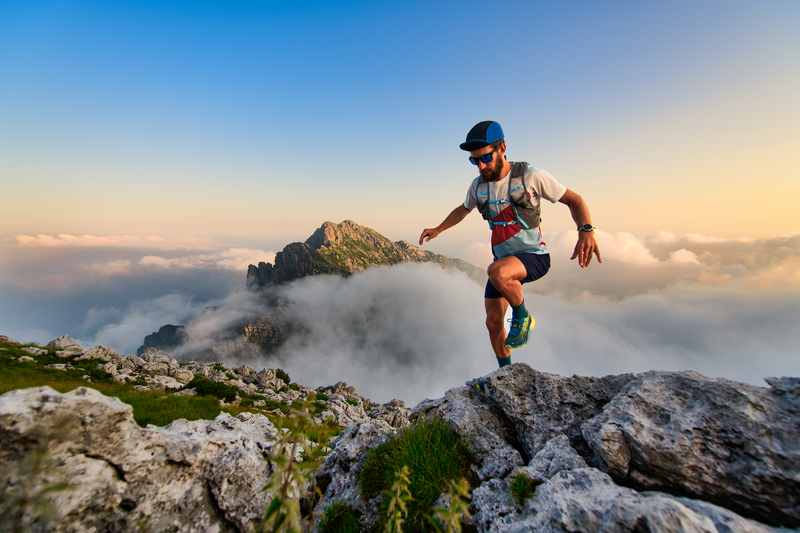
95% of researchers rate our articles as excellent or good
Learn more about the work of our research integrity team to safeguard the quality of each article we publish.
Find out more
ORIGINAL RESEARCH article
Front. Microbiol. , 18 January 2017
Sec. Antimicrobials, Resistance and Chemotherapy
Volume 8 - 2017 | https://doi.org/10.3389/fmicb.2017.00024
The mechanisms for the development and spread of antibacterial resistance (ABR) in bacteria residing in environmental compartments, including the marine environment, are far from understood. The objective of this study was to examine the ABR rates in Escherichia coli and other Enterobacteriaceae isolates obtained from marine bivalve mollusks collected along the Norwegian coast during a period from October 2014 to November 2015. A total of 549 bivalve samples were examined by a five times three tube most probable number method for enumeration of E. coli in bivalves resulting in 199 isolates from the positive samples. These isolates were identified by biochemical reactions and matrix Assisted Laser Desorption Ionization-Time of Flight Mass Spectrometry, showing that 90% were E. coli, while the remaining were species within the genera Klebsiella, Citrobacter, and Enterobacter. All 199 isolates recovered were susceptibility tested following the European Committee on Antimicrobial Susceptibility Testing disk diffusion method. In total, 75 of 199 (38%) isolates showed resistance to at least one antibacterial agent, while multidrug-resistance were seen in 9 (5%) isolates. One isolate conferred resistance toward 15 antibacterial agents. Among the 75 resistant isolates, resistance toward extended-spectrum penicillins (83%), aminoglycosides (16%), trimethoprim (13%), sulfonamides (11%), tetracyclines (8%), third-generation cephalosporins (7%), amphenicols (5%), nitrofurans (5%), and quinolones (5%), were observed. Whole-genome sequencing on a selection of 10 E. coli isolates identified the genes responsible for resistance, including blaCTX-M genes. To indicate the potential for horizontal gene transfer, conjugation experiments were performed on the same selected isolates. Conjugative transfer of resistance was observed for six of the 10 E. coli isolates. In order to compare E. coli isolates from bivalves with clinical strains, multiple-locus variable number tandem repeats analysis (MLVA) was applied on a selection of 30 resistant E. coli isolates. The MLVA-profiles were associated with community-acquired E. coli strains causing bacteremia. Our study indicates that bivalves represent an important tool for monitoring antibacterial resistant E. coli and other members of the Enterobacteriaceae family in the coastal environment.
The development of antibacterial resistance (ABR) is a natural process and ancient among bacteria (Aminov and Mackie, 2007; D’Costa et al., 2011). However, the current global use of antibacterial agents in human and veterinary medicine, as well as in agriculture, are a driving force for ABR development and also increase the release of these substances to the environment (Davies and Davies, 2010).
The intestines of humans and other homeothermic animals are colonized by a dense and diverse microbiota belonging to, among others, the Enterobacteriaceae family (Tancrède, 1992; Dethlefsen et al., 2006). The predominant genus within this family is Escherichia, with Escherichia coli being the main species. E. coli occurs naturally in the large intestine of humans, birds, and terrestrial and marine mammals (Welch, 2006). Most E. coli of the large intestine of humans and other homeothermic animals are commensal strains, however opportunistic and pathogenic strains may be present (Strockbine et al., 2015). E. coli cause morbidity and mortality as a result of common infections, including enteritis, meningitis, urinary tract, or bloodstream infections (Strockbine et al., 2015). The main sources of infections with pathogenic E. coli are consumption of contaminated water and food, as well as through animal contact (ILSI, 2011).
Antibacterial treatments are known to substantially affect the normal intestinal microbiota favoring resistant strains (Sommer and Dantas, 2011). The prevalence of resistant E. coli and other bacteria in the intestinal microbiota of humans are shown to be strongly correlated with the use of antibacterial agents (Murray et al., 1982; Bruinsma et al., 2003; van der Veen et al., 2009).
The microbiological communities in coastal environments can be influenced by sewage and runoff from land, concomitantly containing both fecal bacteria as well as residues of antibacterial substances (Martinez, 2009; Alves et al., 2014; Balière et al., 2015). A significant proportion of the antimicrobial agents are excreted unchanged and in a biologically active form (Dolliver and Gupta, 2008; Gillings, 2013; Michael et al., 2013). During periods with heavy rainfall, increased amount of fecal material from land living animals will reach the sea. In addition, high precipitation could cause an overload and possible leakage from sewage systems. Sewage and manure harbor bacteria of high diversity, have a high concentration of organic substances, as well as anthropogenic pollution as heavy metals and antimicrobial agents, which in combination can favor bacterial growth and promote spread of genetic elements through horizontal gene transfer (Moura et al., 2010; Heuer et al., 2011). Bacteria conferring ABR colonizing the intestines of humans and other homeothermic animals, may contribute to the dissemination of antibiotic resistant bacteria (ABR-B) via sewage to the marine environment (Poeta et al., 2005; Penders et al., 2013). The survival of these bacteria in aquatic environments are affected by both abiotic and biotic factors, e.g., nutrient availability, osmotic stress, variations in temperature and pH, and predation (Barcina et al., 1997; Rozen and Belkin, 2001; Campos et al., 2013). Importantly, E. coli have the ability to persist in the aquatic habitat due to its genetic flexibility (van Elsas et al., 2011).
