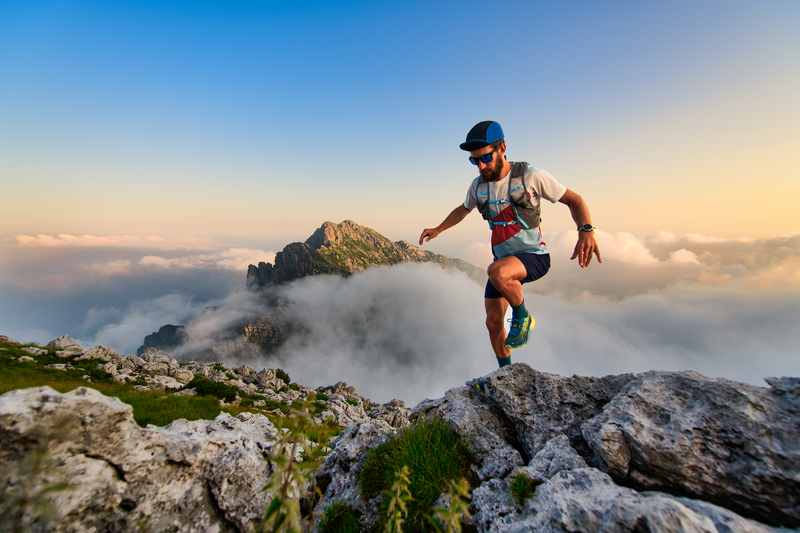
95% of researchers rate our articles as excellent or good
Learn more about the work of our research integrity team to safeguard the quality of each article we publish.
Find out more
REVIEW article
Front. Microbiol. , 20 April 2017
Sec. Extreme Microbiology
Volume 8 - 2017 | https://doi.org/10.3389/fmicb.2017.00683
Concrete corrosion is one of the most significant problems affecting valuable sewer infrastructure on a global scale. This problem occurs in the aerobic zone of the sewer, where a layer of surface corrosion develops on the exposed concrete and the surface pH is typically lowered from around 11–10 (pristine concrete) to pH 2–4. Acidophilic microorganisms become established as biofilms within the concrete corrosion layer and enhance the loss of concrete mass. Until recently, the acidophilic community was considered to comprise relatively few species of microorganisms, however, the biodiversity of the corrosion community is now recognized as being extensive and varying from different sewer environmental conditions. The diversity of acidophiles in the corrosion communities includes chemolithoautotrophs, chemolithoheterotrophs, and chemoorganoheterotrophs. The activity of these microorganisms is strongly affected by H2S levels in the sewer gas phase, although CO2, organic matter, and iron in the corrosion layer influence this acidic ecosystem. This paper briefly presents the conditions within the sewer that lead to the development of concrete corrosion in that environment. The review focuses on the acidophilic microorganisms detected in sewer corrosion environments, and then summarizes their proposed functions and physiology, especially in relation to the corrosion process. To our knowledge, this is the first review of acidophilic corrosion microbial communities, in which, the ecology and the environmental conditions (when available) are considered. Ecological studies of sewer corrosion are limited, however, where possible, we summarize the important metabolic functions of the different acidophilic species detected in sewer concrete corrosion layers. It is evident that microbial functions in the acidic sewer corrosion environment can be linked to those occurring in the analogous acidic environments of acid mine drainage and bioleaching.
In developed countries sewer systems are implemented for the collection of wastewater and transportation of that to wastewater treatment facilities (Figure 1). These piping systems are extensive and are typically constructed of concrete. The total length of sewer in Australian is almost 117,000 km, and approximately 40% of this network is constructed from concrete. Through the piped connections from houses, drains, manholes, pump stations, and storm overflows, the collection system can be further divided into rising mains and gravity flow regions (Figure 1). The rising main sections are designed and operated to pump the sewage to a higher altitude and have no gas phase present within the pipes. In the gravity flow regions, the sewage flows due to gravity and these are always partially filled with sewage and thus have a gas phase (Figure 2). The broad range of pollutants and the high microbial presence within sewage is such that sewers function as ‘microbial reactors’ where substances are transformed and degraded based on their chemical and biological reactivity (Hvitved-Jacobsen et al., 2013). These microbial activities can lead to concrete corrosion, especially in gravity pipes. For many years the process of sewer concrete corrosion in the gravity sewer section was considered to be purely chemical (Olmstead and Hamlin, 1900) until it was revealed that the direct cause of sewer concrete corrosion, sulfuric acid, was generated biologically rather than chemically (Parker, 1945a,b, 1947).
FIGURE 2. A diagram of a cross section of a sewer gravity concrete pipe adapted from Hvitved-Jacobsen et al. (2013), summarizing the major processes that lead to acid formation in the aerobic biofilms and the onset of sewer corrosion.
Since those discoveries of Parker, the process has been termed microbially induced concrete corrosion (MICC) where microorganisms are involved in oxidation of hydrogen sulfide (H2S) that occurs in the headspace gas of the sewer (Parker, 1945a). Hydrogen sulfide production occurs in the sewer through the activity of sulfate reducing bacteria (SRB) mainly in the rising mains (Hvitved-Jacobsen et al., 2013) but also in the anaerobic sections of gravity pipes (Figure 2). After generation, the H2S is partitioned from the liquid into the gas phase in the gravity sewer pipes. The gaseous H2S can then partition into condensation layers on the gas-phase exposed walls of the concrete pipe (Figure 2). In those surface layers it is oxidized into sulfuric acid through the activity of aerobic sulfide-oxidizing microorganisms (Jiang et al., 2016). The sulfuric acid generated reacts with alkaline cement materials and produces gypsum and ettringite that have poor structural capacity and leads to weakened structure and eventual collapse of the concrete. Within the sewer, MICC often occurs in hot spots, which are located in regions just above the sewage flow level and at the ceiling of the headspace (Islander et al., 1991), respectively, referred to as the “tidal region” and the “crown region” (Figure 2). The tidal regions are frequently wetted by wastewater, while the crown region is exposed to the headspace sewer gas and only very occasionally (e.g., during flooding) contacts the wastewater (Figure 2).
Concrete corrosion is regarded as one of the most serious problems currently affecting the sewer infrastructure. Corrosion damage to sewers leads to premature replacement or rehabilitation which are estimated to cost several billion dollars every year globally (Pikaar et al., 2014; Jiang et al., 2015a). In addition, as hydrogen sulfide is toxic to humans and corrosive, the structural failure that emerges can cause damage to adjacent infrastructure, property and potentially to citizens (Jiang et al., 2015a).
When the MICC is well established, acidophilic sulfur-oxidizing microorganisms (ASOM) will be active in the extremely acidic conditions of the surface biofilms (around pH 2–4) (Joseph et al., 2012) and result in the rapid loss of concrete mass, which can be up to 10 mm year-1 (Zhang et al., 2008). Understanding details of the ecology of sulfide-oxidizing microorganisms, especially the ASOM associated with MICC is crucial for the control and mitigation of sewer corrosion. The molecular approaches to determine details of the microbiology have advanced greatly in the past decade and are providing revealing insight. This is especially with the application of high-throughput next generation sequencing (NGS) of small subunit rRNA gene amplicons (see further explanation in Acidophilic Microorganisms Detected in the Established Corrosion Layer) for community profiling and of whole community DNA for metagenomics (Cayford et al., 2012; Gomez-Alvarez et al., 2012). This, to the authors’ knowledge, is the first review to focus on acidophiles in the sewer corrosion process.
Concrete corrosion results from various abiotic and biotic processes, which are facilitated by the corrosive headspace gas present in sewers. During the initiation stage of concrete corrosion, abiotic processes such as carbonation (CO2) and H2S acidification reduce the surface pH of concrete from ∼13 to ∼9 (Joseph et al., 2012). After this initial abiotic acidification, biological processes, primarily sulfide oxidation, cause the direct formation of sulfuric acid which will further lower the concrete surface pH (Okabe et al., 2007).
H2S is ubiquitous in sewers and it is believed at levels above 2 ppm it will cause sewer corrosion (O’Dea, 2007). However, H2S concentrations differ temporally and spatially (Jiang et al., 2014a; Wells and Melchers, 2015) with average concentrations seen to vary between a few ppm up to a few 100 ppm in most sewer systems, although in some extreme situations, concentrations as high as 550 ppm are detected (Wells and Melchers, 2015). Although there are volatile organic compound (VOC) emissions from the wastewater to the sewer gas, the concentrations are actually low at ppb to ppm levels or even less (Corsi et al., 1995; Quigley and Corsi, 1995; Chan and Hanaeus, 2006; Wu et al., 2006; Huang et al., 2012; Decottignies et al., 2013; Sivret et al., 2016). The smell of sewer gas is mainly caused by H2S (Jiang et al., 2015a; Sivret et al., 2016). This sewer gas phase H2S is a potential key energy source for chemolithotrophic sulfur oxidizing bacteria. Also, important for autotrophic microbial growth, elevated levels of CO2 (around 1.0%) have been detected in the headspace in various sewer field locations (Joseph et al., 2012).
Both abiotic and biotic processes heavily depend on the water content of the concrete, which is directly related to the relative humidity (RH) in sewer air. The sewer RH typically ranges between 60 and 100% (Ward et al., 2011; Wells et al., 2012). In addition, the tidal regions in sewer walls are frequently wetted by wastewater flooding, which will increase the water content of the concrete in those regions. At the high RH in the sewer pipes, moisture will condensate on the crown region, as the walls of sewer pipe usually have a lower temperature than the sewer atmosphere due to cooling from the surrounding soil. It is observed that high RH levels shorten the corrosion initiation and also facilitate the corrosion process (Jiang et al., 2014a, 2015b). Frequent wastewater flushing in the tidal regions, occasional flooding, high RH, and condensation water in the crown region facilitate the microbial colonization on the concrete surface as well as providing the essential medium for the chemical and biological sulfide oxidation reactions.
On the corroding concrete surface, a soft (cottage cheese like) moist layer forms comprising largely of crystalline gypsum and other sulfur species (discussed more below), within which microbial biofilms will exist (Figure 3). However, because of the minimal contact with wastewater and the paucity of nutrients innately present in concrete, the corrosion layer is likely very low in nutrients and organic carbon, especially on the concrete surface moving away from the tidal region. At the crown region, the main source of water is condensation, gas diffusion will provide volatile substrates and nutrients, and degrading microorganisms will also provide nutrient for growth. Although the corrosion is often reported to occur in the crown region, it is observed that more severe corrosion can occur in the tidal region where more frequent replenishment of nutrients, microbial inoculum, and moisture will occur in comparison to parts of the wall further away from that region (Jiang et al., 2014a).
FIGURE 3. Scanning electron microscopy image of the corroded concrete surface adapted from Cayford (2012). Small elongated shapes are microbial cells, and angular crystal formations are sulfur-containing corrosion products (Cayford, 2012).
