower or higher than the control conditions as previously described (Desroche et al., 2005).
Metabolite concentrations were subjected to one-way analysis of variance (ANOVA) followed by a Tukey’s (HSD) post hoc test (confidence interval 95%) to test for significance differences between the wines.
Yeast growth dynamics and sugar consumption during must fermentation were monitored for single and sequential cultures (Figure 2). The fermentation kinetics of the control S. cerevisiae PB2023 pure culture indicated that the maximal population was reached after 3 days (1.4 × 108 viable cells ml-1). This cell concentration was maintained until the end of fermentation (Figure 2A). S. cerevisiae completed the alcoholic fermentation in 8 days without remaining sugar. When the alcoholic fermentation was conducted with sequential culture of M. pulcherrima MCR-24 and S. cerevisiae PB2023 (inoculation ratio 10:1), the fermentation progressed to completion in 10 days (Figure 2B). The maximum population reached for S. cerevisiae was 3 × 108 viable cells ml-1 and 4 × 108 viable cells ml-1 for M. pulcherrima. The presence of M. pulcherrima did not affect the growth of the S. cerevisiae PB2023 strain. However, M. pulcherrima MCR 24 population dropped after the inoculation of S. cerevisiae PB2023 and no viable cells were detected after 8 days.
FIGURE 2. Growth kinetics and sugar consumption during mono-culture and sequential culture fermentations: (A) S. cerevisiae PB2023 (––), and (B) M. pulcherrima MCR 24 (–
–)/S. cerevisiae PB2023 (–
–). Glucose (
) and fructose (–
–). Data are representative of three independent trials.
The evolution of ethanol showed different kinetics in sequential and pure fermentations (Figure 3A). During the first 72 h of fermentation, as expected, the S. cerevisiae pure culture produced ethanol faster and in higher concentration than that produced by sequential culture, after which production was progressive and at a lower rate until the end of fermentation (10.58% v/v). M. pulcherrima/S. cerevisiae sequential culture showed a lower but regular trend for ethanol production until the end of fermentation (10.14% v/v). In both cases, the fermentation yields were slightly higher than usual [21 g l-1 sugars for 1% (v/v) ethanol instead 16.8 g l-1]. These data were probably linked to winemaking trials in small volumes (350 ml).
FIGURE 3. Evolution of metabolites production during alcoholic fermentation carried out by S. cerevisiae PB2023 (Sc) and M. pulcherrima MCR 24/S. cerevisiae PB2023 (Mp-Sc). (A) Ethanol production; (B) glycerol production; (C) acetic acid production. Day 0 corresponds to the day of inoculation with S. cerevisiae PB2023. Data are representative of three independent trials.
Saccharomyces cerevisiae pure culture produced a higher amount of glycerol (4.97 g l-1) in the first 4 days of fermentation compared to the sequential culture (3.52 g l-1). After day 4, glycerol was produced gradually until the end of fermentation (5.67 g l-1). Sequential culture exhibited lower concentrations of glycerol in the first 4 days of fermentation, but its concentration was higher at the end of the process (6.46 g l-1) (Figure 3B).
The acetic acid production kinetics of pure and sequential cultures are shown in Figure 3C. Pure culture of S. cerevisiae produced significantly higher amounts of acetic acid (0.35 ± 0.01 g l-1) compared to sequential culture (0.21 ± 0.03 g l-1). For S. cerevisiae pure culture, 57% of the final amount was produced during the first 3 days of fermentation. Interestingly, the presence of M. pulcherrima in culture together with S. cerevisiae led to a reduction of acetic acid production from the beginning of fermentation.
Previous data suggested that the metabolic pathways could be affected by interactions occurring between both yeasts during alcoholic fermentation. In this context, we studied the influence of M. pulcherrima MCR 24 growth on acetic acid and glycerol productions of S. cerevisiae evaluating Pdc, aldehyde dehydrogenase, Acs, and alcohol dehydrogenase gene expression during alcoholic fermentations. These enzymes are the key enzymes involved in the acetic acid production pathway. We have added the analysis of the expression of Gpd. Gene expression in S. cerevisiae was evaluated in sequential culture relative to the gene expression of S. cerevisiae in pure culture (control) (Figure 4). Time 0 corresponds to the day of inoculation of the S. cerevisiae PB2023 strain in the sequential culture.