The presence of Enterobacteriaceae conferring resistance to antibacterial agents in coastal waters may represent a human health issue, especially in areas used for marine food production or recreational activities (Murugaiyan et al., 2015). Multidrug-resistant (MDR) bacteria have been detected in coastal waters, and could result in the transmission of resistance among marine and contaminating bacteria via exchange of genetic elements, such as plasmids (Wright, 2010; Alves et al., 2014; Moura et al., 2014).
Bivalve mollusks are invertebrates that have an external two-part hinged shell that contains the soft parts. Typical bivalve mollusks comprise among others clams, oysters, mussels, and scallops. As these mollusks are suspension feeders, they actively filter, retain, and concentrates particles from their surrounding water, including free living or particle-bound bacteria (Bernard, 1989; Leff et al., 1992; Maugeri et al., 2004). Bivalve associated members of the Enterobacteriaceae family, may originate from humans and other homeothermic animals either via sewage, by runoff from land, or from representatives of the wild fauna such as birds or marine mammals (Bogomolni et al., 2008). These bivalves are therefore excellent indicators for fecal contamination and will reflect the load of E. coli and other bacteria in the Enterobacteriaceae family present in the water column at a given location. However, different environmental conditions, e.g., temperature, water flow rate, and food availability, can affect the filtration rate, consequently also the accumulation of fecal bacteria (Šolić et al., 1999; Strohmeier et al., 2012; Campos et al., 2013).
Bivalve mollusks are good candidate for studies on resistance in bacteria originating from several sources including humans and animals, and gives the possibility of comparing temporal and spatial changes and the potential for exposure to humans by consumption of marine bivalves. The main objective of this study was to examine the ABR rates in Enterobacteriaceae isolates obtained from marine bivalve mollusks collected along the Norwegian coast. In addition, an assessment of the transferability of certain resistance genes, as well as comparing bivalve isolates with clinical isolates of human origin, was performed.
As part of the mandatory EU surveillance program (854/2004/EC, 2004) conducted by the Norwegian Food Safety Authority (NFSA), sampling of bivalve mollusks were performed from 57 localities covering the Norwegian coast on several occasions from October 2014 to November 2015. A standardized most probable number (MPN) reference method for enumeration of E. coli in bivalves (Oblinger and Koburger, 1975), with Minerals Modified Glutamate Broth (MMGB) (Oxoid, UK) as growth media in combination with verification on Tryptone Bile with X-glucuronide (TBX) agar (Oxoid, UK) (Donovan et al., 1998), was performed as described in Grevskott et al. (2016). A total of 549 bivalves were collected and examined at the National Institute of Nutrition and Seafood Research and the Norwegian Institute of Public Health, as presented in Grevskott et al. (2016). More than a half of the bivalve samples (51%) was harvested from commercially active rearing localities, while the rest were collected from positions established by NFSA for long time reference monitoring purposes of shellfish safety. A total number of 199 bacterial isolates, one from each randomly selected culture-positive bivalve sample (n = 335), was grown into pure culture for further analysis.
The bacterial isolates were susceptibility tested by disk diffusion on Mueller-Hinton (MH) agar (Oxoid, UK) according to the European Committee on Antimicrobial Susceptibility Testing (EUCAST) (Matuschek et al., 2014). Each bacterial isolate was tested for 24 antibacterial agents, representing 10 drug classes (WHOCC Server, 2016). The following disks (Oxoid, UK) were applied: ampicillin (10 μg), amoxicillin (10 μg), amoxicillin/clavulanic acid (2/1 μg), mecillinam (10 μg), piperacillin/tazobactam (30/6 μg), chloramphenicol (30 μg), ciprofloxacin (5 μg), levofloxacin (5 μg), nalidixic acid (30 μg), norfloxacin (10 μg), nitrofurantoin (100 μg), gentamicin (10 μg), tobramycin (10 μg), streptomycin (25 μg), kanamycin (30 μg), trimethoprim (5 μg), trimethoprim/sulfamethoxazole (1.25/23.75 μg), cefotaxime (5 μg), ceftazidime (10 μg), doxycycline (30 μg), tetracycline (30 μg), colistin sulfate (25 μg), imipenem (10 μg), and meropenem (10 μg). To monitor the quality for each new batch of MH agar, and antibacterial disks, E. coli CCUG 17620 was included on a regular basis. The inhibition zones were interpreted according to the EUCAST clinical breakpoint tables v.6.0 (EUCAST, 2016). For some substances breakpoints were not available and for these substances clinical breakpoints given by Clinical and Laboratory Standards Institute (CLSI, 2014) or Indian Council of Medical Research (ICMR, 2009), were used.
A selection of 10 isolates was subjected to whole-genome sequencing (WGS). The isolates were selected on the basis of phenotypes showing resistance toward multiple antibacterial agents and/or expressing resistance to critically important agents, such as to third-generation cephalosporins. DNA was isolated by the use of the MagNA Pure 96 DNA and Viral NA Small Volume Kit and a MagNApure 96 instrument (Roche Diagnostics, Germany). The sequencing libraries were prepared using the Kapa HyperPLus Library Preparation Kit (Kapa Biosystems, USA). The isolates were sequenced on an Illumina MiSeq platform (Illumina, USA), producing (2 bp × 250 bp) paired-end reads. The data were adaptor and quality trimmed using Trimmomatic (Bolger et al., 2014), and assembled using SPAdes (Bankevich et al., 2012). The processed sequence data were analyzed for genes encoding resistance to antimicrobial resistance using the web-based ResFinder tool (Zankari et al., 2012), for serotype using the SerotypeFinder tool (Joensen et al., 2015) and for multi-locus sequence types (MLSTs) using the MLSTs tool (Larsen et al., 2012) from Centre for Genomic Epidemiology1, at the Technical University of Denmark.
