- 1Laboratory for Molecular Microbiology, Institute of Molecular Genetics and Genetic Engineering, University of Belgrade, Belgrade, Serbia
- 2Instituto de Productos Lácteos de Asturias – Consejo Superior de Investigaciones Científicas, Villaviciosa, Spain
- 3Center for Electron Microscopy, Faculty of Biology, University of Belgrade, Belgrade, Serbia
The aim of this study was to determine the role of an exopolysaccharide produced by natural dairy isolate Lactobacillus paracasei subsp. paracasei BGSJ2-8, in the adhesion to intestinal epithelial cells and a decrease in Escherichia coli’s association with Caco-2 cells. Annotation of the BGSJ2-8 genome showed the presence of a gene cluster, epsSJ, which encodes the biosynthesis of the strain-specific exopolysaccharide EPS-SJ, detected as two fractions (P1 and P2) by size exclusion chromatography (SEC) coupled with multi-angle laser light scattering (MALLS) detection. SEC-MALLS analysis revealed that an EPS-SJ- mutant (EPS7, obtained by insertion mutagenesis of the glps_2198 gene encoding primary glycosyltransferase) does not produce the P2 fraction of EPS-SJ. Transmission electron microscopy showed that EPS7 mutant has a thinner cell wall compared to the EPS-SJ+ strain BGSJ2-83 (a plasmid free-derivative of BGSJ2-8). Interestingly, strain BGSJ2-83 showed higher adhesion to Caco-2 epithelial intestinal cell line than the EPS7 mutant. Accordingly, BGSJ2-83 effectively reduced E. coli ATCC25922’s association with Caco-2 cells, while EPS7 did not show statistically significant differences. In addition, the effect of EPS-SJ on the proliferation of lymphocytes in gastrointestinal associated lymphoid tissue (GALT) was tested and the results showed that the reduction of GALT lymphocyte proliferation was higher by BGSJ2-83 than by the mutant. To the best of our knowledge this is the first report indicating that the presence of EPS (EPS-SJ) on the surface of lactobacilli can improve communication between bacteria and intestinal epithelium, implying its possible role in gut colonization.
Introduction
The components of the cell wall of Gram-positive bacteria may vary considerably depending on the genus, species, and even the strain (Delcour et al., 1999). Hence, the diverse structures of lactobacillus surface molecules, in large part, reflect the adaptation of these microorganisms to specific ecological and environmental conditions.
The surface polysaccharides of lactobacilli contribute significantly to their interaction with the environment, including interactions with other microorganisms and a variety of hosts, in particular with the intestinal mucosa and IEC, contributing to the probiotic characteristics of a specific strain. Bacterial polysaccharides vary in composition, size and the position of branches, contributing to the structural diversity of surface molecules (Lebeer et al., 2008). The polysaccharide component of a bacterial cell’s surface may form EPS that are weakly attached to the cell surface, as well as CPS usually covalently bound to peptidoglycan, forming a firm coating around the cell. It should be noted that the composition, size, and surface properties of polysaccharides depend on the growth conditions of a given bacterial species, as was recently shown for the surface properties of Lactobacillus acidophilus NCC2628 (Schär-Zammaretti and Ubbnik, 2003). Based on its chemical composition, an EPS may be a homopolysaccharide – consisting of one type of monosaccharide – or heteropolysaccharide – composed of different types of monosaccharides. Generally, heteropolysaccharides synthesized by lactic acid bacteria may differ in composition, structure, function, size, and molecular weight (de Vuyst and Degeest, 1999). The work of Mozzi et al. (2006) revealed huge diversity of EPS produced by 31 strains belonging to different species of lactic acid bacteria. The molecular weight of the EPS varied between 8 to >5,000 kDa. Interestingly, it was shown that low molecular weight-EPS are more common than high molecular weight-EPS in lactic acid bacteria (Mozzi et al., 2006). For instance, it has been shown that the EPS of L. casei YIT 9018 is composed of two types of heteropolysaccharides (Nagaoka et al., 1990).
Generally, the EPS biodiversity could be result of different sugar nucleotide biosynthesis pathways and genetic variability of the strains (Mozzi et al., 2006). The genetic elements involved in the synthesis of the conserved structure of the polymer are organized into operons, located on chromosomal or plasmid DNA (Boels et al., 2001; Zivkovic et al., 2015). The genes present in the eps operons encode proteins with specific functions: assembling of repeating units, chain length determination, polymerization, export, and regulation (de Vuyst and Degeest, 1999). A number of glycosyltransferases involved in the repeating units’ assembly have been revealed (Mozzi et al., 2006). Characterization of a large number of operons for the biosynthesis of surface polysaccharides has revealed that within the conserved structural organization, variations in the number and position of genes in the operon exist (Péant et al., 2005).
Due to their long history of safe use in human consumption, lactobacilli have been given “Qualified Presumption of Safety” status (EFSA, 2007). They have been proposed as beneficial for human and animal health as probiotics, defined as “live microorganisms which when administered in adequate amounts confer a health benefit on the host” (FAO-WHO, 2006).
The literature data suggest that surface polysaccharides indirectly affect the adhesive properties of lactobacilli by means of various interactions such as passive forces, electrostatic interactions, and hydrophobic and steric forces (Servin, 2004). The work of Nikolic et al. (2012) showed that EPS-producing strain L. paraplantarum BGCG11 have lower adhesion to IEC than its non-EPS-producing derivative NB1. The results of Ruas-Madiedo et al. (2006) showed that the adhesion levels depend on the EPS type. For example, the EPS fraction GG showed no effect on the adhesion of L. rhamnosus GG, while at the highest dose it slightly increased the adherence of Bifidobacterium animalis IPLA-R1. However, the EPS fractions NB667 and IPLA-R1 promoted a significant reduction in the adhesion of both probiotic strains B. longum NB667 and B. animalis IPLA-R1. Further, Denou et al. (2008) showed that deletion of the entire EPS cluster slightly increased the gut persistence time of the knock-out mutant in comparison to the parental strain. In addition, the results of Polak-Berecka et al. (2014) indicated that polysaccharides produced by L. rhamnosus E/N hindered adhesion and aggregation by masking protein receptors at the cell surface. Moreover, the reduction in EPS production by L. johnsonii FI9785 increased bacterial adhesion to chicken gut explants (Dertli et al., 2015).
Lactobacillus strains L. paraplantarum BGCG11 and L. fermentum BGHI14 have been shown to have immunomodulatory capacity and to have the ability to induce the production of various cytokines (IL-17, IFN-γ, IL-10, TNF-α, and IL-1β; Nikolic et al., 2012; Lukic et al., 2013). The EPS-producing strain L. paraplantarum BGCG11, as an example, was shown to exhibit an anti-inflammatory and immunosuppressive profile, whereas its non-EPS derivative induced a pro-inflammatory response (Nikolic et al., 2012).
Interest in the health-promoting potential of lactobacilli has significantly increased, especially with regard to their role in the prevention and treatment of disease. Our previous results showed that the natural dairy isolate L. paracasei subsp. paracasei BGSJ2-8 synthesizes BacSJ bacteriocin, PI-type proteinase and AggLb aggregation promoting protein, and it is able to survive in simulated gut conditions, hence it could be used in dairy industry as functional starter culture with potential probiotic traits (Lozo et al., 2007; Miljkovic et al., 2015). In this study, the effects of an EPS produced by BGSJ2-8 (EPS-SJ) on its adhesion to IEC, a decrease of Escherichia coli association with Caco-2 cells, and the proliferation of lymphocytes in GALT were investigated. The knowledge obtained in this study may help to understand the specific contribution of EPS-SJ in gut colonization and pathogen exclusion.
Materials and Methods
Media and Growth Conditions
The strains and plasmids used in this study are listed in Table 1. Lactobacillus strains were grown in De Man-Rogosa-Sharpe (MRS) medium (Merck GmbH, Darmstadt, Germany) at 28°C, 30°C or 37°C when it was appropriate. E. coli strains, used as intermediate hosts for cloning experiments and plasmid propagation, were grown in LBwith aeration at 37°C for 16 h. Solid media were prepared by adding agar (1.5% w/v). When appropriate, the media contained ampicillin (100 μg/ml) or erythromycin (250 μg/ml for E. coli, or 2.5 μg/ml for lactobacilli).
Sequencing and Annotation of the Putative EPS Biosynthetic Cluster
Genome sequencing was performed by Macrogen (Seoul, Korea), a next generation sequencing service. The nucleotide sequence of the gene cluster glps_2198-2211 (epsSJ) was deposited in the “European Nucleotide Archive” database1 (Accession No. LN879393). An in-depth analysis of this gene cluster was performed. The nucleotide sequence of each gene was used for BLAST searches against the NCBI nucleotide database. When the best BLASTN hit had less than 50% coverage, a BLASTP analysis was performed.
DNA Manipulation and Construction of EPS- Mutants
Chromosomal DNA from BGSJ2-8 was isolated as described by Hopwood et al. (1985). For cloning, part of the glps_2198 gene was amplified by PCR using primers 2198 Fw and 2198 Rv complementary to the glps_2198 gene of BGSJ2-8 (Table 1). PCR amplification was performed using a KAPA Taq DNA polymerase kit (Kapa Biosystems, Wilmington, MA, USA, 5 U/ml) and amplified in Gene Amp PCR system 2700 (Applied Biosystems, Waltham, MA, USA) using the following program: predenaturation (7 min, 94°C), 30 successive cycles consisting of denaturation (30 s, 94°C), annealing (1 min, 50°C), elongation (30 s, 72°C), and a final elongation (7 min, 72°C). The resulting PCR amplicon, 2198 Fw/2198 Rv, was purified using QIAquick PCR Purification KIT/250 (QIAGEN GmbH, Hilden, Germany), sequenced [Macrogen, Europe (Amsterdam, The Netherlands)], and ligated into pGEM-T Easy cloning vector (Promega, Fitchburg, WI, USA). General procedures for cloning and DNA manipulation were performed as described by Sambrook et al. (1989). The ligation mixtures were used to transform E. coli DH5α by a standard heat-shock transformation procedure (Sambrook et al., 1989). Transformants were selected on LB plates containing ampicillin (100 μg/ml). The resulting pGEM-T Easy/2198 was sequenced. Using the BLAST algorithm2 for nucleotide sequence analysis, it was confirmed that fragment 2198 cloned into pGEM-T Easy/2198 was identical (100%) to the corresponding sequences in the genome of BGSJ2-8. The construct pGEM-T Easy/2198 was further digested by EcoRI (Fermentas, Vilnius, Lithuania). The obtained 2198 fragment (518 bp) was eluted using a QIAquick Gel Extraction Kit (Qiagen, Hilden, Germany), and ligated into pGhost9 plasmid digested by EcoRI restriction enzyme. The ligation mixture was used to transform E. coli EC101. Transformants were selected on LB plates containing erythromycin (250 μg/ml). The resulting construct, pGhost9/2198, carrying the appropriate fragment, was used for transformation of strain BGSJ2-83 by electroporation (Holo and Nes, 1995) using an Eppendorf electroporator (Eppendorf, Hamburg, Germany). The BGSJ2-83/pGhost9/2198 transformants were selected on MRS plates containing erythromycin (5 μg/ml) at 28°C, and the presence of plasmid pGhost9/2198 was confirmed.