FIGURE 4. Expression levels of GPD1 (A), PDC1 and PDC5 (B), ALD6, ALD4, and ACS2 (C), ADH1 and ADH2 (D), and CAT2 (E) in S. cerevisiae PB2023 at different stages of sequential culture fermentation. Data are representative of three independent trials. The gene expression levels were given as a differential of the expression levels in S. cerevisiae in sequential culture conditions versus expression levels of S. cerevisiae in pure culture.
Figure 4A shows the differential gene expression level of GPD1 in sequential culture condition. Dihydroxyacetone phosphate is converted to glycerol-3-phosphate, an intermediate for glycerol formation, by a Gpd enzyme encoded by the gene GPD1 (Figure 1). The GPD1 gene was over-expressed at 24 h after inoculation of S. cerevisiae, then the transcriptional level dropped and remained stable until the end of fermentation. This observation can be linked to the increase in the quantity of glycerol at the first 24 h of fermentation and then a similar production rate should be observed for S. cerevisiae in both fermentation conditions (pure and sequential culture) but it is hazardous to correlate this hypothesis with the analytical data shown Figure 3B. Indeed, M. pulcherrima MCR 24 produced glycerol (approximately 1 g l-1) before inoculation with S. cerevisiae and the levels measured after 48 h of fermentation may have resulted from the co-production of glycerol by both yeasts.
The differential of PDC1 and PDC5 gene expression levels during fermentation is shown in Figure 4B. The PDC1 gene was slightly over-expressed at 24 and 48 h after inoculation. After that, gene expression decreased gradually until the end of fermentation. However, PDC5 gene expression was not significantly affected by the sequential culture in the first 48 h but it was highly over-expressed at the 3rd day of fermentation (6.6-fold). Then, expression decreased gradually until the end of fermentation. Interestingly, we assume that Pdc encoding by both genes was not induced at the same time but alternately, confirming the hypothesis of their auto-regulation during alcoholic fermentation (Hohmann and Cederberg, 1990; Eberhardt et al., 1999). The alternate over-expression of the PDC1 and PDC5 genes was observed in the first 4 days of fermentation. After that, the transcriptional levels of both genes in sequential culture condition were identical to transcriptional levels of these genes in pure culture conditions. Furthermore, over-expression of these genes suggests that the sequential culture led to an increase in the production of acetaldehyde from pyruvate.
The differential expressions of genes directly involved in acetate production, i.e., ALD6, ALD4, ACS2, are presented in Figure 4C. The ALD6 gene was over-expressed in the first 3 days of fermentation, reaching its maximum level of expression on the 2nd day (7.4-fold; Figure 4B). However, the mitochondrial ALD4 gene was not over-expressed and remained stable during fermentation. This means that mitochondrial aldehyde dehydrogenase was not affected by the mixed culture condition, but cytosolic Ald6 activity could be privileged in order to regenerate the reduced co-enzyme NADPH (Figure 1). The ACS2 gene encoding Acs did not present over-expression in the mixed culture condition. The CAT2 gene encoding carnitine acetyltransferase was twofold lower expressed in sequential culture condition (Figure 4E).
The expression levels of genes ADH1 and ADH2 encoding alcohol dehydrogenase are shown in Figure 4D. No over-expression was observed in the ADH1 gene during fermentation. In contrast, the ADH2 gene was highly over-expressed 24 h after inoculation of S. cerevisiae (fourfold), which is involved in the conversion of ethanol into acetaldehyde (Figure 1). After 24 h, the ADH2 gene expression level dropped rapidly and a down regulation of ADH2 was observed from the 3rd to the 6th day of fermentation.
The early inoculation of M. pulcherrima MCR 24 did not compromise the growth of S. cerevisiae PB2023, preventing the risk of a sluggish or a stuck alcoholic fermentation. Moreover, the M. pulcherrima population dropped after the inoculation of S. cerevisiae and no viable cells were detected after 8 days (Figure 2B). Such an antagonistic effect has been reported previously (Jolly et al., 2003; Rodríguez et al., 2010; Comitini et al., 2011; Sadoudi et al., 2012). This result could not be linked to intolerance to ethanol concentration, since we previously demonstrated that the MCR 24 strain can produce approximately 10% v/v ethanol (Sadoudi et al., 2012). According to Nguyen and Panon (1998), the antagonistic effect could be attributed to killer toxins. Another explanation is the interaction occurring between both yeasts, mediated by the cell–cell contact mechanism (Nissen and Arneborg, 2003) or competition between yeasts for the nutrients available in the must. S. cerevisiae PB2023 grew faster than M. pulcherrima MCR 24 and thus it could impoverish the medium. Sequential inoculation did not affect the ethanol level in the wine despite the death of M. pulcherrima. On the other hand, it induced a significant increase in glycerol content and a decrease in acetic acid concentration (Figure 3). These data confirm the benefits of using M. pulcherrima prior the inoculation of the S. cerevisiae starter, in accordance with previous results (Bely et al., 2008; Comitini et al., 2011), but they do not explain the positive impact of M. pulcherrima on S. cerevisiae metabolism.