The whole-genome sequenced strains were subjected to conjugation experiments in order to investigate the ability of self-transfer of resistance properties to susceptible recipient strains. The 10 donor isolates were mated with one of the two sensitive recipient strains, E. coli DH5α (Culture Collection, University of Göteborg, Sweden) and One Shot E. coli (Thermo Fisher, USA). Eight of the donor E. coli isolates were susceptible to quinolones, and were conjugated with E. coli DH5α resistant to nalidixic acid, as recipient. Two of the donor E. coli isolates were resistant to quinolones, but susceptible to kanamycin, and were therefore conjugated with One Shot E. coli resistant to kanamycin, as recipient. The conjugal transfer was conducted in a Luria-Bertani (LB) broth (Sigma-Aldrich, USA) and the mating was prepared as previously described by Sunde and Sørum (2001). The transconjugant was selected as described by Sunde and Norström (2006), by applying antibacterial disks corresponding to the resistance profile of the donors (Oxoid, UK; Rosco, Denmark) onto the surface of the MH agar plates (BD, USA), with 20 μg/ml nalidixic acid (N-8878 Sigma-Aldrich, USA) or 50 μg/ml kanamycin (K4000 Sigma-Aldrich, USA). The obtained transconjugants were subcultured for inspection of colony morphology as previously described (Sunde and Norström, 2006) and subsequently subjected to susceptibility testing by disk diffusion.
Based on resistance profile, 30 of the 199 isolates were selected for multiple-locus variable number tandem repeats analysis (MLVA). Extraction of DNA was done by dissolving bacterial cells in 350 μl sterile, distilled water (Fresenius Kabi, Germany) and boiling at 100°C for approximately 15 min. Extracted DNA was mixed with reagents from Qiagen Multiplex PCR kit (Qiagen, Germany). The PCR mixture consisted of 12.5 μl of 2x Master mix, 0.5 μl of primer mix and 11 μl of sterile water. Four different primer mixes were used for each DNA sample: EC-5, EC-6, CVN002 and EC-12, where 1 μl extracted DNA was added to the PCR mixtures, to a total volume of 25 μl. The PCR mixtures were placed in the GeneAmp® PCR System 9700 machine (Applied-Biosystems, USA) followed by capillary electrophoresis on an ABI 3130xl Genetic Analyzer (Applied-Biosystems, USA), as described by Løbersli et al. (2012). A control DNA sample (GJ57) was measured along with the unknown DNA samples for quality assurance.
From the MLVA-profiles of the 30 bivalve E. coli isolates, the allele numbers generated were entered into BioNumerics database version 7.6 (Applied Maths, Belgium) as character values, and an analysis based minimal spanning tree (MST) clustering was constructed. As markers of genetic relationships, we included 212 community-acquired E. coli bacteremia isolates, 38 other human strains from the E. coli Reference (ECOR)-collection obtained from the Microbial Evolutionary Laboratory (State University of Michigan, USA), four Enterohemorrhagic E. coli (EHEC) strains associated with hemorrhagic uremic syndrome (HUS) from the strain collection at the Norwegian Institute of Public Health, as described (Wester et al., 2013, 2014). The community-acquired E. coli isolates causing blood stream infection (BSI) were classified as non-severe, early organ failure (≥organs affected within 1 day of admittance to hospital), or in-hospital death within 14 days of admission (Wester et al., 2013). We applied MST for categorical data, with one-locus difference as first priority rule (weight 10,000), and two-loci difference as second priority rule (weight 10).
The majority of the bacterial isolates (90%) were identified as E. coli, both by Analytical Profile Index 20E (Oxoid, UK) and by Matrix Assisted Laser Desorption Ionization-Time of Flight Mass Spectrometry (Bruker, Germany). The remaining isolates (10%) belonged to the three genera Klebsiella, Citrobacter, and Enterobacter.
A total of 75 (38%) of the 199 isolates showed resistance to at least one antibacterial agent, while multidrug-resistance was seen in nine (5%) of the isolates (Figure 1), using the definition by Magiorakos et al. (2012). Among the 75 resistant isolates, resistance toward extended-spectrum penicillins (83%), aminoglycosides (16%), trimethoprim (13%), sulfonamides (11%), tetracyclines (8%), third-generation cephalosporins (7%), amphenicols (5%), nitrofurans (5%), and quinolones (5%), were observed. Amoxicillin-resistance was found in 59 (79%) isolates, while ampicillin-resistance was found in 36 (48%) isolates. The two E. coli isolates B177 and B184 showed phenotypic resistance against nine and 15 antibacterial agents, respectively.
FIGURE 1. Number of E. coli and other bacteria in the Enterobacteriaceae family showing phenotypic resistance to antibacterial agents applied in accordance with the EUCAST, CLSI, and ICMR clinical breakpoint tables. The two E. coli isolates B177 and B184 (marked by arrows) displayed resistance against nine or more antibacterial agents.
Among the 10 bacterial isolates subjected to WGS, eight sequence types (STs) were identified. Two isolates belonged to ST-95, and two isolates belonged to ST-58, the remaining six isolates belonged to ST-10, ST-38, ST-69, ST-88, ST-191, or ST-3572, respectively.
Multiple resistance genes were present as examined by ResFinder (Table 1). Resistance toward extended-spectrum penicillins was observed in all 10 E. coli isolates and they all harbored the blaTEM-1 gene. Isolate B117 and B184 were resistant to third-generation cephalosporins, and carried the blaCTX-M-15 and blaCTX-M-14 genes, respectively. Six isolates possessed genes conferring resistance to aminoglycosides, while five isolates carried genes for resistance against trimethoprim, sulfonamides, and tetracyclines. A gene conferring resistance against amphenicols was observed in two isolates. Two isolates had genes conferring resistance toward quinolones and macrolides, respectively. Notably, three isolates harbored resistance genes (strA-strB, catA1, and qnrS1, respectively) which did not correspond to the phenotypic resistance pattern.
TABLE 1. Distribution of sequence type (ST), resistance genes, and serotype among 10 Escherichia coli isolates by WGS.
Six of 10 E. coli isolates transferred resistance genes by conjugation (Table 2). The three bacterial isolates B2, B158, and B165 transferred trimethoprim- and sulfamethoxazole-resistance, two isolates (B160 and B167) transferred tetracycline-resistance, while one isolate (B117) transferred resistance to cefotaxime and ceftazidime. The resistance patterns of transconjugants were examined by the EUCAST disk diffusion method, in which only a selection of antibacterial agents were employed as determined by the resistance profile of the donor.