All plasmid isolations from E. coli were performed using the QIAprep Spin Miniprep kit according to the manufacturer’s instructions (Qiagen, Hilden, Germany). Plasmids from L. paracasei were isolated using the same kit with the previously described modification for L. lactis (Filipic et al., 2013). Transposition was performed as described by Maguin et al. (1996). A logarithmic culture of BGSJ2-83/pGhost9/2198 was grown at 28°C (a permissive temperature for the replication of plasmid pGhost9), and then transferred to 37°C for 48 h, to allow the integration of the plasmid into the host chromosome. The potential EPS- mutants were screened on MRS plates containing erythromycin (5 μg/ml) as erythromycin-resistant colonies, after 48 h at 37°C. The integration of plasmid pGhost9/2198 into the glps_2198 gene was confirmed by PCR using primers complementary to pGhost9 (pGhF and pGhR) and the 5′ of the glps_2198 gene, upstream of the sequence used for the insertion mutagenesis (Table 1). PCR was performed essentially as described above with an annealing temperature of 55°C. Finally, the integration of plasmid pGhost9/2198 into the mutant’s chromosome was specifically analyzed by Southern hybridization of the chromosomal DNA of strain BGSJ2-83 and its EPS- derivative BGSJ2-83-2198-EPS7 (in further text EPS7) digested by EcoRI or HindIII by using PCR fragment 2198 as a probe. Southern blot hybridization and detection of positive signals was done on the membrane, as recommended by the manufacturer (Roche Diagnostic GmbH, Mannheim, Germany).
EPS-SJ Extraction and Purification
EPS-SJ extraction and purification from strains BGSJ2-83 (epsSJ+), EPS7 (the epsSJ knock-out) and BGHN14 (epsSJ-) was performed essentially as described by Nikolic et al. (2012). Bacterial strains were grown at 25°C for 48 h in a basal minimum medium (Cerning et al., 1994) inoculated with an overnight culture grown in basal minimum medium (10%, v/v) and incubated. After incubation, the bacteria were removed by centrifugation (12,000 × g, 30 min, 4°C) and EPS was extracted and precipitated at 4°C for 48 h by adding two volumes of chilled absolute ethanol. The precipitate was collected by centrifugation (12,000 × g, 20 min, 4°C), dissolved in distilled water and dialyzed against water, using 12–14 kDa MWCO membranes (Sigma Chemical Co., St. Louis, MO, USA), for 24 h at 4°C. The dialyzed retentate was freeze-dried to obtain the EPS-crude fraction which was additionally purified to reduce the DNA and protein content as previously described (Nikolic et al., 2012). The resulting lyophilized powder was the purified EPS, which was used in different concentrations for further analyses. The protein content of this purified EPS was quantified by the BCA method (Pierce, Rockford, IL, USA), the presence of DNA was determined using the a Take3 Multi-volume Plate (Biotek Instruments GmbH, Bad Friedchshall, Germany) and the EPS content was determined by means of SEC coupled with MALLS analysis (SEC-MALLS).
Size Exclusion Chromatography
The molar mass distribution of the purified EPS was analyzed by means of a SEC-MALLS detector (Dawn Heleos II; Wyatt Europe GmbH, Dembach, Germany) as previously described (Nikolic et al., 2012). Briefly, the chromatographic system used (Waters, Milford, MA, USA) has a photodiode array detector, set at 280 nm for detecting proteins, and a 410 refractive index detector for quantifying the different peaks obtained after separation (40°C, 0.45 ml/min, 0.1 M NaNO3) in the TSKgel G3000PWXL and TSKgel G5000 PWXL columns, placed in series (Supelco–Sigma, Bellefonte, PA, USA). Quantification of the EPS-fractions was made from the refractive index signal using the regression equations obtained from Dextran standards of different molar mass. The molar mass distribution of the EPS-fractions was obtained using Astra version 3.5 software (Wyatt Europe GmbH, Dembach, Germany).
Transmission Electron Microscopy
The samples of bacterial colonies were encased in Bacto-agar, and the solidified blocks were cut into 1 mm3 cubes and fixed with 2.5% glutaraldehyde (v/v) in 0.1 M Sørensen PBS. After postfixation with 2% osmium teroxide in the same buffer, samples were dehydrated using increasing concentrations of ethanol and embedded in Araldite resin (Fluka, Neu-Ulm, Germany). Ultra-thin sections were obtained using a Leica UC6 ultramicrotome (Leica Microsystems, Mannheim, Germany) and mounted on copper grids. For the ultrastructural analysis of the cell wall’s structural components, serial sections were counterstained with 5% phosphotungstic acid in distilled water for 30 min at 56°C and subsequently with 0.1% Alcian blue in 3% acetic acid for 30 min at room temperature. Sections were examined on a Philips CM12 transmission electron microscope (Philips/FEI, Eindhoven, The Netherlands) equipped with a SIS MegaView III digital camera (Olympus Soft Imaging Solutions, Hamburg, Germany). The digital images were used for both cell wall ultrastructural analysis and the cell wall thickness measurements. The cell wall thickness was measured by Image J software (NIH, Bethesda, MD, USA) at a magnification of 53,000×. About 50 randomly selected sections of whole bacterial cells were used for the measurements at five different regions of each cell.
Adhesion to Caco-2 and HT29-MTX Cells
The colonocyte-like cell lines Caco-2 and HT29-MTX were used to determine the adhesion ability of the lactobacillus strains to mucosal surfaces in the intestine. A Caco-2 cell line was purchased from the European Collection of Cell Cultures (ECACC No. 86010202) and HT29-MTX was kindly supplied by Dr. T. Lesuffleur (INSERM UMR S 938, Paris, France; Lesuffleur et al., 1990). The culture and maintenance of the cell lines were carried out as previously described (Nikolic et al., 2012) using Advanced DMEM medium (Gibco Invitrogen, Paisley, UK) for Caco-2 and HT29-MTX supplemented with heat inactivated fetal bovine serum (5% for Caco-2, 10% for HT29-MTX), L-glutamine (2 mM) and with a mixture of antibiotics (10 U/ml penicillin, 10 μg/ml streptomycin, 50 μg/ml gentamicin). Media and reagents were purchased from PAA (Pasching, Austria). Intestinal cells (passage 5–6) were seeded in 24-well plates and cultivated until a confluent differentiated state was reached (monolayer). Lactobacillus strains were cultured for 24 h and, after washing twice with Dulbecco’s PBS solution (Sigma, St. Louis, MO, USA), were resuspended in the corresponding cell-line media without antibiotics at a concentration of about 108 CFU/ml. The cellular monolayers were also carefully washed and lactobacillus suspensions were added at a ratio of about 10:1 (lactobacilli:eukaryotic cells). The eukaryotic cells were counted in Tripan blue solution. Adhesion experiments were carried out for 1 h at 37°C, 5% CO2 and afterward the wells were gently washed to release unattached bacteria before proceeding with the lysis of cellular monolayers using 0.25% Trypsin–EDTA solution (PAA, Pasching, Austria). Dilutions of samples, before and after adhesion, were made in PBS solution and lactobacilli counts were performed in MRS agar plates. The adhesion was calculated as a percentage: CFU adhered lactobacilli strain/CFU added lactobacilli strain. Experiments were carried out in two replicated plates and in each plate two wells were used per sample.
Decrease on E. coli Association to Caco-2 by Lactobacilli
The capability of reference strain E. coli ATCC25922 to be associated to the intestinal epithelium in the presence and absence of lactobacilli was tested. Bacterial cultures were washed twice with PBS and resuspended in DMEM without antibiotics at a concentration of ∼1 × 108 CFU/ml; this number was corroborated by plate counting in the agar medium specific for each bacterium. The bacterial suspensions containing E. coli ATCC25922 or a combination of E. coli and lactobacilli (ratio 1:1) were independently added to the Caco-2 monolayers at a ratio of 10:1, (bacteria:eukaryotic cells; in the case of E. coli–lactobacilli combinations, each bacterial type was added at a ratio of 5:1) and incubated at 37°C, with 5% CO2 for 1 h. Afterward, the monolayers were gently washed twice with PBS to remove the unattached bacteria, and the eukaryotic cells were released using 0.25% Trypsin–EDTA solution (Sigma, St. Louis, MO, USA). The samples were diluted in 0.9% (w/v) NaCl buffer and plated to LA to enumerate the associated E. coli. The percentage of E. coli association was calculated as follows: 100 × CFU/ml bacteria associated CFU/ml bacteria added (the dilution of bacteria was taken into account). Each combination was tested in triplicate. To determine the capability of the lactobacilli to decrease the association of E. coli ATCC25922 to Caco-2 monolayers, data were referred to that obtained with the E. coli alone (i.e., 100% association). Each combination of E. coli–lactobacilli, and the E. coli alone as reference, were tested in three replicates.