All previous analytical data suggest that the metabolic pathways could be rerouted by interactions occurring between both yeasts during alcoholic fermentation. During the latter, acetic acid is produced via the cytosolic PDH bypass. In aerobic conditions, the PDH complex leads to the formation of acetyl-CoA in the mitochondria by oxidative decarboxylation of pyruvate. However, in fermentative conditions, the conversion of pyruvate to acetyl-CoA can occur via an indirect route, involving Pdc (which is also a key enzyme in alcoholic fermentation), Ald and Acs. This bypass route is the source in the cytosolic compartment of acetyl-CoA, which is used for lipid synthesis and acetate which can be precursor of volatile esters.
The production of glycerol involves the reduction of dihydroxyacetone phosphate derived from the glycolytic degradation of sugar. The NAD+-dependent Gpd catalyzes the first step in glycerol production. This metabolism also permits the regeneration of reducing equivalents (NADH), more particularly at the beginning of S. cerevisiae growth in fermentative conditions.
The over-expression of PDC1 and PDC5 encoding two isoforms of Pdc and the ALD6 gene encoding cytosolic aldehyde dehydrogenase (Figures 4B,C) leads to the assumption of an over production of acetic acid by-product, which appears inconsistent with the analytical data which shows that acetate was reduced in mixed culture condition. One explanation could be due to the conversion of acetate into acetyl-CoA used in other metabolic pathways such as lipid synthesis or esterification related to the production of esters. Indeed, we previously observed higher levels of acetate esters in Sauvignon wine from a M. pulcherrima/S. cerevisiae sequential culture (Sadoudi et al., 2012). However, it is clear that acetyl-CoA was not transported into mitochondria since the CAT2 gene encoding carnitine acetyltransferase was under-expressed in sequential culture condition (Figure 4E).
The lower acetate production could not be due ethanol production since the ethanol contents are comparable under the two fermentation conditions. Another hypothesis that could explain our analytical data is that a part of dihydroxyacetone phosphate is used for glycerol production at the beginning of fermentation. Glycerol can be produced mostly at the beginning of fermentation in response to hyper osmotic conditions (high concentration in sugars). Moreover, anaerobic conditions require the production of endogenous electron acceptors and glycerol production can serve as a redox valve to eliminate excess reducing power in S. cerevisiae (Ansell et al., 1997). The M. pulcherrima strain MCR 24 may have depleted oxygen in the must during sequential culture, since it was inoculated 48 h before S. cerevisiae. Oxygen depletion (anaerobiotic conditions) could explain the modulation of glyceropyruvic fermentation and the orientation of metabolism to the PDH bypass, leading to the production of acetate and glycerol. These metabolism orientations are necessary to maintain the redox balance by regenerating NAD and NADH co-enzymes. Furthermore, increased glycerol formation requires an equimolar amount of cytoplasmic NADH. This requirement could be satisfied by a lower reduction of acetaldehyde to ethanol on the one hand and an increase in oxidation to acetate on the other (Blomberg and Adler, 1989; Nevoigt and Stahl, 1997). Therefore an increase in acetate production is usually accompanied by an increase in glycerol formation; however, a high levels of glycerol is not necessarily accompanied by high levels of acetic acid or acetaldehyde (Remize et al., 2000).
Independently of the expression of genes involved in acetate and glycerol production pathways, we hypothesized the possible consumption by M. pulcherrima MCR 24 of part of the acetate produced by S. cerevisiae in sequential culture fermentation. We performed a mono-culture with the M. pulcherrima MCR 24 strain using standardized grape juice supplemented with 1.5 g l-1 of acid acetic and observed the consumption of 0.57 g l-1 of the initial acetic acid during 8 days of fermentation (data not shown).