A total of 284 strains were included and MLVA-profiles matching nine specific loci were regarded as phylogenetic related (Figure 2). The ECOR strains of different phylogroups and E. coli isolates causing BSI did not cluster, nor showed to be located in any specific branch of the MST, except from strains belonging to phylogroup A. The 30 E. coli isolates from bivalves seemed to be evenly distributed throughout the MST, together with both the bacteremia E. coli and the ECOR strains and the HUS-associated EHEC strains.
FIGURE 2. Minimal spanning tree showing the phylogenetic relationships between 212 E. coli isolated from blood stream infection (BSI) according to patient outcome (non-severe, 3failure = organ failure ≥3 organs within one day of admission to hospital, death14d = death within 14 days of admittance to hospital), 38 ECOR strains of human origin with main phylogroup, 30 E. coli isolates from bivalves, and four HUS-associated EHEC strains. The distance between circles are indicated by the thickness and dotting of lines, hence a thicker line indicate a closer relation than a thin line, and a thin line indicate a closer relation than a dotted line. Shared MLVA-profiles are shown as shared circles.
Antibacterial resistant fecal bacteria from animals or humans may spread among the human population by direct contact, or via water and food. The transfer of ABR-B in the food production chain may affect the development and spread of resistance among the foodborne pathogens (Sørum and L′Abée-Lund, 2002; VKM, 2015). This could also apply for seafood. Contaminated seafood as fish, bivalves, and crustaceans may cause ABR-B from both marine and fecal origins to reach humans during handling and consumption. A possible risk of transmission of ABR-B may occur from unintentional improper heat treatment, or through bivalves intended for raw- or light preserved consumption. Especially, flat oysters (Ostrea edulis) and great scallops (Pecten maximus) represents a risk, as they are commonly consumed raw. If these food products are consumed without proper heat treatment, resistant bacteria may enter the consumer and subsequently interact with the intestinal microbiota (Sullivan et al., 2001).
In this study, two E. coli isolates displayed phenotypic resistance toward as many as nine or more antibacterial agents, indicating a potential risk of exposure to MDR Enterobacteriaceae during consumption or handling of marine bivalves. In addition, extended spectrum beta-lactamase (ESBL)-producing E. coli isolates were identified from this food source (Table 1). Among the European countries, Norway has the lowest production corrected use of antimicrobial agents in animals (EMA, 2016). Furthermore, as reported in the Norwegian monitoring program for antimicrobial resistance in human pathogens, and in bacteria from food, feed and animals (NORM/NORM-VET, 2015), Norway is a low prevalence country in terms of antimicrobial resistance and it is therefore surprising to detect a high rate of resistant Enterobacteriaceae in marine bivalves, including the ESBL-producing E. coli strains. Notably, this should be taken into account in order to determine if bivalves should be included in annual monitoring of ABR in the coastal environment.
The majority of resistant isolates (n = 75) examined in the current work were resistant to the extended-spectrum penicillins ampicillin and/or amoxicillin (83%) (Figure 1), which is interesting since the use of antimicrobial agents in Norway is dominated by narrow-spectrum penicillins (NORM/NORM-VET, 2015). However, an increase in the use of penicillins with extended spectrum have been reported lately (NORM/NORM-VET, 2015). The increased use of ampicillin and amoxicillin in humans and/or food-producing animals may have led to the development of resistance within the bacterial species observed in this study. Moreover, it is well-known that the blaTEM-1 gene conferring resistance against extended-spectrum penicillins has been widely distributed in bacterial populations for decades (Hedges et al., 1974). All 10 E. coli isolates subjected to in-depth characterization by WGS harbored the blaTEM-1 gene, whereas two isolates had blaCTX-M genes, the latter conferring ESBL-production (Table 1). The various TEM enzymes are mutant derivatives of plasmid-mediated beta-lactamases conferring resistance to penicillins, while the CTX-M enzymes confer resistance to penicillins and cephalosporins and have their origin in environmental bacteria (Cantón et al., 2012). The CTX-M enzymes have become the most prevalent ESBLs in bacteria causing human infection, both in hospital and in community settings (Cantón and Coque, 2006; Cantón et al., 2008). The presence of ESBL-positive E. coli is of great concern due to possible lack of therapeutic success in the treatment of serious infections, hence defined as critically important by the World Health Organization [WHO] (2014). ESBL-positive E. coli have also been recovered from food products for human consumption, as well as from wildlife (Li et al., 2007; Smet et al., 2010; Guenther et al., 2011). A fraction of the bacterial isolates were resistant to aminoglycosides (16%), and six of the 10 sequenced E. coli isolates harbored resistance genes. Resistance toward trimethoprim and sulfonamides were seen in 13 and 11% of the isolates, respectively, and five of the 10 sequenced E. coli isolates harbored genes conferring resistance toward trimethoprim and sulfonamides. All isolates expressing resistance to trimethoprim and sulfonamides contained genes responsible for the resistance phenotype, except isolates B53 and B160. This indicates that resistance among the bacterial isolates could be a result of selection by increased use, since these agents are synthetic and thus not commonly found in the natural environment. However, observations of resistance toward quinolones and sulfonamides have been seen in the intestinal microbiota of an 11th Century pre-Columbian Andean mummy, showing that resistance even to some synthetic agents may date back to Ancient times (Santiago-Rodriguez et al., 2015).
Among the 10 E. coli isolates subjected to conjugation experiments, transferable resistance was detected in six isolates (Table 2). The transfer of genes conferring resistance toward third-generation cephalosporins (cefotaxime and ceftazidime) are especially alarming, since the spread of these genes to clinically relevant E. coli strains will dramatically reduce the possible choice of antibacterial agents for medical treatment. Moreover, transfer of multiple resistance genes may occur with a higher frequency when the bacteria are exposed to antibacterial agents. ABR among, e.g., enteric bacteria may form reservoirs, in which resistance determinants could transfer to non-resistant bacteria, including those responsible for diseases (Salyers et al., 2004; Stecher et al., 2012). Intestinal bacteria from the human microbiota may, in addition to sharing resistance genes among themselves, also exchange resistance genes to other bacteria that are temporary passing through the intestine (Teuber et al., 1999; Salyers et al., 2004). Thus, commensal bacteria may function as a vector in transferring resistance genes between environmental and pathogenic bacteria.