Proliferation of GALT-Isolated Lymphocytes in the Presence of Non-Viable Lactobacilli
The lactobacilli cells were inactivated by UV light for three cycles of 30 min each. Plate counting was carried out after UV treatment to corroborate the absence of live lactobacilli that might be able to recover in the proper medium. UV-inactivated lactobacilli were then divided in single use aliquots, frozen in liquid N2 and stored at –80°C until use (López et al., 2012). This study was approved by the Animal Experimentation Ethical Committee of the Faculty of Pharmacy, University of Belgrade (Serbia), in strict adherence to international directives. A total number of three Wistar rats (healthy female adults between 6 and 8 weeks old) were purchased from the Farm of the Military Medical Academy, Belgrade (Serbia). For the experiments each animal was anesthetized with CO2 and, once assured of the loss of corneal reflex, its intestine was excised from the jejunum to the ileocaecal junction. The whole small intestine was placed in cold Hank’s balanced salt solution (HBSS without calcium and magnesium ions, prepared according to the formulation of Gibco, Invitrogen, Carlsbad, CA, USA) and kept at 4°C until processing. Finally, the animals were sacrificed using the increase of CO2 concentration. The isolation of lymphocytes from GALT (Peyer’s Patches lymphocytes and intestinal epithelium lymphocytes) was carried out as previously described (Hidalgo-Cantabrana et al., 2014a).
To quantify the response of GALT to the different factors tested, 2 × 105 lymphocyte cells were incubated with UV-inactivated lactobacilli (at a ratio of 1:5) for 4 days in complete RPMI medium with antibiotics at 37°C and 5% CO2. All cultures were performed in triplicate in 96-well round-bottom microtiter plates. After 4 days of incubation, the proliferation of GALT-lymphocytes was determined with a Cell Proliferation Assay Kit (Millipore Corporation, Billerica, MA, USA) following the manufacturer’s instructions. Results were compared to a negative control (lymphocytes growing in complete RPMI medium with antibiotics).
Statistical Analysis
After checking the normal distribution of the proliferation data, one-way ANOVA tests were used to determine the differences between each factor and the negative control. Finally, one-way ANOVA tests together with the mean comparison test of least significant difference were used to compare the differences between the three strains. The results are represented as mean ± standard deviation or standard error. The SPSS 15.0 statistical software package (SPSS Inc, Chicago, IL, USA) was used for all determinations and p < 0.05 was considered significant. The Kruskal–Wallis test (Non-parametric ANOVA) followed by Dunn’s multiple comparison test were used to compare the mean values obtained at transmission electron microscopy. Significant differences were set at α = 0.05.
Results
Identification of a glps_2198-2211 (epsSJ) Gene Cluster
The entire genome of L. paracasei subsp. paracasei BGSJ2-8 was sequenced, annotated and partly assembled (unpublished, in house data). Although BGSJ2-8 does not produce visible ropy EPS, genome annotation revealed the presence of a unique chromosomally located gene cluster glps_2198-2211 (epsSJ) encoding for strain-specific EPS-SJ synthesis (Table 2). Most of the 14 genes in the cluster showed similarities to various known proteins involved in the biosynthesis of extracellular or CPS from other lactic acid bacteria, including genes for enzymes responsible for regulation, polymerization, export, assembling repeating units, and chain length determination (Hidalgo-Cantabrana et al., 2014b). The epsSJ operon starts with the genes glps_2211, glps_2210, and glps_2209 that could be putatively involved in spatial and temporal regulation of EPS-SJ synthesis and in chain length determination. The glps_2198 gene, encoding a priming glycosyltransferase, is positioned at the end of the epsSJ gene cluster. The priming glycosyltransferase has a role in the first (priming) step in the EPS biosynthesis. Upstream of the glps_2198 there are four genes (glps_2199, glps_2204, glps_2206, and glps_2207) assigned as glycosyltransferases with function of transferring the sugars of the EPS subunit in an ordered way. The gene glps_2200, as a putative galactoside acetyltransferase, might have be a factor modifying glycosyl residue, while the glps_2202, with homology to CpsIbJ, a putative β1, 3-galactosyltransferase, might have a role in the addition of galactose to the EPS-SJ polymer.
Interestingly, several genes in the epsSJ gene cluster have the best BLAST hits to the gene cluster for biosynthesis of the CPS previously described in probiotic L. casei Shirota (CPS operon Shirota; Yasuda et al., 2008) (Figure 1). The nucleotide sequences of genes glps_2198 (518 bp), glps_2200 (435 bp), glps_2209 (524 bp), and glps_2211 (269 bp) of strain BGSJ2-8 showed identity to the nucleotide sequences of the genes cps1J (74%), encoding primary glycosyltransferases, cps1G (71%), encoding galactoside acetyltransferase, and cps1B (75%) and cps1A (71%), encoding polysaccharide chain length determination proteins, within the Shirota CPS operon.
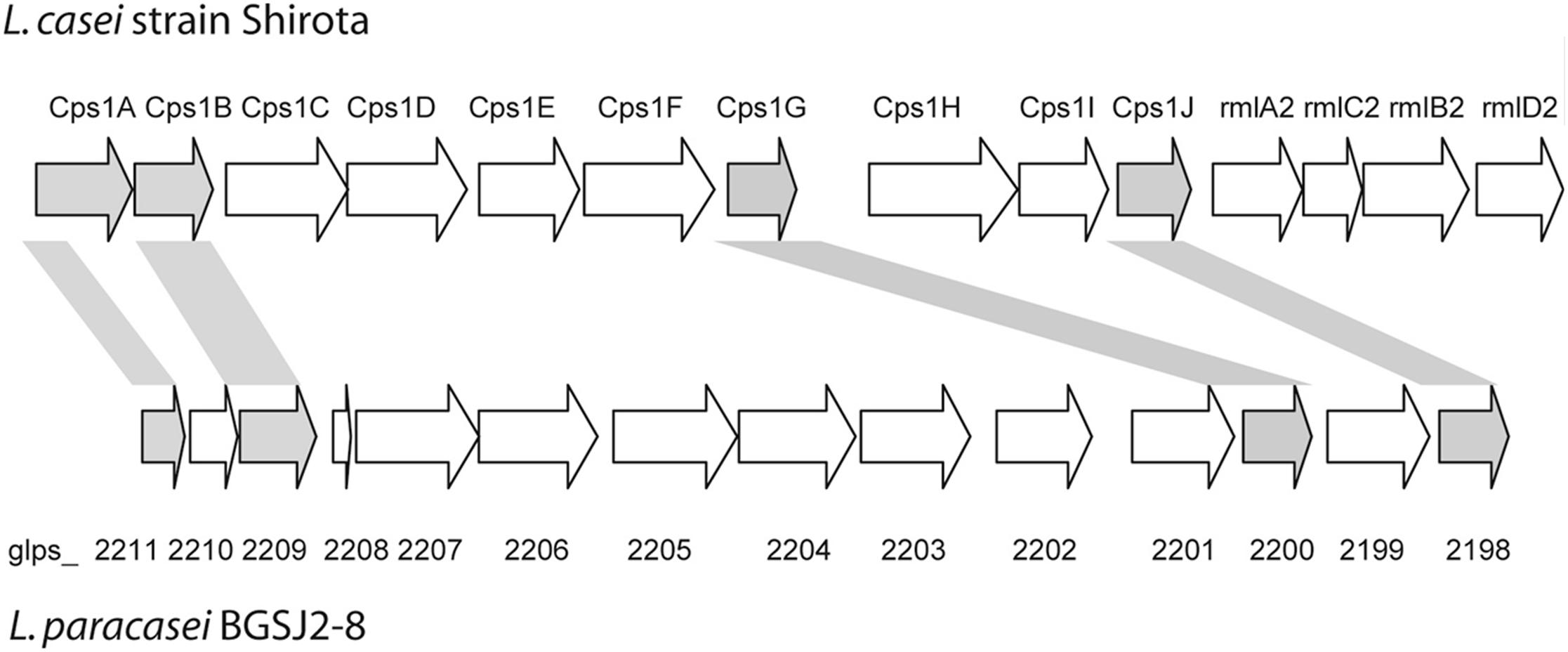
FIGURE 1. Schematic representation of CPS and EPS-SJ synthesis clusters in L. casei Shirota and L. paracasei BGSJ2-8, respectively. Genes sharing high sequence similarity are indicated and highlighted in the same colors. Adapted from Yasuda et al. (2008).
Construction and Analysis of the EPS-SJ- Mutants
In order to determine the functional properties of the annotated epsSJ gene cluster, the gene encoding the primary glycosyltransferase glps_2198 was disrupted by insertional inactivation as described in Section “Materials and Methods.” Initially, the isolation of plasmid DNA from the erythromycin resistant colonies obtained after the transposition experiment indicated that the construct pGhost9/2198 had been integrated into the chromosome of BGSJ2-83. The experimental work was continued with a strain designated as EPS7, a potential mutant in the glps_2198 gene encoding the primary glycosyltransferase. EPS7 showed a similar growth phenotype to the parental strain (data not shown). The chromosomal integration of plasmid pGhost9/2198 was initially verified by isolation of plasmid DNA from the EPS7 mutant and transformation of competent E. coli EC101. After 24 h of incubation at 37°C, transformants were not obtained, indicating the absence of circular forms of plasmid pGhost9/2198 in the EPS7 mutant strain. Additionally, the integration was confirmed by PCR and Southern blot hybridization (as described in section “Materials and Methods”). The results revealed that plasmid pGhost9/2198 had been integrated into the chromosomal DNA of EPS7 at the specific site of the glps_2198 gene (data not shown).
Molar Mass Distribution of EPS
SEC-MALLS analysis was performed to analyze the molar mass distribution of the purified polymeric material and to quantify the EPS fractions. The strain L. paracasei subsp. paracasei BGHN14 was used as a negative reference strain, since PCR analysis confirmed that the epsSJ genes are not present in this strain (data not shown).
The results showed that the polymer purified from strain BGSJ2-83 presents two EPS fractions synthesized in similar amounts (Figure 2). One has a higher molecular weight (P1), about 41,200 Da, whereas the second fraction is smaller, about 13,600 Da (P2). However, it is worth mentioning that the P2 fraction might also contain proteins, since in the elution time corresponding to this peak (around 38 min) there was also an increase in the signal corresponding to the photodiode array detector in the UV spectrum (280 nm). Interestingly, in the EPS purified from EPS7 (the mutant), in which the primary glycosyltransferase glps_2198 gene was disrupted by insertional inactivation, the P2 fraction was not detected (although the increase in the UV signal is present), while the P1 fraction is present in lower amount (2/3 of amount detected in BGSJ2-83) and with higher molecular weight (about 80,000 Da). In the case of EPS purified from strain BGHN14, lacking the entire epsSJ gene cluster, the highest production corresponded to the fraction coinciding with the P2 EPS-SJ (1.6 higher amount than in BGSJ2-83), also presenting the same increase in the UV signal. Indeed, the amount of protein quantified by BCA was similar in both EPS fractions, i.e., 15.6 ± 0.5 μg/ml and 15.1 ± 0.3 μg/ml for BGSJ2-83 and BGHN14, respectively.
