- Department of Medicine, University of California at San Diego, La Jolla, CA, USA
Diarrheal diseases are among the leading causes of morbidity and mortality in the world, particularly among young children. A limited number of infectious agents account for most of these illnesses, raising the hope that advances in the treatment and prevention of these infections can have global health impact. The two most important parasitic causes of diarrheal disease are Cryptosporidium and Giardia. Both parasites infect predominantly the small intestine and colonize the lumen and epithelial surface, but do not invade deeper mucosal layers. This review discusses the therapeutic challenges, current treatment options, and drug development efforts against cryptosporidiosis and giardiasis. The goals of drug development against Cryptosporidium and Giardia are different. For Cryptosporidium, only one moderately effective drug (nitazoxanide) is available, so novel classes of more effective drugs are a high priority. Furthermore, new genetic technology to identify potential drug targets and better assays for functional evaluation of these targets throughout the parasite life cycle are needed for advancing anticryptosporidial drug design. By comparison, for Giardia, several classes of drugs with good efficacy exist, but dosing regimens are suboptimal and emerging resistance begins to threaten clinical utility. Consequently, improvements in potency and dosing, and the ability to overcome existing and prevent new forms of drug resistance are priorities in antigiardial drug development. Current work on new drugs against both infections has revealed promising strategies and new drug leads. However, the primary challenge for further drug development is the underlying economics, as both parasitic infections are considered Neglected Diseases with low funding priority and limited commercial interest. If a new urgency in medical progress against these infections can be raised at national funding agencies or philanthropic organizations, meaningful and timely progress is possible in treating and possibly preventing cryptosporidiosis and giardiasis.
The two most frequent parasitic causes of diarrheal disease worldwide are Cryptosporidium and Giardia. Other parasites, such as Entamoeba histolytica, which is highly invasive and causes amebic colitis (as discussed in other chapters of this volume), and the coccidia, Cystoisospora belli, and Cyclospora cayetanensis, have clinical relevance but are less common causes of diarrheal illness. For instance, the two coccidian parasites are mostly associated with chronic diarrhea in HIV-positive individuals (Dash et al., 2013; Mathur et al., 2013) and other forms of immunodeficiency, but are less important causes of diarrhea in immunocompetent individuals. This review focuses on the therapeutic challenges, current treatment options, and ongoing drug development efforts against cryptosporidiosis and giardiasis.
Cryptosporidium
Cryptosporidium is a major cause of diarrheal disease in humans and animals. It became clinically first known as a causative agent of profuse and persistent diarrhea and morbidity in immunocompromised patients, particularly those with acquired immunodeficiency syndrome, and as a major cause of waterborne diarrheal disease in outbreaks in otherwise healthy individuals. More recently, large population studies have shown that Cryptosporidium is among the five leading causes of diarrheal disease in young children worldwide (Checkley et al., 2015), underlining the urgency of addressing the medical needs posed by this parasite, particularly since current treatment options are severely limited.
Parasite
Cryptosporidium belongs to the phylum of apicomplexan protists, along with Plasmodium and Toxoplasma, although it displays significant differences to those parasites. Most notably, it has lost the phylum-defining apicomplexan plastid and lacks mitochondria. Genome comparisons suggest that Cryptosporidium is more closely related to gregarines, intestinal protozoa of invertebrates (Carreno et al., 1999; Ryan and Hijjawi, 2015). The parasite is an obligate endosymbiont, depending on invasion of host cells for numerous metabolic functions. Consistent with the exploitation of this metabolically rich biological niche, it has a relatively small eukaryotic genome of ∼9 Mb with ∼4,000 genes (Abrahamsen et al., 2004; Xu et al., 2004). Cryptosporidium represents a species complex comprising at least 27 individual species and over 40 genotypes with varying degrees of host specificity (Ryan and Hijjawi, 2015). Humans can be infected by nearly 20 of these species, but only two, Cryptosporidium hominis and C. parvum, cause the majority of clinically relevant infections. C. hominis is limited to humans, so the infectious cycle is strictly anthroponotic, while C. parvum has several subtypes of which some are human-specific and others have a broader host range and zoonotic transmission. Importantly, new drugs must be active against C. parvum and C. hominis as both species have worldwide distribution.
The entire Cryptosporidium life cycle occurs in a single host (monoxenous) and involves both asexual multiplication and sexual reproduction (Laurent et al., 1999) (Figure 1). Infectious oocysts are ingested by the host, and sporozoites emerge from the oocysts upon exposure to acidic conditions followed by neutralization and exposure to pancreatic enzymes and bile (Smith et al., 2005). Sporozoites attach to intestinal epithelial cells, are enveloped by the host cell apical cell membrane, and differentiate into spherical trophozoites, which occupy a location that is commonly described as intracellular but extracytoplasmic (Smith et al., 2005). The parasites reside in a parasitophorous vacuole, which contains membrane components from the host and parasite, and allows acquisition of nutrients from the host cell (Tzipori and Griffiths, 1998). Importantly, the parasite is completely covered by host cell membrane during its epithelial growth phase, so drugs have to cross this membrane to be effective at that stage of the growth cycle.