Oxygen diffusion into the corrosion layer is crucial for the activity of the aerobic microorganisms of MICC. Microsensors have been used to monitor the pH and oxygen changes that occur into the depth of a cement coupon corrosion layer (Figure 4) (Okabe et al., 2007). It was seen that the pH of the heavily corroded layer was around 2.6, down to a depth of 2 mm. Oxygen concentrations rapidly decreased with depth, accompanying a decrease of cell numbers (Figure 4), suggesting in this instance that because of the gas diffusion limitations (O2 and/or H2S), the microbes occurred mainly on the concrete surface where the biofilm was denser nearer the surface of the corrosion layer (Okabe et al., 2007).
FIGURE 4. Cell density, oxygen and pH profiles occurring in a corrosion layer developed on the surface of a cement coupon exposed to sewer conditions for 1 year (Okabe et al., 2007). (A) Density profiles of cells detected in the gypsum layer. The non-specific DAPI stain and the specific probes Thio820, ACD840, and LF655 target Acidithiobacillus spp., Acidiphilium spp., and Leptospirillum spp., respectively. (B) Concentration profiles of dissolved oxygen and pH in the top 2,000 micrometers of the corrosion layer (Okabe et al., 2007) (reproduced with permission from American Society for Microbiology).
The activity of the biofilms in the top regions of the corrosion layer will produce acid (Okabe et al., 2007) which diffuses toward the intact concrete and reacts with the calcium silicates and calcium hydroxides of the concrete. Thus, the conditions within the corrosion layer vary with depth and are characterized by a large pH gradient (Figure 5). Gypsum (CaSO4) is formed preferentially in acidic conditions (at pH lower than 3), hence it is usually more prevalent nearer the surface of the corrosion layer (Mori et al., 1992). The gypsum within the corrosion layer reacts with calcium aluminate hydrate to form ettringite at higher pH, and thus the ettringite layer is beyond gypsum layer nearer the intact concrete. The depth of gypsum and ettringite layers depends on the sulfate concentration and pH. With increased acid production in the corrosion layer, ettringite may be converted to gypsum (Mori et al., 1992) and in some types of concrete, no ettringite is detected (Gutiérrez-Padilla et al., 2010). Unlike the original concrete matrix, gypsum and ettringite are non-structure-supporting materials and are both highly expansive (Monteny et al., 2000). The formation of gypsum and ettringite thus weakens the concrete structure and is believed to cause cracking of the concrete due to the expansive nature of the materials. Acid will diffuse into the corrosion layer to depths where oxygen diffusion is limited (Figure 4) and iron species, either from within the concrete or from iron rebar become dissolved and could transfer in the concrete pores and micro-cracks. Additional cracking of the concrete can also be caused by the rust precipitation in iron salt rich areas near the intact concrete (Figure 5) (Jiang et al., 2014b). Based on these observations, a conceptual model for the corrosion layer is proposed (Figure 5). The microbial biofilm is denser near the surface of the corrosion layer where the oxygen and H2S levels are highest (Okabe et al., 2007). Sulfuric acid is generated by ASOM in the biofilm and this diffuses through the corrosion layer toward the surface of the intact concrete and can penetrate this to a depth of at least 2 mm (Okabe et al., 2007). With the acid diffusion, the gypsum (pH < 3) and ettringite zones (pH > 3) are formed and as they are expansive, together with iron mineral precipitation, cause cracking of the intact concrete.
FIGURE 5. A conceptual model for the sewer concrete corrosion layer as adapted from Jiang et al. (2014b). See the text for description of the activities within the zones.
Important microbial protagonists of the corrosion process are sulfur-oxidizing microorganisms as they accelerate the reduction of surface pH through the production of various sulfur compounds including sulfuric acid. As these processes lead to a gradual decrease of the concrete surface pH, microorganisms colonize the concrete and the composition of the microbial communities will change with time. Two types of microorganisms relevant for sewer corrosion are neutrophilic sulfur-oxidizing microorganisms (NSOM) and acidophilic sulfur-oxidizing microorganisms (ASOM) both of which use reduced forms of sulfur as energy sources, but respectively, have a preference for growth at a neutral or acidic pH (Islander et al., 1991; Roberts et al., 2002). After the pH of the sewer surface is abiotically reduced to 9, NSOM will colonize the surface and produce acid to eventually lower the pH to 5-3 (Roberts et al., 2002). The various dominant NSOM species detected include: Thiothrix spp., Thiobacillus plumbophilus (formally as Sulfuriferula plumbophilus) (Watanabe et al., 2015), Thiomonas sp., and Halothiobacillus neapolitanus (Okabe et al., 2007; Santo Domingo et al., 2011; Ling et al., 2015). Thiothrix spp. and Thiomonas spp. have the ability to grow chemoorganoheterotrophically or chemolithoautotrophically using either organic carbon or the inorganic sulfur species H2S, S2O32-, and S0 as electron donors. In comparison, Halothiobacillus sp. are obligate chemolithoautotrophic sulfur-oxidizing bacteria. The initial activity of NSOM on the sewer surface provides suitable conditions for subsequent colonization by ASOM (Mild et al., 1983; Islander et al., 1991; Davis et al., 1998; Okabe et al., 2007; Jiang et al., 2015a).
As mentioned above, there is a succession of microbial types involved in the concrete corrosion from the time of initial exposure, when the concrete surface is alkaline (pH 9–13), to when an established corroding concrete has developed and this is lowered to around pH 2–4. This review focuses on the acidophilic microorganisms of the established corrosion layer when the surface pH is acidic. Currently much importance is placed on the readily cultured bacterium Acidithiobacillus thiooxidans (formerly Thiobacillus thiooxidans) as a main biological component of sewer corrosion. Sulfur oxidation, as performed by these bacteria is undoubtedly a key metabolic activity, however, as revealed below, recent investigations of sewer corrosion ecology using culture independent detection techniques indicate that a diverse range of microorganisms is present. Recent studies performing microbial community profiling by NGS have advanced and are providing incredible new insight, even though molecular studies of the acidic concrete corrosion environment are still in their infancy.
Conventionally, research of the acidophilic communities involved in MICC has been mainly based on culture-dependent methods, where microorganisms are grown from corrosion samples using various culture media (Davis et al., 1998; Keller and Zengler, 2004). Although these results provide insight into the cultivable microorganisms involved in MICC (Parker, 1945a,b; Harrison, 1984; Islander et al., 1991), they can be misleading due to the bias and limitations of the culture-based techniques. Only a small fraction (in the order of 1%) of bacteria present in the environment are readily obtained in pure culture in the laboratory (Staley and Konopka, 1985). The choice of growth substrates, supplements and conditions for cultivation heavily influences what will grow, and hence only a limited number of microorganisms are detected in individual culturing attempts.
The most typical genus of acidophilic microorganisms associated with biogenic acid production detected by culture dependent methods is Acidithiobacillus (Kelly and Wood, 2000), and the prominent species include A. ferrooxidans, A. thiooxidans, and A. caldus (Parker, 1947; Harrison, 1984; Islander et al., 1991; Cho and Mori, 1995; Davis et al., 1998; Nica et al., 2000; Hernandez et al., 2002; Yamanaka et al., 2002; Okabe et al., 2007). Acidithiobacilli are autotrophic sulfur-oxidizing bacteria (Nica et al., 2000) which is an essential advantage in an environment with low organic carbon availability and high levels of reduced sulfur compounds. A. thiooxidans is the most commonly cultured organism among Acidithiobacillus from sewer concrete corrosion (Islander et al., 1991).
Heterotrophic bacteria have been detected in sewer corroding concrete (Davis et al., 1998; Nica et al., 2000). In one study, these aerobic heterotrophs were detected to be in the range of 105 cells/g of corrosion layer (Davis et al., 1998). Although the type of heterotrophs was not mentioned, this study reported significant quantities of heterotrophs in the sewer corrosion environment. That study also detected similar levels of NSOM but lower levels of ASOM in the corrosion layers. However, the possibility that these heterotrophs could be acidophiles was not examined as they were cultured on neutrophilic media (Davis et al., 1998). A subsequent study also isolated aerobic heterotrophs from corrosion layers (Nica et al., 2000). This includes isolates of the genera Bacillus and Microbacterium and of the species Ochrobactrum anthropi. These isolated heterotrophs were not shown to oxidize sulfur compounds during pure culture growth at or below pH 5 (Nica et al., 2000). Thus the nature of the growth of these heterotrophs in the acidic corrosion environment is not clear, although, it is suggested that these bacteria are scavenging organic compounds produced by growth of the NSOM and ASOM (Nica et al., 2000).
Heterotrophic fungi, have also been cultured from sewer corrosion samples (Cho and Mori, 1995; Nica et al., 2000), however, the fungi isolated in these studies were not formally identified. Fungi isolated by Cho and Mori (1995) were reported to grow at acidic conditions (pH 2.6), although neutral pH was the optimum for growth. Additionally, it is suggested that these fungi exist in a symbiotic relationship with ASOM by oxidizing H2S to thiosulfate and by utilizing organic compounds produced by the bacteria (Cho and Mori, 1995). Another study isolated a fungus from corroding concrete which was identified as a Fusarium sp. (Gu et al., 1998). However, Fusarium sp. are not reported to be acidophilic, although they are suggested to contribute to the corrosion process by production of organic acids (Gu et al., 1998).
The development of culture-independent methods has revolutionized studies of microbial ecology (Ward et al., 1990). These methods focus on analysis of DNA sequences of particular genes [amplified by the polymerase chain reaction (PCR)] and microbial community profiling is often performed by analysis of the small subunit rRNA genes. These are the 16S rRNA genes, for detection of bacteria and archaea, and the analogous 18S rRNA genes for detection of eukaryotes. NGS provides very efficient methodology to rapidly obtain thousands of gene reads that represent operational taxonomic units (OTUs) within the sample. Although, typically these are incomplete reads of a few hundred bases, so that the resolution for identification of the OTU is limited to the order, family, or genus level at best. More recently, whole or near complete genome sequences are obtained directly from various environmental samples through metagenomics (Hugenholtz and Tyson, 2008). This also provides basic community composition information, but more importantly individual genomic information is obtained from which functional capabilities of the microorganisms in the sample can be estimated. Presently there are few metagenomic studies of the sewer corrosion environment.
