- 1Department of Biochemistry and Molecular Biology, Federal University of Ceara, Fortaleza, Brazil
- 2Department of Biology, Federal University of Ceara, Fortaleza, Brazil
- 3School of Pharmacy, University of Fortaleza, Fortaleza, Brazil
- 4Department of Pathology and Legal Medicine, Federal University of Ceara, Fortaleza, Brazil
Candida species are opportunistic pathogens that infect immunocompromised and/or immunosuppressed patients, particularly in hospital facilities, that besides representing a significant threat to health increase the risk of mortality. Apart from echinocandins and triazoles, which are well tolerated, most of the antifungal drugs used for candidiasis treatment can cause side effects and lead to the development of resistant strains. A promising alternative to the conventional treatments is the use of plant proteins. M. oleifera Lam. is a plant with valuable medicinal properties, including antimicrobial activity. This work aimed to purify a chitin-binding protein from M. oleifera seeds and to evaluate its antifungal properties against Candida species. The purified protein, named Mo-CBP2, represented about 0.2% of the total seed protein and appeared as a single band on native PAGE. By mass spectrometry, Mo-CBP2 presented 13,309 Da. However, by SDS-PAGE, Mo-CBP2 migrated as a single band with an apparent molecular mass of 23,400 Da. Tricine-SDS-PAGE of Mo-CBP2 under reduced conditions revealed two protein bands with apparent molecular masses of 7,900 and 4,600 Da. Altogether, these results suggest that Mo-CBP2 exists in different oligomeric forms. Moreover, Mo-CBP2 is a basic glycoprotein (pI 10.9) with 4.1% (m/m) sugar and it did not display hemagglutinating and hemolytic activities upon rabbit and human erythrocytes. A comparative analysis of the sequence of triptic peptides from Mo-CBP2 in solution, after LC-ESI-MS/MS, revealed similarity with other M. oleifera proteins, as the 2S albumin Mo-CBP3 and flocculating proteins, and 2S albumins from different species. Mo-CBP2 possesses in vitro antifungal activity against Candida albicans, C. parapsilosis, C. krusei, and C. tropicalis, with MIC50 and MIC90 values ranging between 9.45–37.90 and 155.84–260.29 μM, respectively. In addition, Mo-CBP2 (18.90 μM) increased the cell membrane permeabilization and reactive oxygen species production in C. albicans and promoted degradation of circular plasmid DNA (pUC18) from Escherichia coli. The data presented in this study highlight the potential use of Mo-CBP2 as an anticandidal agent, based on its ability to inhibit Candida spp. growth with apparently low toxicity on mammalian cells.
Introduction
Candida species encompass a group of yeast that normally lives on the skin and mucous surfaces of healthy individuals (Mavor et al., 2005). Fortunately, just around 10% of approximately 200 Candida species identified to date are considered pathogenic to man. Most medically important Candida species include C. albicans, C. glabrata, C. parapsilosis, C. tropicalis, and C. krusei, which colonize the human body surface (Miceli et al., 2011). Candidiasis is the name given to the infections caused by the yeasts that belong to the genus Candida and avoidance of this disease constitutes a big challenge for modern medicine, with a significant impact on morbidity and overall mortality, especially among immunocompromised patients (Gil-Alonso et al., 2016). Incidentally, C. albicans is the principal causative agent of candidiasis (Pierce et al., 2015). The indiscriminate use of antibiotics, the incidence of diabetes mellitus type 1 and 2, and diseases or conditions which debilitate the immune system (chemotherapy, seropositive individuals, etc.) are within the factors associated with the increasing risk of developing candidiasis. In addition, patients subjected to surgery associated with the use of probes are also routinely affected by this infection (Schelenz, 2008; Achkar and Fries, 2010; Sanitá et al., 2014; Zhang et al., 2014; Alnuaimi et al., 2015).
Antifungal drugs currently available on the market for candidiasis treatment are often effective, but they can lead to the development of resistant strains, which could compromise the treatment efficiency. Drug resistance is related to three mechanisms: (1) decrease of intracellular drug concentration, (2) drug target alterations, and (3) metabolic bypasses (Sanglard, 2016). Furthermore, there are studies showing that these compounds may promote nephrotoxic (Niemirowicz et al., 2016), teratogenic (Dimopoulou et al., 2017), and cardiotoxic effects (Koch et al., 2015). Thus, it is very important to search for new compounds with antifungal activity, allowing the development of drugs and/or alternative treatments (Vandeputte et al., 2012; Salas et al., 2015). In this context, plants emerge as promising sources of bioactive compounds against Candida spp., since they have a large variety of primary (especially proteins) and secondary metabolites that are effective in defending plants against herbivores and/or pathogens (Wong et al., 2010). For instance, several plant proteins are known to possess harmful effects against Candida spp., which led to the suggestion of their biotechnological applications toward the candidiasis treatment. In this regard, chitin-binding proteins (CBP) can be highlighted due to their ability to interact with chitin, the main structural polysaccharide of fungal cell wall (Kanokwirron et al., 2008). The presence of chitin in fungal cell wall, where it performs relevant structural role for cell survival, and its absence in human cells, heighten chitin as a promising target for new antifungal drugs (Preechasuth et al., 2015; Campoy and Adrio, 2016). In literature, there are some reports on the antifungal effect of CBP against phytopathogenic fungi (Wang et al., 2012; Batista et al., 2014; Freitas C. D. et al., 2016), as well as against Candida spp. (Bertini et al., 2012; Gomes et al., 2012; Berthelot et al., 2016). Therefore, CBP are promising agents that have the potential to be used as antifungal compounds.
Moringa oleifera Lamarck (Moringaceae) is a fast-growing perennial species native to northeastern India that has a small to medium stature and is adapted to a variety of climates (Anwar et al., 2007). This species is well known in the world, particularly in tropical and subtropical regions, for its flocculation, nutritional, and pharmacological properties (Ndabigengesere et al., 1995; Santos et al., 2015; Freitas J. H. et al., 2016). Recently, our research group purified two CBP from M. oleifera seeds, eluted from a cation-exchange matrix with 0.5 M and 0.6 M NaCl, respectively, named Mo-CBP3 and Mo-CBP4 (Mo: M. oleifera; CBP: “Chitin-Binding Protein”), both of glycoprotein nature (Pereira et al., 2011; Gifoni et al., 2012). Mo-CBP3 displayed in vitro antifungal activity against Fusarium solani, F. oxysporum, Colletotrichum musae, and C. gloesporioides, phytopathogenic fungi to economically and nutritionally important crops (Batista et al., 2014; Freire et al., 2015), whereas Mo-CBP4 showed anti-inflammatory and antinociceptive activities in rats (Pereira et al., 2011). In our present investigation on M. oleifera seed proteins, we found an additional CBP eluted at a lower NaCl concentration (0.4 M) from the cation-exchange matrix, named Mo-CBP2, which displayed antifungal activity against Candida species. In this study, a purification protocol of Mo-CBP2 and its biochemical properties are presented. In addition, to evaluate the antifungal mode of action of Mo-CBP2, its ability to increase the cell membrane permeability and to induce endogenous production of reactive oxygen species in C. albicans, used as a model, were analyzed, as well as its DNAse activity. In view of the potential biotechnological applications of Mo-CBP2 as an antifungal agent, the hemolytic activity on red blood cells (cytotoxicity) was also investigated.
Materials and Methods
Biological Material and Chemical Reagents
Moringa oleifera seeds were collected from trees at the Campus do Pici of the Federal University of Ceará – UFC (Ceará, Brazil) under authorization (number: 47766) of the Chico Mendes Institute for Conservation of Biodiversity – ICMBio. A voucher specimen (N EAC34591) was deposited in the Prisco Bezerra Herbarium (UFC). The yeasts C. albicans (ATCC 10231), C. parapsilosis (ATCC 22019), C. krusei (ATCC 6258), and C. tropicalis (clinical isolate) were obtained from the culture collection of the Laboratory of Emergent and Reemergent Pathogens – LAPERE, Department of Pathology and Legal Medicine, UFC. Rabbit blood was obtained from animals of colonies maintained at the UFC. Human blood samples (ABO system) were obtained from healthy donors in the blood bank HEMOCE (Hemotherapy Center of Ceará). Experimental protocols were approved by the Ethics Committee of UFC, Brazil (protocol number: 77/2016).
Molecular mass markers, chromatographic matrices, IPG buffer, and immobilized pH gradient gel strips were obtained from GE Healthcare Life Sciences (New York, NY, United States). All other chemicals were purchased from Sigma-Aldrich Co. (St. Louis, MO, United States).
Protein Quantification
Quantification of soluble protein was determined following the method described by Bradford (1976), using bovine serum albumin (BSA) as a protein standard. Absorbance at 280 nm was also used to detect the presence of protein in the chromatographic eluates.
Purification of a Chitin-binding Protein from M. oleifera Seeds (Mo-CBP2)
Purification of Mo-CBP2 followed the procedure described by Gifoni et al. (2012), with modifications. Defatted seed flour was extracted with 0.05 M Tris-HCl buffer, pH 8.0, containing 0.15 M NaCl (1:10, m/v), for 3 h at 4°C under constant stirring. The resulting suspension was filtered and centrifuged at 15,000 × g, 4°C, 30 min. The supernatant was exhaustively dialyzed against distilled water at 4°C and centrifuged again under the same conditions. An aliquot of the supernatant (albumin fraction; 1.0 g) was loaded on a chitin column (3.5 cm × 20.0 cm) previously equilibrated with the above buffer. The M. oleifera chitin-binding proteins (Mo-CBPs) were eluted with 0.05 M acetic acid, pooled, dialyzed against distilled water at 4°C, and lyophilized. Mo-CBPs (400 mg) were dissolved in 20 mL of 0.05 M sodium acetate buffer, pH 5.2, and applied to a CM-SepharoseTM Fast Flow column, pre-equilibrated with the same buffer. The adsorbed proteins were recovered by stepwise elution with increasing NaCl concentrations. Mo-CBP2 (Mo: M. oleifera; CBP: Chitin-Binding Protein) was dialyzed against distilled water at 4°C and subject to further analysis.