Whole-genome sequencing and subsequent analysis showed that two isolates belonged to ST-95, while two isolates belonged to ST-38 and to ST-69, respectively (Table 1). These STs are associated with bacteremia and urinary tract infection in humans (Adams-Sapper et al., 2012; Alghoribi et al., 2015; Hertz et al., 2016). The MLVA-profiles of the bivalve E. coli isolates displayed a seemingly high degree of diversity (Figure 2). Furthermore, they scattered among BSI-causing, including those leading to death within 14 days of admission to hospital, as well as among representatives of all E. coli main phylogroups. Both instances indicate no common source, but also that the bacteria have the potential for causing serious infection in humans. Consequently, the presence of pathogenic E. coli isolates in the coastal environment represent a risk to human health, especially in areas use for aquaculture or recreational activities. This is supported by the findings of Balière et al. (2015) who reported that a few E. coli strains of EHEC and Enteropathogenic E. coli (EPEC) isolated from bivalve mollusks harbored resistance toward amoxicillin, cefotaxime, and imipenem. The World Health Organization [WHO] (2014) have stated that infections with E. coli strains, e.g., EHEC and EPEC, are among the most frequent foodborne causative agents worldwide.
Allochthonous bacteria from different sources (e.g., urban, industrial, and agriculture waste), and residues of antimicrobial agents, will ultimately be transported to the marine environment through waste water effluents, rivers, or streams, and mixed with the indigenous bacterial population (Baquero et al., 2008; Wellington et al., 2013). This can result in the rise of resistance due to selection pressure, and/or genetic exchange between environmental and intestinal bacteria. Bivalves may promote gene transfer among bacteria in the marine environment, by collecting bacteria from various sources and concentrate them within a stable micro-environment at a high density (Taylor et al., 2011). The increasing pressure exerted by antimicrobial agents affects the acquisition, selection, and transmission of resistance determinants among a wide range of bacteria.
Our study indicates that marine bivalves may represent an important tool for monitoring antibacterial resistant E. coli and other members of the Enterobacteriaceae family in coastal environments. Bivalves may furthermore act as a “hot spot” for resistance transfer between Enterobacteriaceae and indigenous bacteria, as the conditions they offer may facilitate the conjugational frequency. As continuous EU programs for the detection of E. coli from bivalves are currently implemented, an additional characterization of their ABR profile would represent a good cross-compartment added value indicator of spatial and temporal trends in resistance rates.
BL, CS, and DG designed the experimental set up and DG, MS, and AW performed the experiments. DG, CS, MS, AW, and BL wrote the manuscript. All authors agree to be accountable for the content of the work and gave final approval to the manuscript.
The funding of the work described in this research was supported by the participants involved in this publication.
The authors declare that the research was conducted in the absence of any commercial or financial relationships that could be construed as a potential conflict of interest.
The authors would like to thank Betty Irgens, Leikny Fjeldstad, and Tone Galluzzi at the National Institute of Nutrition and Seafood Research, and Irene Rauk, Marit Hindrum, Gina Ilaug Guldahl, Nadia Debech, and Ingerid Kirkeleite at the Norwegian Institute of Public Health for excellent technical help. We also want to thank the NFSA for providing the samples.
854/2004/EC (2004). Regulation (EC) No. 854/2004 of the European Parliament and of the Council laying down specific rules for the organisation of official controls on products of animal origin intended for human consumption. Official J. Eur. Union L 139 854/2004, 83–127.
Adams-Sapper, S., Diep, B. A., Perdreau-Remington, F., and Riley, L. W. (2012). Clonal composition and community clustering of drug-susceptible and resistant Escherichia coli isolates from blood stream infections. J. Antimicrob. Agents Chemother. 57, 490–497. doi: 10.1128/AAC.01025-12
Alghoribi, M. F., Gibreel, T. M., Farnham, G., Al Johani, S. M., Balkhy, H. H., and Upton, M. (2015). Antibiotic-resistant ST38, ST131 and ST405 strains are the leading uropathogenic Escherichia coli clones in Riyadh, Saudi Arabia. J. Antimicrob. Chemother. 70, 1–6. doi: 10.1093/jac/dkv188
Alves, M. S., Pereira, A., Araújo, S. M., Castro, B. B., Correia, A., and Henriques, I. (2014). Seawater is a reservoir of multi-resistant Escherichia coli, including strains hosting plasmid-mediated quinolones resistance and extended-spectrum beta-lactamases genes. Front. Microbiol. 5:426. doi: 10.3389/fmicb.2014.00426
Aminov, R. I., and Mackie, R. I. (2007). Evolution and ecology of antibiotic resistance genes. FEMS Microbiol. Lett. 271, 147–161. doi: 10.1111/j.1574-6968.2007.00757.x
Balière, C., Rincé, A., Blanco, J., Dahbi, G., Harel, J., Vogeleer, P., et al. (2015). Prevalence and characterization of shiga toxin-producing and enteropathogenic Escherichia coli in shellfish-harvesting areas and their watersheds. Front. Microbiol. 6:1356. doi: 10.3389/fmicb.2015.01356
Bankevich, A., Nurk, S., Antipov, D., Gurevich, A. A., Dvorkin, M., Kulikov, A. S., et al. (2012). SPAdes: a new genome assembly algorithm and its applications to single-cell sequencing. J. Comput. Biol. 19, 455–477. doi: 10.1089/cmb.2012.0021
Baquero, F., Martínez, J.-L., and Cantón, R. (2008). Antibiotics and antibiotic resistance in water environments. Curr. Opin. Biotechnol. 19, 260–265. doi: 10.1016/j.copbio.2008.05.006
Barcina, I., Lebaron, P., and Vives-Rego, J. (1997). Survival of allochthonous bacteria in aquatic systems: a biological approach. FEMS Microbiol. Ecol. 23, 1–9. doi: 10.1111/j.1574-6941.1997.tb00385.x
Bernard, F. (1989). Uptake and elimination of coliform bacteria by four marine bivalve mollusks. Can. J. Fish. Aquat. Sci. 46, 1592–1599. doi: 10.1139/f89-203
Bogomolni, A. L., Gast, R. J., Ellis, J. C., Dennett, M. R., Pugliares, K. R., Lentell, B. J., et al. (2008). Victims or vectors: a survey of marine vertebrate zoonoses from coastal waters of the Northwest Atlantic. Dis. Aquat. Org. 81, 13–38. doi: 10.3354/dao01936
Bolger, A. M., Lohse, M., and Usadel, B. (2014). Trimmomatic: a flexible trimmer for Illumina sequence data. Bioinformatics 30, 2114–2120. doi: 10.1093/bioinformatics/btu170
Bruinsma, N., Stobberingh, E., De Smet, P., and Van Den Bogaard, A. (2003). Antibiotic use and the prevalence of antibiotic resistance in bacteria from healthy volunteers in the Dutch community. Infection 31, 9–14. doi: 10.1007/s15010-002-3035-8
Campos, C. J., Kershaw, S. R., and Lee, R. J. (2013). Environmental influences on faecal indicator organisms in coastal waters and their accumulation in bivalve shellfish. Estuaries Coast 36, 834–853. doi: 10.1007/s12237-013-9599-y
Cantón, R., and Coque, T. M. (2006). The CTX-M β-lactamase pandemic. Curr. Opin. Microbiol. 9, 466–475. doi: 10.1016/j.mib.2006.08.011
Cantón, R., González-Alba, J. M., and Galán, J. C. (2012). CTX-M enzymes: origin and diffusion. Front. Microbiol. 3:110. doi: 10.3389/fmicb.2012.00110
Cantón, R., Novais, A., Valverde, A., Machado, E., Peixe, L., Baquero, F., et al. (2008). Prevalence and spread of extended-spectrum β-lactamase-producing Enterobacteriaceae in Europe. Clin. Microbiol. Infect. 14(Suppl. 1), 144–153. doi: 10.1111/j.1469-0691.2007.01850.x
CLSI (2014). Performance Standards for Antimicrobial Susceptibility Testing; Twenty-Fourth Informational Supplement. Clinical and Laboratory Standards Institute (CLSI). CLSI document M100-S24 (ISBN 1-56238-897-5 [Print]; ISBN 1-56238-898-3 [Electronic]). Wayne, PA: Clinical and Laboratory Standards Institute.