As mentioned, molecular based studies may utilize PCR amplification of genes from corrosion samples. However, it is reported that the success of the amplification can be variable, and this likely relates to the quality and quantity of DNA extracted from such samples (Cayford et al., 2012). The relatively low levels of microorganisms in the samples and the co-extraction of molecules inhibitory to the PCR amplification can limit these molecular based studies. Consequently, the non-cultivation techniques suffer from biases and the best studies will include multiple approaches, such as verifying sequencing results with FISH and other non-PCR based methods. The molecular based approach has been extremely enlightening and has led to significant new insights into the microbial communities involved in sewer concrete corrosion. Congruent with findings of culture dependent methods DNA sequencing directly from corrosion samples indicates that Acidithiobacillus spp. are often highly abundant in corrosion samples (Okabe et al., 2007; Satoh et al., 2009; Gomez-Alvarez et al., 2012; Ling et al., 2015; Jiang et al., 2016). According to culturing or by cloning and full 16S rRNA gene sequencing, these Acidithiobacillus spp. are likely A. thiooxidans (Parker, 1947; Harrison, 1984; Islander et al., 1991; Davis et al., 1998; Nica et al., 2000). However, there are instances where NGS analyses report low abundance or no detection of A. thiooxidans in sewer corrosion samples (Santo Domingo et al., 2011; Cayford et al., 2012).
High microbial diversity is not characteristic of acidic corrosion samples, as the communities can be dominated by few species (Pagaling et al., 2014). However, sequencing has revealed numerous different microbial types in these acidic samples. Mycobacterium spp. are often detected as abundant in acidophilic communities of sewer corrosion layers (Vincke et al., 2001; Okabe et al., 2007; Cayford et al., 2012; Pagaling et al., 2014). Cayford et al. (2012) investigated MICC communities in two sewers in Sydney and found Acidiphilium spp. and Mycobacterium spp. to be the most dominant acidophilic microbial groups across the 10 samples examined. In contrast, Acidithiobacillus spp. was prominent in only one sample and made up<3% of the total populations in all others. Previously, these acidophilic Mycobacterium were assumed to have a heterotrophic role in MICC, however, there are suggestions they may oxidize sulfur (Cayford et al., 2012), and recently an acidophilic Mycobacterium sp. has been observed to oxidize sulfur and produce acid (Kusumi et al., 2011). In Cayford’s study, wall and ceiling samples of the sewers were analyzed and in general higher microbial diversity was observed in the wall samples where significant proportions of Xanthomonadales spp., Burkholderiales spp., and Sphingobacteriales spp. were detected (Cayford et al., 2012). The Xanthomonadales are reported in other sewer corrosion environments (Satoh et al., 2009; Santo Domingo et al., 2011; Gomez-Alvarez et al., 2012), and are considered primarily as heterotrophs. Although the Xanthomonadales sequence from Cayford forms a phylogenetic cluster with no cultured representatives, the cluster affiliates with Xanthomonadales spp. which are shown to be capable of sulfur oxidation (Lee et al., 2007).
In a more recent study, crown samples were examined at six points located downstream from the outlet of a rising main (Pagaling et al., 2014). High acidity (averaging between pH 0.3–1.5) and low microbial diversity was reported, the lowest diversity coinciding with the more acidic samples. In five of the six crown samples Mycobacterium spp. dominated, being between 45 and 98% of the detected bacteria. Acidithiobacillus spp. were prominent in only two crown samples, and these being detected at between 28 and 36% of the total bacteria (Pagaling et al., 2014). Methylacidiphilum spp. were prominent in 2 of the crown samples. These are known acidophilic methanotrophs (Hou et al., 2008), and are likely utilizing methane that was detected in the headspace of that sewer.
On one occasion Ferroplasma spp. are reported in severely corroded concrete biofilms with an extremely low pH (Ling et al., 2015). Ferroplasma spp. are extreme acidophiles and versatile heterotrophs that can oxidize iron (Dopson et al., 2004), although they are not known for sulfur oxidation capabilities. Sequencing also detected an alga in an extremely acidic sewer corrosion layer (Pagaling et al., 2014). This was identified as Cyanidium caldarium, an organism detected in other acidic environments, and is thought to be growing heterotrophically in the sewer environment. Due to the depth of NGS and discovery by clone sequencing from sewer corrosion samples these molecular-based studies are detecting a wide range of microorganisms and many of those are considered to be primarily heterotrophic acidophiles and sometimes halotolerant (Okabe et al., 2007; Satoh et al., 2009; Santo Domingo et al., 2011). In addition to those mentioned above these proposed heterotrophs includes species of Pseudoxanthomonas (Satoh et al., 2009), Ochrobactrum, Achromobacter, Azonexus, Acinetobacter, and Clostridium (Okabe et al., 2007). In a study, sequencing a large number of cloned genes from crown and manhole samples, a wide range of taxa is detected (Santo Domingo et al., 2011). While considerable diversity can be detected in these studies of biofilms of sewer corrosion layers, often the matching reporting of environmental conditions is poor, even the pH may not be mentioned. These environmental factors vary considerably from site to site and are crucial for understanding the nature and extent of MICC and acidity that occurs. However, even from the imperfect data from these studies, a summary of core microorganisms important for acidic sewer concrete corrosion can be suggested (Table 1).
TABLE 1. Specific microorganisms detected in acidic concrete corrosion layers and their functions within sewers using culture independent methods.
Cells have been detected directly in corrosion samples using probes targeting rRNA molecules by fluorescence in situ hybridisation (FISH). While this approach is likely to provide the most real quantitative estimations of cells in samples, unfortunately it is only very rarely applied to study concrete corrosion. FISH of concrete samples is conceivably a difficult procedure due to combination of relatively meager cell densities, variable cell activity, and interfering autofluorescence from mineral particles. Nonetheless, a successful FISH approach has been applied by performing the hybridisation in solution (rather than directly on samples dried onto microscope slides) and filtering portions of the solution onto black filters for microscopy (Hernandez et al., 2002). Total cells in corrosion samples of a sewer manhole and a pipe crown were determined to be 7.2 × 107 cells/g of dry corrosion product and 4.5 × 108 cells/g of dry corrosion product, respectively. Acidithiobacillus were detected at 9 and 13% of the total cells in the manhole and crown samples, respectively (Hernandez et al., 2002). In another study using FISH, corroded mortar samples were exposed in a sewer manhole for 1 year where a thick gypsum corrosion layer formed (10 mm) and pH in the range of 1.6–2.7 was detected (Okabe et al., 2007). Sequencing indicated A. thiooxidans was prominent, and FISH verified that Acidithiobacillus were 6.1 × 109 cells/cm3 of corrosion layer, this being about 50% of the total cells. Both these studies verified Acidithiobacillus spp. as important acidophiles in MICC, however, use of a more extensive range of probes is required to obtain a more complete picture of community compositions. Both studies did use genus level probes for Acidiphilium and Leptospirillum, however, these were not detected, or detected at only small as amounts, in these corroded layers.
Another culture independent method for understanding microbial community composition and function is by metagenomic sequencing. This approach sequences the total genomic component and utilizes bioinformatics tools to directly access the genetic content of the entire community (Thomas et al., 2012). Compared with the 16S rRNA gene method, metagenomics not only reveals the taxonomic diversity of a community, but also allows the detection of functional genes and the compilation of complete and near complete genomes of the microorganisms within a sample. So far only one metagenomics study describing the composition of a concrete corrosion community in sewer crown has been performed (Gomez-Alvarez et al., 2012). The analysis used biofilm samples from the crown of a corroded concrete sewer pipe and showed that the dominant members were from a diverse range of aerobic and facultative anaerobic bacteria that included Acidiphilium, Xanthomonas, and even photosynthetic organisms of the Cyanobacteria, however, no hyper-acidophilic SOMs sequence was found (Gomez-Alvarez et al., 2012). The study highlights that the composition of species involved in concrete corrosion is very site specific.
In the sewer, H2S from the gas phase, will dissolve into the surface water of the corrosion layer to form bisulfide (HS-) and sulfide (S2-) ions. At pH 2–3 most will be in the form of H2S which will undergo chemical and biological oxidations, through a number of electron transfer steps to produce sulfuric acid, see below (Iron Oxidation). The oxidation of reduced sulfur compounds would be the major energy source in the oxidative corrosion layers of the dark sewer, an environment that is low in other inorganic or organic electron donating molecules. Reduced iron is another electron source that may be present mainly due to the reinforcing bar used in the concrete pipe. However, very little energy is gained from the oxidation of both reduced sulfur and reduced iron compounds and these organisms may utilize much energy for carbon fixation for growth (Johnson and Hallberg, 2008). Consequently, while the growth of these chemolithotrophs may be slow, their activity of substrate turnover (S and Fe) may be very high.
In relation to the limited types of electron donors, this would seemingly limit the range of metabolic guilds of microorganisms that proliferate in sewer corrosion layers. However, the diversity of microorganisms of corrosion communities can include chemolithoautotrophs, chemolithoheterotrophs, and chemoorganoheterotrophs (the later often referred to as heterotrophs). We have summarized the important metabolic functions of different acidophilic species found in the sewer corrosion layer of concrete (Table 1). Publications that focus on the detailed roles of acidophiles in the MICC layer are limited. Some functions can be inferred from the rare metagenomic studies of sewer corrosion. Often the microbial functions (presented in Table 1) are inferred from pure culture studies of related microorganisms obtained from other similar acidic environments, such as acid mine drainage and bioleaching (Table 1).
Generally, Acidithiobacillus spp., as chemolithoautotrophs are a keystone group in the corrosion community. This group undertaking activities of sulfur-oxidation (and iron-oxidation for some species), carbon-fixation, production of extracellular polymeric substances (EPS) and the generation of sulfuric acid. The production of EPS would be an important activity that enables the biofilms in the corrosion layer to exist. As a major component of the biofilm matrix it would provide favorable habitat for the EPS producers and for other non-EPS producing microorganisms (Flemming and Wingender, 2010). However, there is diversity in microbial metabolisms in the corrosion layer as suggested by the diversity of microorganisms detected therein (Acidophilic Microorganisms Detected in the Established Corrosion Layer and Table 1). It is estimated that Acidithiobacillus spp. excrete as much as 20% of the CO2 they fix as small organic substances (Karavaiko and Pivovarova, 1973), and these excreted organic molecules can be inhibitory to their own growth (Okabe et al., 2007). Typically, the corrosion layer is expected to be low in organic carbon levels and the molecules excreted by Acidithiobacillus spp. would be important organic carbon source for energy and growth of the heterotrophic members of the microbial communities. Mutualistic relationships between Acidithiobacillus spp. and heterotrophs, that can degrade such inhibitory organic compounds, are proposed (Cho and Mori, 1995; Peccia et al., 2000). Therefore, simple roles for the proposed heterotrophs, such as Mycobacterium spp., Xanthomonadales, Ferroplasma spp., and fungi found in the sewer corrosion communities, would be the degradation of the secreted organic compounds and the production of CO2 (Figure 6). These activities would maintain favorable conditions for the growth of the chemolithoautotrophs, and enable their activities to continue and enhance the sewer corrosion. Some heterotrophs in this environment are also found to have the ability to oxidize H2S or iron. Acidiphilium spp. are sulfur oxidizing heterotrophs that are often detected in corrosion layer communities. Additionally, Acidiphilium are known to reduce ferric iron in anaerobic conditions (Hallberg and Johnson, 2001), although, that activity is likely negligible in the aerobic concrete corrosion layers. Leptospirillum spp. are also detected in corroding concrete. They are common in very acidic, iron-rich environments, and are obligate iron oxidisers (Hallberg and Johnson, 2001). Their presence in this environment may be related to the dissolution of iron bar used for sewer pipe reinforcement.