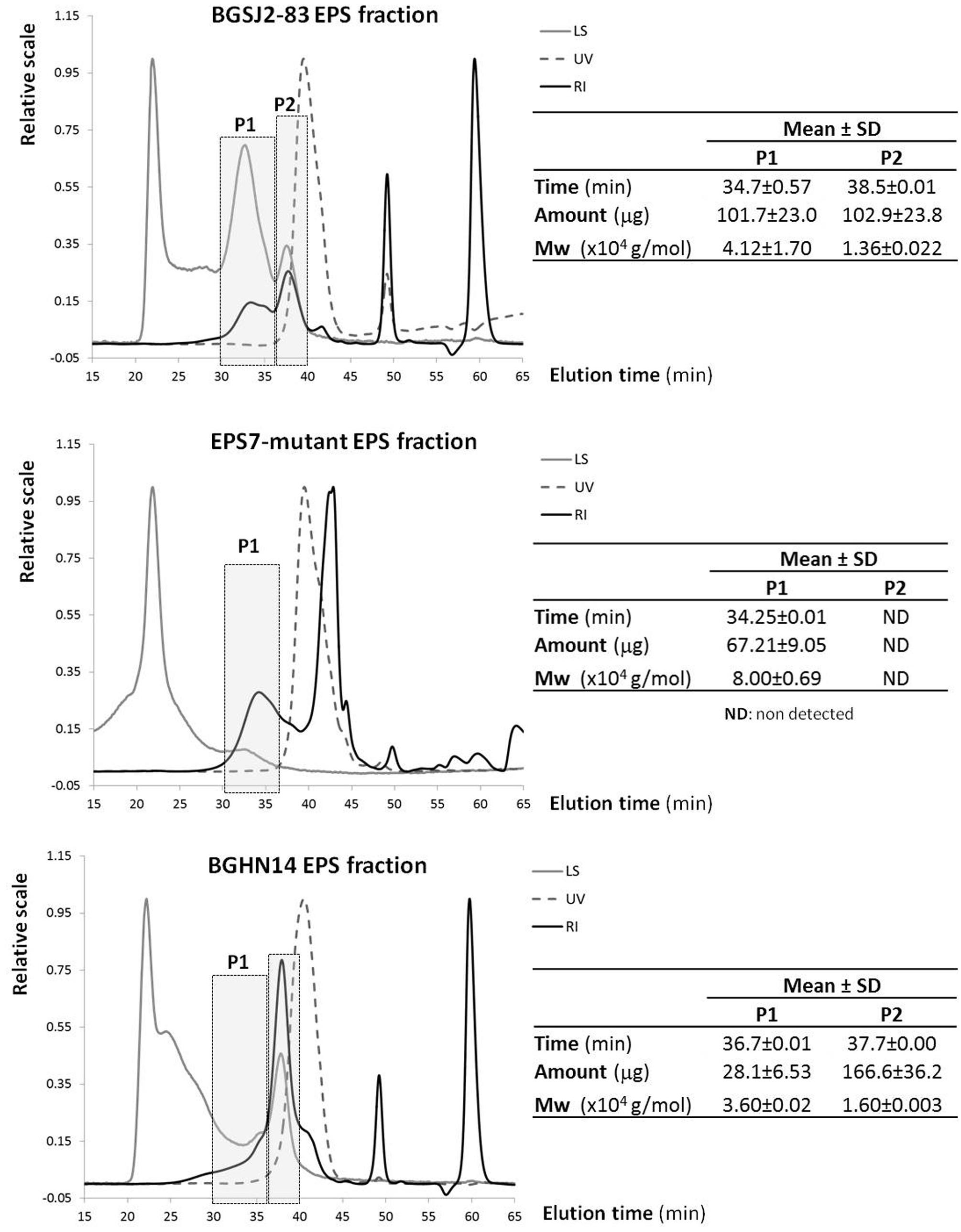
FIGURE 2. SEC-MALLS of the EPS fractions purified from L. paracasei subsp. paracasei BGSJ2-83 and from strain BGHN14, lacking the epsSJ gene cluster. LS, light scattering detector at 90o angle; UV, photodiode array detector set at 280 nm; RI, refraction index detector; Mw, weight/molar mass.
Electron-Microscopic Analysis and Cell Wall Thickness Measurement
Both, electron-microscopic analysis (Figure 3A), and cell wall thickness measurement (Figure 3B) of L. paracasei subsp. paracasei strains, showed a significant decrease of cell wall thickness in EPS7 and BGHN14 strains compared to BGSJ2-83 (∼40 nm). Indeed, it was observed that EPS7, lacking the P2 EPS fraction (∼30 nm), had even thinner cell wall than BGHN14 lacking P1 (∼35 nm).
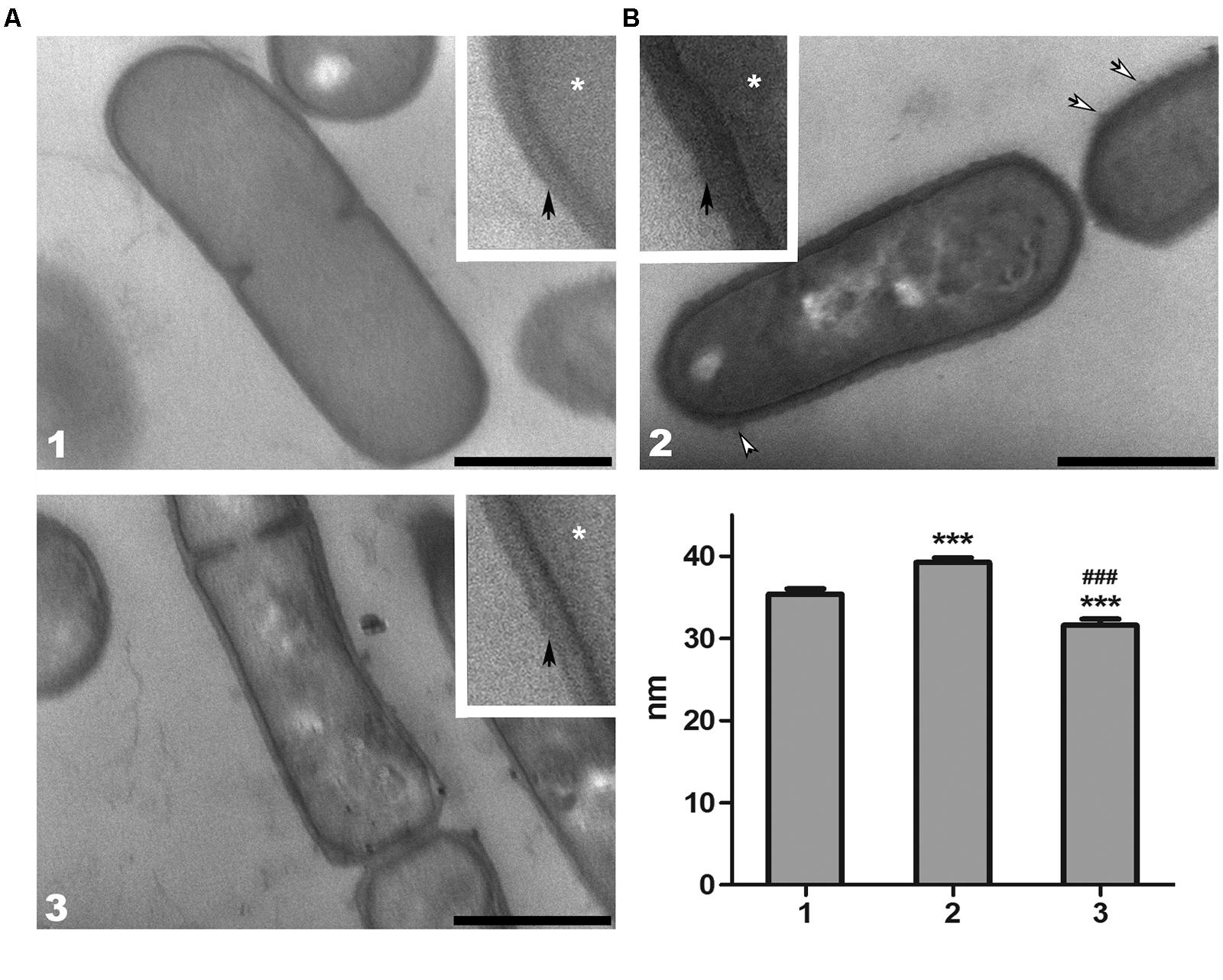
FIGURE 3. (A) Representative transmission electron micrographs of thin sections of L. paracasei subsp. paracasei (1) BGHN14, (2) BGSJ2-83, and (3) EPS7. The samples were prepared for ultrastructural analysis and ultra-thin sections were counterstained with phosphotungstic acid/Alcian blue. The details of the cell walls were enlarged (insets). White arrows indicate the fibrillar polysaccharide protrusions of the cell wall, while asterisks indicate cytoplasm. The thin, electron-dense layer at the surface of the cell wall suggests the presence of an S-layer (black arrows) in all analyzed strains. Scale bars: 500 nm. (B) Cell wall thickness of L. paracasei subsp. paracasei (1) BGHN14, (2) BGSJ2-83, and (3) EPS7 strains. Values are presented as means ± standard error of the mean. Statistically significant differences vs. non-EPS-SJ producing BGHN14: ∗∗∗p < 0.001; statistically significant differences vs. BGSJ2-83: ###p < 0.001.
In addition, ultrastructural analysis of the different strains of L. paracasei subsp. paracasei demonstrated an underlying alteration in cell wall structure. In BGHN14 and EPS7, the cell walls appeared pale, with low electron-density, particularly in the EPS7 mutant. The outer surface of the cell wall of these groups was smooth, without visible protrusions. Quite the opposite, the cell wall of BGSJ2-83 had a dark, electron-dense appearance. Also, the cell wall of BGSJ2-83 was slightly curved in shape with additional protrusions of fibrillar polysaccharide material at the extracellular surface.
Furthermore, detailed examination revealed the presence of a thin, electron-dense layer at the outermost surface of the cell wall in all of the groups, suggesting the presence of proteins, particularly in EPS7 and BGHN14, where this layer was clearly visible. In contrast, the presence of proteins in BGSJ2-83 was harder to resolve, and in most cases it was visibly absent.