Characterization of Mo-CBP2
Polyacrylamide Gel Electrophoresis Analysis
The purity of Mo-CBP2 was observed after polyacrylamide gel electrophoresis (PAGE) in the absence (native-PAGE) and presence of sodium dodecyl sulfate (SDS-PAGE). Native-PAGE was performed according to Reisfeld et al. (1962). Mo-CBP2 (5.0 μg) was loaded on 15% (m/v) polyacrylamide gel (10.0 cm × 8.0 cm) prepared in 1.5 M potassium acetate, pH 4.3. SDS-PAGE was performed as previously described (Laemmli, 1970). Mo-CBP2 samples (5.0 μg) were prepared in the sample buffer, in the presence or absence of 8% (v/v) 2-mercaptoetanol (2-ME) or 0.1 M dithiothreitol (DTT), and boiled at 98°C for 4 h. The samples were loaded on 15% (m/v) polyacrylamide gel prepared in 0.025 M Tris-HCl buffer, pH 8.9, containing 1% (m/v) SDS. The protein bands were detected by staining with 0.025% (m/v) Coomassie Brilliant Blue R-250 in methanol, acetic acid, and water (1.0:3.5:8.0, v/v/v). Molecular mass standards (GE Healthcare) were also loaded on the gel. The electrophoretic profile of reduced Mo-CBP2 was analyzed by tricine-SDS-PAGE (Schägger and von Jagow, 1987). The samples (10.0 μg) were incubated in 0.1 M DTT for 4 h at 98°C and alkylated with 0.2 M iodoacetamide (IAA) for 45 min at room temperature, followed by additional treatment with 4% (v/v) 2-ME for 10 min at 98°C. The samples were loaded and the electrophoretic run was carried out. Proteins were stained as above. Low molecular mass markers (Sigma–Aldrich) were also used.
Mass Spectrometric Analysis
Electrospray ionization mass spectrometry (ESI-MS) was performed using a Synapt G1 HDMS mass spectrometer (Waters) which was coupled to a nanoUPLC system. The intact mass of Mo-CBP2 (1 mg/mL in 0.1% [v/v] formic acid) was fractioned by reverse-phase chromatography using a gradient from 3 to 70% (v/v) acetonitrile with 0.1% (v/v) formic acid on HSS T3 C18 column (1.8 μm, 75 μm × 20 mm). Data were collected using MassLynx 4.1 (Waters). The acquired MS data were processed using a maximum-entropy technique (MaxEnt) to obtain a deconvoluted spectrum (Ferrige et al., 1991).
Isoelectric Point Determination
Isoelectric focusing (IEF) of Mo-CBP2 was performed according to Görg et al. (2000). Mo-CBP2 (150.0 μg) was solubilized in 250 μL of rehydration buffer, incubated with an immobilized pH gradient gel strip (11 cm, pH 6.0–11.0), and left standing for 12 h at room temperature. IEF was performed on a Multiphor II Electrophoresis system (GE Healthcare) using a stepwise protocol: 200 V/60 min, 500 V/90 min, 5,000 V/2.5 h, and 10,000 V/5.5 h. After IEF, the gel strip was brought in contact with the equilibration buffer containing 2.5% (m/v) DTT, for 15 min, followed by incubation with 7.5% (m/v) IAA, for 15 min, and submitted to SDS-PAGE (15%) on a vertical system (16.0 cm × 18.0 cm, Hoefer SE 600 Ruby, GE Healthcare). Molecular mass markers were run in the same gel. Proteins were stained with colloidal Coomassie (Candiano et al., 2004) and visualized using the Image Master TM 2D Platinum v.7.0 (GE Healthcare).
Carbohydrate Determination
The presence of covalently bound carbohydrate in the Mo-CBP2 structure was evaluated by periodic acid-Schiff staining (Zacharius et al., 1969). Briefly, Mo-CBP2 (15.0 μg) electrophoresis was performed as described above, the gel was fixed in 7.5% (v/v) acetic acid for 2 h and immersed in 0.2% (v/v) periodic acid for 45 min at 4°C, followed by staining in the periodic acid-Schiff reagent for 45 min at 4°C. Next, the gel was submerged in a 0.5% (m/v) potassium metabisulfite solution prepared in 0.05 M HCl. Fetuin (30.0 μg) was used as positive control. The neutral sugar content of Mo-CBP2 was estimated spectrophotometrically by the phenol-sulfuric acid method with reference to D-glucose (DuBois et al., 1956).
Amino Acid Sequencing
The N-terminal sequence analysis was done using an Automated Protein Sequencer (Shimadzu PPSQ-23A) via Edman degradation. The phenylthiohydantoin amino acid derivatives were detected at 269 nm after separation on an RP-HPLC C18 column (4.6 mm × 2.5 mm) under isocratic conditions, according to the supplier’s instructions. To obtain internal protein sequences, Mo-CBP2 (50.0 μg) was digested with 1.0 μg trypsin (Promega®) at 37°C for 16 h. The tryptic peptides were fractioned by reverse-phase chromatography using a gradient from 3 to 40% (v/v) acetonitrile with 0.1% (v/v) formic acid on HSS T3 C18 column (1.8 μm, 75 μm × 20 mm). After elution, data-dependent analysis (DDA) of peptides was performed using a Synapt HDMS mass spectrometer (nanoESI-Qq-oaTOF; Waters), where the three top peaks were subjected to MS/MS. The data were processed using ProteinLynx Global Server v.2.4 software (PLGS) and subjected to database search using the Mascot search engine (Perkins et al., 1999). MS/MS ion searches were performed against the NCBI non-redundant database (last accessed on January 14, 2017) using a significance threshold of p < 0.05. Searches for similar proteins in public sequence databases were performed using BLASTp (Altschul et al., 1990).
Evaluation of Hemagglutinating Activity
Hemagglutinating activity was assessed using untreated or trypsin-treated rabbit and human (ABO system) erythrocytes (Lis and Sharon, 1972). A serial 2-fold dilution (100 μL) of Mo-CBP2 (5.0 mg/mL in 0.15 M NaCl) in a 96-well plate was mixed with 100 μL of a 4% (v/v) erythrocyte suspension in 0.15 M NaCl from 1 h up to 24 h at 37°C (Raja et al., 2011). Hemagglutination was checked in comparison to a blank in which Mo-CBP2 had been omitted. The minimal protein concentration after serial dilution still promoting visible agglutination with naked eyes was taken to calculate the hemagglutinating activity.
Evaluation of Antifungal Activity and Mode of Action of Mo-CBP2
In Vitro Antifungal Activity Test
The antifungal activity of Mo-CBP2 was tested against the yeasts C. albicans, C. krusei, C. parapsilosis, and C. tropicalis. The susceptibility of Candida spp. to Mo-CBP2 was evaluated using the broth microdilution method, as described by the Clinical and Laboratory Standards Institute (Clinical and Laboratory Standards Institute [CLSI], 2012), with modifications. Potato dextrose broth (PDB) medium and nystatin (EMS) were used instead of Roswell Park Memorial Institute (RPMI) medium and amphotericin B. Inocula (final concentration of 0.5 – 2.5 × 103 CFU/mL) were prepared from 1-day-old cultures grown on potato dextrose agar (PDA) at 35°C in PDB medium. Mo-CBP2, Mo-CBP3 (Gifoni et al., 2012), Mo-CBP4 (Pereira et al., 2011) (0.1–1,346.46 μM), and nystatin (0.085–1,067.04 μM) were prepared in ultrapure water and sterilized using a 0.22 μm membrane filter. Aliquots (100 μL) of each yeast suspension (0.5–2.5 × 103 CFU/mL) were incubated in 96-well flat plates with 50 μL of Mo-CBP2 and additional 50 μL of PDB medium (4-fold concentrated). Plates were incubated at 37°C for 24 h and the yeast growth was monitored at 620 nm using an automated microplate reader (Epoch, BioTek). Nystatin and 0.15 M NaCl were used as positive and negative controls, respectively. Mo-CBP3 and Mo-CBP4 were used as reference proteins, as they are also CBP from M. oleifera seeds. Yeast-free control was also included. The Minimum Inhibitory Concentrations (MIC50 and MIC90) were defined as the lowest protein or nystatin concentration capable of inhibiting 50% and 90% fungal growth, respectively.
Evaluation of Cell Membrane Integrity of C. albicans Cells after Mo-CBP2 Treatment
Plasma membrane permeabilization was measured by propidium iodide uptake (Regente et al., 2014). C. albicans cells (0.5–2.5 × 103 CFU/mL) were cultured in the presence of 18.9 μM Mo-CBP2 or 11.11 μM nystatin (positive control), whose concentrations corresponding to their respective MIC50 calculated in this study, or 0.15 M NaCl (negative control), for 24 h at 37°C. The Mo-CBP2 and nystatin-treated cell suspensions (100 μL) were incubated with 0.001 M propidium iodide in 96-well microplates for 30 min at 30°C, under constant and moderate agitation. Next, the cells were observed under a fluorescence microscope (Olympus System BX 60; excitation wavelength, 400–500 nm; emission wavelength, 600–700 nm).
Evaluation of Reactive Oxygen Species (ROS) Production by C. albicans Cells after Mo-CBP2 Treatment
This was done according to Thordal-Christensen et al. (1997), with modifications (Batista et al., 2014). Aliquots (100 μL) of the cell suspensions (0.5–2.5 × 103 CFU/mL) previously treated with Mo-CBP2, nystatin or NaCl, as described above, were incubated with 100 μL of 3,3′-diaminobenzidine (DAB, 1.0 mg/mL in H2O) for 1 h at 30°C. Next, the cells were observed under a light microscope (Olympus System Microscope BX 60).
Assessment of DNase Activity of Mo-CBP2
The effect of Mo-CBP2 on DNA degradation was assessed as previously described (Tomar et al., 2014a), using the pUC18 plasmid of E. coli. The plasmid (500.0 ng) was incubated with Mo-CBP2 (500.0 ng) in 0.05 M Tris-HCl buffer, pH 7.4, in a total reaction volume of 20 μL, for 1 h at 37°C. Recombinant DNase I (2 units, RNase-free, Roche), BSA (500.0 ng), and 0.05 M Tris-HCl buffer, pH 7.4, all equally incubated with the plasmid, were used as controls. The samples were loaded on 1% (m/v) agarose gel (10.0 cm × 10.0 cm), prepared in the TAE buffer (0.05 M Tris-HCl, 0.02 M sodium acetate, and 0.001 M EDTA), mounted on a horizontal system (MultiSUB Midi10, Cleaver Scientific), and run at 6 V/cm. The DNA bands were visualized under UV light after staining the gel with 0.5 μg/mL ethidium bromide solution for 30 min.