FIGURE 6. Functions of the acidophilic microbes detected on the corrosion layer. This is an imaginary microbial community that includes representatives of the chemolithoautrophic- Acidithiobacillus and the Chemoorganoheterotrophic- Mycobacterium, Acidiphilium and fungi etc.
The Baas-Becking hypothesis suggests ‘everything is everywhere, but the environment selects’ (De Wit and Bouvier, 2006). The typical low pH (<3) in advanced sewer concrete corrosion layer, accompanied with the limited availability of nutrients and organic carbon, pose a huge selective and evolutionary pressure on microorganisms (Sand and Bock, 1984; Islander et al., 1991; Okabe et al., 2007). To survive in such an environment, certain adaptations and physiological capabilities would be required.
Acidity is a main stress element in the concrete corrosion layer. Acidophilic microorganisms will try to maintain an intracellular pH that is near circumneutral, around pH 6.5–7.5. Many of the cells processes will be sensitive to pH, including those of DNA replication, protein synthesis and various enzyme activities (Baker-Austin and Dopson, 2007). Consequently, there is a large proton gradient across a thin cell envelope, that may be just 50–100 nm thick, and acidophilic microbes must have various adaptations to survive. The cell envelope in these organisms provides the primary and relatively impermeable barrier to protons (Baker-Austin and Dopson, 2007). While acidity is a stress, the large indigenous proton gradient would be utilized by acidophiles to generate ATP using membrane-bound ATPases (Johnson and Hallberg, 2008). However, this ATP production needs to be linked to proton efflux via electron transfer reactions (see Sulfur Oxidation) to avoid cellular acidification.
Modified membranes and membrane components are detected in these acidophiles. The archaea Ferroplasma have highly impermeable membranes composed of tetraether lipids (Batrakov et al., 2002). A. ferrooxidans is seen to reduce the pore size of an outer membrane porin in response increased acidity (Guiliani and Jerez, 2000), a mechanism also proposed to lower membrane permeability to protons. Another modification is to form an external capsule layer. The capsule layer is external to the membrane and consists of homogeneous polysaccharides and proteins (Schleifer and Kandler, 1972; Tuson et al., 2012). It is reported to help maintain cellular integrity and protect the organisms from various damaging external factors such as acidity and high concentrations of toxic heavy metals (Plante, 2000; Gao et al., 2014). A. thiooxidans, is reported to derive protection through the effect of a capsule layer. As the primary barrier to acid stress, this capsule layer is seen to thicken, in response to increasing acidity from pH 1.2–0.8 (Feng et al., 2015).
The oxidation of inorganic sulfur compounds (ISCs) would be the major energy conserving reaction in aerobic sewer corrosion environments. As mentioned above, this environment is comparatively low in other energy rich substrates. The oxidation pathways have been studied in only a few ASOM that are relevant to sewer concrete corrosion. Autotrophic acidophiles of Acidithiobacillus, A. thiooxidans, A. caldus, and A. ferrooxidans can oxidize a range of ISCs that includes H2S, elemental sulfur, tetrathionate (S4O62-), trithionate (S3O62-), and sulfite (SO32-) (Johnson and Hallberg, 2008). The heterotrophic Acidiphilium spp. are also capable of sulfur oxidation in the presence of organic carbon (Hallberg et al., 2001). The current knowledge of ISCs oxidations by sewer acidophiles is drawn from pure culture studies, proven evidence of enzyme activities, and from speculative inferences through bioinformatic genome and metagenome analyses (Mangold et al., 2011; Cárdenas et al., 2016).
Oxidation pathways of acidophiles in sulfur-rich environments have been reviewed recently (Dopson and Johnson, 2012). Various energy conservation pathways for the oxidation of ISCs to SO42- have been detected in sulfur oxidisers in general, and for ASOM, the pathways vary (Johnson and Hallberg, 2008). It is thought that the initial part of the scheme is similar for A. ferrooxidans, A. thiooxidans, and Acidiphilium acidophilum in that H2S and S0 enter the periplasm and are activated by membrane bound thiols to form persulfide groups (Figure 7), such as S-sulfanylglutathione (GSSH) (Rohwerder and Sand, 2003). The persulfide groups can release H2S which is oxidized to S0 and to sulfite by sulfide:quinone oxidoreductase (Sqr) and persulfide dioxygenase (Pdo), respectively. These oxidation steps pass electrons onto the cytoplasmic membrane quinone pool and then to various terminal oxidases to reduce oxygen and produce a membrane proton motive force (Quatrini et al., 2009). In A. ferrooxidans sulfite is then oxidized to sulfate for substrate level phosphorylation that is partly catalyzed by adenylyl-sulfate reductase (Sat) (Figure 7). In addition, an alternate tetrathionate intermediate (S4I) pathway occurs in Acidithiobacillus (Dam et al., 2007). As a central intermediate of H2S oxidation, thiosulfate is metabolized either through the sulfur oxidizing enzyme system (Sox) or the tetrathionate intermediate (S4I) pathway. In the S4I pathway thiosulfate is firstly oxidized into tetrathionate through thiosulfate:quinol oxidoreductase and then tetrathionate is hydrolysed by tetrathionate hydrolase to thiosulfate and other products (Figure 7) (Ghosh and Dam, 2009).
FIGURE 7. A simplified scheme of the main pathways and enzymes involved in the oxidation of inorganic sulfur compounds by ASOM as explained in the text. The enzymes in the pathway lines are: Sqr, sulfide: quinone oxidoreductase; Sor, sulfur oxygenase reductase; Pdo, persulfide dioxygenase; Sat, ATP sulfurylase; Sox, sulfur oxidation complex; Tqo, thiosulfate:quinol oxidoreductase; TetH, tetrathionate hydrolase.
The genome sequence of A. caldus indicates it has some components of ISC metabolism similar to A. ferrooxidans, such as Sqr, truncated Sox, S4I and terminal oxidases (Wang et al., 2016). However, in contrast to A. ferrooxidans, it has genes coding the Sox enzyme complex to catalyze the oxidation of sulfide and thiosulfate to sulfate (Figure 7) (Mangold et al., 2011). Another difference in A. caldus is that S0 is oxidized to sulfite through the disproportionation reaction by sulfur oxygenase reductase (Sor) (Figure 7). The acidophiles, A. thiooxidans and Acidiphilium spp. may be similar to A. caldus, as genome analysis indicates they may also use the Sox pathway for oxidation of ISCs to sulfate (Rohwerder and Sand, 2007; Valdes et al., 2011). In addition, for the S4I pathway, a two-component system RsrS-RsrR has been reported to regulate the tetrathione intermediate pathway for thiosulfate oxidation in A. caldus (Wang et al., 2016).
Mycobacterium are primarily assumed a heterotrophic role in MICC. However, recently an acidophilic Mycobacterium sp. has been observed to oxidize sulfur and produce acid, although, there is no knowledge yet of the pathway involved (Kusumi et al., 2011). Other presumed heterotrophs may also partake in oxidation of ISCs. Ferroplasma spp. are not known for sulfur oxidizing activity (Tyson et al., 2004). However, they have recently been detected in acidic biofilms in sulfide-rich caves, and some genes for sulfur oxidation are found within Ferroplasma genomes such as sulfur oxygenase reductase (Sor) homologs (Jones et al., 2014). Additionally, as already mentioned (see Acidophilic Microorganisms Detected in the Established Corrosion Layer), Xanthomonadales spp., primarily considered as heterotrophs in the sewer corrosion environment, may also have ISC oxidation capabilities (Lee et al., 2007).
In addition to the selection pressure presented by the acidic environment, the expected low level of nutrients in the corrosion layer is also likely to limit microbial growth and activity, and it could be expected that autotrophic microbes will be dominant.
Acting as a predominant producer in the acidic corrosion layer, Acidithiobacillus spp. fix inorganic carbon via the Calvin–Benson–Bassham (CBB) cycle (Calvin and Benson, 1948). Genes coding for enzymes of the CBB cycle are detected in A. ferrooxidans, A. thiooxidans, and A. caldus (Wood et al., 2004; Valdés et al., 2007). As well, activity of the key enzyme, ribulose bisphosphate carboxylase/oxygenase (RUBISCO), has been detected in these organisms (Shively et al., 1986; Esparza et al., 2010; You et al., 2011). In obligate autotrophs, RUBISCO may be packaged into organelles called carboxysomes, particularly during CO2-limiting conditions (Shively et al., 1998). Carboxysomes are considered to enhance CO2-fixation by increasing the concentration of HCO3- in the organelle, and these have been detected in Acidithiobacillus spp. (Shively et al., 1998) and genes coding for carboxysomes are present in A. ferrooxidans (Esparza et al., 2010). For A. ferrooxidans there are three different gene clusters encoding RUBISCO in its genome. It is suggested that these are expressed and utilized in response to different environmental concentrations of CO2 (Yoshizawa et al., 2004). This may be important in sewer biofilms where microbial growth and diffusion restrictions within the corrosion layer could cause localized low levels of CO2 to occur.
In relation to carbon fixation, NAD(P)H, is an important cofactor in that process (Johnson and Hallberg, 2008). In the ASOM, the oxidation of ISCs provides electrons at a redox level high enough to drive NADPH formation directly. This means that the energy driven electron transfers (uphill) that are used by many autotrophs, are not required by various acidophilic sulfur oxidizing autotrophs (Johnson and Hallberg, 2008).
Other microorganisms in the corrosion layer, such as Acidiphilium spp., Mycobacterium spp. and fungi, are described as heterotrophic microorganisms that are scavenging organic compounds (Okabe et al., 2007). As mentioned, Acidithiobacillus spp. are known to produce organic exudates (Harrison, 1984) and these exudates, EPS and debris from dead cells will make up organic components of the biofilm matrix, which will enable growth and activity of the heterotrophic microorganisms therein. In turn the CO2 produced by the heterotrophs will be utilized by the chemolithoautotrophs (Figure 6). Additionally, it is well known that small organic molecules excreted by ASOM can be toxic and inhibit the growth and activity of those bacteria (Norris and Ingledew, 1992; Johnson, 1998). Thus the association of heterotrophic microorganisms is crucial for removal of these inhibitory organic compounds and enable the continued growth of the chemolithoautotrophs (Vincke et al., 2001). The importance of this in sewer corrosion is yet to be determined. There is also the potential that VOCs present in the sewer atmosphere could support the growth of heterotrophic microorganisms on corroding concrete. However, VOC are detected only at low concentrations in the sewer gas (Corsi et al., 1995; Quigley and Corsi, 1995; Chan and Hanaeus, 2006; Wu et al., 2006; Huang et al., 2012; Decottignies et al., 2013; Sivret et al., 2016).