Characterization of the Role of EPS-SJ in Adhesion to Epithelial Intestinal Cells
To estimate the role of EPS-SJ in the adhesion ability of strain BGSJ2-8 to IEC, in vitro comparative analysis of strain BGSJ2-83 and its EPS-SJ- derivative EPS7 was performed. The strains BGCG11, producing high molecular weight EPS-CG11, and BGHN14, lacking epsSJ gene cluster, were used as controls. In particular, the EPS-SJ-producing strain BGSJ2-83 and strain BGHN14 showed higher percentages of adhesion to the Caco-2 cell line than the mutant, EPS7 (Figure 4A).
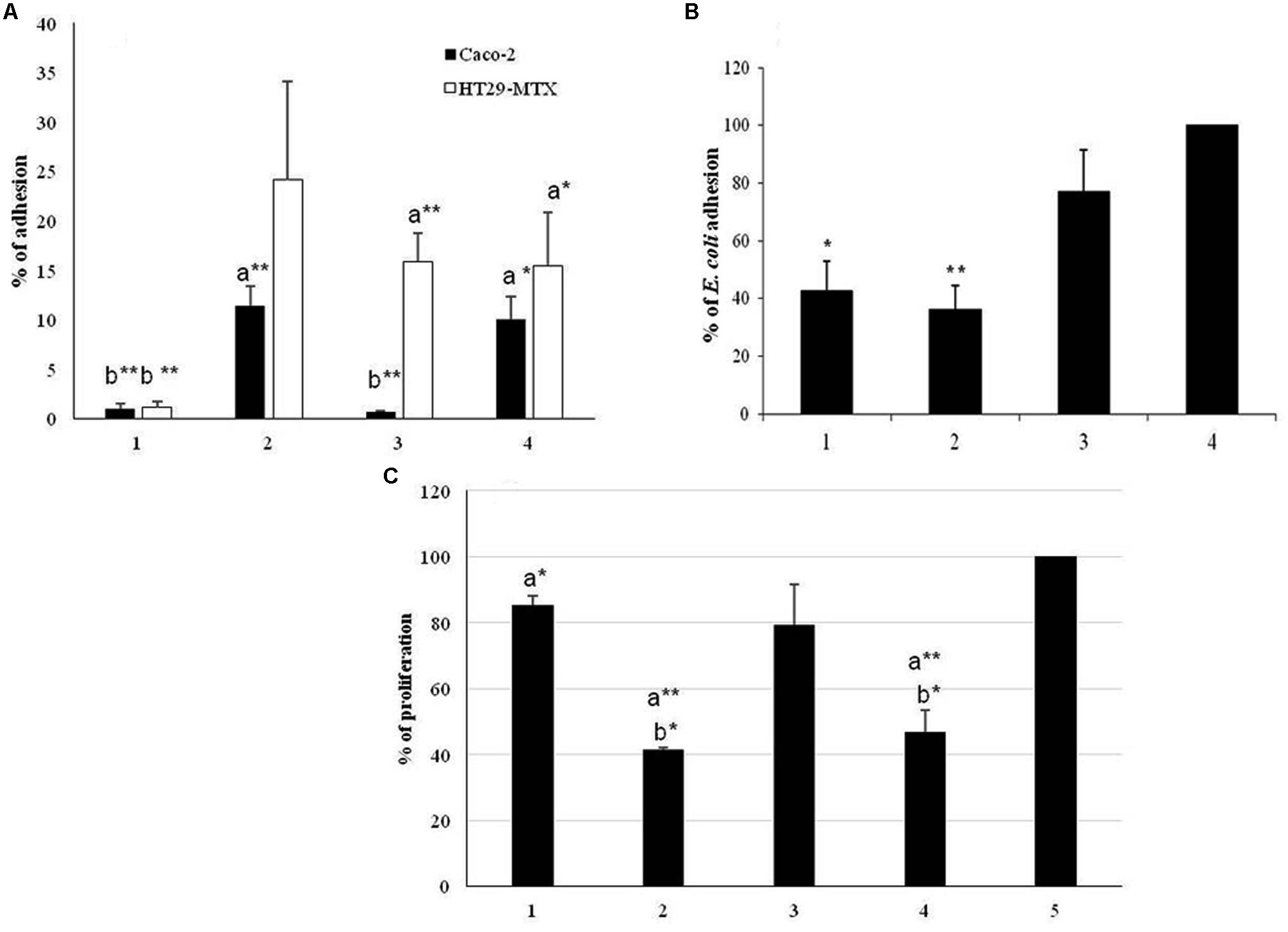
FIGURE 4. (A) Adhesion of the strain L. paracasei subsp. paracasei BGSJ2-83 and the EPS-SJ- mutant EPS7 to the human IEC lines Caco-2 (black bars) and HT29-MTX (white bars). 1. BGCG11; 2. BGSJ2-83; 3. EPS7; 4. BGHN14. The statistical differences between each strain with respect to the control strains are annotated with asterisks (∗p < 0.05, ∗∗p < 0.001) and letters (a – BGCG11 and b – BGHN14). (B) Association of E. coli to Caco-2 cells in the presence of L. paracasei subsp. paracasei BGSJ2-83, EPS7 and L. paraplantarum BGCG11. 1. E. coli/BGCG11; 2. E. coli/BGSJ2-83; 3. E. coli/EPS7; 4. E. coli. The statistical differences between each strain with respect to E. coli are annotated with asterisks (∗p < 0.05, ∗∗p < 0.001). (C) Proliferation of GALT cells in the presence of L. paracasei subsp. paracasei BGSJ2-83 and EPS7. 1. BGCG11; 2. BGSJ2-83; 3. EPS7; 4. BGHN14; 5. L cells. The statistical differences between each strain with respect to the control lymphocytes and control strain BGCG11 are annotated with asterisks (∗p < 0.05, ∗∗p < 0.001) and letters (a – GALT, b – BGCG11).
In parallel, the adhesion ability of strains BGSJ2-83 and EPS7 was tested on the HT29-MTX cell line, characterized by mucus production, which is the first barrier to the interaction of bacteria with IEC (Lesuffleur et al., 1990). Again, BGSJ2-83 showed a higher percentage of adhesion to HT29-MTX cells than EPS7, although in this case BGHN14 showed low adhesion ability, similar to EPS7 (Figure 4A).
Characterization of the Role of EPS-SJ in Decrease on E. coli Association to Caco-2 Cells
In this study, we analyzed the potential of EPS-SJ-producing and non-producing strains to compete with E. coli and to reduce the association capability of E. coli to Caco-2 epithelial cells (Figure 4B). The E. coli strain ATCC25922 was significantly less associated to Caco-2 cells in the presence of BGSJ2-83 and control strain BGCG11 than when it was analyzed alone, indicating the ability of all lactobacilli strains to diminish the association of E. coli to Caco-2 cells. E. coli exhibited the lowest association rate to the Caco-2 cell line when co-incubated with the strain BGSJ2-83, which produces EPS-SJ, as well as in the presence of control strain BGCG11. No significant differences were detected between the two strains. In contrast, when E. coli was co-incubated with EPS7 the association of E. coli to Caco-2 cells was reduced, but not to the same extent as in the presence of the EPS producing strains.
Effect of Insertional Mutagenesis on the Role of EPS-SJ on Proliferation of GALT-Isolated Lymphocytes
In order to monitor the effect of EPS-SJ’s presence on the cell surface of BGSJ2-83, the proliferation of the primary GALT culture in the presence of UV-irradiated cells of BGSJ2-83 and EPS7 was followed during 3 days of co-incubation. The strains BGCG11, producing high molecular weight EPS-CG11, and BGHN14, lacking epsSJ, were used as reference strains. The results are expressed as a percentage relative to the cells incubated in RPMI medium alone, with a standard error (Figure 4C). The results show that UV-irradiated cells of both BGSJ2-83 and EPS7 induced lower levels of lymphocyte proliferation compared to the control GALT-isolated lymphocytes. Interestingly, the level of proliferation of GALT-isolated lymphocytes determined for the EPS7 mutant was higher compared to BGSJ2-83, indicating that EPS-SJ P1, present on the cell surface of BGSJ2-83 and absent on the cell surface of EPS7, contributed to the lower proliferation of GALT-isolated lymphocytes.
Discussion
In this study, we have investigated the role of exopolysaccharide EPS-SJ, produced by the natural dairy isolate L. paracasei subsp. paracasei BGSJ2-8, in adhesion to IEC, decreased association of E. coli to Caco-2 cells and proliferation of GALT-isolated lymphocytes. Our results show that the unique epsSJ gene cluster identified in the chromosome of L. paracasei subsp. paracasei BGSJ2-8 is involved in the biosynthesis of strain-specific EPS-SJ, containing two EPS fractions, P1 and P2. Yasuda et al. (2008) remarked that L. casei Shirota produced EPS with two peaks of different molecular weight, named polysaccharide-peptidoglycan (PS-PG) polymer. In addition, Yasuda et al. (2008) showed that the mutation of different genes in the cluster from cps1A to cps1J modified the synthesis of high molecular weight-EPS (named PS1), but a smaller fraction (PS2) remained intact.
In our study, the results of a SEC-MALLS analysis revealed that insertional mutagenesis of the glps_2198 gene, encoding priming glycosyltransferase, led to the absence of the P2 fraction in EPS7, related to low molecular weight EPS-SJ (13,600 Da) present in BGSJ2-83, as well as to an increase in molecular weight of the P1 and its reduced production. According to literature data the gene encoding priming glycosyltransferase is highly evolutionarily conserved (Jolly and Stingele, 2001) and could be located at the beginning of the eps operons, as reported for L. helveticus NCC2745 (Jolly et al., 2002) and L. paraplantarum BGCG11 (Zivkovic et al., 2015), in the middle of the eps clusters, as in Streptococcus thermophilus strains (Stingele et al., 1996; Almiron-Roig et al., 2000), L. lactis NIZO B40 (Kleerebezem et al., 1999), and L. delbrueckii subsp. bulgaricus Lfi5 (Lamothe et al., 2002), or in the vicinity of the end of the eps clusters as in the case of L. rhamnosus (Péant et al., 2005) and here in the case of BGSJ2-8. The priming glycosyltransferase is involved in the first step of biosynthesis of an oligosaccharide “block.” It is a member of the EPS biosynthesis polyprenyl glycosyl phosphotransferases family with the role of transferring the sugar from UDP-glucose or UDP-galactose to a lipid carrier. The results obtained in this study revealed that interruption of the glps_2198 gene completely affected the biosynthesis of the low molecular weight P2 fraction of EPS-SJ, rather than the high molecular weight P1 fraction, although the P1 fraction is synthesized in lower amount. The results are in accordance to work of Mozzi et al. (2006) where it was reported that low molecular weight EPS are more common in lactic acid bacteria than high molecular weight. Hence, it could be deduced that the gene glps_2198 encoding priming glycosyltransferase in BGSJ2-83 is necessary for the first steps in biosynthesis of EPS-SJ.