Evaluation of Hemolytic Activity of Mo-CBP2
The hemolytic assay was performed using rabbit and human (ABO system) erythrocytes collected in heparinized tubes (Choi and Lee, 2014). Erythrocytes were separated from plasma by centrifugation (3,000 × g, 10 min, 25°C) and washed three times with 0.15 M NaCl. An aliquot (100 μL) of a 4% (v/v) suspension was incubated with an equal volume of Mo-CBP2 (1.9–1000 μg/mL), both prepared in 0.15 M NaCl, for 1 h at 37°C. After incubation, the mixtures were centrifuged at 3,000 × g for 10 min at 25°C and aliquots of the supernatants transferred to Eppendorf tubes. Absorbance readings were taken at 414 nm (spectrophotometer Novaspec II, Pharmacia) to monitor the release of hemoglobin. Triton X-100 (0.1%, v/v) and 0.15 M NaCl were used as positive and negative controls, respectively. The hemolysis index was calculated using the following equation: Hemolysis (%) = ([Aprotein – ANaCl]/[ATriton – ANaCl]) × 100, where A means absorbance at 414 nm.
Statistical Analysis
Data were obtained from three independent experiments, each one done in triplicate. The results are expressed as the mean ± standard deviation (SD). Tukey’s test was used to compare means and the results were considered to be significant at p < 0.05. GraphPad Prism 5.02 software was used for statistical analysis.
Results
Purification of Mo-CBP2
Mo-CBP2 was purified by a combination of albumin separation from the crude extract and two chromatographic steps. The crude extract obtained from M. oleifera seeds presented 205.41 mg protein/g of defatted seed. Exhaustive dialysis of the crude extract against distilled water produced the albumin fraction that concentrated approximately 55% of the soluble proteins. Chromatography of albumin on a chitin column produced a chitin-binding protein peak (Mo-CBPs) (Supplementary Figure 1A), which represented 23.2% of the seed extract soluble proteins. Mo-CBPs chromatographed on a CM-SepharoseTM Fast Flow column emerged as a through fraction and three adsorbed protein peaks (Supplementary Figure 1B). Mo-CBP2 was recovered after elution of the adsorbed proteins from the column with 0.4 M NaCl included in 0.05 M sodium acetate buffer, pH 5.2, yielding 0.32 mg protein/g of the defatted flour, or 0.2% of the soluble proteins of the seed crude extract (Table 1).
Molecular and Physicochemical Properties of Mo-CBP2
Purity, Molecular Mass, Isoelectric Point, and Carbohydrate Content
A single polypeptide band appeared after native electrophoresis of Mo-CBP2 (Supplementary Figure 2, inset), suggesting that the purification procedure employed yielded a contaminant-free, homogenous protein. ESI-MS of the intact protein revealed a major peak at 13,309 Da (Supplementary Figure 2). However, SDS-PAGE of Mo-CBP2 under non-reducing conditions showed a 23,400 Da protein band (Supplementary Figure 3, lane 1). Mo-CBP2 treated with 8% (v/v) 2-ME or 0.1 M DTT presented the same prominent band of 23,400 Da and additional faint bands (Supplementary Figure 3, lanes 4 and 7). In tricine-SDS-PAGE, Mo-CBP2 treated with 0.1 M DTT, alkylated with 0.2 M IAA followed by treatment with 4% (v/v) 2-ME revealed the generation of two protein bands with apparent molecular masses of 7,900 and 4,600 Da (Supplementary Figure 4, lanes 2, 4, and 6). Two-dimensional electrophoresis analysis of Mo-CBP2 revealed the presence of a protein spot of 22,300 Da apparent molecular mass and isoelectric point (pI) of 10.9 (data not shown). Moreover, Mo-CBP2 was stained by periodic acid-Schiff’s reagent on SDS-PAGE, suggesting it is a glycoprotein (Supplementary Figure 5), confirmed by quantitative analysis that showed 4.1% (m/m) covalently linked neutral carbohydrates to the Mo-CBP2 structure.
Amino Acid Sequence
Edman degradation of Mo-CBP2 did not give any sequence, suggesting that its N-terminal residue was blocked. However, after tryptic hydrolysis of Mo-CBP2 followed by LC-ESI-MS/MS analysis of the resulting fragments, six peptides were identified (Table 2) and their sequences deposited in the Swiss-Prot database (access number: C0HKC5). By aligning the sequences of these fragments against the non-redundant protein sequence database of NCBI, limited to Brassicales for the first three peptides and Viridiplantae for the last three peptides, these Mo-CBP2 sequences were more closely related (75–100% identity) to other proteins purified from M. oleifera, as 2S albumin precursors (Mo-CBP3 isoforms: AHG99684.1; AHG99683.1; AHG99682.1), a flocculating protein (prf| | 2111235A), and MO 2.1 (AAB34890.1). Similarities (63–83%) were also found with 2S albumins of other plant species (Capparis masaikai [BAA12204.1, P80351.1], Bertholletia excelsa [ACI70207.1], Ziziphus jujuba [XP_015899022.1]).
Assessment of Hemagglutination Activity of Mo-CBP2
Mo-CBP2 did not agglutinate rabbit and human erythrocytes, even at 5.0 mg/mL. These results did not differ regardless of whether the erythrocytes were trypsin-treated or untreated.
Antifungal Activity and Mode of Action of Mo-CBP2
Mo-CBP2 inhibited the development of C. krusei, C. albicans, C. tropicalis, and C. parapsilosis with MIC50 values varying from 9.45 to 37.90 μM, whereas Mo-CBP3 and Mo-CBP4, also CBP, had MIC50 between 261.67 and 310.06 μM. To cause 90% fungal growth inhibition, the MIC90 for Mo-CBP2, Mo-CBP3, and Mo-CBP4 were in the ranges of 155.84–260.29 μM, 560.32–600.23 μM, and 564.89–598.10 μM, respectively. MIC50 for nystatin varied from 11.11 to 22.23 μM and MIC90 from 55.55 to 133.38 μM (Table 3).

TABLE 3. Antifungal activitya of chitin-binding proteins from M. oleifera seeds and nystatin against Candida species.
Treatment of C. albicans, used as a representative model, with 11.11 μM nystatin or 18.90 μM Mo-CBP2 for 24 h altered the cell membrane permeability as revealed by propidium iodide uptake (Figure 1).
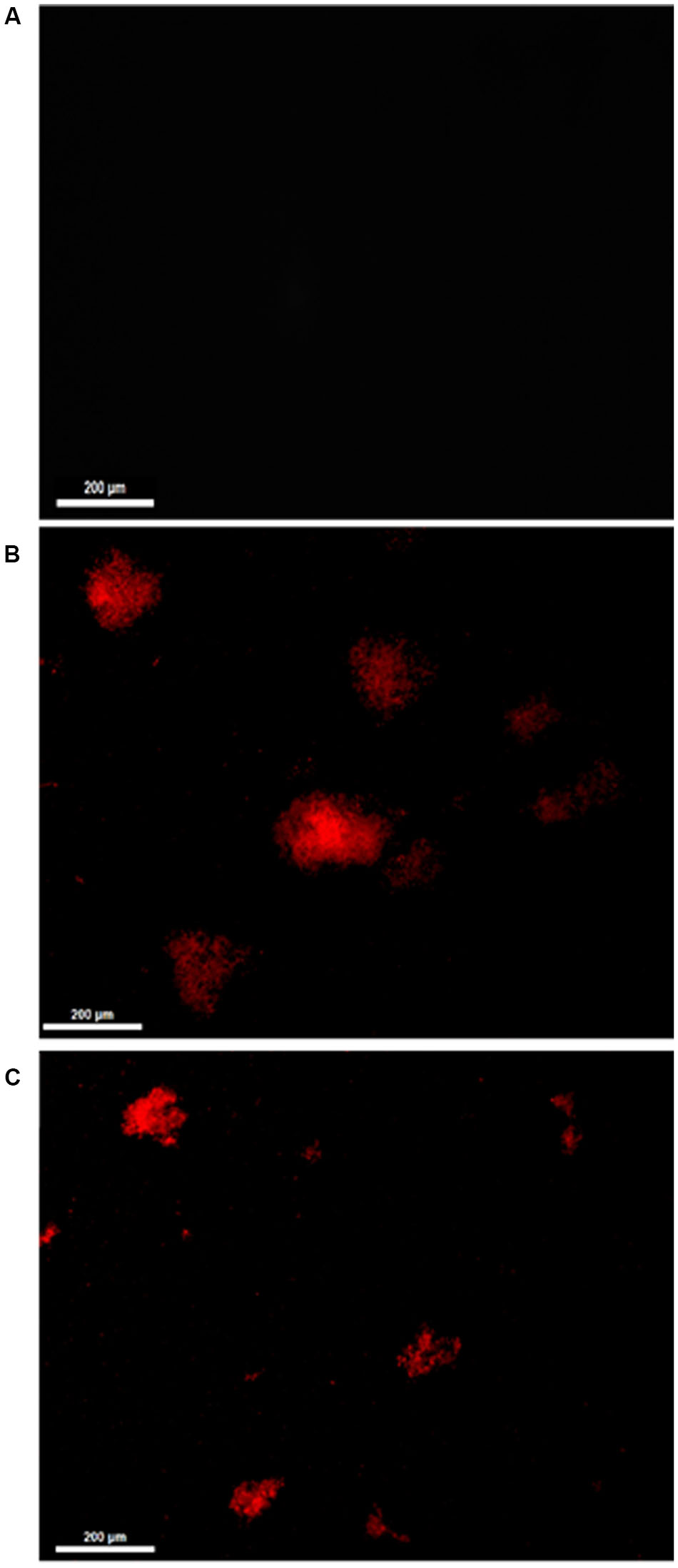
FIGURE 1. Fluorescence microscopy of C. albicans cells treated with 0.15 M NaCl (A), 11.11 μM nystatin (B), or 18.90 μM Mo-CBP2 (C), followed by incubation with 0.001 M propidium iodide. Bars = 200 μm.