This work is the first attempt to investigate M. pulcherrima and S. cerevisiae yeast–yeast metabolic interaction, reflected by gene expression in the acetic acid and glycerol production pathway in S. cerevisiae during controlled sequential fermentation in winemaking. The environmental changes in must induced by the presence of M. pulcherrima induced the alteration of the entire acetic acid and glycerol metabolic pathway of S. cerevisiae.
Future accession to the M. pulcherrima genome may provide very interesting investigative leads on the nature of interactions occurring in sequential fermentations at the transcriptomic level.
MS designed the experiments, analyzed the data, and wrote the manuscript. SR analyzed the data and wrote the manuscript. VD analyzed the data. HA and RT-M supervised the study.
The authors declare that the research was conducted in the absence of any commercial or financial relationships that could be construed as a potential conflict of interest.
The authors would like to thank the SPINDAL-AEB group and the Regional Council of Burgundy for their financial support. We also thank Célia Matray for her contribution to the experiments.
Albergaria, H., and Arneborg, N. (2016). Dominance of Saccharomyces cerevisiae in alcoholic fermentation process. Appl. Microbiol. Biotechnol. 100, 2035–2046. doi: 10.1007/500253-015-7255-0
Alexandre, H., Costello, P. J., Remize, F., Guzzo, J., and Guilloux-Benatier, M. (2004). Saccharomyces cerevisiae-Oenococcus oeni interactions in wine: current knowledge and perspectives. Int. J. Food Microbiol. 93, 141–154. doi: 10.1016/j.ijfoodmicro.2003.10.013
Ansell, R., Granath, K., Hohman, S., Thevelein, J., and Adler, L. (1997). The two isoenzymes for yeast NAD+-dependent glycerol 3-phosphate dehydrogenase encoded by GPD1 and GPD2 have distinct role in osmoadaptation and redox regulation. EMBO J. 16, 2179–2187. doi: 10.1093/emboj/16.9.2179
Baburina, I., Gao, Y., Hu, Z., Jordan, F., Hohmann, S., and Furey, W. (1994). Substrate activation of brewers’ yeast pyruvate decarboxylase is abolished by mutation of cysteine 221 to serine. Biochemistry 33, 5630–5635. doi: 10.1021/bi00184a035
Barbosa, C., Falco, V., Mendes-Faia, A., and Mendes-Ferreira, A. (2009). Nitrogen addition influences formation of aroma compounds, volatile acidity and ethanol in nitrogen deficient media fermented by Saccharomyces cerevisiae wine strains. J. Biosci. Bioeng. 108, 99–104. doi: 10.1016/j.jbiosc.2009.02.017
Bely, M., Stoeckle, P., Masneuf-Pomarède, I., and Dubourdieu, D. (2008). Impact of mixed Torulaspora delbrueckii–Saccharomyces cerevisiae culture on high-sugar fermentation. Int. J. Food Microbiol. 122, 312–320. doi: 10.1016/j.ijfoodmicro.2007.12.023
Blomberg, A., and Adler, L. (1989). Roles of glycerol and glycerol-3-phosphate dehydrogenase (NAD+) in acquired osmotolerance of Saccharomyces cerevisiae. J. Bacteriol. 171, 1087–1092. doi: 10.1128/jb.171.2.1087-1092.1989
Ciani, M., Capece, A., Comitini, F., Canonico, L., Siesto, G., and Romano, P. (2016). Yeast interactions in inoculated wine fermentation. Front. Microbiol. 7:555. doi: 10.3389/fmicb.2016.00555
Comitini, F., Gobbi, M., Domizio, P., Romani, C., Lencioni, L., Mannazzu, I., et al. (2011). Selected non-Saccharomyces wine yeasts in controlled multistarter fermentations with Saccharomyces cerevisiae. Food Microbiol. 28, 873–882. doi: 10.1016/j.fm.2010.12.001
Delfini, C., and Costa, A. (1993). Effects of the grape must lees and insoluble materials on the alcoholic fermentation rate and the production of acetic acid, pyruvic acid, and acetaldehyde. Am. J. Enol. Vitic. 44, 86–92.