Iron oxidation is considered less important with regard to concrete sewer corrosion as iron is generally in low concentrations in this environment. However, iron species, either in the cement or from the rebar imbedded to reinforce the concrete, become dissolved due to the diffusion of sulfuric acid and could transfer into the pores and micro-cracks of the concrete (Jiang et al., 2014b). Recently it is detected that concrete cracks can be caused by precipitation of iron oxides that occur ahead of the corrosion front. Thus, this activity can be an important part of the initial development of concrete corrosion (Jiang et al., 2014b).
For a few key reasons, microbial iron oxidation becomes more favorable at low pH and likely this occurs in the conditions of the corrosion layer. At low pH ferrous iron is highly soluble and the chemical oxidation of ferrous iron becomes very slow (Stumm and Morgan, 1970). Additionally, in the low pH high sulfate conditions, the redox potential of the Fe2+/Fe3+ couple is lowered and that of the oxygen/water couple is raised. Consequently, at pH 2 the net potential difference for the electron transfer from ferrous iron to oxygen is increased to 420 mV and the reaction is more thermodynamically favorable (Johnson et al., 2012).
Iron oxidizing microbes detected in sewer corrosion include A. ferrooxidans (Hernandez et al., 2002; Yamanaka et al., 2002; Okabe et al., 2007), Ferroplasma spp. (Ling et al., 2015) and Leptospirillum spp. (Okabe et al., 2007), the latter being an obligate iron oxidiser (Harrison, 1984). A. ferrooxidans is the most well studied of all acidophiles with regard to iron oxidation. Through oxidation of ferrous iron to ferric iron in A. ferrooxidans electrons are transferred to reduce oxygen and this utilizes protons within the cell. The membrane proton gradient that is generated is then utilized to transfer protons into the cytoplasm through the ATPase to synthesize ATP (Apel et al., 1980). The blue copper protein rusticyanin, located in the periplasm, is a key participant in the electron transfer process (Blake and Shute, 1994). Based on genetic and biochemical analysis, the model for the electron transfer indicates that, in the periplasm, electrons from ferrous iron oxidation first flow through a high-molecular-weight c-type cytochrome Cyc2 onto the protein rusticyanin. From rusticyanin, electrons then flow through the periplasmic dihemic c-type cytochrome Cyc1, and finally onto an aa3 type cytochrome oxidase on the inner membrane, passing electrons onto O2 which is reduced within the cell (Castelle et al., 2008). This is a well characterized respiratory system of bacterial acidophilic iron oxidation. However, it is seen that other acidophiles possess iron oxidizing mechanisms that are different to the A. ferrooxidans model.
In Leptospirillum spp. a periplasmic heme-containing protein cytochrome 579 is thought to be the key respiratory enzyme for iron oxidation (Ram et al., 2005; Blake and Griff, 2012). This was discovered in a groundbreaking study of an acid mine drainage biofilm using metagenomic and community proteomic analyses (Ram et al., 2005). It is proposed that cyt579 accepts electrons directly from ferrous iron, passing those onto other periplasmic cytochromes and then onto the inner membrane bound cbb3-type cytochrome oxidase for the intracellular reduction of oxygen (Ram et al., 2005). As for the A. ferrooxidans model, intracellular protons would be utilized in the process to maintain the membrane proton motive force. Ferroplasma spp. also use iron oxidation as an energy conservation process (Dopson and Lindström, 2004). Again, through metagenome analysis of acid mine drainage biofilm, a model is proposed for Fe(II) oxidation by Ferroplasma spp. (Allen et al., 2007). The model describes a blue copper sulfocyanin, a member of the protein family to which rusticyanin belongs, that transfers electrons to a cbb3-type terminal oxidase for the intracellular reduction of O2. At this stage details of other cytochromes or electron carriers involved in the respiration by Ferroplasma spp. are yet to be determined (Bonnefoy and Holmes, 2012).
In certain circumstances, it may be the case that acidophilic microorganisms in sewer corrosion are utilizing iron oxidation as the primary mechanism for energetic and growth requirements. Leptospirillum spp. have no other energy conserving capability, and Ferroplasma spp. is not known for sulfur oxidation (Tyson et al., 2004). Additionally, A. ferrooxidans may also perform iron oxidation. In this situation, electronegative potential is required to produce NAD(P)H, which are important reductants in various anabolic reactions of these organisms, including the role of carbon fixation in Leptospirillum spp. and A. ferrooxidans. Consequently, to produce these reducing equivalents, these iron oxidisers are forced to use energy and drive electrons in “uphill” reactions driven by the proton motive force (Bonnefoy and Holmes, 2012). In A. ferrooxidans this “uphill” electron transfer is seen to occur through the reverse functions of inner membrane enzymes, a bc1 complex and an NADH-Q oxidoreductase complex, in an ATP dependent reduction of NAD+ (Elbehti et al., 2000). Interestingly, the reduction of iron (III) is recently reported for some Acidithiobacillus spp. grown aerobically on elemental sulfur and for Acidiphilium cryptum grown micro-aerobically on glucose (Johnson et al., 2017). Although the reduction of iron (III) has not been reported in the oxic sewer corrosion layer, there is potential for this to occur in the iron transformations there.
In the sewer concrete corrosion layers, microbial cells are present in associations that are consistent of biofilm formations. However, to our knowledge, EPS is yet to be explicitly studied directly in these aerobic corrosion layers. There are numerous reports from the similar acid mine drainage environment, where the biofilm state is seen to enhance dissolution of the mineral ore (Sand et al., 2001). Similarly, it may be expected that EPS would provide important attributes for microbial activity and proliferation in the sewer corrosion environment. This could be important for initial attachment of cells from the sewage to the concrete. In the corrosion layer, the acidophiles will grow in favorable positions, i.e., access to substrates, oxygen and H2S and suitable pH (Okabe et al., 2007), with EPS being important for immobilizing cells. It may also provide other features typical of biofilms, such as stable conditions for moisture content and hold cells in close proximity for syntrophic interactions and DNA transfer (Gehrke et al., 2001).
There are few studies that characterize EPS from acidophiles that are known to inhabit sewer corrosion. For adhesion to pyrite and sulfur, A. ferrooxidans is seen to produce EPS that is hydrophobic and composed mostly of lipids and polysaccharides (Gehrke et al., 1998; Gehrke et al., 2001). Similarly, A. thiooxidans produces hydrophobic lipopolysaccharide EPS compounds that are important for its biofilm formation (González et al., 2012). Acidithiobacillus spp. are important components of sewer corrosion and likely their EPS production contributes to their proliferation in that environment. Genes and proteins for EPS production have been detected from Leptospirillum spp. in acid mine drainage biofilms (Ram et al., 2005). There it is suggested that certain members of the mixed culture biofilm are playing the important role of producing the binding matrix. It is seen that various acidophilic fungi including basidiomycetes, filamentous fungi, and yeasts will synthesize EPS (Mahapatra and Banerjee, 2013). Thus, fungi may also play a role for EPS production in the sewer corrosion environment.
Sewer corrosion is universally accepted as a microbial corrosion process. The primary and direct cause is the biological sulfide oxidation by ASOM. While Acidithiobacillus spp. are often predominant in sewer concrete corrosion layers, recent studies using culture-independent methods identify diverse communities from these acidic biofilms that includes species such as Acidiphilium spp., Mycobacterium spp., Xanthomonadales, Ferroplasma spp. and fungi. In aerobic sewer biofilms, the ASOM play an important role in primary production through the oxidation of ISCs to conserve energy that is used for fixing carbon dioxide, for the synthesis of cell components and for production of extracellular materials (EPS). EPS would aid the attachment of the bacteria to the concrete surface and is the major component in microbial biofilms. Heterotrophic bacteria are an essential part of the sewer corrosion communities, as they degrade the extracellular organic molecules to support their growth, which also removes the presence of small organic molecules that may be toxic to ASOM.
Environmental factors such H2S, humidity, temperature, pH, and wastewater flooding play crucial roles on the corrosion process. It is essential that future molecular studies include more details of the environmental conditions that relate to corrosion. This is important so that valuable correlations can be made between the observed ecology and corrosion activity.
The corrosion process and core members of the microorganism community is summarized in this review. There is much interest to limit the activity of MICC to abate the destructive sewer corrosion process. Current control measures either try to lower the H2S concentration in the sewer and/or inhibit the activity of ASOM. To mitigate the generation and partition of H2S, liquid and gas-phase technologies using chemicals such as nitrates or iron salts are applied (Jiang et al., 2015a). Free nitrous acid (FNA), is an antimicrobial agent at ppm level, and is applied to limit the activity of SRB in the liquid phase of the sewer (Jiang et al., 2013) and has also been applied to directly inhibit the activity of ASOM in corrosion layers (Sun et al., 2015). Other technologies include the manufacture of corrosion-resistant concrete through the addition of admixtures, alternative binders or aggregates for new sewers. Additionally, existing sewer surfaces can be coated using epoxy, polymers, and various types of corrosion-resist materials (Bacelar-Nicolau and Johnson, 1999; Hewayde et al., 2007; Haile and Nakhla, 2008; De Muynck et al., 2009). However, it would be important to better understand how robust these applications are, such as the inhibitors and coatings. An improved understanding of how the microbial constituents of the sewer corrosion respond to these control measures is required. For example, this could determine the recovery potential or the survival and resistance of some species to the control measures.
The idea of this review paper was proposed by PB and GJ. The structure and whole paper was written by XL. PB made great contribution on the function of microorgansims. GJ helped with the process of concrete corrosion. UK helped with the details and language in this review paper.
Queensland State Government’s Early Career Accelerate Fellowship, Chinese Scholarship Council.
The authors declare that the research was conducted in the absence of any commercial or financial relationships that could be construed as a potential conflict of interest.
The authors acknowledge the financial support provided by the Australian Research Council and many members of the Australian water industry through LP0882016 and LP150101337. Dr. GJ is the recipient of a Queensland State Government’s Early Career Accelerate Fellowship. XL acknowledge the Chinese Scholarship Council for providing the Living Allowance Scholarship.
Allen, E. E., Tyson, G. W., Whitaker, R. J., Detter, J. C., Richardson, P. M., and Banfield, J. F. (2007). Genome dynamics in a natural archaeal population. Proc. Natl. Acad. Sci. U.S.A. 104, 1883–1888. doi: 10.1073/pnas.0604851104
Apel, W. A., Dugan, P., and Tuttle, J. (1980). Adenosine 5′-triphosphate formation in Thiobacillus ferrooxidans vesicles by H+ ion gradients comparable to those of environmental conditions. J. Bacteriol. 142, 295–301.
Bacelar-Nicolau, P., and Johnson, D. B. (1999). Leaching of pyrite by acidophilic heterotrophic iron-oxidizing bacteria in pure and mixed cultures. Appl. Environ. Microbiol. 65, 585–590.