In addition, ROS overproduction was observed, recognized as internal dark staining, after incubation of C. albicans cells with 11.11 μM nystatin or 18.90 μM Mo-CBP2 (Figure 2). Similar to the recombinant DNase I, Mo-CBP2 (500.0 ng) also promoted DNA degradation of the E. coli plasmid (pUC18), whereas both BSA and 0.05 M Tris-HCl buffer, pH 7.4, were inactive (Figure 3).
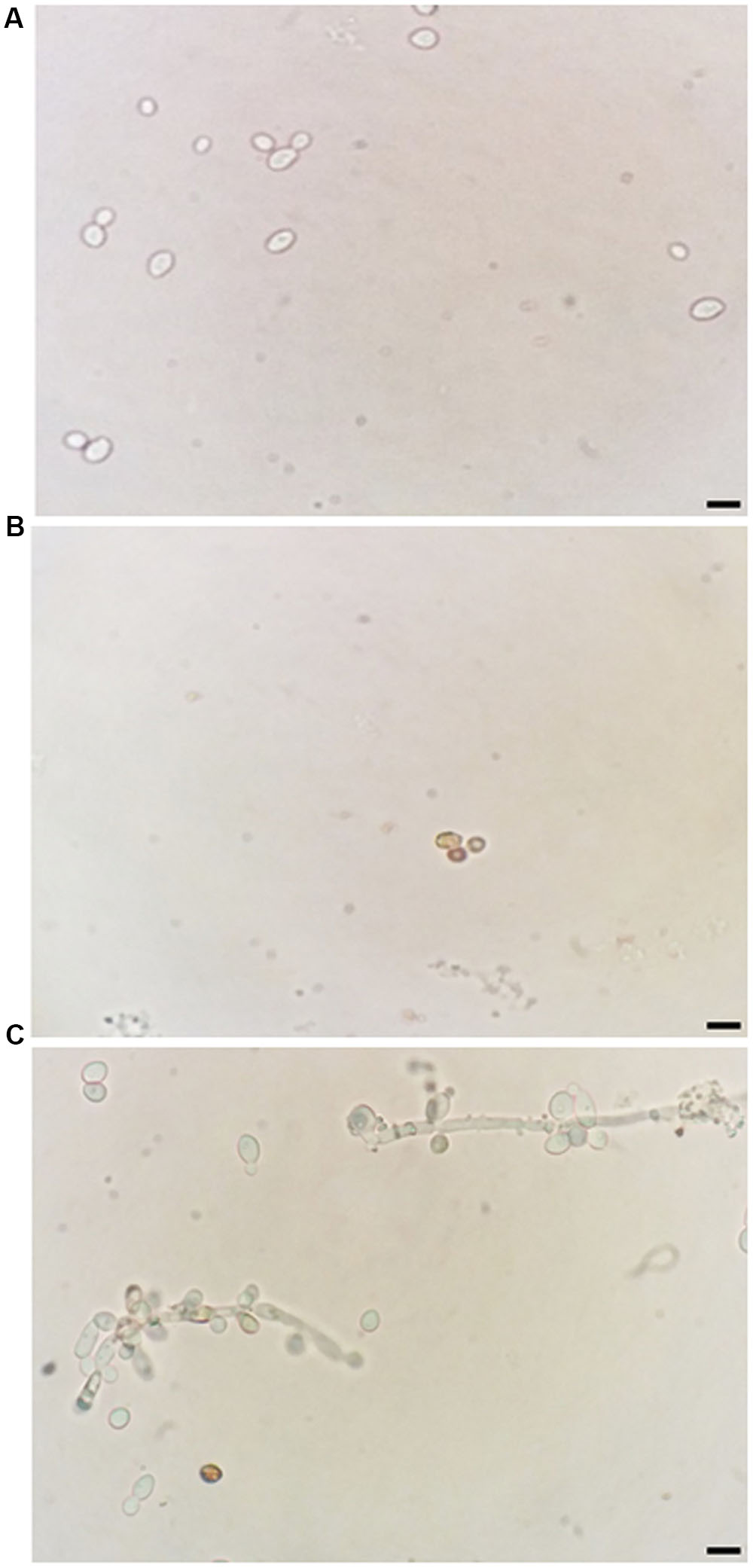
FIGURE 2. Induction of ROS generation in C. albicans. Light micrography of cells previously treated with 0.15 M NaCl (A), 11.11 μM nystatin (B), or 18.90 μM Mo-CBP2 for 24 h at 37°C (C), followed by incubation with DAB. The presence of ROS was observed by dark staining (reddish-brown) reaction inside cells. Bars = 10 μm.
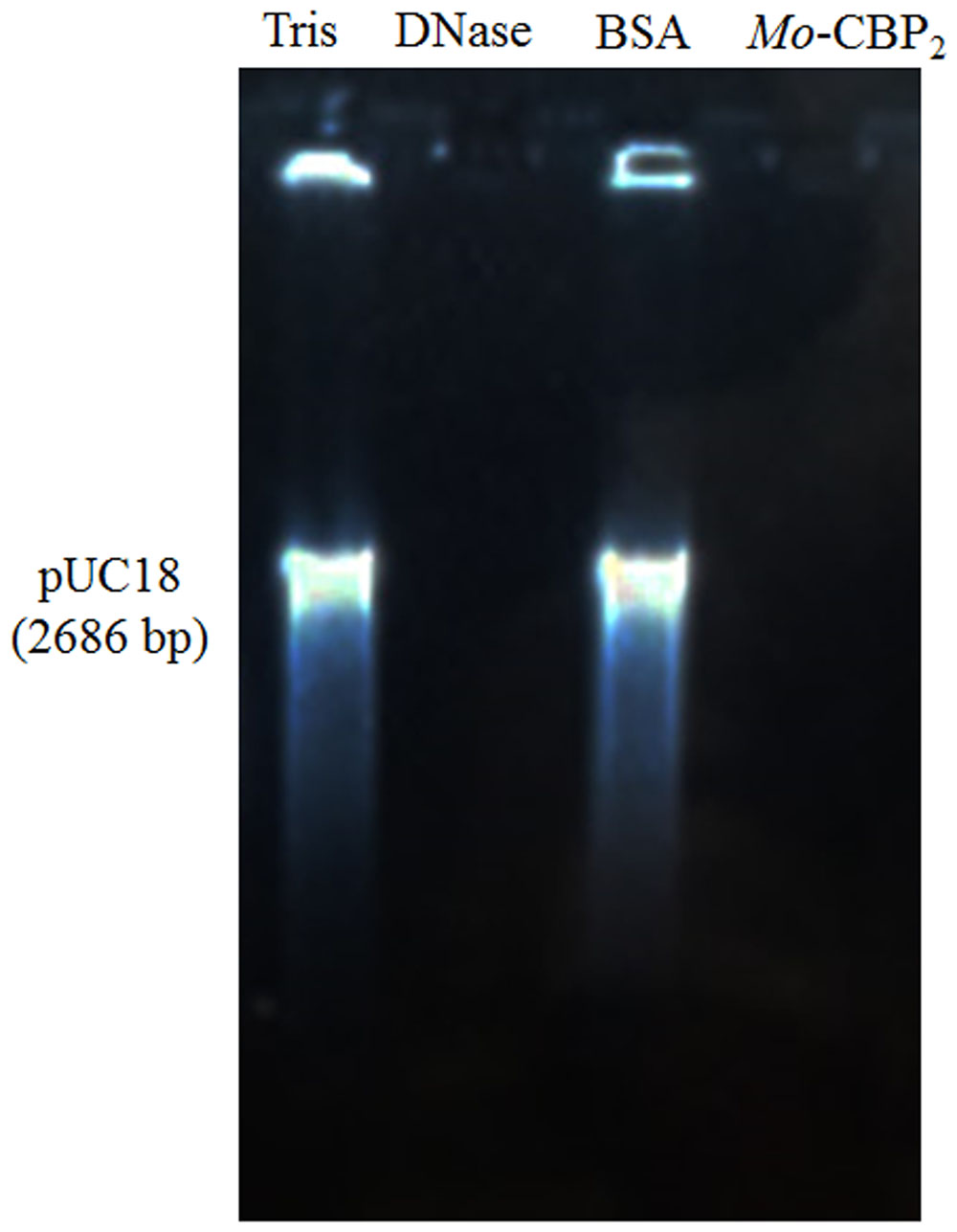
FIGURE 3. DNase activity of Mo-CBP2. The pUC18 plasmid (500.0 ng) of E. coli was incubated with Mo-CBP2 (500.0 ng); BSA (500.0 ng) and 0.05 M Tris-HCl buffer, pH 7.4 (negative controls); and the recombinant DNase I (2 units, positive control) for 1 h and loaded in 1% (m/mL) agarose gel electrophoresis. Gel was stained with ethidium bromide and observed under UV light.
Assessment of the Cytotoxicity Effect of Mo-CBP2
Bovine serum albumin and Mo-CBP2 did not exhibited any hemolytic activity on rabbit and human erythrocytes in all concentrations analyzed, contrary to the positive control, Triton X-100, which caused strong hemolysis on the tested erythrocytes (data not shown).
Discussion
The World Health Organization (WHO) has long been concerned with the rational use of antibiotics and the emergence of super-resistant microorganisms. World Health Organization [WHO] (2015) launched the first “World Antibiotics Awareness Week” with the theme “Antibiotics: Handle with care,” from which a list with recommendations to prevent antibiotic resistance was produced. In consonance with this concern, several research groups have been searching for new antimicrobial agents to efficiently overcome the development of microbial resistance, devoid of side effects, and also not expensive. It has long been known that plants are rich sources of new compounds with potential for the treatment of various diseases, including infectious diseases. Studies exploring the mechanism of action and structure-activity aspects of such natural compounds are important toward the discovery and development of novel antimicrobial agents (Hayashi et al., 2013). In connection with this global antimicrobial resistance dilemma the current study reported on the purification and characterization of Mo-CBP2, and evaluated its anticandidal effect.