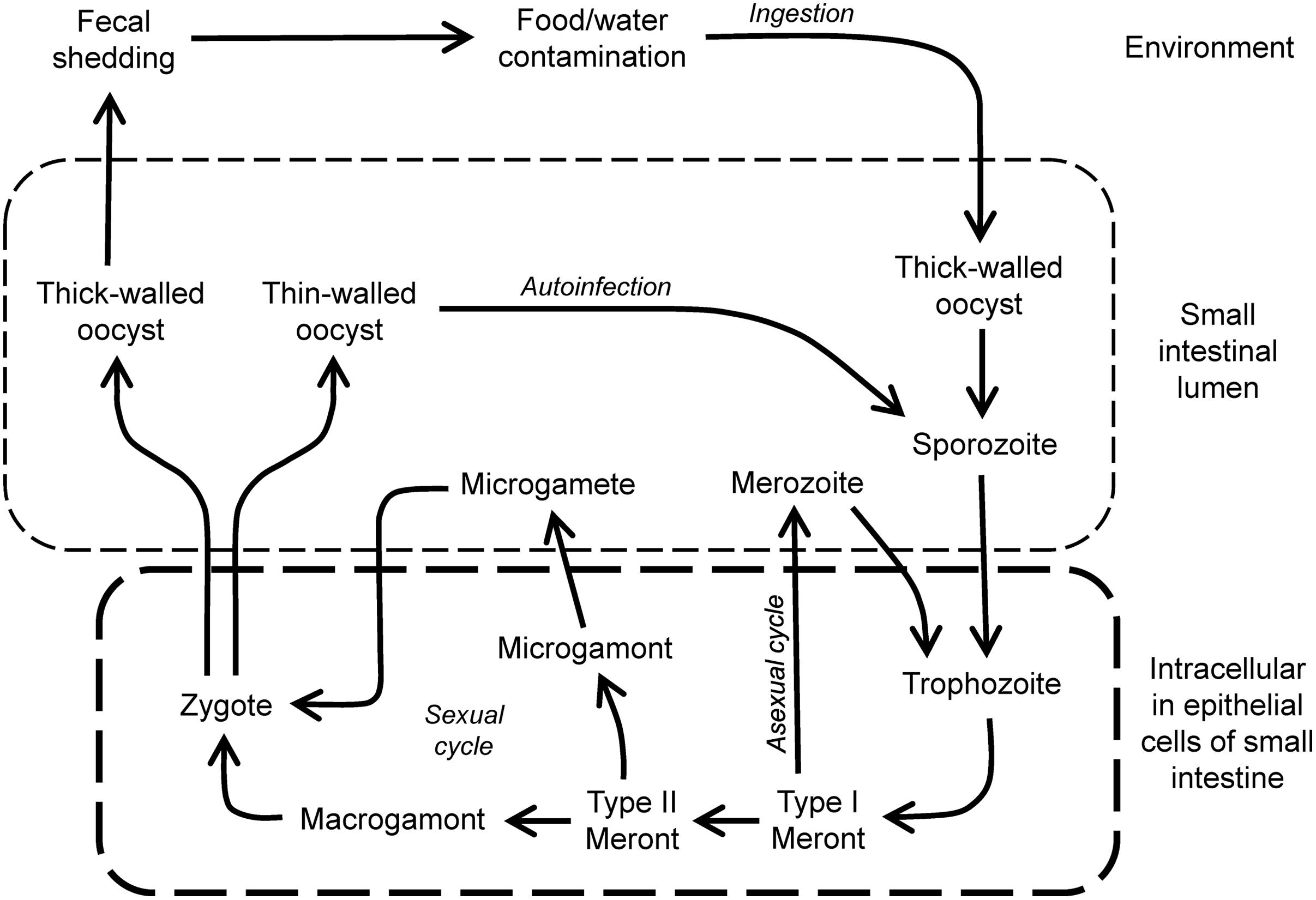
FIGURE 1. Life cycle of Cryptosporidium. Infection is initiated by ingestion of thick-walled and environmentally resistant oocysts that give rise to sporozoites in the lumen of the small intestine. These invade epithelial cells to form trophozoites in the apical domain of the cells. Growth occurs by asexual multiplication, leading to further cycles of infection and growth, or sexual multiplication involving gamonts and gametes, and leading to fertilized zygotes. These can differentiate into thin-walled oocysts that can initiate further rounds of autoinfection, or to thick-walled oocysts that are shed in the feces.
During maturation of the Cryptosporidium trophozoite, asexual multiplication occurs and results in the formation of a type I schizont that contains six to eight merozoites. Rupture of the schizont results in the release of merozoites that, in turn, can invade adjacent host epithelial cells, where they develop subsequently into type I schizonts, leading to further rounds of asexual multiplication, or into type II schizonts, which initiate sexual reproduction by differentiating into male microgamonts or female macrogamonts (Current and Reese, 1986). Male microgamonts release microgametes that can fertilize the macrogametes inside the female macrogamont. After fertilization, two types of oocysts form, thin-walled oocysts, which are important in reinfection of the host and expansion of parasite numbers, and thick-walled oocysts, which exit the intestinal tract and are infectious for new hosts.
Pathogenesis and Disease
Transmission occurs by the fecal–oral spread of oocysts. In particular, fecal contamination of drinking water can serve as a vehicle for transmission of oocysts and is a substantial public health concern. Large-scale outbreaks have been associated with contamination of community drinking water (Widerstrom et al., 2014; Painter et al., 2015). Cryptosporidium invades and resides for major parts of its life cycles within epithelial cells, most commonly in the small intestine. The parasite can be viewed as a “minimally invasive” mucosal pathogen, because it does not usually penetrate into the deeper mucosal layers. This restricted epithelial localization has potential implications for drug design, as it raises the possibility that orally administered drugs might be effective locally in the intestine without extensive systemic absorption. Under conditions of immunodeficiency, Cryptosporidium infection can be more widespread and involve epithelial cells of the biliary tract, pancreatic duct, stomach, esophagus, and even respiratory tract (Chen et al., 2002). Under these conditions, systemic drug absorption is probably required, as a drug with limited intestinal activity would be unlikely to reach these other mucosal sites (Abubakar et al., 2007).