Baker-Austin, C., and Dopson, M. (2007). Life in acid: pH homeostasis in acidophiles. Trends Microbiol. 15, 165–171. doi: 10.1016/j.tim.2007.02.005
Batrakov, S. G., Pivovarova, T. A., Esipov, S. E., Sheichenko, V. I., and Karavaiko, G. I. (2002). β-D-Glucopyranosyl caldarchaetidylglycerol is the main lipid of the acidophilic, mesophilic, ferrous iron-oxidising archaeon Ferroplasma acidiphilum. Biochim. Biophys. Acta 1581, 29–35. doi: 10.1016/S1388-1981(01)00199-8
Blake, R. C., and Griff, M. N. (2012). In situ spectroscopy on intact Leptospirillum ferrooxidans reveals that reduced cytochrome 579 is an obligatory intermediate in the aerobic iron respiratory chain. Front. Microbiol. 3:136. doi: 10.3389/fmicb.2012.00136
Blake, R. C., and Shute, E. A. (1994). Respiratory enzymes of Thiobacillus ferrooxidans. Kinetic properties of an acid-stable iron: rusticyanin oxidoreductase. Biochemistry 33, 9220–9228. doi: 10.1021/bi00197a025
Bonnefoy, V., and Holmes, D. S. (2012). Genomic insights into microbial iron oxidation and iron uptake strategies in extremely acidic environments. Environ. Microbiol. 14, 1597–1611. doi: 10.1111/j.1462-2920.2011.02626.x
Calvin, M., and Benson, A. A. (1948). The path of carbon in photosynthesis. Science 107, 476–480. doi: 10.1126/science.107.2784.476
Cárdenas, J. P., Quatrini, R., and Holmes, D. S. (2016). Genomic and metagenomic challenges and opportunities for bioleaching: a mini-review. Res. Microbiol. 167, 529–538. doi: 10.1016/j.resmic.2016.06.007
Castelle, C., Guiral, M., Malarte, G., Ledgham, F., Leroy, G., Brugna, M., et al. (2008). A new iron-oxidizing/O2-reducing supercomplex spanning both inner and outer membranes, isolated from the extreme acidophile Acidithiobacillus ferrooxidans. J. Biol. Chem. 283, 25803–25811. doi: 10.1074/jbc.M802496200
Cayford, B. I. (2012). Investigation of the Microbial Community and Processes Responsible for the Corrosion of Concrete in Sewer Systems. Ph.D. thesis, University of Queensland, St Lucia, QLD.
Cayford, B. I., Dennis, P. G., Keller, J., Tyson, G. W., and Bond, P. L. (2012). High-throughput amplicon sequencing reveals distinct communities within a corroding concrete sewer system. Appl. Environ. Microbiol. 78, 7160–7162. doi: 10.1128/AEM.01582-12
Cho, K.-S., and Mori, T. (1995). A newly isolated fungus participates in the corrosion of concrete sewer pipes. Water Sci. Technol. 31, 263–271. doi: 10.1016/0273-1223(95)00343-L
Corsi, R. L., Quigley, C. J., Melcer, H., and Bell, J. (1995). Aromatic VOC emissions from a municipal sewer interceptor. Water Sci. Technol. 31, 137–145. doi: 10.1016/0273-1223(95)00331-G
Dam, B., Mandal, S., Ghosh, W., Gupta, S. K. D., and Roy, P. (2007). The S4-intermediate pathway for the oxidation of thiosulfate by the chemolithoautotroph Tetrathiobacter kashmirensis and inhibition of tetrathionate oxidation by sulfite. Res. Microbiol. 158, 330–338. doi: 10.1016/j.resmic.2006.12.013
Davis, J. L., Nica, D., Shields, K., and Roberts, D. J. (1998). Analysis of concrete from corroded sewer pipe. Int. Biodeterior. Biodegradation 42, 75–84. doi: 10.1016/S0964-8305(98)00049-3
De Muynck, W., De Belie, N., and Verstraete, W. (2009). Effectiveness of admixtures, surface treatments and antimicrobial compounds against biogenic sulfuric acid corrosion of concrete. Cem. Concr. Compos. 31, 163–170. doi: 10.1016/j.cemconcomp.2008.12.004
De Wit, R., and Bouvier, T. (2006). ‘Everything is everywhere, but, the environment selects’; what did Baas Becking and Beijerinck really say? Environ. Microbiol. 8, 755–758. doi: 10.1111/j.1462-2920.2006.01017.x
Decottignies, V., Huyard, A., Kelly, R., and Barillon, B. (2013). Development of a diagnostic tool: the wastewater collection network odour wheel. Water Sci. Technol. 68, 839–847. doi: 10.2166/wst.2013.265
Dopson, M., Baker-Austin, C., Hind, A., Bowman, J. P., and Bond, P. L. (2004). Characterization of Ferroplasma isolates and Ferroplasma acidarmanus sp. nov., extreme acidophiles from acid mine drainage and industrial bioleaching environments. Appl. Environ. Microbiol. 70, 2079–2088. doi: 10.1128/AEM.70.4.2079-2088.2004
Dopson, M., and Johnson, D. B. (2012). Biodiversity, metabolism and applications of acidophilic sulfur-metabolizing microorganisms. Environ. Microbiol. 14, 2620–2631. doi: 10.1111/j.1462-2920.2012.02749.x
Dopson, M., and Lindström, E. (2004). Analysis of community composition during moderately thermophilic bioleaching of pyrite, arsenical pyrite, and chalcopyrite. Microb. Ecol. 48, 19–28. doi: 10.1007/s00248-003-2028-1
Elbehti, A., Brasseur, G., and Lemesle-Meunier, D. (2000). First evidence for existence of an uphill electron transfer through the bc(1) and NADH-Q oxidoreductase complexes of the acidophilic obligate chemolithotrophic ferrous ion-oxidizing Bacterium Thiobacillus ferrooxidans. J. Bacteriol. 182, 3602–3606. doi: 10.1128/JB.182.12.3602-3606.2000
Esparza, M., Cárdenas, J. P., Bowien, B., Jedlicki, E., and Holmes, D. S. (2010). Genes and pathways for CO2 fixation in the obligate, chemolithoautotrophic acidophile, Acidithiobacillus ferrooxidans, carbon fixation in A. ferrooxidans. BMC Microbiol. 10:1. doi: 10.1186/1471-2180-10-229
Feng, S., Yang, H., and Wang, W. (2015). System-level understanding of the potential acid-tolerance components of Acidithiobacillus thiooxidans ZJJN-3 under extreme acid stress. Extremophiles 19, 1029–1039. doi: 10.1007/s00792-015-0780-z
Flemming, H.-C., and Wingender, J. (2010). The biofilm matrix. Nat. Rev. Microbiol. 8, 623–633. doi: 10.1038/nrmicro2415
Gao, S., Lewis, G. D., Ashokkumar, M., and Hemar, Y. (2014). Inactivation of microorganisms by low-frequency high-power ultrasound: 1. Effect of growth phase and capsule properties of the bacteria. Ultrason. Sonochem. 21, 446–453. doi: 10.1016/j.ultsonch.2013.06.006
Gehrke, T., Hallmann, R., Kinzler, K., and Sand, W. (2001). The EPS of Acidithiobacillus ferrooxidans-a model for structure-function relationships of attached bacteria and their physiology. Water Sci. Technol. 43, 159–167.
Gehrke, T., Telegdi, J., Thierry, D., and Sand, W. (1998). Importance of extracellular polymeric substances from Thiobacillus ferrooxidans for bioleaching. Appl. Environ. Microbiol. 64, 2743–2747.
Ghosh, W., and Dam, B. (2009). Biochemistry and molecular biology of lithotrophic sulfur oxidation by taxonomically and ecologically diverse bacteria and archaea. FEMS Microbiol. Rev. 33, 999–1043. doi: 10.1111/j.1574-6976.2009.00187.x
Gomez-Alvarez, V., Revetta, R. P., and Santo Domingo, J. W. (2012). Metagenome analyses of corroded concrete wastewater pipe biofilms reveal a complex microbial system. BMC Microbiol. 12:122. doi: 10.1186/1471-2180-12-122
González, D. M., Lara, R. H., Alvarado, K. N., Valdez-Pérez, D., Navarro-Contreras, H. R., Cruz, R., et al. (2012). Evolution of biofilms during the colonization process of pyrite by Acidithiobacillus thiooxidans. Appl. Microbiol. Biotechnol. 93, 763–775. doi: 10.1007/s00253-011-3465-2
Gu, J.-D., Ford, T. E., Berke, N. S., and Mitchell, R. (1998). Biodeterioration of concrete by the fungus Fusarium. Int. Biodeterior. Biodegradation 41, 101–109. doi: 10.1016/S0964-8305(98)00034-1
Guiliani, N., and Jerez, C. A. (2000). Molecular cloning, sequencing, and expression of omp-40, the gene coding for the major outer membrane protein from the acidophilic Bacterium Thiobacillus ferrooxidans. Appl. Environ. Microbiol. 66, 2318–2324. doi: 10.1128/AEM.66.6.2318-2324.2000
Gutiérrez-Padilla, M. G. D., Bielefeldt, A., Ovtchinnikov, S., Hernandez, M., and Silverstein, J. (2010). Biogenic sulfuric acid attack on different types of commercially produced concrete sewer pipes. Cem. Concr. Res. 40, 293–301. doi: 10.1016/j.cemconres.2009.10.002
Haile, T., and Nakhla, G. (2008). A novel zeolite coating for protection of concrete sewers from biological sulfuric acid attack. Geomicrobiol. J. 25, 322–331. doi: 10.1080/01490450802258428
Hallberg, K. B., and Johnson, D. B. (2001). Biodiversity of acidophilic prokaryotes. Adv. Appl. Microbiol. 49, 37–84. doi: 10.1016/S0065-2164(01)49009-5
Hallberg, K. B., Thomson, H. E., Boeselt, I., and Johnson, D. B. (2001). Aerobic and anaerobic sulfur metabolism by acidophilic bacteria. Process Metall. 11, 423–432.