Desroche, N., Beltramo, C., and Guzzo, J. (2005). Determination of an internal control to apply reverse transcription quantitative PCR to study stress response in the lactic acid bacterium Oenococcus oeni. J. Microbiol. Methods 60, 325–333. doi: 10.1016/j.mimet.2004.10.010
Dickinson, F. M. (1996). The purification and some properties of the Mg(2+)-activated cytosolic aldehyde dehydrogenase of Saccharomyces cerevisiae. Biochem. J. 315, 393–399. doi: 10.1042/bj3150393
Eberhardt, I., Cederberg, H., Li, H., König, S., Jordan, F., and Hohmann, S. (1999). Autoregulation of yeast pyruvate decarboxylase gene expression requires the enzyme but not its catalytic activity. Eur. J. Biochem. 262, 191–201. doi: 10.1046/j.1432-1327.1999.00370.x
Erasmus, D., Cliff, M., and van Vuuren, H. (2004). Impact of yeast strain on the production of acetic acid, glycerol, and the sensory attributes of Icewine. Am. J. Enol. Vitic. 55, 371–378.
Eriksson, P., Alipour, H., Adler, L., and Blomberg, A. (2000). Rap1p-binding sites in the Saccharomyces cerevisiae GPD1 promoter are involved in its response to NaCl. J. Biol. Chem. 275, 29368–29376. doi: 10.1074/jbc.M001663200
Fleet, G. (2003). Yeast interactions and wine flavour. Int. J. Food Microbiol. 86, 11–22. doi: 10.1016/S0168-1605(03)00245-9
Gancedo, C., and Serrano, R. (1989). “Energy-yielding metabolism,” in The Yeasts, 2nd Edn, Vol. 3, eds A. Rose and J. Harrison (London: Academic Press), 205–259.
Hohmann, S. (1991). Characterization of PDC6, a third structural gene for pyruvate decarboxylase in Saccharomyces cerevisiae. J. Bacteriol. 173, 7963–7969. doi: 10.1128/jb.173.24.7963-7969.1991
Hohmann, S., and Cederberg, H. (1990). Auto-regulation may control the expression of yeast pyruvate decarboxylase structural genes PDC1 and PDC5. Eur. J. Biochem. 188, 615–621. doi: 10.1111/j.1432-1033.1990.tb15442.x
Jacobson, M. K., and Bernofsky, C. (1974). Mitochondrial acetaldehyde dehydrogenase from Saccharomyces cerevisiae. Biochim. Biophys. Acta Enzymol. 350, 277–291. doi: 10.1016/0005-2744(74)90502-6
Jolly, N., Pretorius, I., and Augustyn, O. (2003). The use of Candida pulcherrima in combination with Saccharomyces cerevisiae for the production of Chenin blanc wine. S. Afr. J. Enol. Vitic. 24, 63–69.
Lin, Y. (1975). Detection of wild yeasts in the brewery. Efficiency of differential media. J. Inst. Brew. 81, 410–417. doi: 10.1002/j.2050-0416.1975.tb06414.x
Liu, Y., Rousseaux, S., Tourdot-Maréchal, R., Sadoudi, M., Gougeon, R., Schmitt-Kopplin, P., et al. (2015). Wine microbiome: a dynamic world of microbial interactions. Crit. Rev. Food Sci. Nutr. 57, 856–873. doi: 10.1080/10408398.2014.983591
Livak, K., and Schmittgen, T. (2001). Analysis of relative gene expression data using real-time quantitative PCR and the 2(-Delta Delta C(T)) method. Methods 25, 402–408. doi: 10.1006/meth.2001.1262
Luo, Z., Walkey, J., Madilao, L., Measday, V., and van Vuuren, H. (2013). Functional improvement of Saccharomyces cerevisiae to reduce volatile acidity in wine. FEMS Yeast Res. 13, 485–494. doi: 10.1111/1567-1364.12053
Meaden, P. G., Dickinson, F. M., Mifsud, A., Tessier, W., Westwater, J., Bussey, H., et al. (1997). The ALD6 gene of Saccharomyces cerevisiae encodes a cytosolic, Mg2+-activated acetaldehyde dehydrogenase. Yeast 13, 1319–1327. doi: 10.1002/(SICI)1097-0061(199711)13:14<1319::AID-YEA183>3.0.CO;2-T