Clinical manifestations of Cryptosporidium infection, which occur after an incubation period of 2–14 days, include watery and often profuse diarrhea, as well as abdominal cramps, nausea, vomiting, weight loss, and a low-grade fever (Chen et al., 2002). In immunocompetent individuals, disease is usually self-limited lasting 1–3 weeks, whereas the illness is typically prolonged in immunocompromised hosts. In addition, in severe infections, malabsorption can be present due to decreased absorptive surface, which can contribute to the wasting syndrome in infected AIDS patients (Hunter and Nichols, 2002). Immunocompromised patients with bile duct infection may also present with jaundice secondary to biliary tract obstruction or with symptoms of pancreatitis. Furthermore, in young children, particularly if malnourished, the infectious diarrhea can be extensive and associated with significant mortality (Hunter and Nichols, 2002). In fact, cryptosporidiosis has recently been identified as the second leading cause (after rotavirus) of diarrheal disease in children <5 years in developing countries (Kotloff et al., 2013; Liu et al., 2014). With increasing use of the highly effective rotavirus vaccine it can be expected that Cryptosporidium will soon become the most important worldwide cause of childhood diarrhea.
Several mechanisms are thought to contribute to the pathogenesis of Cryptosporidium-induced diarrhea. In patients having large volume diarrhea, a secretory process appears to be important. An enterotoxic activity released by the parasite has been described (Guarino et al., 1995), but these findings remain controversial. In animal models of C. parvum infection, glucose-stimulated sodium absorption was inhibited and this paralleled the extent of villous and epithelial cell damage (Kapel et al., 1997). In addition, increased mucosal prostaglandin production (e.g., PGE2 and PGI2), which can inhibit neutral NaCl absorption and result in secretory diarrhea, has been demonstrated in the porcine model (Argenzio et al., 1990). Such effects may be due, in part, to PGI2 acting on components of the enteric nervous system (i.e., cholinergic and VIPergic neuronal pathways), and a direct action of PGE2 on enterocytes (Argenzio et al., 1993, 1996). The mechanisms that lead to increased prostaglandin production and the cellular source of the prostaglandins are not known, although the latter may include mesenchymal and/or epithelial cells (Laurent et al., 1998). In addition, resident and recruited leukocytes in the mucosa (e.g., macrophages) have the potential to produce high levels of prostaglandins, and may also be a source of prostaglandin production during C. parvum infection. Alterations in intestinal permeability may also play a role in the diarrhea in C. parvum-infected individuals (Zhang et al., 2000).
Current Treatment Options
Current therapeutic options for cryptosporidiosis are limited and only one drug, nitazoxanide, has been approved by the Food and Drug Administration (FDA). Efficacy is variable, with resolution of diarrheal symptoms and parasitological cure in 50–90% of HIV-negative children and adults (Rossignol et al., 1998, 2001; Amadi et al., 2002; Hussien et al., 2013). Typical oral doses are 500-mg twice daily for adults and adolescents, 200-mg doses twice daily for children aged 4–11 years, and 100-mg doses twice daily for children aged 1–3 years, with treatment duration of 3–14 days. The drug is generally well tolerated. Notably, placebo treatment resulted in symptom improvements in a significant fraction (30–40%) of patients (Rossignol et al., 2001; Amadi et al., 2002), underlining that the infection is usually self-limited in immunocompetent patients. In contrast, nitazoxanide is not effective in HIV-infected children (Amadi et al., 2002), and is not FDA approved for this indication.
Other pharmacological interventions have been used clinically against Cryptosporidium, but they are not FDA-approved for treating cryptosporidiosis and their efficacy is generally lower than nitazoxanide. For example, paromomycin (500 mg oral, four times daily for 2–3 weeks) led to symptom cessation and microbiological cure in 60–70% of immunocompetent patients (Vandenberg et al., 2012; Hussien et al., 2013). Results in HIV-infected adults have been variable, with some studies showing similar (60–70%) efficacy of paromomycin (Bissuel et al., 1994; Blanshard et al., 1997), while others reporting no effectiveness (Hewitt et al., 2000). Immunotherapy has shown some promise. Most notably, restoration of immune competence in immunocompromised hosts, particularly an increase toward normal in the CD4 T cell levels in patients with AIDS, is associated with clearance of Cryptosporidium (Flanigan et al., 1992). Passive immunotherapy by oral administration of colostrum-derived bovine immunoglobulins (which presumably contained anti-cryptosporidial antibodies as cows get commonly infected with Cryptosporidium) led to significant improvement of diarrheal symptoms, although microbiological cure was not evaluated (Greenberg and Cello, 1996). However, nitazoxanide remains the most effective current therapeutic agent available against cryptosporidiosis in immunocompetent individuals, while no consistently effective drugs exist against infection under conditions of immunodeficiency.
Assays for Drug Development
Antimicrobial drug development depends on suitable assays that recapitulate critical growth phases of the microbe in the host. This is not usually a problem for growth autonomous microorganisms, such as most bacterial pathogens, but can be a challenge for symbionts such as Cryptosporidium that rely partially or completely on host cells for growth and survival. Nonetheless, an array of cell culture and animal models is available for drug testing against the parasite.