Harrison, A. P. Jr. (1984). The acidophilic thiobacilli and other acidophilic bacteria that share their habitat. Annu. Rev. Microbiol. 38, 265–292. doi: 10.1146/annurev.mi.38.100184.001405
Hernandez, M., Marchand, E. A., Roberts, D., and Peccia, J. (2002). In situ assessment of active Thiobacillus species in corroding concrete sewers using fluorescent RNA probes. Int. Biodeterior. Biodegradation 49, 271–276. doi: 10.1016/S0964-8305(02)00054-9
Hewayde, E. H., Nakhla, G. F., Allouche, E. N., and Mohan, P. K. (2007). Beneficial impact of coatings on biological generation of sulfide in concrete sewer pipes. Struct. Infrastruct. Eng. 3, 267–277. doi: 10.1080/15732470500424260
Hou, S., Makarova, K. S., Saw, J. H., Senin, P., Ly, B. V., Zhou, Z., et al. (2008). Complete genome sequence of the extremely acidophilic methanotroph isolate V4, Methylacidiphilum infernorum, a representative of the bacterial phylum Verrucomicrobia. Biol. Direct 3:26. doi: 10.1186/1745-6150-3-26
Huang, C.-H., Chen, K.-S., and Wang, H.-K. (2012). Measurements and PCA/APCS analyses of volatile organic compounds in Kaohsiung municipal sewer systems, southern Taiwan. Aerosol Air Qual. Res. 12, 1315–1326. doi: 10.4209/aaqr.2012.02.0035
Hugenholtz, P., and Tyson, G. W. (2008). Microbiology: metagenomics. Nature 455, 481–483. doi: 10.1038/455481a
Hvitved-Jacobsen, T., Vollertsen, J., and Nielsen, A. H. (2013). Sewer Processes: Microbial and Chemical Process Engineering of Sewer Networks. Boca Raton, FL: CRC press. doi: 10.1201/b14666
Islander, R. L., Devinny, J. S., Mansfeld, F., Postyn, A., and Shih, H. (1991). Microbial ecology of crown corrosion in sewers. J. Environ. Eng. 117, 751–770. doi: 10.1061/(ASCE)0733-9372(1991)117:6(751)
Jiang, G., Keating, A., Corrie, S., O’halloran, K., Nguyen, L., and Yuan, Z. (2013). Dosing free nitrous acid for sulfide control in sewers: results of field trials in Australia. Water Res. 47, 4331–4339. doi: 10.1016/j.watres.2013.05.024
Jiang, G., Keller, J., and Bond, P. L. (2014a). Determining the long-term effects of H2S concentration, relative humidity and air temperature on concrete sewer corrosion. Water Res. 65, 157–169. doi: 10.1016/j.watres.2014.07.026
Jiang, G., Sun, J., Sharma, K. R., and Yuan, Z. (2015a). Corrosion and odor management in sewer systems. Curr. Opin. Biotechnol. 33, 192–197. doi: 10.1016/j.copbio.2015.03.007
Jiang, G., Sun, X., Keller, J., and Bond, P. L. (2015b). Identification of controlling factors for the initiation of corrosion of fresh concrete sewers. Water Res. 80, 30–40. doi: 10.1016/j.watres.2015.04.015
Jiang, G., Wightman, E., Donose, B. C., Yuan, Z., Bond, P. L., and Keller, J. (2014b). The role of iron in sulfide induced corrosion of sewer concrete. Water Res. 49, 166–174. doi: 10.1016/j.watres.2013.11.007
Jiang, G., Zhou, M., Chiu, T. H., Sun, X., Keller, J., and Bond, P. L. (2016). Wastewater enhanced microbial corrosion of concrete sewers. Environ. Sci. Technol. 50, 8084–8092. doi: 10.1021/acs.est.6b02093
Johnson, D. B. (1998). Biodiversity and ecology of acidophilic microorganisms. FEMS Microbiol. Ecol. 27, 307–317. doi: 10.1111/j.1574-6941.1998.tb00547.x
Johnson, D. B., and Hallberg, K. B. (2008). Carbon, iron and sulfur metabolism in acidophilic micro-organisms. Adv. Microb. Physiol. 54, 201–255. doi: 10.1016/S0065-2911(08)00003-9
Johnson, D. B., Hedrich, S., and Pakostova, E. (2017). Indirect redox transformations of iron, copper, and chromium catalyzed by extremely acidophilic bacteria. Front. Microbiol. 8:211. doi: 10.3389/fmicb.2017.00211
Johnson, D. B., Kanao, T., and Hedrich, S. (2012). Redox transformations of iron at extremely low pH: fundamental and applied aspects. Front. Microbiol. 3:96. doi: 10.3389/fmicb.2012.00096
Jones, D. S., Schaperdoth, I., and Macalady, J. L. (2014). Metagenomic evidence for sulfide oxidation in extremely acidic cave biofilms. Geomicrobiol. J. 31, 194–204. doi: 10.1080/01490451.2013.834008
Joseph, A. P., Keller, J., Bustamante, H., and Bond, P. L. (2012). Surface neutralization and H2S oxidation at early stages of sewer corrosion: influence of temperature, relative humidity and H2S concentration. Water Res. 46, 4235–4245. doi: 10.1016/j.watres.2012.05.011
Karavaiko, G., and Pivovarova, T. (1973). Oxidation of elementary sulfur by Thiobacillus thiooxidans. Mikrobiologiia 42, 389–395.
Keller, M., and Zengler, K. (2004). Tapping into microbial diversity. Nat. Rev. Microbiol. 2, 141–150. doi: 10.1038/nrmicro819
Kelly, D. P., and Wood, A. P. (2000). Reclassification of some species of Thiobacillus to the newly designated genera Acidithiobacillus gen. nov., Halothiobacillus gen. nov. and Thermithiobacillus gen. nov. Int. J. Syst. Evol. Microbiol. 50, 511–516. doi: 10.1099/00207713-50-2-511
Kusumi, A., Li, X. S., and Katayama, Y. (2011). Mycobacteria isolated from Angkor monument sandstones grow chemolithoautotrophically by oxidizing elemental sulfur. Front. Microbiol. 2:104. doi: 10.3389/fmicb.2011.00104
Lee, C. S., Kim, K. K., Aslam, Z., and Lee, S.-T. (2007). Rhodanobacter thiooxydans sp. nov., isolated from a biofilm on sulfur particles used in an autotrophic denitrification process. Int. J. Syst. Evol. Microbiol. 57, 1775–1779. doi: 10.1099/ijs.0.65086-0
Ling, A. L. (2013). Characterization and Control of Microbially Induced Concrete Corrosion. Ph.D. thesis, University of Colorado at Boulder, Boulder, CO.
Ling, A. L., Robertson, C. E., Harris, J. K., Frank, D. N., Kotter, C. V., Stevens, M. J., et al. (2015). High-resolution microbial community succession of microbially induced concrete corrosion in working sanitary manholes. PLoS ONE 10:e0116400. doi: 10.1371/journal.pone.0116400
Maeda, T., Negishi, A., Komoto, H., Oshima, Y., Kamimura, K., and Sugio, T. (1999). Isolation of iron-oxidizing bacteria from corroded concretes of sewage treatment plants. J. Biosci. Bioeng. 88, 300–305. doi: 10.1016/S1389-1723(00)80013-4
Mahapatra, S., and Banerjee, D. (2013). Fungal exopolysaccharide: production, composition and applications. Microbiol. Insights 6, 1–16. doi: 10.4137/MBI.S10957
Mangold, S., Valdés, J., Holmes, D. S., and Dopson, M. (2011). Sulfur metabolism in the extreme acidophile Acidithiobacillus caldus. Front. Microbiol. 2:17. doi: 10.3389/fmicb.2011.00017
Mild, K., Sand, W., Wolff, W., and Bock, E. (1983). Thiobacilli of the corroded concrete walls of the hamburg sewer system. J. Gen. Microbiol. 129, 1327–1333. doi: 10.1099/00221287-129-5-1327
Monteny, J., Vincke, E., Beeldens, A., De Belie, N., Taerwe, L., Van Gemert, D., et al. (2000). Chemical, microbiological, and in situ test methods for biogenic sulfuric acid corrosion of concrete. Cem. Concr. Res. 30, 623–634. doi: 10.1016/S0008-8846(00)00219-2
Mori, T., Nonaka, T., Tazaki, K., Koga, M., Hikosaka, Y., and Noda, S. (1992). Interactions of nutrients, moisture and pH on microbial corrosion of concrete sewer pipes. Water Res. 26, 29–37. doi: 10.1016/0043-1354(92)90107-F
Nica, D., Davis, J. L., Kirby, L., Zuo, G., and Roberts, D. J. (2000). Isolation and characterization of microorganisms involved in the biodeterioration of concrete in sewers. Int. Biodeterior. Biodegradation 46, 61–68. doi: 10.1016/S0964-8305(00)00064-0
Norris, P., and Ingledew, W. (1992). “Acidophilic bacteria: adaptations and applications,” in Molecular Biology and Biotechnology of Extremophiles, eds R. A. Herbert and R. J. Sharp (Glasgow: Blackie), 115–142. doi: 10.1007/978-94-011-2274-0_4
O’Dea, V. (2007). Understanding biogenic sulfide corrosion. Mater. Perform. 46, 36–39. doi: 10.1016/j.copbio.2010.12.005
Okabe, S., Odagiri, M., Ito, T., and Satoh, H. (2007). Succession of sulfur-oxidizing bacteria in the microbial community on corroding concrete in sewer systems. Appl. Environ. Microbiol. 73, 971–980. doi: 10.1128/AEM.02054-06
Olmstead, W., and Hamlin, H. (1900). Converting portions of the Los Angeles outfall sewer into a septic tank. Eng. News 44, 317–318.