Davies, J., and Davies, D. (2010). Origins and evolution of antibiotic resistance. Microbiol. Mol. Biol. Rev. 74, 417–433. doi: 10.1128/MMBR.00016-10
D’Costa, V. M., King, C. E., Kalan, L., Morar, M., Sung, W. W., Schwarz, C., et al. (2011). Antibiotic resistance is ancient. Nature 477, 457–461. doi: 10.1038/nature10388
Dethlefsen, L., Eckburg, P. B., Bik, E. M., and Relman, D. A. (2006). Assembly of the human intestinal microbiota. Trends Ecol. Evol. 21, 517–523. doi: 10.1016/j.tree.2006.06.013
Dolliver, H., and Gupta, S. (2008). Antibiotic losses in leaching and surface runoff from manure-amended agricultural land. J. Environ. Qual. 37, 1227–1237. doi: 10.2134/jeq2007.0392
Donovan, T., Gallacher, S., Andrews, N., Greenwood, M., Graham, J., Russell, J., et al. (1998). Modificiation of the standard method used in the United Kingdom for counting Escherichia coli in live bivalve molluscs. Commun. Dis. Public Health 1, 188–196.
EMA (2016). European Medicines Agency, European Surveillance of Veterinary Antimicrobial Consumption, 2016. ’Sales of veterinary antimicrobial agents in 29 European countries in 2014’. (EMA/61769/2016). London: European Medicines Agency, 35.
EUCAST (2016). Breakpoint Tables for Interpretation of MICs and Zone Diameters. Version 6.0. [Online]. European Committee on Antimicrobial Susceptibility Testing (EUCAST). Available at: http://www.eucast.org/fileadmin/src/media/PDFs/EUCAST_files/Breakpoint_tables/v_6.0_Breakpoint_table.pdf [accessed January 1, 2016]
Gillings, M. (2013). Evolutionary consequences of antibiotic use for the resistome, mobilome and microbial pangenome. Front. Microbiol. 4:4. doi: 10.3389/fmicb.2013.00004
Grevskott, D. H., Svanevik, C. S., Wester, A. L., and Lunestad, B. T. (2016). The species accuracy of the most probable number (MPN) European Union reference method for enumeration of Escherichia coli in marine bivalves. J. Microbiol. Methods 131, 73–77. doi: 10.1016/j.mimet.2016.10.006
Guenther, S., Ewers, C., and Wieler, L. (2011). Extended-spectrum beta-lactamases producing E. coli in Wildlife, yet Another Form of Environmental Pollution?. Front. Microbiol. 2:246. doi: 10.3389/fmicb.2011.00246
Hedges, R., Datta, N., Kontomichalou, P., and Smith, J. (1974). Molecular specificities of R factor-determined beta-lactamases: correlation with plasmid compatibility. J. Bacteriol. 117, 56–62.
Hertz, F. B., Nielsen, J. B., Schønning, K., Littauer, P., Knudsen, J. D., Løbner-Olesen, A., et al. (2016). Population structure of drug-susceptible,-resistant and ESBL-producing Escherichia coli from community-acquired urinary tract. BMC Microbiol. 16:63. doi: 10.1186/s12866-016-0681-z
Heuer, H., Schmitt, H., and Smalla, K. (2011). Antibiotic resistance gene spread due to manure application on agricultural fields. Curr. Opin. Microbiol. 14, 236–243. doi: 10.1016/j.mib.2011.04.009
ICMR (2009). Detection of Antimicrobial Resistance in Common Gram-Negative and Gram-Positive Bacteria Encountered in Infectious Diseases-an Update. New Delhi: Indian Council of Medical Research (ICMR), 7.
ILSI (2011). The Enterobacteriaceae and Their Significance to the Food Industry. Brussel: Internation Life Sciences Institute (ILSI), 1–29.