Milanovic, V., Ciani, M., Oro, L., and Comitini, F. (2012). Starmerella bombicola influences the metabolism of Saccharomyces cerevisiae at pyruvate decarboxylase and alcohol dehydrogenase level during mixed wine fermentation. Microb. Cell Fact. 11:18. doi: 10.1186/1475-2859-11-18
Moreira, N., Mendes, F., Hogg, T., and Vasconcelos, I. (2005). Alcohols, esters and heavy sulphur compounds production by pure and mixed cultures of apiculate wine yeasts. Int. J. Food Microbiol. 103, 285–294. doi: 10.1016/j.ijfoodmicro.2004.12.029
Navarro-Aviño, J. P., Prasad, R., Miralles, V. J., Benito, R. M., and Serrano, R. (1999). A proposal for nomenclature of aldehyde dehydrogenases in Saccharomyces cerevisiae and characterization of the stress-inducible ALD2 and ALD3 genes. Yeast 15, 829–842. doi: 10.1002/(SICI)1097-0061(199907)15:10A<829::AID-YEA423>3.0.CO;2-9
Nevoigt, E., and Stahl, U. (1997). Osmoregulation and glycerol metabolism in the yeast Saccharomyces cerevisiae. FEMS Microbiol. Rev. 21, 231–241. doi: 10.1111/j.1574-6976.1997.tb00352.x
Nguyen, H. V., and Panon, G. (1998). The yeast Metschnikowia pulcherrima has an inhibitory effect against various yeast species. Sci. Aliments 18, 515–526. doi: 10.1016/j.fm.2014.11.013
Nissen, P., and Arneborg, N. (2003). Characterization of early deaths of non-Saccharomyces yeasts in mixed cultures with Saccharomyces cerevisiae. Arch. Microbiol. 180, 257–263. doi: 10.1007/s00203-003-0585-9
Orlić, S., Arroyo-López, F., Huić-Babić, K., Lucilla, I., Querol, A., and Barrio, E. (2010). A comparative study of the wine fermentation performance of Saccharomyces paradoxus under different nitrogen concentrations and glucose/fructose ratios. J. Appl. Microbiol. 108, 73–80. doi: 10.1111/j.1365-2672.2009.04406.x
OIV (2010). Actualisation du Recueil des Methodes Internationales D’analyse des Boissons Spiritueuses D’origine Vitivinicoles. Paris: OIV.
Pérez-Torrado, R., Oliveira, B., Zemanèikovà, J., Sychrovà, H., and Querol, A. (2016). Alternative glycerol balance strategies among Saccharomyces species in response to winemaking stress. Front. Microbiol. 7:435. doi: 10.3389/fmicb.2016.00435
Pronk, J., Yde Steensma, H., and Van Dijken, J. (1996). Pyruvate metabolism in Saccharomyces cerevisiae. Yeast 12, 1607–1633. doi: 10.1002/(SICI)1097-0061(199612)12:16<1607::AID-YEA70>3.0.CO;2-4
Rantsiou, K., Dolci, P., Giacosa, S., Torchio, F., Tofalo, R., Torriani, S., et al. (2012). Candida zemplinina can reduce acetic acid produced by Saccharomyces cerevisiae in sweet wine fermentations. Appl. Environ. Microbiol. 78, 1987–1994. doi: 10.1128/AEM.06768-11
Rasmussen, J. E., Schultz, E., Snyder, R. E., Jones, R. S., and Smith, C. R. (1995). Acetic acid as a causative agent in producing stuck fermentations. Am. J. Enol. Vitic. 46, 278–280.
Remize, F., Sablayrolles, J. M., and Dequin, S. (2000). Re-assessment of the influence of yeast strain and environmental factors on glycerol production in wine. J. Appl. Microbiol. 88, 371–378. doi: 10.1046/j.1365-2672.2000.00964.x
Ribéreau-Gayon, P., Dubourdieu, D., Donéche, B., and Lonvaud, A. (eds). (2006). “The microbiology of wine and vinifications,” in Handbook of Enology, 2nd Edn, Vol. 1 (Hoboken, NJ: John Wiley & Sons).