Asexual and sexual development of Cryptosporidium can be achieved in vitro using several different cell lines, although the life cycle is incomplete and the number of oocysts produced is generally low. A widely used model is the human ileocecal adenocarcinoma cell line, HCT-8 (Upton et al., 1994; Alcantara Warren et al., 2008). Monolayers of the cells can be readily infected in culture (Figure 2), and infection can be optimized by adding nutritional supplements to the culture media (Upton et al., 1995). Other mammalian cell lines (e.g., MDCK and Vero) are also infectable, although parasite development tends to be less robust than in HCT-8 cells (Upton et al., 1994). Several of the epithelial cell lines, including human T84 and Caco-2 cells, can be grown as differentiated polarized monolayers on microporous filter supports, which permits studies of the effects of Cryptosporidium infection on barrier functions, ion absorption and secretion, and cytokine responses (Griffiths et al., 1994; Laurent et al., 1997, 1998). A recent report has also used primary human intestinal epithelial cells, grown as self-sustaining organoids (enteroids), for in vitro infections with Cryptosporidium (Castellanos-Gonzalez et al., 2013a). These cultures, unlike epithelial cell lines, display the normal range of intestinal epithelial cell differentiation, which should allow more complete analysis of parasite–host interactions than possible in transformed cell lines, although establishment and maintenance of primary cultures are technically demanding at this time and may not yet be readily adaptable to high-throughput screens. Overall, epithelial cell cultures are poised to remain an indispensable tool for new drug development against Cryptosporidium. It must be borne in mind, however, that current culture systems probably do not capture all stages of the cryptosporidial life cycle with equal efficiency and physiological impact, so drug screening efforts may be skewed toward compounds that are particularly active in the intracellular life cycle stages, but not any extracellular stages (Hijjawi et al., 2002).
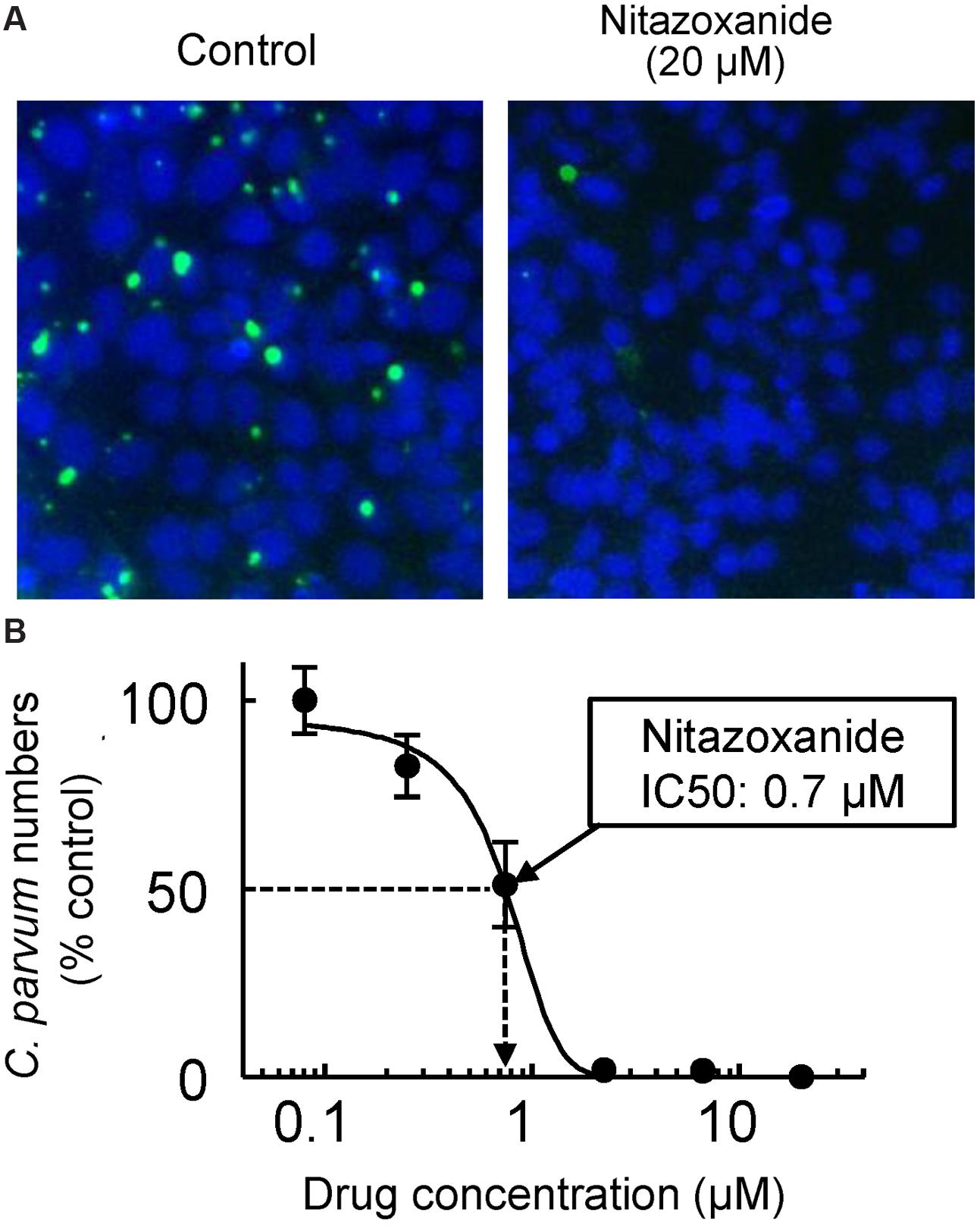
FIGURE 2. In vitro drug assay for Cryptosporidium. HCT-8 human intestinal epithelial cells were grown as monolayers in 96-well plates to 60–80% confluence. Cryptosporidium parvum oocysts, pretreated for 10 min at 37°C with 10 mM HCl and 10 min at 15°C with 200 μM sodium taurocholate to promote excystation, were added to the wells (105 oocysts/well). After a 4 h infection period, monolayers were washed, serial dilutions of nitazoxanide were added, and cultures were incubated for 48 h at 37°C in 5% CO2 and 95% air. Cells were fixed with 3% formaldehyde, permeabilized with Triton X-100, and stained with FITC-conjugated Vicia villosa lectin to detect C. parvum and counterstained with Hoechst 33342 to detect nuclei. A representative image of host cell nuclei (blue) and parasites (green) is shown in (A) for controls (left) and after exposure to 20 μM nitazoxanide (right). A concentration-response curve is shown in (B). Data are mean ± SD of triplicate wells, and are expressed relative to the parasite load in untreated controls. The half-maximal inhibitory concentration (IC50) for nitazoxanide was 0.7 μM.