Pagaling, E., Yang, K., and Yan, T. (2014). Pyrosequencing reveals correlations between extremely acidophilic bacterial communities with hydrogen sulphide concentrations, pH and inert polymer coatings at concrete sewer crown surfaces. J. Appl. Microbiol. 117, 50–64. doi: 10.1111/jam.12491
Parker, C. D. (1945a). The corrosion of concrete 2. The function of Thiobacillus concretivorus (nov. spec.) in the corrosion of concrete exposed to atmospheres containing hydrogen sulphide. Aust. J. Exp. Biol. Med. Sci. 23, 91–98. doi: 10.1038/icb.1945.14
Parker, C. D. (1945b). The corrosion of concrete. Aust. J. Exp. Biol. Med. 23, 81–90. doi: 10.1038/icb.1945.13
Parker, C. T. (1947). Species of sulphur bacteria associated with the corrosion of concrete. Nature 159, 439–440. doi: 10.1038/159439b0
Peccia, J., Marchand, E. A., Silverstein, J., and Hernandez, M. (2000). Development and application of small-subunit rRNA probes for assessment of selected Thiobacillus species and members of the genus Acidiphilium. Appl. Environ. Microbiol. 66, 3065–3072. doi: 10.1128/AEM.66.7.3065-3072.2000
Pikaar, I., Sharma, K. R., Hu, S., Gernjak, W., Keller, J., and Yuan, Z. (2014). Reducing sewer corrosion through integrated urban water management. Science 345, 812–814. doi: 10.1126/science.1251418
Plante, C. J. (2000). Role of bacterial exopolymeric capsules in protection from deposit-feeder digestion. Aquat. Microb. Ecol. 21, 211–219. doi: 10.3354/ame021211
Quatrini, R., Appia-Ayme, C., Denis, Y., Jedlicki, E., Holmes, D. S., and Bonnefoy, V. (2009). Extending the models for iron and sulfur oxidation in the extreme acidophile Acidithiobacillus ferrooxidans. BMC Genomics 10:394. doi: 10.1186/1471-2164-10-394
Quigley, C. J., and Corsi, R. L. (1995). Emissions of VOCs from a municipal sewer. J. Air Waste Manag. Assoc. 45, 395–403. doi: 10.1080/10473289.1995.10467371
Ram, R. J., Verberkmoes, N. C., Thelen, M. P., Tyson, G. W., Baker, B. J., Blake, R. C., et al. (2005). Community proteomics of a natural microbial biofilm. Science 308, 1915–1920. doi: 10.1126/science.1109070
Roberts, D., Nica, D., Zuo, G., and Davis, J. (2002). Quantifying microbially induced deterioration of concrete: initial studies. Int. Biodeterior. Biodegradation 49, 227–234. doi: 10.1016/S0964-8305(02)00049-5
Rohwerder, T., and Sand, W. (2003). The sulfane sulfur of persulfides is the actual substrate of the sulfur-oxidizing enzymes from Acidithiobacillus and Acidiphilium spp. Microbiology 149, 1699–1710. doi: 10.1099/mic.0.26212-0
Rohwerder, T., and Sand, W. (2007). Oxidation of inorganic sulfur compounds in acidophilic prokaryotes. Eng. Life Sci. 7, 301–309. doi: 10.1002/elsc.200720204
Sand, W., and Bock, E. (1984). Concrete corrosion in the Hamburg sewer system. Environ. Technol. 5, 517–528. doi: 10.1080/09593338409384307
Sand, W., Gehrke, T., Jozsa, P.-G., and Schippers, A. (2001). Biochemistry of bacterial leaching—direct vs. indirect bioleaching. Hydrometallurgy 59, 159–175. doi: 10.1016/S0304-386X(00)00180-8
Santo Domingo, J. W., Revetta, R. P., Iker, B., Gomez-Alvarez, V., Garcia, J., Sullivan, J., et al. (2011). Molecular survey of concrete sewer biofilm microbial communities. Biofouling 27, 993–1001. doi: 10.1080/08927014.2011.618637
Satoh, H., Odagiri, M., Ito, T., and Okabe, S. (2009). Microbial community structures and in situ sulfate-reducing and sulfur-oxidizing activities in biofilms developed on mortar specimens in a corroded sewer system. Water Res. 43, 4729–4739. doi: 10.1016/j.watres.2009.07.035
Schleifer, K. H., and Kandler, O. (1972). Peptidoglycan types of bacterial cell walls and their taxonomic implications. Bacteriol. Rev. 36, 407–477.
Shively, J., Devore, W., Stratford, L., Porter, L., Medlin, L., and Stevens, S. (1986). Molecular evolution of the large subunit of ribulose-1, 5-bisphosphate carboxylase/oxygenase (RuBisCO). FEMS Microbiol. Lett. 37, 251–257. doi: 10.1111/j.1574-6968.1986.tb01804.x
Shively, J. M., Van Keulen, G., and Meijer, W. G. (1998). Something from almost nothing: carbon dioxide fixation in chemoautotrophs. Annu. Rev. Microbiol. 52, 191–230. doi: 10.1146/annurev.micro.52.1.191
Sivret, E. C., Wang, B., Parcsi, G., and Stuetz, R. M. (2016). Prioritisation of odorants emitted from sewers using odour activity values. Water Res. 88, 308–321. doi: 10.1016/j.watres.2015.10.020
Staley, J. T., and Konopka, A. (1985). Measurement of in situ activities of nonphotosynthetic microorganisms in aquatic and terrestrial habitats. Annu. Rev. Microbiol. 39, 321–346. doi: 10.1146/annurev.mi.39.100185.001541
Stumm, W., and Morgan, J. J. (1970). Aquatic Chemistry; An Introduction Emphasizing Chemical Equilibria in Natural Waters. London: Wiley-Interscience.
Sun, X., Jiang, G., Bond, P. L., Keller, J., and Yuan, Z. (2015). A novel and simple treatment for control of sulfide induced sewer concrete corrosion using free nitrous acid. Water Res. 70, 279–287. doi: 10.1016/j.watres.2014.12.020
Thomas, T., Gilbert, J., and Meyer, F. (2012). Metagenomics - a guide from sampling to data analysis. Microb. Inform. Exp. 2:3. doi: 10.1186/2042-5783-2-3
Tuson, H. H., Auer, G. K., Renner, L. D., Hasebe, M., Tropini, C., Salick, M., et al. (2012). Measuring the stiffness of bacterial cells from growth rates in hydrogels of tunable elasticity. Mol. Microbiol. 84, 874–891. doi: 10.1111/j.1365-2958.2012.08063.x
Tyson, G. W., Chapman, J., Hugenholtz, P., Allen, E. E., Ram, R. J., Richardson, P. M., et al. (2004). Community structure and metabolism through reconstruction of microbial genomes from the environment. Nature 428, 37–43. doi: 10.1038/nature02340
Valdes, J., Ossandon, F., Quatrini, R., Dopson, M., and Holmes, D. S. (2011). Draft genome sequence of the extremely acidophilic biomining bacterium Acidithiobacillus thiooxidans ATCC 19377 provides insights into the evolution of the Acidithiobacillus genus. J. Bacteriol. 193, 7003–7004. doi: 10.1128/JB.06281-11
Valdés, J. H., Pedroso, I., Quatrini, R., Hallberg, K. B., Valenzuela, P. D., and Holmes, D. S. (2007). Insights into the metabolism and ecophysiology of three Acidithiobacilli by comparative genome analysis. Adv. Mater. Res. 20–21, 439–442. doi: 10.4028/www.scientific.net/AMR.20-21.439
Vincke, E., Boon, N., and Verstraete, W. (2001). Analysis of the microbial communities on corroded concrete sewer pipes–a case study. Appl. Microbiol. Biotechnol. 57, 776–785. doi: 10.1007/s002530100826
Wang, Z.-B., Li, Y.-Q., Lin, J.-Q., Pang, X., Liu, X.-M., Liu, B.-Q., et al. (2016). The two-component system RsrS-RsrR regulates the tetrathionate intermediate pathway for thiosulfate oxidation in Acidithiobacillus caldus. Front. Microbiol. 7:1755. doi: 10.3389/fmicb.2016.01755
Ward, D. M., Weller, R., and Bateson, M. M. (1990). 16S rRNA sequences reveal numerous uncultured microorganisms in a natural community. Nature 345, 63–65. doi: 10.1038/345063a0
Ward, M., Corsi, R., Morton, R., Knapp, T., Apgar, D., Quigley, C., et al. (2011). Characterization of natural ventilation in wastewater collection systems. Water Environ. Res. 83, 265–273. doi: 10.2175/106143010X12780288628859
Watanabe, T., Kojima, H., and Fukui, M. (2015). Sulfuriferula multivorans gen. nov., sp. nov., isolated from a freshwater lake, reclassification of ‘Thiobacillus plumbophilus’ as Sulfuriferula plumbophilus sp. nov., and description of Sulfuricellaceae fam. nov. and Sulfuricellales ord. nov. Int. J. Syst. Evol. Microbiol. 65, 1504–1508. doi: 10.1099/ijs.0.000129
Wells, T., Melchers, R., Joseph, A., Bond, P., Vitanage, D., Bustamante, H., et al. (2012). “A collaborative investigation of the microbial corrosion of concrete sewer pipe in Australia,” in Proceedings of OzWater-12 Australia’s National Water Conference and Exhibition, Sydney, NSW, 8–10.
Wells, T., and Melchers, R. E. (2015). Modelling concrete deterioration in sewers using theory and field observations. Cem. Concr. Res. 77, 82–96. doi: 10.1016/j.cemconres.2015.07.003
Wood, A. P., Aurikko, J. P., and Kelly, D. P. (2004). A challenge for 21st century molecular biology and biochemistry: what are the causes of obligate autotrophy and methanotrophy? FEMS Microbiol. Rev. 28, 335–352. doi: 10.1016/j.femsre.2003.12.001
Wu, B.-Z., Feng, T.-Z., Sree, U., Chiu, K.-H., and Lo, J.-G. (2006). Sampling and analysis of volatile organics emitted from wastewater treatment plant and drain system of an industrial science park. Anal. Chim. Acta 576, 100–111. doi: 10.1016/j.aca.2006.03.057
Yamanaka, T., Aso, I., Togashi, S., Tanigawa, M., Shoji, K., Watanabe, T., et al. (2002). Corrosion by bacteria of concrete in sewerage systems and inhibitory effects of formates on their growth. Water Res. 36, 2636–2642. doi: 10.1016/S0043-1354(01)00473-0
Yoshizawa, Y., Toyoda, K., Arai, H., Ishii, M., and Igarashi, Y. (2004). CO2-responsive expression and gene organization of three ribulose-1, 5-bisphosphate carboxylase/oxygenase enzymes and carboxysomes in Hydrogenovibrio marinus strain MH-110. J. Bacteriol. 186, 5685–5691. doi: 10.1128/JB.186.17.5685-5691.2004
You, X.-Y., Guo, X., Zheng, H.-J., Zhang, M.-J., Liu, L.-J., Zhu, Y.-Q., et al. (2011). Unraveling the Acidithiobacillus caldus complete genome and its central metabolisms for carbon assimilation. J. Genet. Genomics 38, 243–252. doi: 10.1016/j.jgg.2011.04.006
Keywords: sewer, concrete corrosion, acidophile, Acidithiobacillus thiooxidans, Acidiphilium
Citation: Li X, Kappler U, Jiang G and Bond PL (2017) The Ecology of Acidophilic Microorganisms in the Corroding Concrete Sewer Environment. Front. Microbiol. 8:683. doi: 10.3389/fmicb.2017.00683
Received: 03 January 2017; Accepted: 04 April 2017;
Published: 20 April 2017.
Edited by:
Axel Schippers, Federal Institute for Geosciences and Natural Resources, GermanyReviewed by:
Wolfgang Sand, University of Duisburg-Essen, GermanyCopyright © 2017 Li, Kappler, Jiang and Bond. This is an open-access article distributed under the terms of the Creative Commons Attribution License (CC BY). The use, distribution or reproduction in other forums is permitted, provided the original author(s) or licensor are credited and that the original publication in this journal is cited, in accordance with accepted academic practice. No use, distribution or reproduction is permitted which does not comply with these terms.
*Correspondence: Guangming Jiang, Zy5qaWFuZ0Bhd21jLnVxLmVkdS5hdQ== Philip L. Bond, cGhpbC5ib25kQGF3bWMudXEuZWR1LmF1
Disclaimer: All claims expressed in this article are solely those of the authors and do not necessarily represent those of their affiliated organizations, or those of the publisher, the editors and the reviewers. Any product that may be evaluated in this article or claim that may be made by its manufacturer is not guaranteed or endorsed by the publisher.
Research integrity at Frontiers
Learn more about the work of our research integrity team to safeguard the quality of each article we publish.