Increased electron-density as a consequence of specific polysaccharide phosphotungstic acid/Alcian blue counterstaining suggests a significant ratio of EPS in the cell wall of this strain. These fibrillar protrusions probably correspond to EPS-SJ, the presence of which was subsequently confirmed by SEC-MALLS analysis, detected in BGSJ2-83.
The results from electron-microscopic analysis and cell wall measurement clearly indicate that both fractions of EPS-SJ, present on the cell surface of BGSJ2-83, contribute to the thickness of the cell wall in this strain. In fact, the absence of the P1 and P2 fractions in BGHN14 and EPS7, respectively, influenced the decrease of cell wall thickness by approximately 5 and 10 nm. Besides its lower content, this could also be a consequence of increased electron-density of the peptidoglycan layer due to increased polysaccharide content.
Probiotic lactobacilli exert their positive effect on human health by various mechanisms. The adhesive properties of probiotic strains provide several important abilities: the colonization of intestinal mucosa, persistence in the intestine, the competitive exclusion of pathogenic microorganisms, and immunomodulation (Muñoz-Provencio et al., 2009). In fact, the colonization of intestinal mucosa is an important criterion proposed by FAO/WHO guidelines for the selection of strains with probiotic potential, since their health-promoting effects might be partly dependent on their persistence in the intestine and adhesion to mucosal surfaces (Collado et al., 2009). Hence, the influence of EPS-SJ on the potential to adhere to the intestinal mucosal surface was examined using widely accepted in vitro models, Caco-2 and HT29-MTX cells.
It has been suggested that the adhesive properties of lactobacilli largely depend on the surface properties of probiotic strains (e.g., EPS), enabling them to interact with the mucosa and IEC (Segers and Lebeer, 2014). It can be assumed that the better adhesion of BGSJ2-83 and BGHN14 than the EPS7 mutant to Caco-2 cells is probably due to the presence of EPS-SJ P2 on the cell surface of strains BGSJ2-83 and BGHN14, which ensures better communication between bacteria and IEC. In contrast, the strain BGCG11, producing ropy EPS-CG11, was previously described to have low adhesion ability, which was correlated with the presence of EPS-CG11 on the cell surface (Nikolic et al., 2012).
It has been shown previously that the adhesion of bacteria to IEC is better in the absence of cell surface polysaccharides, due to better exposure of cell surface proteins in bacteria to the adhesin in eukaryotic cells (Ruas-Madiedo et al., 2006; Denou et al., 2008; Nikolic et al., 2012; Polak-Berecka et al., 2014; Dertli et al., 2015). Interestingly, EPS-SJ P2 is most likely positively involved in the adhesion of the BGSJ2-8 strain to IEC. Specifically, our results revealed that the presence of the EPS-SJ P2 around the bacteria increases adhesion to Caco-2 cells, in contrast to previously published data. Particularly, Nikolic et al. (2012) showed that presence of EPS-CG11 negatively affected adhesion of L. paraplantarum BGCG11 to IEC. In addition, Polak-Berecka et al. (2014) suggested that polysaccharides produced by L. rhamnosus E/N interfere with adhesion by masking protein receptors at the cell surface. However, the results of Ruas-Madiedo et al. (2006) demonstrated that the adhesion of bacteria to IEC depends on the EPS type and the dose. Such, the EPS GG produced by L. rhamnosus GG at the highest dose increased the adherence of Bifidobacterium animalis IPLA-R1. Probably it could be referred to differences in physicochemical and/or structural characteristics of the EPS polymers, as well as to the surface characteristics of the strains that contribute to the differences in adhesion (Ruas-Madiedo et al., 2006). Hence, it is likely that the molecular structure of EPS-SJ P2 matrix contributes to its adhesion to IEC, although the role of EPS molecules in the adhesion of bacteria to IEC still remains unclear.
Due to the fact that the presence of mucin in the HT29-MTX cell line simulates the bacterial interaction with gut mucus (McGuckin et al., 2011), the adhesion to HT29-MTX cells was also tested. In general, the tested strains showed a higher percentage of adhesion to the HT29-MTX cell line, while their adhesion ability to the Caco-2 cell line was lower. Similarly, the adhesion levels of strains L. rhamnosus DR20, L. acidophilus HN017, and Bifidobacterium lactis DR10 were two to three times higher to HT29-MTX cells compared to the degree of adhesion to Caco-2 cells (Gopal et al., 2001). These findings are in contrast to our previous results obtained with other putative probiotic strains, where the adhesion to Caco-2 cells was significantly higher than to HT29-MTX cells (Uroić et al., 2014). It is possible that the presence of the glycoprotein layer (mucin) on the surface of HT29-MTX cells participates in the interaction with the cell surface compounds of bacterial cells, which contribute to their hydrophobicity. Furthermore, the adhesion of strain BGHN14 and the EPS7 mutant, lacking the P1 and P2, respectively, was lower than the adhesion of BGSJ2-83 to HT29-MTX cells. However, there is no significant difference between the adhesion of BGHN14 and EPS7 strains to HT29-MTX, indicating that EPS-SJ P2 more specifically contributes to the adhesion of BGSJ2-83 and EPS7 to IEC than to the gut mucus matrix.
The other important health promoting property of probiotic strains is their capability to neutralize the negative effects of pathogens (FAO-WHO, 2006). This characteristic is shown to be strain-dependent and several mechanisms of action have been proposed to explain it: (i) a physical blocking of the pathogen’s entry (colonization competition), (ii) induction of mucus production, (iii) reinforcement of the selective permeability of the epithelium by increasing tight-junctions, (iv) production of antimicrobial factors, and, among others, (v) stimulation of the innate immune response (Gareau et al., 2010; Liévin-Le Moal and Servin, 2014). In this study we have shown that EPS-SJ-producing strain BGSJ2-83 exhibits higher adhesion to Caco-2 cells and is more capable of competing for the colonization of the intestinal niche than E. coli strain ATCC25922. Similarly, non-EPS producing derivative of EPS-CG11 (NB1) more efficiently reduced the association of Clostridium difficile or Yersinia enterocolitica than parental BGCG11 strain (Zivkovic et al., 2015). Interestingly, the presence of EPS-CG11-producing strain BGCG11, exhibiting low adhesion to Caco-2 cells, decreased the association of E. coli ATCC25922 to Caco-2 cells, similarly to previously obtained results by Zivkovic et al. (2015). Hence, it could be concluded that both EPS-CG11 and EPS-SJ are effective macromolecules protecting the IEC from pathogen binding regardless of their adhesion ability (Zivkovic et al., 2015). It is most likely that E. coli cells failed to access the IEC surface because they were trapped within the EPS matrix. It has been previously reported that different lactobacillus species have the ability to reduce the association of diverse enteropathogens to intestinal cells (García-Cayuela et al., 2014). This capability has also been attributed to antimicrobial activity related to the production of bacteriocins or organic acids (Satish-Kumar et al., 2011; Kaewnopparat et al., 2013). Since strain BGSJ2-83 is a BGSJ2-8 derivative lacking the plasmid carrying the genes responsible for bacteriocin production and does not exhibit antimicrobial activity, it is more likely that its capability is related to the presence of specific structural components on the cell surface. It has been proposed that the cell surface components involved in this feature could be cell surface associated proteins (Varma et al., 2010), S-layer macromolecules built from proteins (Zhang et al., 2010) and aggregation factors (Kaewnopparat et al., 2013), as well as EPS (Zivkovic et al., 2015). Hence, the results obtained in this study indicate that EPS-SJ could be involved in the decreased association of E. coli to Caco-2 cells. Since the results of this study show that association of E. coli with Caco-2 cells is higher in the presence of EPS7 than in the presence of BGSJ2-83, it can be concluded that EPS-SJ P2, lacking in EPS7, specifically contributes to this feature.
Finally, an important feature of potential probiotic candidates is the capacity to modulate the host’s immune response either in the terms of strengthening the immunological barrier against pathogen, or decreasing the immune responsiveness in exaggerated inflammatory conditions. The study on L. casei Shirota’s cps gene cluster has shown that this operon determines the synthesis of a specific polysaccharide moiety relevant for immune regulation (Yasuda et al., 2008). The production of high molecular weight PS1 was directly related to the suppressive effect of this strain upon IL-6 production in macrophages stimulated with LPS (Yasuda et al., 2008). The results of our study indicate that the presence of EPS-SJ on the cell surface contributes to the lower level of GALT-isolated lymphocyte proliferation. The level of GALT-isolated lymphocyte proliferation stimulated with EPS7 mutant was higher compared to BGSJ2-83, indicating that EPS-SJ P2, present on the cell surface of BGSJ2-83, has an inhibitory effect on GALT-isolated lymphocyte proliferation. The GALT is intestinal frontier and essential component of systemic immune response, which protects body from pathogens and foreign antigens. On the other hand it enables tolerance to commensal bacteria and dietary antigens and protects mucosa from detrimental inflammatory immune responses (Spahn and Kucharzik, 2004; Jung et al., 2010). Hence, GALT has a pivotal role in the decision between immune response and tolerance. The lower level of GALT-isolated lymphocytes proliferation in the presence of EPS-SJ-producing strain, BGSJ2-83, could indicate modulation of the immune response toward the tolerogenic environment. This feature could be useful in treatment of excessive inflammatory condition such as Crohn’s disease and other inflammatory disorders which occur as a result of an inappropriate innate and/or adaptive immune response. However, further experiments are needed in order to decipher the molecular mechanism of this feature.