Antimicrobial activity in vitro is usually necessary for a new drug candidate, but it is not sufficient since many candidates that work in vitro fail to be active in vivo. Appropriate animal models are therefore critical for drug development. A number of mammals have been used for Cryptosporidium infections, including small (mice, rats) and large animals (pigs, cows), each with advantages and disadvantages. Most animal models reproduce the cryptosporidial growth cycle and lead to sustained infection and shedding of infectious oocysts, which is adequate for drug efficacy studies. Small animal models, particularly mice, are resource-effective, but are not associated with the characteristic infection-induced diarrhea seen in humans and can thus not be used for investigations of this clinical manifestation and its treatment. On the other hand, large animals such as piglets and calves develop marked diarrhea (Tzipori et al., 1981, 1983; Argenzio et al., 1993; Gookin et al., 2008; Adell et al., 2013; Operario et al., 2015), but require greater resources and are less amenable to genetic and pharmacological manipulations for mechanistic studies.
Mice are the most commonly used models for in vivo testing of drug candidates against Cryptosporidium. Newborn mice are susceptible to infection with C. parvum during the initial 3 weeks after birth, but subsequently become resistant to infection. Consequently, adult wild-type mice are generally not suitable for drug testing, which is presumably due to effective innate or acquired immune defenses. However, adult mice with selected immunodeficiencies can be readily infected with the parasite. For example, SCID mice (Kuhls et al., 1992) and mice deficient in IFN-γ or its receptor (Theodos et al., 1997; von Oettingen et al., 2008), major histocompatibility complex class II (Aguirre et al., 1994), CD40 or CD40 ligand (Cosyns et al., 1998; Hayward et al., 2001), or IL-12 p40 (Urban et al., 1996; Campbell et al., 2002) are all susceptible to intestinal C. parvum infection and have been used to varying degrees for drug testing. The observation that nitazoxanide has efficacy in immunocompetent patients, but less so or not at all in immunodeficient individuals suggests that intact host immunity contributes to drug effectiveness. It follows that the nature of an immune defect that renders adult mice susceptible to Cryptosporidium may impact the predictive value of in vivo drug testing. However, few if any comparative drug efficacy studies have been done between different murine models, so it is not clear whether any particular model is more representative of the human situation than others and thus potentially more predictive of clinical efficacy.
New Agents against Cryptosporidium
Antimicrobial drug development commonly proceeds along one of two pathways, focused either on whole-cell activity or on specific molecular targets. In an activity-centered approach, compound libraries are screened for activity against the live parasite in a suitable in vitro assay. Upon identification of biologically active “hits”, the respective compounds are systematically optimized for in vitro and ultimately in vivo activity. In a target-centered approach, the focus is on exploiting molecular targets that are indispensable and, ideally, unique to the parasite. Target identification may come from genomic analyses or genetic screens, and is followed by pharmacological exploration of their “druggability” with suitable inhibitors. Both approaches have advantages and shortcomings. Activity-centered drug development can rapidly lead to strong hits and lead compounds, but if those fail in subsequent preclinical or clinical trials, development might run into a dead end because the molecular target is often not known can thus not be further exploited with alternative chemical scaffolds. Target-centered drug development is conceptually compelling, as it promises systematic exploitation of genome information without the uncertainty of large library screens. However, targets may not prove as physiologically critical as initially predicted, and development of specific inhibitors with adequate activity in vitro and in vivo may not be straightforward. Furthermore, tools for genetic manipulation of Cryptosporidium are not presently available, precluding genetic screens as a strategy for identifying critical target genes in this parasite. To date, activity-centered approaches have yielded most if not all clinically utilized antimicrobials, while target-centered approaches remain an intriguing but unfilled promise.
Both drug development strategies have been applied to Cryptosporidium. In particular, the obligate endosymbiotic growth phase has sparked great interest in the systematic exploitation of this apparent “Achilles’ heel” of the parasite. Cryptosporidium has degenerate mitosomes instead of mitochondria and has lost the mitochondrial genome and nuclear genes for many mitochondrial proteins, including those required for the tricarboxylic acid cycle, oxidative phosphorylation, and fatty acid oxidation (Abrahamsen et al., 2004; Xu et al., 2004). Also lacking are genes for de novo biosynthesis of amino acids, nucleotides, and sugars (Abrahamsen et al., 2004; Xu et al., 2004). Loss of genes from multiple metabolic pathways indicates that the parasite relies heavily on scavenging nutrients from the host, salvage rather than de novo biosynthesis, and glycolysis or substrate-level rather than oxidative phosphorylation for energy production (Ryan and Hijjawi, 2015). Consequently, the parasite must depend on these essential core metabolic pathways, which are absent in or highly divergent from those in humans and animals, making many of the enzymes in these pathways potential drug targets.