Mo-CBP2 analyzed by PAGE under native condition appeared as a single band protein. After tricine-SDS-PAGE under reducing conditions, Mo-CBP2 dissociated in two protein bands with apparent molecular masses of 7,900 and 4,600 Da, values that summed up (12,500 Da) result in a molecular mass near to that determined by mass spectrometry analysis (13,309 Da). However, Mo-CBP2 analyzed by SDS-PAGE under non-reducing conditions migrated as a 23,400 Da protein. These findings suggest the formation of dimer species of Mo-CBP2, with each monomer composed of the 7,900 and 4,600 Da subunits probably linked by dissulfide bond. The other CBP previously purified by our research group, Mo-CBP3 (Gifoni et al., 2012) and Mo-CBP4 (Pereira et al., 2011), also behaved as oligomeric proteins. Actually, several other similarities between Mo-CBP2 and Mo-CBP3 and Mo-CBP4 exist, like the molecular mass of the intact proteins (∼13,300, 12,200, and 11,800 Da, respectively) and subunits (∼4,600/7,900, 4,100/8,100, and 3,900/8,400 Da, respectively) (Pereira, 2014; Freire et al., 2015), which suggest that they are part of the same family of CBP. Similar oligomeric behavior was also observed for other proteins from M. oleifera seeds. Indeed, both the hemagglutinin MoL and the flocculating lectin cMoL presented molecular profiles similar to Mo-CBP2, appearing in dimer or trimer conformations (Katre et al., 2008; Luz et al., 2013). The in silico analysis of the recombinant protein MO 2.1 showed that the dimer form was the most stable structural arrangement (Pavankumar et al., 2014). Thus, it is plausible to suggest that the M. oleifera proteins aggregate into oligomers to attain a stable and lower energy structural state.
Mo-CBP2 is a glycoprotein as are other CBP from M. oleifera purified by our research group (Pereira et al., 2011; Gifoni et al., 2012). However, a difference in relation to Mo-CBP3 and Mo-CBP4 is that the carbohydrate content was approximately two times higher in Mo-CBP2. Another similar characteristic to Mo-CBP3 and Mo-CBP4 is that Mo-CBP2 does not present any hemagglutinating activity upon human or rabbit erythrocytes, contrary to Mol and cMol proteins (Katre et al., 2008; Luz et al., 2013). Therefore, Mo-CBP2 is a merolectin as it apparently possesses a unique carbohydrate binding site (Peumans and Van Damme, 1995) which binds to N-acetyl-D-glucosamine and its derived polysaccharide chitin, thus incapable of establishing bridges between two of more blood cells toward agglutinating them (Gifoni et al., 2012)
The MS/MS analysis done with Mo-CBP2 after tryptic digestion, as an attempt to disclose its primary structure, allowed identification of 6 peptide fragments that together comprise 55 amino acid residues, or approximately 45% of the total amino acid residues of the studied protein. Four cysteine (C) residues were present, which might form inter or intrachain disulphide bonds. Glutamine (Q) was the most abundant amino acid residue (about 31%), which could be related to dimer formation tendency of Mo-CBP2. It was previously reported that glutamine-rich regions may cause aggregation by formation of β-pleated sheets held together by hydrogen bonds (Perutz et al., 1994; Michelitsch and Weissman, 2000; Broin et al., 2002). In addition, the presence of glutamine at the N-terminus of Mo-CBP2 could also explain the failure to identify the protein sequence by Automated Edman degradation. Indeed, cyclization of N-terminal glutamine to pyroglutamate leads to a blocked chain, and this event has been described for several 2S albumins (Moreno et al., 2005). Particularly noteworthy is that among the identified amino acid residues of Mo-CBP2, 14.5% corresponded to the positively charged arginine (R) and histidine (H), whereas negatively charged, polar uncharged, and non-polar amino acid residues correspond to 1.8, 45.5, and 38.2%, respectively. This is compatible with the cationic property of Mo-CBP2 (pI = 10.9) and its adsorption to the cation exchange chromatography column equilibrated at pH 5.2, during the purification process.
Searches against the non-redundant protein sequence database of NCBI using BLASTp revealed that Mo-CBP2 is closely related to Mo-CBP3 and flocculating proteins from M. oleifera, and 2S albumins from different plant sources. Sequence alignment of the six identified Mo-CBP2 peptides with the sequences of four Mo-CBP3 isoforms showed 63 to 100% of similarity, except the QPDFQR peptide that aligned exclusively with the isoform 2 (Mo-CBP3-2), with 83% of similarity. Among the Mo-CBP3 isoforms, the mean percentage sequence identity was found to Mo-CBP3-3 (NCBI accession number AHG99684.1), the most abundant of them in the seeds of M. oleifera (Freire et al., 2015). With the M. oleifera genome recently available (Tian et al., 2015), we tried to localize the nucleotide sequence that corresponds to Mo-CBP2 using the peptides generated after its tryptic digestion. However, as the primary structures of all Mo-CBPs proteins are very similar, it was not possible to predict the Mo-CBP2 sequence. For instance, it was reported that the flocculent peptides MOCP 2.1 and MOCP 2.2 are distinguished by a single amino acid residue (Shebek et al., 2015). Similar situation might occur with Mo-CBP2 and other M. oleifera proteins. Besides of being a CBP, Mo-CBP3 is also a member of the 2S albumin family, on the base of their structural similarities (Freire et al., 2015). Therefore, it is reasonable to suggest that Mo-CBP2 is also a member of the 2S albumin family. The similarities between the Mo-CBP2 peptide sequences and 2S albumins from C. masaikai (63–83%), B. excelsa (73%), and Z. jujuba (63%) reinforce this hypothesis, although these plant species belong to other botany families different from Moringaceae. 2S albumins are reserve proteins found in mono- or dicotyledonous plants (Youle and Huang, 1981), which apparently are also related to plant defense and some have antifungal activity (Agizzio et al., 2006; Moreno and Clemente, 2008; Cândido et al., 2011), including against Candida species (Ribeiro et al., 2012).
The antimicrobial properties of M. oleifera have been previously reported. For instance, the aqueous extracts from seeds and other plant parts exhibited antimicrobial activity (Saadabi and Zaid, 2011; Onsare et al., 2013), particularly for Gram positive and Gram negative bacteria rather than for Candida spp. Recently, flavonoids extracted from M. oleifera seed coat were successfully tested as they exhibited antibiofilm potential against Staphylococcus aureus (Gram positive), Pseudomonas aeruginosa (Gram negative) and the yeast C. albicans (Onsare and Arora, 2015). Moreover, organic extracts from several M. oleifera parts also showed antibacterial activity (Brilhante et al., 2015).
Although the antibacterial and anti-Candida activities of M. oleifera are well known, to the best of our knowledge, this is the first report of a protein from M. oleifera seeds with anticandidal activity. Interestingly, all chitin binding proteins (Mo-CBP2, Mo-CBP3, and Mo-CBP4) evaluated in this work exhibited inhibitory activity through Candida spp. Comparative analyses between anticandidal effects displayed by Mo-CBPs evidenced Mo-CBP2 as the most potent protein amongst them. Mo-CBP2 inhibited C. albicans, C. parapsilosis, C. krusei, and C. tropicalis growth with MIC50 much lower (9.45–37.90 μM) than the other proteins (261.67–310.06 μM). However, much higher concentrations of Mo-CBPs (155.84–600.23 μM) were needed to inhibit 90% of fungal growth. C. krusei cells were the most sensitive to Mo-CBP2 (MIC50 9.45 μM and MIC90 155.84 μM). This is an important finding since C. krusei is a potentially multi-drug resistant pathogenic yeast due to its intrinsic resistance to fluconazole and tendency to develop reduced echinocandin susceptibility during prolonged therapy and under selection pressure (Scorzoni et al., 2013; Tavernier et al., 2015). The antifungal mode of action of Mo-CBP2 is probably linked to its ability to disrupt the cell membrane integrity of C. albicans, as propidium iodide, which is membrane impermeable, was taken up by cells exposed to Mo-CBP2, and interacted with nucleic acids as revealed by the appearance of red fluorescence (Wang et al., 2015). Increasing permeability of cell membrane can result from depolarization, disruption of lipid domain organization, pore formation, and unbalance of intracellular electrochemical gradients, leading to loss of membrane functions and cell death (Lee and Lee, 2015; Lee et al., 2015). We hypothesize that the increased permeability of C. albicans cell membrane by Mo-CBP2 exposure might be due to interaction of this protein with chitin, which is one of the major components of fungal cell walls. As suggested for some antifungal peptides, chitin binding ability could help Mo-CBP2 targets fungal cells efficiently and kill them by disruption of the plasma membrane integrity that increases permeabilization, or by forming pores directly (Bahar and Ren, 2013). Mo-CBP2 could also gain access to the cell membrane by crossing the cell wall during the exponential growth phase of the yeast cells, when they exhibit increased porosity allowing the passage of compounds up to 70,000 Da (Klis et al., 2014). Once there, Mo-CBP2, as a basic protein rich in positively charged amino acid residues, could establish electrostatic interaction with the negatively charged cell membrane leading to its disarrangement and cell lysis. Alternatively, Mo-CBP2 could form transient pores through which it could gain access to the cell interior and enters into contact with intracellular targets (Li et al., 2012; Taniguchi et al., 2013; Choi and Lee, 2014). Regardless whether Mo-CBP2 gained access or not to the Candida cell interior it promoted ROS generation, which are toxic to microorganisms (Mello et al., 2011; Wang et al., 2015). Like for other various antifungal proteins the mechanism of ROS generation after Mo-CBP2 treatment of Candida cells is yet unknown. Nevertheless, ROS are natural compounds produced during the cell metabolism and play important roles in cell signaling and homeostasis, and high levels of ROS generated under environmental stress can result in significant damage to cell structures (Wang et al., 2015). Additionally, excessive ROS damages proteins, lipids, and DNA (Deavall et al., 2012) that besides increasing cell membrane permeability can lead ultimately to cell death. For instance, nystatin, an antifungal, disrupted the cell membrane integrity and induced increased ROS levels in C. albicans cells.
Moreover, in the case of internalization into the cell interior, Mo-CBP2 could interact directly with DNA and exert DNase activity as it broken down in vitro the E. coli plasmid pUC18, like the commercial recombinant DNase. This finding corroborates with previous studies on the DNase activity of other CBP (Guevara-Morato et al., 2010; Menezes et al., 2014) and 2S albumins from different plant sources (Odintsova et al., 2010; Tomar et al., 2014a,b). Thus, besides its low molecular mass, positive net charge, ability to disrupt cell membrane, and DNase activity, Mo-CBP2 may also exhibit anticandidal effect by interacting with the genetic material of C. albicans cells, leading to its degradation.