Conclusion
The results obtained in this study indicate that EPS-SJ, specifically the P2 fraction, interacts with a certain host cell component, providing better gut colonization and pathogen exclusion. These results may contribute to the previous knowledge of the role of EPS in adhesion to IEC. Namely, it was established previously that polysaccharides hindered bacterial adhesion to host by masking protein receptors involved in the adherence to IEC. Here, we report that EPS may positively or negatively affect adherence of bacteria to the host cells.
Author Contributions
MŽ – conception and design of the work, performed main work, analyzed, interpreted and critically revised the data; MSM and MT – construction and characterization of the knock-out, draft of the work related to molecular genetics; PR-M – conception and design of SEC-MALLS, performed the SEC-MALLS analyses, draft related to SEC-MALLS and critically revised the manuscript; MBM – performed work and made part of the draft related to transmission electron microscopy; KV and MT – performed a work related to adhesion, E. coli association to Caco-2 cells, and GALT proliferation, made part of the draft and critically revised the manuscript; SS – EPS characterization; AK – conception and design, performed the work related to transmission electron microscopy, made the draft and critically revised the manuscript; NG – conception and the design of the work, supervised the complete work, analyzed and interpreted the data, draft the work, critically revised the manuscript. All authors finally approved the version to be published and agreed to be accountable for all aspects of the work in ensuring that questions related to the accuracy or integrity of any part of the work are appropriately investigated and resolved.
Funding
This work was funded by the Ministry of Education, Science and Technological Development of the Republic of Serbia, grant OI 173019. The founders had no role in study design, data collection and interpretation, or the decision to submit the work for publication.
Conflict of Interest Statement
The authors declare that the research was conducted in the absence of any commercial or financial relationships that could be construed as a potential conflict of interest.
Acknowledgments
T. Lesuffleur (INSERM U843, Paris, France) is acknowledged for the kind supply of HT29-MTX cellular line. Mengjin Liu and Roland Siezen are acknowledged for the Lactobacillus paracasei subsp. paracasei BGSJ2-8 genome annotation. We are grateful to Nathaniel Aaron Sprinkle, native English editor for the proofreading of the manuscript.
Abbreviations
CFU, colony forming units; CPS, capsular polysaccharides; EPS, extracellular polysaccharides; IEC, intestinal epithelial cells; GALT, gastrointestinal associated lymphoid tissue; LB, Luria Broth; MALLS, multi-angle laser light scattering; MRS, De Man-Rogosa-Sharpe medium; PBS, phosphate buffer solution; SEC, size exclusion chromatography.
Footnotes
References
Almiron-Roig, E., Mulholland, F., Gasson, M. J., and Griffin, A. M. (2000). The complete cps gene cluster from Streptococcus thermophilus NCFB 2392 involved in the biosynthesis of a new exopolysaccharide. Microbiology 146, 2793–2802. doi: 10.1099/00221287-146-11-2793
Boels, I. C., Ramos, A., Kleerebezem, M., and de Vos, W. M. (2001). Functional analysis of the Lactococcus lactis galU and galE genes and their impact on sugar nucleotide and exopolysaccharide biosynthesis. Appl. Environ. Microbiol. 67, 3033–3040. doi: 10.1128/AEM.67.7.3033-3040.2001
Cerning, J., Renard, C. M. G. C., Thibault, J. F., Bouillanne, C., Landon, M., Desmazeaud, M., et al. (1994). Carbon source requirements for exopolysaccharide production by Lactobacillus casei CG11 and partial structure analysis of the polymer. Appl. Environ. Microbiol. 60, 3914–3919.
Collado, M. C., Isolauri, E., Salminen, S., and Sanz, Y. (2009). The impact of probiotic on gut health. Curr. Drug Metab. 10, 68–78. doi: 10.2174/138920009787048437
de Vuyst, L., and Degeest, B. (1999). Heteropolysaccharides from lactic acid bacteria. FEMS Microbiol. Rev. 23, 153–177. doi: 10.1111/j.1574-6976.1999.tb00395.x
Delcour, J., Ferain, T., Deghorain, M., Palumbo, E., and Hols, P. (1999). The biosynthesis and functionality of the cell-wall of lactic acid bacteria. Antonie Van Leeuwenhoek 76, 159–184. doi: 10.1023/A:1002089722581
Denou, E., Pridmore, R. D., Berger, B., Panoff, J. M., Arigoni, F., and Brüssow, H. (2008). Identification of genes associated with the long-gut-persistence phenotype of the probiotic Lactobacillus johnsonii strain NCC533 using a combination of genomic and transcriptome analysis. J. Bacteriol. 190, 3161–3168. doi: 10.1128/JB.01637-07
Dertli, E., Mayer, M. J., and Narbad, A. (2015). Impact of the exopolysaccharide layer on biofilms, adhesion and resistance to stress in Lactobacillus johnsonii FI9785. BMC Microbiol. 15:8. doi: 10.1186/s12866-015-0347-2
EFSA (2007). Introduction of a qualified presumption of safety (QPS) approach for assessment of selected microorganisms referred to EFSA. EFSA J. 587, 1–16. doi: 10.2903/j.efsa.2007.587
FAO-WHO (2006). Probiotics in Food: Health and Nutritional Properties and Guidelines for Evaluation. FAO Food and Nutritional Paper No. 85 (ISBN 92-5-105513–105510). Rome: Food and Agriculture Organization of the United Nations, World Health Organization.
Filipic, B., Golic, N., Jovcic, B., Tolinacki, M., Bay, D. C., Turner, R. J., et al. (2013). The cmbT gene encodes a novel major facilitator multidrug resistance transporter in Lactococcus lactis. Res. Microbiol. 164, 46–54. doi: 10.1016/j.resmic.2012.09.003
García-Cayuela, T., Korany, A. M., Bustos, I., Gómez de Cadiñanos, L. P., Requena, T., Peláez, C., et al. (2014). Adhesion abilities of dairy Lactobacillus plantarum strains showing an aggregation phenotype. Food Res. Int. 57, 44–50. doi: 10.1016/j.foodres.2014.01.010
Gareau, M. G., Sherman, P. M., and Walker, W. A. (2010). Probiotics and the gut microbiota in intestinal health and disease. Nat. Rev. Gastroenterol. Hepatol. 7, 503–514. doi: 10.1038/nrgastro.2010.117
Gopal, P. K., Prasad, J., Smart, J., and Gill, H. S. (2001). In vitro adherence properties of Lactobacillus rhamnosus DR20 and Bifidobacterium lactis DR10 strains and their antagonistic activity against an enterotoxigenic Escherichia coli. Int. Food Microbiol. 67, 207–216. doi: 10.1016/S0168-1605(01)00440-8
Hanahan, D. (1983). Studies of transformation of Escherichia coli with plasmids. J. Mol. Biol. 166, 557–580. doi: 10.1016/S0022-2836(83)80284-8
Hidalgo-Cantabrana, C., Nikolic, M., López, P., Suárez, A., Miljkovic, M., Kojic, M., et al. (2014a). Exopolysaccharide-producing Bifidobacterium animalis subsp. lactis strains and their polymers elicit different responses on immune cells from blood and gut associated lymphoid tissue. Anaerobe 26, 24–30. doi: 10.1016/j.anaerobe.2014.01.003
Hidalgo-Cantabrana, C., Sánchez, B., Milani, C., Ventura, M., Margolles, A., and Ruas-Madiedo, P. (2014b). Genomic overview and biological functions of exopolysaccharide biosynthesis in Bifidobacterium spp. Appl. Environ. Microbiol. 80, 9–18. doi: 10.1128/AEM.02977-13
Holo, H., and Nes, I. F. (1995). “Transformation of Lactococcus by electroporation,” in Electroporation Protocols for Microorganisms: Methods in Molecular Biology, Vol. 47, ed. J. A. Nickoloff (New York City, NY: Humana Press), 195–199.
Hopwood, D. A., Bibb, J. M., Chater, K. F., Kieser, T., Bruton, C. J., Kieser, H. M., et al. (1985). Genetic Manipulation of Streptomyces. A Laboratory Manual. Norwich: The John Innes Foundation. doi: 10.1002/9780471729259.mc10e03s19
Jolly, L., Newell, J., Porcelli, I., Vincent, S. J., and Stingele, F. (2002). Lactobacillus helveticus glycosyltransferases: from genes to carbohydrate synthesis. Glycobiology 12, 319–327. doi: 10.1093/glycob/12.5.319
Jolly, L., and Stingele, F. (2001). Molecular organization and functionality of exopolysaccharide gene clusters in lactic acid bacteria. Int. Dairy J. 11, 733–745. doi: 10.1016/S0958-6946(01)00117-0
Jung, C., Hugot, J. P., and Barreau, F. (2010). Peyer’s Patches: the immune sensors of the intestine. Int. J. Inflam. 2010, 823710. doi: 10.4061/2010/823710
Kaewnopparat, S., Dangmanee, N., Kaewnopparat, N., Srichana, T., Chulasiri, M., and Settharaksa, S. (2013). In vitro probiotic properties of Lactobacillus fermentum SK5 isolated from vagina of a healthy woman. Anaerobe 22, 6–13. doi: 10.1016/j.anaerobe.2013.04.009
Kleerebezem, M., van Kranenburg, R., Tuiner, R., Boels, I. C., Looijesteijn, E., Hugenholtz, J., et al. (1999). Exopolysaccharides produced by Lactococcus lactis: from genetic engineering to improved reological properties? Antonie Van Leeuwenhoek 77, 357–365. doi: 10.1023/A:1002084822851
Kojic, M., Fira, D., Banina, A., and Topisirovic, L. (1991). Characterization of the cell-wall bound proteinases of Lactobacillus casei HN14. Appl. Environ. Microbiol. 57, 1753–1757.
Kojic, M., Lozo, J., Jovcic, B., Strahinic, I., Fira, D., and Topisirovic, L. (2010). Construction of a new shuttle vector and its use for cloning and expression of two plasmid-encoded bacteriocins from Lactobacillus paracasei subsp. paracasei BGSJ2-8. Int. J. Food Microbiol. 140, 117–124. doi: 10.1016/j.ijfoodmicro.2010.04.010
Lamothe, G. T., Jolly, L., Mollet, B., and Stingele, F. (2002). Genetic and biochemical characterization of exopolysaccharide biosynthesis by Lactobacillus delbrueckii subsp. bulgaricus. Arch. Microbiol. 178, 218–228. doi: 10.1007/s00203-002-0447-x
Law, J., Buist, G., Haandrikmann, A., Kok, J., Venema, G., and Leenhoust, K. (1995). A system to generate chromosomal mutations in Lactococcus lactis which allows fast analysis of targeted genes. J. Bacteriol. 177, 7011–7018.