Perhaps the most extensively studied metabolic pathway for drug development is the salvage of purines for nucleic acid synthesis. Cryptosporidium cannot synthesize purine nucleotides de novo, but instead converts adenosine salvaged from the host into guanine nucleotides via a pathway involving inosine 5′-monophosphate dehydrogenase (IMPDH; Umejiego et al., 2004). This enzyme catalyzes the conversion of inosine-5′-monophosphate into xanthosine-5′-monophosphate as the rate-determining step in guanine nucleotide biosynthesis. Interestingly, the parasites appear to have obtained their IMPDH gene by lateral gene transfer from bacteria, making it structurally distinct from mammalian IMPDH enzymes (Striepen et al., 2004). The exclusive reliance on this guanine salvage pathway makes IMPDH a potential drug target. Indeed, generic inhibitors of the enzyme have significant anticryptosporidial activity in vitro (Striepen et al., 2004). Subsequent studies have systematically advanced the development of more specific inhibitors. Based on high throughout screens, a number of structurally diverse IMPDH inhibitors were identified (Umejiego et al., 2008), and several of the most promising scaffolds were then explored for optimal activity in vitro and in vivo. For example, a number of derivatives of urea, benzoxazole, and benzopyrano[4,3-c]pyrazole are active against purified IMPDH in the low to mid nM range (Gorla et al., 2012, 2013; Sun et al., 2014), and several have activity against C. parvum in cell culture and in a mouse infection model in vivo (Gorla et al., 2014). As an alternative strategy to conventional hit identification and lead optimization of small “drug-like” molecules, peptides have been explored as IMPDH inhibitors. In particular, phylomer peptides, which represent naturally stable protein segments from phylogenetically diverse bacterial genomes with an evolutionarily optimized ability to bind protein surfaces (Watt, 2009), have been screened for inhibitory activity against C. parvum IMPDH (Jefferies et al., 2015). Peptides that interact with IMPDH were identified in a yeast two hybrid screen, and then tested for parasite inhibition in vitro. The best peptides suppressed C. parvum growth with 50% efficiency at 8–46 μM, but had only negligible cytotoxicity in human cells (Jefferies et al., 2015). Although the in vivo efficacy of these peptides remains to be established, the data underline that IMPDH is a valid pharmacological target for parasite inhibition. Taken together, the recent studies suggest that further preclinical development of IMPDH inhibitors, using either current scaffolds or perhaps yet to be discovered ones, holds great promise for a new class of anticryptosporidial agents.
Several other molecular targets have been explored against Cryptosporidium. For example, the C. parvum genome encodes three long chain fatty acyl-coenzyme A synthetases, which are essential in the fatty acid metabolism of the parasite (Guo et al., 2014). Their inhibition with triacsin C was highly effective against parasite growth in vitro and reduced oocyst production by 88% in infected IL-12 knockout mice (Guo et al., 2014). Another target, calcium-dependent protein kinase 1 (CDPK1), plays a role in calcium-mediated signaling of the parasite, which is important for regulating vital functions in Cryptosporidium and other apicomplexan parasites (Murphy et al., 2010; Ojo et al., 2010). Inhibition of CDPK1 with pyrazolopyrimidine derivatives blocked enzyme activity in vitro in the low nM range and parasite growth in epithelial cells in the low μM range (Murphy et al., 2010). Moreover, at least one of the derivatives exhibited significant efficacy after oral dosing in a mouse infection model (Castellanos-Gonzalez et al., 2013b). A third group of molecular targets are cysteine proteases, which have been associated with excystation and host cell invasion of C. parvum (Nesterenko et al., 1995; Perez-Cordon et al., 2011). Selective protease inhibitors have shown promising activity against the parasite in vitro and in vivo (Kang et al., 2012; Ndao et al., 2013), although it remains to be established which out of the twenty or more members of the papain-like family of cysteine proteases are responsible for these therapeutic effects.
Beyond target-centered drug development, several activity-centered drug screening approaches have been undertaken for Cryptosporidium. For example, a library of 727 FDA-approved drugs or drug-like compounds with a history of use in human clinical trials was tested for activity on parasite growth in HCT-8 epithelial cells (Bessoff et al., 2013). Approximately twenty compounds, representing a hit rate of ∼3%, showed significant activity at <10 μM, with the best compounds displaying in vitro IC50s in the range of 0.2–5 μM (Bessoff et al., 2013). Mechanistic pursuit of one of these leads revealed that the drug target is 3-hydroxy-3-methyl-glutaryl-coenzyme A (HMG-CoA) reductase, which is required for the de novo synthesis of isoprenoids (Bessoff et al., 2013). Importantly, the parasite lacks all known enzymes required for the synthesis of isoprenoid precursors, so inhibition of the critical enzyme in the host blocks parasite growth because Cryptosporidium is dependent on the host cell for synthesis of isoprenoid precursors (Bessoff et al., 2013). This finding nicely supports the concept mentioned above that the obligate endosymbiosis of the parasite is a potential “Achilles’ heel” that may be exploited for drug development. It must be noted, however, that drugs that exclusively target host processes also have an increased risk for adverse effects, so it may be more difficult for such drugs compared to conventional microbe-targeted drugs to find an acceptable balance between parasite clearance and other drug effects.
In another screen, an existing library from the Medicines for Malaria Venture Open Access Malaria Box was tested for activity against C. parvum (Bessoff et al., 2014). The library represents a collection of 400 compounds selected from 19,000 structurally unique molecules with activity against the erythrocytic stage of Plasmodium falciparum in three large phenotypic high-throughput screening campaigns undertaken by large pharmaceutical companies. The screen yielded 19 compounds, representing a ∼5% hit rate, with significant activity against C. parvum growth in HCT-8 cells at 6.6 μM (Bessoff et al., 2014), suggesting that the relatively high hit rate of the Plasmodium-active compounds against Cryptosporidium may be attributed to the general similarities between the two apicomplexan parasites. The most active compounds belonged to three chemical series, derived from the quinolin-8-ol, allopurinol-based, and 2,4-diaminoquinazoline, and several of the derivatives exhibited submicromolar potency in vitro (Bessoff et al., 2014). Further studies will have to explore their in vivo efficacy.
Outlook
Taken together, recent studies clearly demonstrate that new classes of antimicrobials against Cryptosporidium are biologically possible and pharmacologically feasible, either by advancing leads against already identified targets or by screening new and larger compound libraries for activity against the parasite. In addition, analyses of the genomes and metabolomes of different Cryptosporidium species and genotypes are likely to reveal more molecular drug targets. However, as scientifically promising as drug development against Cryptosporidium is at this time, the major challenge for these efforts is the underlying economics (Striepen, 2013). As a disease that affects mostly developing countries and which had up to recently been significantly underestimated in its clinical prevalence and importance (Lal and Hales, 2015), cryptosporidiosis remains in the category of Neglected Diseases with low funding priority and limited commercial interest. If a new urgency in medical progress against the disease can be established at national funding agencies or philanthropic organizations, meaningful and timely progress could be made in treating and possibly preventing cryptosporidiosis.