In addition to broad spectrum of action on pathogenic microorganisms, low toxicity is a desirable feature of new candidates as antifungal molecules. Hemolytic effect is often considered when antimicrobial safety of new compounds is tested as drugs for human and animal use (Kannan et al., 2013; Sellami et al., 2013; Christoffersen et al., 2015). Although Mo-CBP2 is toxic to Candida spp., this protein did not cause hemolysis in human and rabbit erythrocytes, even in the highest concentration tested (1000 μg/mL). Despite the nystatin effectiveness as antifungal agent and prescription in superficial candidiasis treatment, its clinical use is limited due to its toxicity to human erythrocytes (Bassi and Kaur, 2015). Melittin, other potent antimicrobial peptide against Candida spp., causes hemolysis in human blood cells even in low concentrations, which limits its use in antifungal therapy (Lee and Lee, 2015; Lee et al., 2015). Thus, discover of potent antimicrobial agents with high selectivity and reduced or no toxicity to mammalian cells is still a challenge for the scientific community.
In summary, the data presented in this study highlight the potential use of Mo-CBP2 as an anticandidal agent, based on its ability to inhibit Candida spp. growth with apparently low toxicity on mammalian cells.
Author Contributions
Conceived and designed the study and experiments: JN, MP, JO, LR-B, BR, TG, AM-M, RB, and IV. Performed the experiments: JN, MP, LR-B, TL, HC, JF, ML, and IV. Analyzed the data: JN, MP, JO, LR-B, HC, DS, BR, TG, ML, IV. Contributed reagents/materials/analysis tools: JO, DS, BR, TG, AM-M, RB, and IV. Wrote the paper: JN, MP, JO, LR-B, and IV. All authors reviewed the manuscript.
Funding
This study was supported by the National Council for Scientific and Technological Development (CNPq) and the Coordination of Improvement of Higher Education (CAPES, Toxinology Project), Brazil.
Conflict of Interest Statement
The authors declare that the research was conducted in the absence of any commercial or financial relationships that could be construed as a potential conflict of interest.
Acknowledgments
The authors gratefully acknowledge the support from the Laboratory of Emergent and Reemergent Pathogens – LAPERE, Department of Pathology and Legal Medicine, UFC (Fortaleza, CE, Brazil).
Supplementary Material
The Supplementary Material for this article can be found online at: http://journal.frontiersin.org/article/10.3389/fmicb.2017.00980/full#supplementary-material
References
Achkar, J. M., and Fries, B. C. (2010). Candida infections of the genitourinary tract. Clin. Microbiol. Rev. 23, 253–273. doi: 10.1128/CMR.00076-09
Agizzio, A. P., da Cunha, M., Carvalho, A. O., Oliveira, M. A., Ribeiro, S. F. F., and Gomes, V. M. (2006). The antifungal properties of a 2S albumin-homologous protein from passion fruit seeds involve plasma membrane permeabilization and ultrastructural alterations in yeast cells. Plant Sci. 171, 515–522. doi: 10.1016/j.plantsci.2006.06.001
Alnuaimi, A. D., Wiesenfeld, D., O’Brien-Simpson, N. M., Reynolds, E. C., and McCullough, M. J. (2015). Oral Candida colonization in oral cancer patients and its relationship with traditional risk factors of oral cancer: a matched case-control study. Oral Oncol. 51, 139–145. doi: 10.1016/j.oraloncology.2014.11.008
Altschul, S. F., Gish, W., Miller, W., Myers, E. W., and Lipman, D. J. (1990). Basic local alignment search tool. J. Mol. Biol. 215, 403–410. doi: 10.1016/S0022-2836(05)80360-2
Anwar, F., Latif, S., Ashraf, M., and Gilani, A. H. (2007). Moringa oleifera: a food plant with multiple medicinal uses. Phytother. Res. 21, 17–25. doi: 10.1002/ptr.2023
Bahar, A. A., and Ren, D. (2013). Antimicrobial peptides. Pharmaceuticals 6, 1543–1575. doi: 10.3390/ph6121543
Bassi, P., and Kaur, G. (2015). Bioadhesive vaginal drug delivery of nystatin using a derivatized polymer: development and characterization. Eur. J. Pharm. Biopharm. 96, 173–184. doi: 10.1016/j.ejpb.2015.07.018
Batista, A. B., Oliveira, J. T. A., Gifoni, J. M., Pereira, M. L., Almeida, M. G. G., Gomes, V. M., et al. (2014). New insights into the structure and mode of action of Mo-CBP3, an antifungal chitin-binding protein of Moringa oleifera seeds. PLoS ONE 9:e111427. doi: 10.1371/journal.pone.0111427
Berthelot, K., Peruch, F., and Lecomte, S. (2016). Highlights on Hevea brasiliensis (pro)hevein proteins. Biochimie 127, 258–270. doi: 10.1016/j.biochi.2016.06.006
Bertini, L., Proietti, S., Aleandri, M. P., Mondello, F., Sandini, S., Caporale, C., et al. (2012). Modular structure of HEL protein from Arabidopsis reveals new potential functions for PR-4 proteins. Biol. Chem. 393, 1533–1546. doi: 10.1515/hsz-2012-0
Bradford, M. M. (1976). A rapid and sensitive method for the quantitation of microgram quantities of protein utilizing the principle of protein-dye binding. Anal. Biochem. 72, 248–254. doi: 10.1016/0003-2697(76)90527-3
Brilhante, R. S. N., Sales, J. A., Sampaio, C. M. S., Barbosa, F. G., Paiva, M. A. N., Guedes, G. M. M., et al. (2015). Vibrio spp. from Macrobrachium amazonicum prawn farming are inhibited by Moringa oleifera extracts. Asian Pac. J. Trop. Med. 11, 919–922. doi: 10.1016/j.apjtm.2015.10.012
Broin, M., Santaella, C., Cuine, S., Kokou, K., Peltier, G., and Jöet, T. (2002). Flocculent activity of a recombinant protein from Moringa oleifera Lam. seeds. Appl. Microbiol. Biotechnol. 60, 114–119. doi: 10.1007/s00253-002-1106-5
Campoy, S., and Adrio, J. L. (2016). Antifungals. Biochem. Pharmacol. 133, 86–96. doi: 10.1016/j.bcp.2016.11.019
Candiano, G., Bruschi, M., Musante, L., Santucci, L., Ghiggeri, G. M., Carnemolla, B., et al. (2004). Blue silver: a very sensitive colloidal Coomassie G-250 staining for proteome analysis. Electrophoresis 25, 1327–1333. doi: 10.1002/elps.200305844
Cândido, E. S., Pinto, M. F. S., Pelegrini, P. B., Lima, T. B., Silva, O. N., Pogue, R., et al. (2011). Plant storage proteins with antimicrobial activity: novel insights into plant defense mechanisms. FASEB J. 25, 3290–3305. doi: 10.1096/fj.11-184291
Choi, H., and Lee, D. G. (2014). Antifungal activity and pore-forming mechanism of astacidin 1 against Candida albicans. Biochimie 105, 58–63. doi: 10.1016/j.biochi.2014.06.014
Christoffersen, H. F., Hansen, S. K., Vad, B. S., Nielsen, E. H., Nielsen, J. T., Vosegaard, T., et al. (2015). The natural, peptaibolic peptide SPF-5506-A4 adopts a β-bend spiral structure, shows low hemolytic activity and targets membranes through formation of large pores. Biochim. Biophys. Acta 1854, 882–889. doi: 10.1016/j.bbapap.2015.03.003
Clinical and Laboratory Standards Institute [CLSI] (2012). Reference Method for Broth Dilution Antifungal Susceptibility Testing of Yeasts; 4th Informational Supplement. CLSI Document M27-S4. Wayne, PA: Clinical and Laboratory Standards Institute.