Joensen, K. G., Tetzschner, A. M., Iguchi, A., Aarestrup, F. M., and Scheutz, F. (2015). Rapid and easy in silico serotyping of Escherichia coli using whole genome sequencing (WGS) data. J. Clin. Microbiol. 54, 1–41. doi: 10.1128/JCM.00008-15
Larsen, M. V., Cosentino, S., Rasmussen, S., Friis, C., Hasman, H., Marvig, R. L., et al. (2012). Multilocus sequence typing of total genome sequenced bacteria. J. Clin. Microbiol. 50, 1355–1361. doi: 10.1128/JCM.06094-11
Leff, L. G., McArthur, J. V., and Shimkets, L. J. (1992). Information spiraling: movement of bacteria and their genes in streams. Microb. Ecol. 24, 11–24. doi: 10.1007/BF00171967
Li, X.-Z., Mehrotra, M., Ghimire, S., and Adewoye, L. (2007). β-Lactam resistance and β-lactamases in bacteria of animal origin. Vet. Microbiol. 121, 197–214. doi: 10.1016/j.vetmic.2007.01.015
Løbersli, I., Haugum, K., and Lindstedt, B.-A. (2012). Rapid and high resolution genotyping of all Escherichia coli serotypes using 10 genomic repeat-containing loci. J. Microbiol. Methods 88, 134–139. doi: 10.1016/j.mimet.2011.11.003
Magiorakos, A.-P., Srinivasan, A., Carey, R., Carmeli, Y., Falagas, M., Giske, C., et al. (2012). Multidrug-resistant, extensively drug-resistant and pandrug-resistant bacteria: an international expert proposal for interim standard definitions for acquired resistance. Clin. Microbiol. Infect. 18, 268–281. doi: 10.1111/j.1469-0691.2011.03570.x
Martinez, J. L. (2009). Environmental pollution by antibiotics and by antibiotic resistance determinants. Environ. Pollut. 157, 2893–2902. doi: 10.1016/j.envpol.2009.05.051
Matuschek, E., Brown, D. F. J., and Kahlmeter, G. (2014). Development of the EUCAST disk diffusion antimicrobial susceptibility testing method and its implementation in routine microbiology laboratories. Clin. Microbiol. Infect. 20, O255–O266. doi: 10.1111/1469-0691.12373
Maugeri, T., Carbone, M., Fera, M., Irrera, G., and Gugliandolo, C. (2004). Distribution of potentially pathogenic bacteria as free living and plankton associated in a marine coastal zone. J. Appl. Microbiol. 97, 354–361. doi: 10.1111/j.1365-2672.2004.02303.x
Michael, I., Rizzo, L., McArdell, C., Manaia, C., Merlin, C., Schwartz, T., et al. (2013). Urban wastewater treatment plants as hotspots for the release of antibiotics in the environment: a review. Water Res. 47, 957–995. doi: 10.1016/j.watres.2012.11.027
Moura, A., Araújo, S., Alves, M. S., Henriques, I., Pereira, A., and Correia, A. C. (2014). The contribution of Escherichia coli from human and animal sources to the integron gene pool in coastal waters. Front. Microbiol. 5:419. doi: 10.3389/fmicb.2014.00419
Moura, A., Henriques, I., Smalla, K., and Correia, A. (2010). Wastewater bacterial communities bring together broad-host range plasmids, integrons and a wide diversity of uncharacterized gene cassettes. Res. Microbiol. 161, 58–66. doi: 10.1016/j.resmic.2009.11.004
Murray, B. E., Rensimer, E. R., and DuPont, H. L. (1982). Emergence of high-level trimethoprim resistance in fecal Escherichia coli during oral administration of trimethoprim or trimethoprim-sulfamethoxazole. Engl. J. Med. 306, 130–135. doi: 10.1056/NEJM198201213060302
Murugaiyan, J., Krueger, K., Roesler, U., Weinreich, J., and Schierack, P. (2015). Assessment of species and antimicrobial resistance among Enterobacteriaceae isolated from mallard duck faeces. Environ. Monit. Assess. 187, 1–11. doi: 10.1007/s10661-015-4346-4
NORM/NORM-VET (2015). Usage of Antimicrobial Agents and Occurrence of Antimicrobial Resistance in Norway. Tromsø: Norwegian Veterinary Institute.
Oblinger, J. L., and Koburger, J. A. (1975). Understanding and teaching the most probable number technique. J. Food Microbiol. 38, 540–545.
Penders, J., Stobberingh, E., Savelkoul, P., and Wolffs, P. (2013). The human microbiome as a reservoir of antimicrobial resistance. Front. Microbiol. 4:87. doi: 10.3389/fmicb.2013.00087
Poeta, P., Costa, D., Sáenz, Y., Klibi, N., Ruiz-Larrea, F., Rodrigues, J., et al. (2005). Characterization of antibiotic resistance genes and virulence factors in faecal enterococci of wild animals in Portugal. J. Vet. Med. 52, 396–402. doi: 10.1111/j.1439-0450.2005.00881.x
Rozen, Y., and Belkin, S. (2001). Survival of enteric bacteria in seawater. FEMS Microbiol. Rev. 25, 513–529. doi: 10.1111/j.1574-6976.2001.tb00589.x
Salyers, A. A., Gupta, A., and Wang, Y. (2004). Human intestinal bacteria as reservoirs for antibiotic resistance genes. Trends Microbiol. 12, 412–416. doi: 10.1016/j.tim.2004.07.004
Santiago-Rodriguez, T. M., Fornaciari, G., Luciani, S., Dowd, S. E., Toranzos, G. A., Marota, I., et al. (2015). Gut microbiome of an 11th century A.D. pre-columbian andean mummy. PLoS ONE 10:e138135. doi: 10.1371/journal.pone.0138135
Smet, A., Martel, A., Persoons, D., Dewulf, J., Heyndrickx, M., Herman, L., et al. (2010). Broad-spectrum β-lactamases among Enterobacteriaceae of animal origin: molecular aspects, mobility and impact on public health. FEMS Microbiol. Rev. 34, 295–316. doi: 10.1111/j.1574-6976.2009.00198.x
Šolić, M., Krstulović, N., Jozić, S., and Curać, D. (1999). The rate of concentration of faecal coliforms in shellfish under different environmental conditions. Environ. Int. 25, 991–1000. doi: 10.1016/S0160-4120(99)00067-7
Sommer, M. O., and Dantas, G. (2011). Antibiotics and the resistant microbiome. Curr. Opin. Microbiol. 14, 556–563. doi: 10.1016/j.mib.2011.07.005
Sørum, H., and L′Abée-Lund, T. M. (2002). Antibiotic resistance in food-related bacteria—a result of interfering with the global web of bacterial genetics. Int. J. Food Microbiol. 78, 43–56. doi: 10.1016/S0168-1605(02)00241-6
Stecher, B., Denzler, R., Maier, L., Bernet, F., Sanders, M. J., Pickard, D. J., et al. (2012). Gut inflammation can boost horizontal gene transfer between pathogenic and commensal Enterobacteriaceae. Proc. Natl. Acad. Sci. U.S.A. 109, 1269–1274. doi: 10.1073/pnas.1113246109
Strockbine, N. A., Bopp, C. A., Fields, P. I., Kaper, J. B., and Nataro, J. P. (2015). “Escherichia, Shigella, and Salmonella,” in Manual of Clinical Microbiology, 11th Edn, eds J. H. Jorgensen, M. A. Pfaller, K. C. Carroll, G. Funke, M. L. Landry, S. S. Richter, et al. (Washington, DC: ASM Press), 685–686.