Giardia
Giardiasis is a major cause of diarrheal illness worldwide with hundreds of millions annual cases. Compared to cryptosporidiosis, giardiasis is often associated with more prolonged, chronic diarrhea and disease even in immunocompetent individuals, which, particularly in children, can lead to long-term sequelae including intellectual deficits and failure to thrive. Furthermore, treatment options are currently broader than those for cryptosporidiosis, but resistance to existing antimicrobial drugs is becoming a clinical problem that has not yet been described for cryptosporidiosis.
Parasite
Giardiasis is caused by Giardia lamblia (syn. G. duodenalis or G. intestinalis), which was first discovered in the 1600s by van Leeuwenhoek in his own stool. G. lamblia is a flagellated, enteric parasite that belongs to the order of diplomonads in the phylum of metamonads, a large group of flagellate amitochondriate protozoa. The parasite has a comparatively simple life cycle (Figure 3), which occurs in a single host (monoxenous) and involves only two forms, the infectious cyst and the replicating trophozoite. Cysts are spread through contaminated drinking water or food, or person-to-person contact. G. lamblia is highly contagious, as evidenced by experimental human infection after oral ingestion of as few as 10 cysts (Nash et al., 1987). The low infectious dose, combined with the resistance of cysts to many common disinfectants (Betancourt and Rose, 2004; Erickson and Ortega, 2006), makes G. lamblia a constant threat to the safety of public water supplies. After passing through the stomach, trophozoites emerge from the cysts and colonize the upper small intestine. The sequence of exposure to an acidic environment, followed by neutral conditions and the presence of bile is sufficient to trigger excystation, as has been shown in vitro (Boucher and Gillin, 1990).
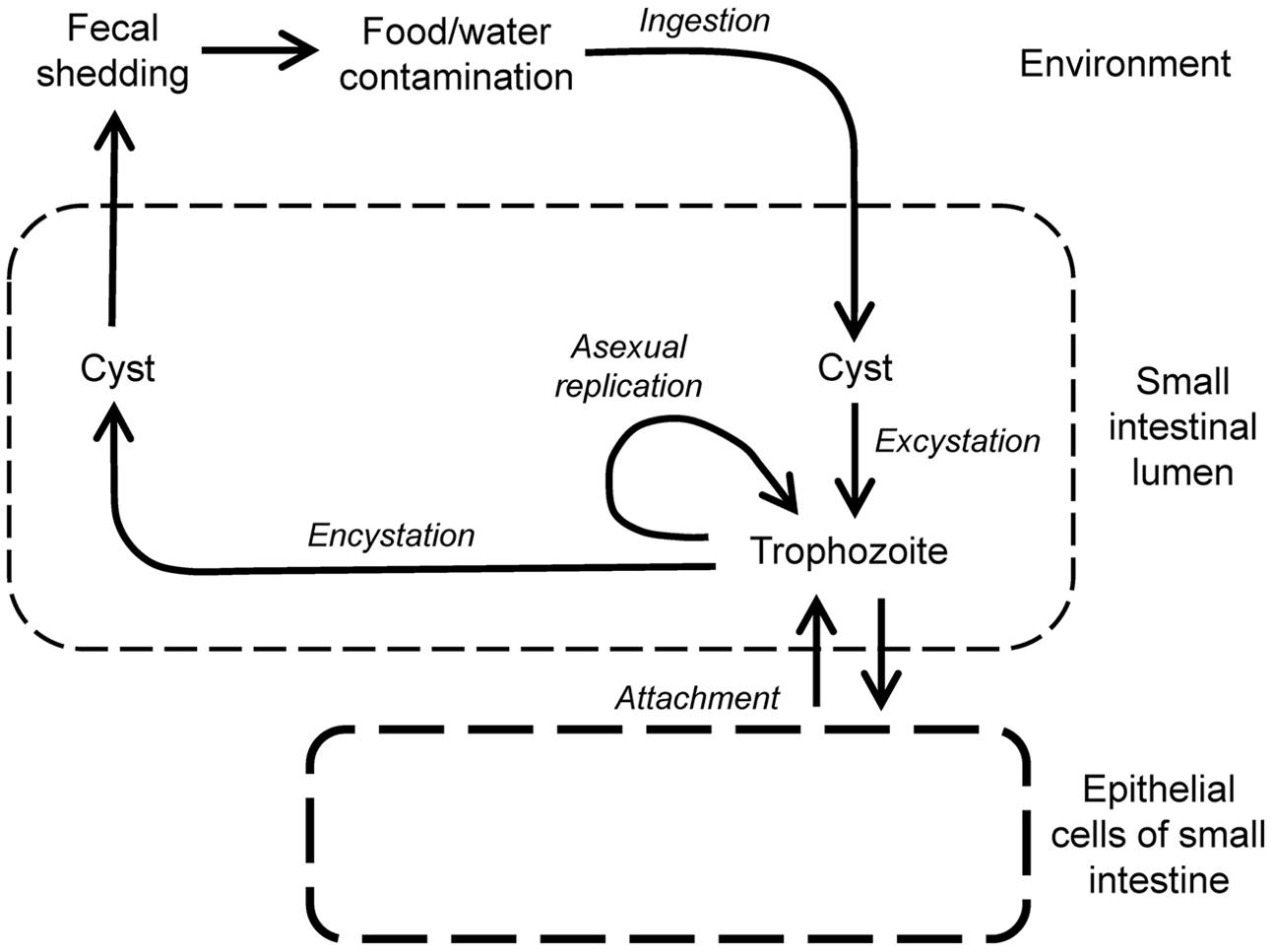
FIGURE 3. Life cycle of Giardia lamblia. Infection begins by oral uptake of cysts, which excyst in stomach and small intestine to form trophozoites, the replicating, disease-causing form of the parasite. Trophozoites are motile, and maintain their presence in the small intestine by regulated attachment to and detachment from the small intestinal epithelium, but they do not invade epithelial cells. Trophozoites can also differentiate by encystation in the small intestine into cysts, which are shed in the feces and are infectious for new hosts.