Deavall, D. G., Martin, E. A., Horner, J. M., and Roberts, R. (2012). Drug-induced oxidative stress and toxicity. J. Toxicol. 2012:645460. doi: 10.1155/2012/645460
Dimopoulou, M., Verhoef, A., Pennings, J. L. A., Van Ravenzwaay, B., Rietjens, I. M. C. M., and Piersma, H. A. (2017). Embryotoxic and pharmacologic potency ranking of six azoles in the rat whole embryo culture by morphological and transcriptomic analysis. Toxicol. Appl. Pharm. 322, 15–26. doi: 10.1016/j.taap.2017.03.001
DuBois, M., Gilles, K. A., Hamilton, J. K., Rebers, P. A., and Smith, F. (1956). Colorimetric method for determination of sugars and related substances. Anal. Chem. 28, 350–356. doi: 10.1021/ac60111a017
Ferrige, A. G., Seddon, M. J., and Jarvis, S. (1991). Maximum entropy deconvolution in electrospray mass spectrometry. Rapid Commun. Mass Spectrom. 5, 374–379. doi: 10.1002/rcm.1290050810
Freire, J. E., Vasconcelos, I. M., Moreno, F. B., Batista, A. B., Lobo, M. D., Pereira, M. L., et al. (2015). Mo-CBP3, an antifungal chitin-binding protein from Moringa oleifera seeds, is a member of the 2S albumin family. PLoS ONE 10:e0119871. doi: 10.1371/journal.pone.0119871
Freitas, C. D., Viana, C. A., Vasconcelos, I. M., Moreno, F. B. B., Lima-Filho, J. V., Oliveira, H. D., et al. (2016). First insights into the diversity and functional properties of chitinases of the latex of Calotropis procera. Plant Physiol. Biochem. 108, 361–371. doi: 10.1016/j.j.plaphy.2016.07.028
Freitas, J. H., de Santana, K. V., Nascimento, A. C., de Paiva, S. C., de Moura, M. C., Coelho, L. C., et al. (2016). Evaluation of using aluminum sulfate and water-soluble Moringa oleifera seed lectin to reduce turbidity and toxicity of polluted stream water. Chemosphere 163, 133–141. doi: 10.1016/j.chemosphere.2016.08.019
Gifoni, J. M., Oliveira, J. T. A., Oliveira, H. D., Batista, A. B., Pereira, M. L., Gomes, A. S., et al. (2012). A novel chitin-binding protein from Moringa oleifera seed with potential for plant disease control. Biopolymers 98, 406–415. doi: 10.1002/bip.22068
Gil-Alonso, S., Jauregizar, N., Ortega, I., Eraso, E., Suárez, E., and Quindós, G. (2016). In vitro pharmacodynamic modelling of anidulafungin against Candida spp. Int. J. Antimicrob. Agents 47, 178–183. doi: 10.1016/j.ijantimicag.2015.12.011
Gomes, S. F., Procópio, T. F., Napoleão, T. H., Coelho, L. C. B. B., and Paiva, P. M. G. (2012). Antimicrobial lectin from Schinus terebinthifolius leaf. J. Appl. Microbiol. 114, 672–679. doi: 10.1111/jam.12086
Görg, A., Obermaier, C., Boguth, G., Harder, A., Scheibe, B., Wildgruber, R., et al. (2000). The current state of two-dimensional electrophoresis with immobilized pH gradients. Electrophoresis 21, 1037–1053. doi: 10.1002/(SICI)1522-2683(20000401)21:6<1037::AID-ELPS1037>3.0.CO;2-V
Guevara-Morato, M. A., Lacoba, M. G., García-Luque, I., and Serra, M. T. (2010). Characterization of a pathogenesis-related protein 4 (PR-4) induced in Capsicum chinense L3 plants with dual RNase and DNase activities. J. Exp. Bot. 61, 3259–3271. doi: 10.1093/jxb/erq148
Hayashi, M. A., Bizerra, F. C., and Da Silva, P. I. Jr. (2013). Antimicrobial compounds from natural sources. Front. Microbiol. 4:195. doi: 10.3389/fmicb.2013.00195
Kannan, R. R. R., Arumugam, R., Iyapparaj, P., Thangaradjou, T., and Anantharaman, P. (2013). In vitro antibacterial, cytotoxicity and haemolytic activities and phytochemical analysis of seagrasses from the Gulf of Mannar. South India. Food Chem. 136, 1484–1489. doi: 10.1016/j.foodchem.2012.09.006
Kanokwirron, K., Teanpaisan, R., Wititsuwannakul, D., Hooper, A. B., and Wititsuwannakul, R. (2008). Antimicrobial activity of a protein purified from the latex of Hevea brasiliensis on oral microorganisms. Mycoses 51, 301–307. doi: 10.1111/j.1439-0507.2008.01490.x
Katre, U. V., Suresh, C. G., Khan, M. I., and Gaikwad, S. M. (2008). Structure-activity relationship of a hemagglutinin from Moringa oleifera seeds. Int. J. Biol. Macromol. 42, 203–207. doi: 10.1016/j.ijbiomac.2007.10.024
Klis, F. M., de Koster, C. G., and Brul, S. (2014). Cell wall-related bionumbers and bioestimates of Saccharomyces cerevisiae and Candida albicans. Eukaryot. Cell 13, 2–9. doi: 10.1128/EC.00250-13
Koch, C., Uhle, F., Wolff, M., Arens, C., Schulte, A., Li, L., et al. (2015). Cardiac effects of echinocandins after central venous administration in adult rats. Antimicrob. Agents Chemother. 59, 1612–1619. doi: 10.1128/AAC.04446-14
Laemmli, U. K. (1970). Cleavage of structural proteins during the assembly of the head of bacteriophage T4. Nature 227, 680–685. doi: 10.1038/227680a0
Lee, H., Hwang, J.-S., Lee, J., Kim, J. I., and Lee, D. G. (2015). Scolopendin 2, a cationic antimicrobial peptide from centipede, and its membrane-active mechanism. Biochim. Biophys. Acta 1848, 634–642. doi: 10.1016/j.bbamem.2014.11.016
Lee, W., and Lee, D. G. (2015). Fungicidal mechanisms of the antimicrobial peptide Bac8c. Biochim. Biophys. Acta 1848, 673–679. doi: 10.1016/j.bbamem.2014.11.024
Li, Y., Xiang, Q., Zhang, Q., Huang, Y., and Su, Z. (2012). Overview on the recent study of antimicrobial peptides: origins, functions, relative mechanisms and application. Peptides 37, 207–215. doi: 10.1016/j.peptides.2012.07.001
Lis, H., and Sharon, N. (1972). Soy bean (Glycine max) agglutinin. Methods Enzymol. 28, 360–365. doi: 10.1016/0076-6879(72)28046-6
Luz, L. A., Silva, M. C. C., Ferreira, R. S., Santana, L. A., Silva-Lucca, R. A., Mentele, R., et al. (2013). Structural characterization of coagulant Moringa oleifera lectin and its effect on hemostatic parameters. Int. J. Biol. Macromol. 58, 31–36. doi: 10.1016/j.ijbiomac.2013.03.044
Mavor, A. L., Thewes, S., and Hube, B. (2005). Systemic fungal infections caused by Candida species: epidemiology, infection process and virulence attributes. Curr. Drug Targets 6, 863–874. doi: 10.2174/138945005774912735
Mello, E. O., Ribeiro, S. F. F., Carvalho, A. O., Santos, I. S., da Cunha, M., Santa-Catarina, C., et al. (2011). Antifungal activity of PvD1 defensin involves plasma membrane permeabilization, inhibition of medium acidification, and induction of ROS in fungi cells. Curr. Microbiol. 62, 1209–1217. doi: 10.1007/s00284-010-9847-3
Menezes, S. P., Silva, E. M. A., Lima, E. M., Sousa, A. O., Andrade, B. S., Lemos, L. S. L., et al. (2014). The pathogenesis-related protein PR-4b from Theobroma cacao presents RNase activity, Ca2+ and Mg2+ dependent-DNase activity and antifungal action on Moniliophthora perniciosa. BMC Plant Biol. 14:161. doi: 10.1186/1471-2229-14-161
Miceli, M. H., Díaz, J. A., and Lee, S. A. (2011). Emerging opportunistic yeast infections. Lancet Infect. Dis. 11, 142–151. doi: 10.1016/S1473-3099(10)70218-8
Michelitsch, M. D., and Weissman, J. S. (2000). A census of glutamine/asparagine-rich regions: implications for their conserved function and the prediction of novel prions. Proc. Natl. Acad. Sci. U.S.A. 97, 11910–11915. doi: 10.1073/pnas.97.22.11910
Moreno, F. J., and Clemente, A. (2008). 2S albumin storage proteins: what makes them food allergens? Open Biochem. J. 2, 16–28. doi: 10.2174/1874091X00802010016
Moreno, F. J., Maldonado, B. M., Wellner, N., and Mills, E. N. C. (2005). Thermostability and in vitro digestibility of a purified major allergen 2S albumin (Ses i 1) from white sesame seeds (Sesamum indicum L.). Biochim. Biophys. Acta 1752, 142–153. doi: 10.1016/j.bbapap.2005.07.022
Ndabigengesere, A., Narasiah, K. S., and Talbot, B. G. (1995). Active agents and mechanism of coagulation of turbid waters using Moringa oleifera. Water Res. 29, 703–710. doi: 10.1016/0043-1354(94)00161-Y
Niemirowicz, K., Durnaś, B., Tokajuk, G., Guszek, K., Wilczewska, A. Z., Misztalewska, I., et al. (2016). Magnetic nanoparticles as a drug delivery system that enhance fungicidal activity of polyene antibiotics. Nanomedicine 12, 2395–2404. doi: 10.1016/j.nano.2016.07.006
Odintsova, T. I., Rogozhin, E. A., Sklyar, I. V., Musolyamov, A. K., Kudryavtsev, A. M., Pukhalsky, V. A., et al. (2010). Antifungal activity of storage 2S albumins from seeds of the invasive weed dandelion Taraxacum officinale Wigg. Protein Pept. Lett. 17, 522–529. doi: 10.2174/092986610790963591
Onsare, J. G., and Arora, D. S. (2015). Antibiofilm potential of flavonoids extracted from Moringa oleifera seed coat against Staphylococcus aureus, Pseudomonas aeruginosa and Candida albicans. J. Appl. Microbiol. 118, 313–325. doi: 10.1111/jam.12701
Onsare, J. G., Kaur, H., and Arora, D. S. (2013). Antimicrobial activity of Moringa oleifera from different locations against some human pathogens. Acad. J. Med. Plants 1, 80–91. doi: 10.15413/ajmp.2013.0105
Pavankumar, A. R., Kayathri, R., Murugan, N. A., Zhang, Q., Srivastava, V., Okoli, C., et al. (2014). Dimerization of a flocculent protein from Moringa oleifera: experimental evidence and in silico interpretation. J. Biomol. Struct. Dyn. 32, 406–415. doi: 10.1080/07391102.2013.770374
Pereira, M. L. (2014). Aspectos Estruturais, Farmacológicos e Toxicológicos de Mo-CBP4, Uma Proteína Ligante à Quitina de Moringa oleifera com Atividade Anti-inflamatória e Antinociceptiva via Oral. Doctoral’s thesis, Federal University of Ceará, Fortaleza.