Strohmeier, T., Strand,Ø., Alunno-Bruscia, M., Duinker, A., and Cranford, P. J. (2012). Variability in particle retention efficiency by the mussel Mytilus edulis. J. Exp. Mar. Biol. Ecol. 412, 96–102. doi: 10.1016/j.jembe.2011.11.006
Sullivan,Å., Edlund, C., and Nord, C. E. (2001). Effect of antimicrobial agents on the ecological balance of human microflora. Lancet Infect. Dis. 1, 101–114. doi: 10.1016/S1473-3099(01)00066-4
Sunde, M., and Norström, M. (2006). The prevalence of, associations between and conjugal transfer of antibiotic resistance genes in Escherichia coli isolated from Norwegian meat and meat products. J. Antimicrob. Chemother. 58, 741–747. doi: 10.1093/jac/dkl294
Sunde, M., and Sørum, H. (2001). Self-transmissible multidrug resistance plasmids in Escherichia coli of the normal intestinal flora of healthy swine. Microb. Drug Resist. 7, 191–196. doi: 10.1089/10766290152045075
Tancrède, C. (1992). Role of human microflora in health and disease. J. Clin. Microbiol. Infect. Dis. 11, 1012–1015. doi: 10.1007/bf01967791
Taylor, N. G., Verner-Jeffreys, D. W., and Baker-Austin, C. (2011). Aquatic systems: maintaining, mixing and mobilising antimicrobial resistance? Trends Ecol. Evol. 26, 278–284. doi: 10.1016/j.tree.2011.03.004
Teuber, M., Meile, L., and Schwarz, F. (1999). Acquired antibiotic resistance in lactic acid bacteria from food. Antonie Van Leeuwenhoek 76, 115–137. doi: 10.1023/A:1002035622988
van der Veen, E. L., Schilder, A. G., Timmers, T. K., Rovers, M. M., Fluit, A. C., Bonten, M. J., et al. (2009). Effect of long-term trimethoprim/sulfamethoxazole treatment on resistance and integron prevalence in the intestinal flora: a randomized, double-blind, placebo-controlled trial in children. J. Antimicrob. Chemother. 63, 1011–1016. doi: 10.1093/jac/dkp050
van Elsas, J. D., Semenov, A. V., Costa, R., and Trevors, J. T. (2011). Survival of Escherichia coli in the environment: fundamental and public health aspects. ISME J. 5, 173–183. doi: 10.1038/ismej.2010.80
VKM (2015). Assessment of Antimicrobial Resistance in the Food Chains in Norway. Scientific Opinion of the Panel on microbiological hazards of the Norwegian Scientific Committee for Food Safety, ISBN: 978-82-8259-184-3. Oslo: Norwegian Scientific Committee for Food Safety (VKM).
Welch, R. A. (2006). “The genus Escherichia,” in The Prokaryotes: A Handbook on the Biology of Bacteria: Proteobacteria: Gamma subclass, eds M. Dworkin, S. Falkow, E. Rosenberg, K.-H. Schleifer, and E. Stackebrandt (New York, NY: Springer Science & Business Media), 60–68.
Wellington, E. M., Boxall, A. B., Cross, P., Feil, E. J., Gaze, W. H., Hawkey, P. M., et al. (2013). The role of the natural environment in the emergence of antibiotic resistance in Gram-negative bacteria. Lancet Infect. Dis. 13, 155–165. doi: 10.1016/S1473-3099(12)70317-1
Wester, A., Melby, K., Wyller, T., and Dahle, U. (2014). E. coli bacteremia strains-high diversity and associations with age-related clinical phenomena. Clin. Microbiol. 3, 1–7. doi: 10.4172/2327-5073.1000140
Wester, A. L., Dunlop, O., Melby, K. K., Dahle, U. R., and Wyller, T. B. (2013). Age-related differences in symptoms, diagnosis and prognosis of bacteremia. BMC Infect. Dis. 13:346. doi: 10.1186/1471-2334-13-346
WHOCC Server (2016). ATC/DDD Index 2016 [Online]. WHO Collaborating Centre for Drug Statistics Methodology (WHOCC). Available at: http://www.whocc.no/atc_ddd_index/ [accessed November 3, 2016].
World Health Organization [WHO] (2014). Antimicrobial Resistance: Global Report on Surveillance. Geneva: World Health Organization (WHO).
Wright, G. D. (2010). Antibiotic resistance in the environment: a link to the clinic? Curr. Opin. Microbiol. 13, 589–594. doi: 10.1016/j.mib.2010.08.005
Keywords: bivalve mollusks, Enterobacteriaceae, Escherichia coli, antibacterial resistance, horizontal gene transfer
Citation: Grevskott DH, Svanevik CS, Sunde M, Wester AL and Lunestad BT (2017) Marine Bivalve Mollusks As Possible Indicators of Multidrug-Resistant Escherichia coli and Other Species of the Enterobacteriaceae Family. Front. Microbiol. 8:24. doi: 10.3389/fmicb.2017.00024
Received: 24 November 2016; Accepted: 05 January 2017;
Published: 18 January 2017.
Edited by:
Jose L. Martinez, Spanish National Research Council, SpainReviewed by:
Patrícia Poeta, University of Trás-os-Montes and Alto Douro, PortugalCopyright © 2017 Grevskott, Svanevik, Sunde, Wester and Lunestad. This is an open-access article distributed under the terms of the Creative Commons Attribution License (CC BY). The use, distribution or reproduction in other forums is permitted, provided the original author(s) or licensor are credited and that the original publication in this journal is cited, in accordance with accepted academic practice. No use, distribution or reproduction is permitted which does not comply with these terms.
*Correspondence: Bjørn T. Lunestad, Ymx1QG5pZmVzLm5v
Disclaimer: All claims expressed in this article are solely those of the authors and do not necessarily represent those of their affiliated organizations, or those of the publisher, the editors and the reviewers. Any product that may be evaluated in this article or claim that may be made by its manufacturer is not guaranteed or endorsed by the publisher.
Research integrity at Frontiers
Learn more about the work of our research integrity team to safeguard the quality of each article we publish.