Rodríguez, M., Lopes, C., Barbagelata, R., Barda, N., and Caballero, A. (2010). Influence of Candida pulcherrima Patagonian strain on alcoholic fermentation behaviour and wine aroma. In. J. Food Microbiol. 138, 19–25. doi: 10.1016/j.ijfoodmicro.2009.12.025
Sadoudi, M., Tourdot-Maréchal, R., Rousseaux, S., Steyer, D., Gallardo-Chacón, J.-J., Ballester, J., et al. (2012). Yeast–yeast interactions revealed by aromatic profile analysis of Sauvignon Blanc wine fermented by single or co-culture of non-Saccharomyces and Saccharomyces yeasts. Food Microbiol. 32, 243–253. doi: 10.1016/j.fm.2012.06.006
Saigal, D., Cunningham, S. J., Farrés, J., and Weiner, H. (1991). Molecular cloning of the mitochondrial aldehyde dehydrogenase gene of Saccharomyces cerevisiae by genetic complementation. J. Bacteriol. 173, 3199–3208. doi: 10.1128/jb.173.10.3199-3208.1991
Saint-Prix, F., Bönquist, L., and Dequin, S. (2004). Functional analysis of the ALD gene family of Saccharomyces cerevisiae during anaerobic growth on glucose: the NADP+-dependent Ald6p and Ald5p isoforms play a major role in acetate formation. Microbiology 150, 2209–2220. doi: 10.1099/mic.0.26999-0
Van den Berg, M., de Jong-Gubbels, P., Kortland, C., van Dijken, J., Pronk, J., and de Steensma, H. (1996). The two acetyl-coenzyme A synthetases of Saccharomyces cerevisiae differ with respect to kinetic properties and transcriptional regulation. J. Biol. Chem. 271, 28953–28959. doi: 10.1074/jbc.271.46.28953
Van den Berg, M., and Steensma, H. (1995). ACS2, a Saccharomyces cerevisiae gene encoding acetyl-coenzyme A synthetase, essential for growth on glucose. Eur. J. Biochem. 231, 704–713. doi: 10.1111/j.1432-1033.1995.tb20751.x
Vasserot, Y., Mornet, F., and Jeandet, P. (2010). Acetic acid removal by Saccharomyces cerevisiae during fermentation in oenological conditions. Metabolic consequences. Food Chem. 119, 1220–1223. doi: 10.1016/j.foodchem.2009.08.008
Vaudano, E., Noti, O., Costantini, A., and Garcia-Moruno, E. (2011). Identification of reference genes suitable for normalization of RT-qPCR expression data in Saccharomyces cerevisiae during alcoholic fermentation. Biotechnol. Lett. 33, 1593–1599. doi: 10.1007/s10529-011-0603-y
Walkey, J., Luo, Z., Madilao, L., and van Vuuren, H. (2012). The fermentation stress response protein Aaf1p/ Yml081Wp regulates acetate production in Saccharomyces cerevisiae. PLoS ONE 7:e51551. doi: 10.1371/journal.pone.0051551
Wang, X., Mann, C. J., Bai, Y., Ni, L., and Weiner, H. (1998). Molecular cloning, characterization, and potential roles of cytosolic and mitochondrial aldehyde dehydrogenases in ethanol metabolism in Saccharomyces cerevisiae. J. Bacteriol. 180, 822–830.
Keywords: sequential culture Metschnikowia pulcherrima/Saccharomyces cerevisiae, acetic acid, glycerol, alcoholic fermentation, quantitative RT-PCR
Citation: Sadoudi M, Rousseaux S, David V, Alexandre H and Tourdot-Maréchal R (2017) Metschnikowia pulcherrima Influences the Expression of Genes Involved in PDH Bypass and Glyceropyruvic Fermentation in Saccharomyces cerevisiae. Front. Microbiol. 8:1137. doi: 10.3389/fmicb.2017.01137
Received: 05 April 2017; Accepted: 06 June 2017;
Published: 28 June 2017.
Edited by:
Giovanna Suzzi, University of Teramo, ItalyReviewed by:
Patrizia Romano, University of Basilicata, ItalyCopyright © 2017 Sadoudi, Rousseaux, David, Alexandre and Tourdot-Maréchal. This is an open-access article distributed under the terms of the Creative Commons Attribution License (CC BY). The use, distribution or reproduction in other forums is permitted, provided the original author(s) or licensor are credited and that the original publication in this journal is cited, in accordance with accepted academic practice. No use, distribution or reproduction is permitted which does not comply with these terms.
*Correspondence: Raphaëlle Tourdot-Maréchal, dG91cmRvdEB1LWJvdXJnb2duZS5mcg==
Disclaimer: All claims expressed in this article are solely those of the authors and do not necessarily represent those of their affiliated organizations, or those of the publisher, the editors and the reviewers. Any product that may be evaluated in this article or claim that may be made by its manufacturer is not guaranteed or endorsed by the publisher.
Research integrity at Frontiers
Learn more about the work of our research integrity team to safeguard the quality of each article we publish.