Pereira, M. L., Oliveira, H. D., Oliveira, J. T. A., Gifoni, J. M., Rocha, R. O., Sousa, D. O. B., et al. (2011). Purification of a chitin-binding protein from Moringa oleifera seeds with potential to relieve pain and inflammation. Protein Pept. Lett. 18, 1078–1085. doi: 10.2174/092986611797200959
Perkins, D. N., Pappin, D. J. C., Creasy, D. M., and Cottrell, J. S. (1999). Probability based protein identification by searching sequence databases using mass spectrometry data. Electrophoresis 20, 3551–3567. doi: 10.1002/(SICI)1522-2683(19991201)20:18<3551::AID-ELPS3551>3.0.CO;2-2
Perutz, M. F., Johnson, T., Suzuki, M., and Finch, J. T. (1994). Glutamine repeats as polar zippers: their possible role in inherited neurodegenerative diseases. Proc. Natl. Acad. Sci. U.S.A. 91, 5355–5358. doi: 10.1073/pnas.91.12.5355
Peumans, W. J., and Van Damme, E. J. M. (1995). Lectins as plant defense proteins. Plant Physiol. 109, 347–352. doi: 10.1104/pp.109.2.347
Pierce, C. G., Chaturvedi, A. K., Lazzell, A. L., Powell, A. T., Saville, S. P., McHardy, S. F., et al. (2015). A novel small molecule inhibitor of Candida albicans biofilm formation, filamentation and virulence with low potential for the development of resistance. NPJ Biofilms Microbiomes 1:15012. doi: 10.1038/npjbiofilms.2015.12
Preechasuth, K., Anderson, J. C., Peck, S. C., Brown, A. J. P., Gow, N. A. R., and Lenardon, M. D. (2015). Cell wall protection by the Candida albicans class I chitin synthases. Fungal Genet. Biol. 82, 264–276. doi: 10.1016/j.fgb.2015.08.001
Raja, S. B., Murali, M. R., Kumar, N. K., and Devaraj, S. N. (2011). Isolation and partial characterisation of a novel lectin from Aegle marmelos fruit and its effect on adherence and invasion of Shigella to HT29 cells. PLoS ONE 6:e16231. doi: 10.1371/journal.pone.0016231
Regente, M., Taveira, G. B., Pinedo, M., Elizalde, M. M., Ticchi, A. J., Diz, M. S. S., et al. (2014). A sunflower lectin with antifungal properties and putative medical mycology applications. Curr. Microbiol. 69, 88–95. doi: 10.1007/s00284-014-0558-z
Reisfeld, R. A., Lewis, U. J., and Williams, D. E. (1962). Disk electrophoresis of basic proteins and peptides on polyacrylamide gels. Nature 195, 281–283. doi: 10.1038/195281a0
Ribeiro, S. F. F., Silva, M. S., da Cunha, M., Carvalho, A. O., Dias, G. B., Rabelo, G., et al. (2012). Capsicum annuum L. trypsin inhibitor as a template scaffold for new drug development against pathogenic yeast. Antonie Van Leeuwenhoek 101, 657–670. doi: 10.1007/s10482-011-9683-x
Saadabi, A. M., and Zaid, I. E. A. (2011). An in vitro antimicrobial activity of Moringa oleifera L. seed extracts against different groups of microorganisms. Aust. J. Basic Appl. Sci. 5, 129–134.
Salas, C. E., Badillo-Corona, J. A., Ramírez-Sotelo, G., and Oliver-Salvador, C. (2015). Biologically active and antimicrobial peptides from plants. BioMed Res. Int. 2015:102129. doi: 10.1155/2015/102129
Sanglard, D. (2016). Emerging threats in antifungal-resistant fungal pathogens. Front. Med. 3:11. doi: 10.3389/fmed.2016.00011
Sanitá, P. V., Zago, C. E., Mima, E. G. O., Pavarina, A. C., Jorge, J. H., Machado, A. L., et al. (2014). In vitro evaluation of the enzymatic activity profile of non-albicans Candida species isolated from patients with oral candidiasis with or without diabetes. Oral Surg. Oral Med. Oral Pathol. Oral Radiol. 118, 84–91. doi: 10.1016/j.oooo.2014.03.020
Santos, A. F. S., Luz, L. A., Pontual, E. V., Napoleão, T. H., Paiva, P. M. G., and Coelho, L. C. B. B. (2015). Moringa oleifera: resource management and multiuse life tree. Adv. Res. 4, 388–402. doi: 10.9734/AIR/2015/18177
Schägger, H., and von Jagow, G. (1987). Tricine-sodium dodecyl sulfate-polyacrylamide gel electrophoresis for the separation of proteins in the range from 1 to 100 kDa. Anal. Biochem. 166, 368–379. doi: 10.1016/0003-2697(87)90587-2
Schelenz, S. (2008). Management of candidiasis in the intensive care unit. J. Antimicrob. Chemother. 61, 31–34. doi: 10.1093/jac/dkm430
Scorzoni, L., Lucas, M. P., Mesa-Arango, A. C., Fusco-Almeida, A. M., Lozano, E., Cuenca-Estrella, M., et al. (2013). Antifungal efficacy during Candida krusei infection in non-conventional models correlates with the yeast in vitro susceptibility profile. PLoS ONE 8:e60047. doi: 10.1371/journal.pone.0060047
Sellami, M., Châari, A., Aissa, I., Bouaziz, M., Gargouri, Y., and Miled, N. (2013). Newly synthesized dopamine ester derivatives and assessment of their antioxidant, antimicrobial and hemolytic activities. Process Biochem. 48, 1481–1487. doi: 10.1016/j.procbio.2013.07.022
Shebek, K., Schantz, A. B., Sines, I., Lauser, K., Velegol, S., and Kumar, M. (2015). The flocculating cationic polypetide from Moringa oleifera seeds damages bacterial cell membranes by causing membrane fusion. Langmuir 31,4496–4502. doi: 10.1021/acs.langmuir.5b00015
Taniguchi, M., Ikeda, A., Nakamichi, S., Ishiyama, Y., Saitoh, E., Kato, T., et al. (2013). Antimicrobial activity and mechanism of action of a novel cationic α-helical octadecapeptide derived from heat shock protein 70 of rice. Peptides 48, 147–155. doi: 10.1016/j.peptides.2013.08.011
Tavernier, E., Desnos-Ollivier, M., Honeyman, F., Srour, M., Fayard, A., Cornillon, J., et al. (2015). Development of echinocandin resistance in Candida krusei isolates following exposure to micafungin and caspofungin in a BM transplant unit. Bone Marrow Transplant. 50, 158–160. doi: 10.1038/bmt.2014.230
Thordal-Christensen, H., Zhang, Z., Wei, Y., and Collinge, D. B. (1997). Subcellular localization of H2O2 in plants. H2O2 accumulation in papillae and hypersensitive response during the barley-powdery mildew interaction. Plant J. 11, 1187–1194. doi: 10.1046/j.1365-313X.1997.11061187.x
Tian, Y., Zeng, Y., Zhang, J., Yang, C., Yan, L., Wang, X., et al. (2015). High quality reference genome of drumstick tree (Moringa oleifera Lam.), a potential perennial crop. Sci. China Life Sci. 58, 627–638. doi: 10.1007/s11427-015-4872-x
Tomar, P. P. S., Chaudhary, N. S., Mishra, P., Gahloth, D., Patel, G. K., Selvakumar, P., et al. (2014a). Purification, characterisation and cloning of a 2S albumin with DNase, RNase and antifungal activities from Putranjiva roxburghii. Appl. Biochem. Biotechnol. 174, 471–482. doi: 10.1007/s12010-014-1078-9
Tomar, P. P. S., Nikhil, K., Singh, A., Selvakumar, P., Roy, P., and Sharma, A. K. (2014b). Characterization of anticancer, DNase and antifungal activity of pumpkin 2S albumin. Biochem. Biophys. Res. Commun. 448, 349–354. doi: 10.1016/j.bbrc.2014.04.158
Vandeputte, P., Ferrari, S., and Coste, A. T. (2012). Antifungal resistance and new strategies to control fungal infections. Int. J. Microbiol. 2012:713687. doi: 10.1155/2012/713687
Wang, K., Dang, W., Xie, J., Zhu, R., Sun, M., Jia, F., et al. (2015). Antimicrobial peptide protonectin disturbs the membrane integrity and induces ROS production in yeast cells. Biochim. Biophys. Acta 1848, 2365–2373. doi: 10.1016/j.bbamem.2015.07.008
Wang, S., Xiuyun, Y., Chen, J., and Rao, P. (2012). A novel chitinase isolated from Vicia faba and its antifungal activity. Food Res. Int. 45, 116–122. doi: 10.1016/j.foodres.2011.10.010
Wong, J. H., Ng, T. B., Cheung, R. C. F., Ye, X. J., Wang, H. X., Lam, S. K., et al. (2010). Proteins with antifungal properties and other medicinal applications from plants and mushrooms. Appl. Microbiol. Biotechnol. 87, 1221–1235. doi: 10.1007/s00253-010-2690-4
World Health Organization [WHO] (2015). World Antibiotic Awareness Week. Available at: http://www.Who.int/mediacentre/events/2015/world-antibiotic-awarenessweek/event/en/ (accessed January 27, 2017).
Youle, R. J., and Huang, A. H. C. (1981). Occurrence of low molecular weight and high cysteine containing albumin storage protein in oilseeds of diverse species. Am. J. Bot. 68, 44–48. doi: 10.2307/2442990
Zacharius, R. M., Zell, T. E., Morrison, J. H., and Woodlock, J. J. (1969). Glycoprotein staining following electrophoresis on acrylamide gels. Anal. Biochem. 30, 148–152. doi: 10.1016/0003-2697(69)90383-2
Keywords: prospection, moringa, plant protein, antifungal, candidiasis
Citation: Neto JXS, Pereira ML, Oliveira JTA, Rocha-Bezerra LCB, Lopes TDP, Costa HPS, Sousa DOB, Rocha BAM, Grangeiro TB, Freire JEC, Monteiro-Moreira ACO, Lobo MDP, Brilhante RSN and Vasconcelos IM (2017) A Chitin-binding Protein Purified from Moringa oleifera Seeds Presents Anticandidal Activity by Increasing Cell Membrane Permeability and Reactive Oxygen Species Production. Front. Microbiol. 8:980. doi: 10.3389/fmicb.2017.00980
Received: 31 January 2017; Accepted: 16 May 2017;
Published: 06 June 2017.
Edited by:
Yuji Morita, Aichi Gakuin University, JapanReviewed by:
Karin Thevissen, KU Leuven, BelgiumOsmar Nascimento Silva, Universidade Católica Dom Bosco, Brazil
Miguel Cacho Teixeira, Universidade de Lisboa, Portugal
Copyright © 2017 Neto, Pereira, Oliveira, Rocha-Bezerra, Lopes, Costa, Sousa, Rocha, Grangeiro, Freire, Monteiro-Moreira, Lobo, Brilhante and Vasconcelos. This is an open-access article distributed under the terms of the Creative Commons Attribution License (CC BY). The use, distribution or reproduction in other forums is permitted, provided the original author(s) or licensor are credited and that the original publication in this journal is cited, in accordance with accepted academic practice. No use, distribution or reproduction is permitted which does not comply with these terms.
*Correspondence: Ilka M. Vasconcelos, aW12YXNjb0B1ZmMuYnI=