Lebeer, S., Vanderleyden, J., and De Keersmaecker, S. C. (2008). Genes and molecules of lactobacilli supporting probiotic action. Microbiol. Mol. Biol. Rev. 72, 728–764. doi: 10.1128/MMBR.00017-08
Lesuffleur, T., Barbat, A., Dussaulx, E., and Zweibaum, A. (1990). Growth adaptation to methotrexate of HT-29 human colon-carcinoma cells is associated with their ability to differentiate into columnar and mucus-secreting cells. Cancer Res. 50, 6334–6343.
Liévin-Le Moal, V., and Servin, A. L. (2014). Anti-infective activities of Lactobacillus strains in the human intestinal microbiota: from probiotics to gastrointestinal anti-infectious biotherapeutic agents. Clin. Microbiol. Rev. 27, 167–199. doi: 10.1128/CMR.00080-13
López, P., Monteserín, D. C., Gueimonde, M., de los Reyes-Gavilán, C. G., Margolles, A., Suárez, A., et al. (2012). Exopolysaccharide-producing Bifidobacterium strains elicit different in vitro responses upon interaction with human cells. Food Res. Int. 46, 99–107. doi: 10.1016/j.foodres.2011.11.020
Lozo, J., Jovcic, B., Kojic, M., Dalgalarrondo, M., Chobert, J., Haertlé, T., et al. (2007). Molecular characterization of a novel bacteriocin and an unusually large aggregation factor of Lactobacillus paracasei subsp. paracasei BGSJ2-8, a natural isolate from home-made cheese. Curr. Microbiol. 55, 266–271. doi: 10.1007/s00284-007-0159-1
Lukic, J., Strahinic, I., Milenkovic, M., Golic, N., Kojic, M., Topisirovic, L., et al. (2013). Interaction of Lactobacillus fermentum BGHI14 with rat colonic mucosa: implications for colitis induction. Appl. Environ. Microbiol. 79, 5735–5744. doi: 10.1128/AEM.01807-13
Maguin, E., Prevost, H., Ehrlich, S. D., and Gruss, A. (1996). Efficient insertional mutagenesis in lactococci and other gram-positive bacteria. J. Bacteriol. 178, 931–935.
McGuckin, M. A., Lindén, S. K., Sutton, P., and Florin, T. H. (2011). Mucin dynamics and enteric pathogens. Nat. Rev. Microbiol. 9, 265–278. doi: 10.1038/nrmicro2538
Miljkovic, M., Strahinic, I., Tolinacki, M., Zivkovic, M., Kojic, S., Golic, N., et al. (2015). AggLb is the largest cell-aggregation factor from Lactobacillus paracasei subsp. paracasei BGNJ1-64, functions in collagen adhesion, and pathogen exclusion in vitro. PLoS ONE 10:e0126387. doi: 10.1371/journal.pone.0126387
Mozzi, F., Vaningelgem, F., Hébert, E. M., Van der Meulen, R., Moreno, M. R. F., de Valdez, G., et al. (2006). Diversity of heteropolysaccharide-producing lactic acid bacterium strains and their biopolymers. Appl. Environ. Microbiol. 72, 4431–4435. doi: 10.1128/AEM.02780-05
Muñoz-Provencio, D., Liopis, M., Antolin, M., de Torres, I., Guarner, F., Perez-Martinez, G., et al. (2009). Adhesion properties of Lactobacillus casei strain to resected intenstinal fragments and components of the extracellular matrix. Arch. Microbiol. 191, 153–161. doi: 10.1007/s00203-008-0436-9
Nagaoka, M., Muto, M., Nomoto, K., Matsuzaki, T., Watanabe, T., and Yokokura, T. (1990). Structure of polysaccharide-peptidoglycan complex from the cell wall of Lactobacillus casei YIT 9018. J. Biochem. 108, 568–571.
Nikolic, M., López, P., Strahinic, I., Suárez, A., Kojic, M., Fernández-García, M., et al. (2012). Characterisation of the exopolysaccharide (EPS)-producing Lactobacillus paraplantarum BGCG11 and its non-EPS producing derivative strains as potential probiotics. Int. J. Food Microbiol. 158, 155–162. doi: 10.1016/j.ijfoodmicro.2012.07.015
Péant, B., LaPointe, G., Gilbert, C., Atlan, D., Ward, P., and Roy, D. (2005). Comparative analysis of the exopolysaccharide biosynthesis gene clusters from four strains of Lactobacillus rhamnosus. Microbiology 151, 1839–1851. doi: 10.1099/mic.0.27852-0
Polak-Berecka, M., Waśko, A., Paduch, R., Skrzypek, T., and Sroka-Bartnicka, A. (2014). The effect of cell surface components on adhesion ability of Lactobacillus rhamnosus. Antonie Van Leeuwenhoek 106, 751–762. doi: 10.1007/s10482-014-0245-x
Ruas-Madiedo, P., Gueimonde, M., Margolles, A., de los Reyes-Gavilán, C. G., and Salminen, S. (2006). Exopolysaccharides produced by probiotic strains modify the adhesion of probiotics and enteropathogens to human intestinal mucus. J. Food Prot. 69, 2011–2015.
Sambrook, J., Fritsch, E. F., and Maniatis, T. (1989). Molecular Cloning: A Laboratory Manual, 2nd Edn. New York, NY: Cold Spring Harbor Laboratory.
Satish-Kumar, R., Kanamani, P., Yuvaraj, N., Paari, K. A., Pattukumar, V., and Arul, V. (2011). Lactobacillus plantarum AS1 binds to cultured human intestinal cell line HT-29 and inhibits cell attachment by enterovirulent bacterium Vibrio parahaemolyticus. Lett. Appl. Microbiol. 53, 481–487. doi: 10.1111/j.1472-765X.2011.03136.x
Schär-Zammaretti, P., and Ubbnik, J. (2003). The cell wall of lactic acid bacteria: surface constituents and macromolecular conformations. Biophys. J. 85, 4076–4092. doi: 10.1016/S0006-3495(03)74820-6
Segers, M. E., and Lebeer, S. (2014). Towards a better understanding of Lactobacillus rhamnosus GG – host interactions. Microb. Cell Fact. 13(Suppl. 1), S7. doi: 10.1186/1475-2859-13-S1-S7
Servin, A. L. (2004). Antagonistic activities of lactobacilli and bifidobacteria against microbial pathogens. FEMS Microbiol. Rev. 28, 405–440. doi: 10.1016/j.femsre.2004.01.003
Spahn, T. W., and Kucharzik, T. (2004). Modulating the intestinal immune system: the role of lymphotoxin and GALT organs. Gut 53, 456–465. doi: 10.1136/gut.2003.023671
Stingele, F., Neeser, J. R., and Mollet, B. (1996). Identification and characterization of the eps (Exopolysaccharide) gene cluster from Streptococcus thermophilus Sfi6. J. Bacteriol. 178, 1680–1690.
Uroić, K., Nikolić, M., Kos, B., Leboš Pavunc, A., Beganović, J., Lukić, J., et al. (2014). Probiotic properties of lactic acid bacteria isolated from Croatian fresh soft cheese and Serbian white pickled cheese. Food Technol. Biotechnol. 52, 232–241.
Varma, P., Dinesh, K. R., Menon, K. K., and Biswas, R. (2010). Lactobacillus fermentum isolated from human colonic mucosal biopsy inhibits the growth and adhesion of enteric and foodborne pathogens. J. Food Sci. 75, M546–M551. doi: 10.1111/j.1750-3841.2010.01818.x
Yasuda, E., Serata, M., and Sako, T. (2008). Suppressive effect on activation of macrophages by Lactobacillus casei strain Shirota genes determining the synthesis of cell wall-associated polysaccharides. Appl. Environ. Microbiol. 74, 4746–4755. doi: 10.1128/AEM.00412-08
Zhang, Y. C., Zhang, L. W., Tuo, Y. F., Guo, C. F., Yi, H. X., Li, J. Y., et al. (2010). Inhibition of Shigella sonnei adherence to HT29 cells by lactobacilli from Chinese fermented food and preliminary characterization of S-layer protein involvement. Res. Microbiol. 161, 667–672. doi: 10.1016/j.resmic.2010.06.005
Zivkovic, M., Hidalgo-Cantabrana, C., Kojic, M., Gueimonde, M., Golic, N., and Ruas-Madiedo, P. (2015). Capability of exopolysaccharide-producing Lactobacillus paraplantarum BGCG11 and its non-producing isogenic strain NB1, to counteract the effect of enteropathogens upon the epithelial cell line HT29-MTX. Food Res. Int. 74, 199–207. doi: 10.1016/j.foodres.2015.05.012
Keywords: Lactobacillus paracasei, EPS-SJ, adhesion, E. coli association, GALT lymphocytes
Citation: Živković M, Miljković MS, Ruas-Madiedo P, Markelić MB, Veljović K, Tolinački M, Soković S, Korać A and Golić N (2016) EPS-SJ Exopolisaccharide Produced by the Strain Lactobacillus paracasei subsp. paracasei BGSJ2-8 Is Involved in Adhesion to Epithelial Intestinal Cells and Decrease on E. coli Association to Caco-2 Cells. Front. Microbiol. 7:286. doi: 10.3389/fmicb.2016.00286
Received: 06 October 2015; Accepted: 23 February 2016;
Published: 09 March 2016.
Edited by:
Andrea Gomez-Zavaglia, Center for Research and Development in Food Cryotechnology – National Scientific and Technical Research Council, ArgentinaReviewed by:
Michael Gänzle, University of Alberta, CanadaMicaela Medrano, Consejo Nacional de Investigaciones Científicas y Técnicas, Argentina
Copyright © 2016 Živković, Miljković, Ruas-Madiedo, Markelić, Veljović, Tolinački, Soković, Korać and Golić. This is an open-access article distributed under the terms of the Creative Commons Attribution License (CC BY). The use, distribution or reproduction in other forums is permitted, provided the original author(s) or licensor are credited and that the original publication in this journal is cited, in accordance with accepted academic practice. No use, distribution or reproduction is permitted which does not comply with these terms.
*Correspondence: Nataša Golić, bmF0YXNhZ0BpbWdnZS5iZy5hYy5ycw==