The haploid genome of G. lamblia comprises ∼12 Mb of DNA encoding ∼5,000 genes (Morrison et al., 2007; Franzen et al., 2009). The parasite is polyploid throughout its life cycle. Trophozoites have two nuclei, each carrying a diploid genome, so the overall genome is tetraploid (4N) in the trophozoite stage (Bernander et al., 2001). During encystation, the genome content acquires 16N ploidy through two successive rounds of chromosome replication without intervening cell division. Upon excystation, the cells have four nuclei, each with a ploidy of 4N, and divide twice to form four trophozoites containing two diploid nuclei each (Bernander et al., 2001). Besides the polyploidy, evidence exists for allelic sequence heterozygosity within single parasites (Ankarklev et al., 2012), further complicating the interpretation of genome information, as well as the use of genetic tools for identification of potential new drug targets. Moreover, G. lamblia displays considerable genetic diversity. Isolates are grouped into genetic Assemblages, A–F, with all human pathogens belonging to groups A and B, while the others are pathogens in dogs, birds, and other species. Genome comparison of isolates from Assemblage A and B shows only 77% nucleotide and 78% amino acid identity in protein-coding regions (Franzen et al., 2009), indicating that these two assemblages truly represent different Giardia species, despite the historic convention of a single species name (Jerlstrom-Hultqvist et al., 2010). Importantly, Assemblage A and B strains infect humans throughout the world, making it critical that new drugs are active against divergent G. lamblia strains of both Assemblages.
Pathogenesis and Disease
Giardia lamblia causes hundreds of millions of annual cases of diarrheal disease in endemic and epidemic fashion worldwide (Karanis et al., 2007; Baldursson and Karanis, 2011). In the United States, the parasite is one of the two most common causes of outbreaks of parasitic disease, with prevalence rates of 1–7% depending on the population sampled (Furness et al., 2000; Barry et al., 2013). Infections are more frequent and severe in young children, particularly in day-care centers, and among travelers, hikers, and military personnel in the field (Overturf, 1994; Sharp et al., 1995). Following cyst ingestion, symptoms occur after a 1–2 weeks incubation period, although only about half of all stool-positive Giardia infections are symptomatic. Frequent clinical manifestations of giardiasis include watery diarrhea, epigastric pain, nausea, and vomiting. The acute phase typically lasts 1–3 weeks, although some patients can have persistent symptoms for months. Most infections are self-limited but recurrences are common in endemic areas. Chronic infections can lead to weight loss and malabsorption and may be mistaken for inflammatory bowel disease or anorexia nervosa (Thomas Iv et al., 2014). Importantly, chronic giardiasis is associated with stunting (low height for age), wasting (low weight for height) and cognitive impairment in children in developing countries (Berkman et al., 2002; Nematian et al., 2008; Al-Mekhlafi et al., 2013), a finding that has been reproduced in murine models of infection (Bartelt et al., 2013). Furthermore, acute giardiasis may disable patients for extended periods and can elicit protracted post-infectious syndromes, including irritable bowel syndrome and chronic fatigue (Hanevik et al., 2014).
After emergence from cysts, the flagellated G. lamblia trophozoites colonize the upper small intestine, although colonization of the lower small intestine, as well as stomach and colon can occur. Trophozoites reside and replicate in the intestinal lumen and at the intestinal epithelial surface, but do not invade deeper layers of the mucosa. The parasite’s ability to attach to the mucosal surface is critical for its persistence in the host (Lauwaet et al., 2010), yet the underlying mechanisms remain poorly defined. The ventral disk of the parasite can assume a domed conformation that is important for robust attachment (Woessner and Dawson, 2012), whereas flagellar motility is important for positioning and orienting trophozoites prior to attachment, but is not directly required for attachment (House et al., 2011). Despite its “off-shore” location outside the mucosa, the parasite actively engages mucosal immunity, although frank inflammation is typically absent in most cases (Oberhuber et al., 1997). However, villus and brush border microvillus atrophy are common, leading to digestive enzyme deficiencies (Solaymani-Mohammadi and Singer, 2011), and chronic giardiasis can lead to overt mucosal inflammation with pronounced villus loss (Hanevik et al., 2007).
Giardiasis is self-limiting in >85% of cases in non-endemic areas, indicating that effective immune defenses exist. Furthermore, symptoms of giardiasis are much less severe in endemic than non-endemic regions, suggesting gradual build-up of immunity (Faubert, 2000). Secretory IgA (Langford et al., 2002; Davids et al., 2006), intestinal hypermotility (Andersen et al., 2006; Li et al., 2006), and antimicrobial peptides and nitric oxide (Eckmann et al., 2000; Tako et al., 2013) have all been shown to have direct effector activity against the parasite, although their relative contributions to clearance of Giardia may be variable (Singer and Nash, 2000a; Langford et al., 2002). Beyond direct effectors, several immune cells and regulators are known to be involved in antigiardial immune defense. Mast cells and CD4+ T cells, but not CD8+ T cells, are required for clearing Giardia infection (Singer and Nash, 2000a; Roxstrom-Lindquist et al., 2006; Solaymani-Mohammadi and Singer, 2010). CD4+ T cells may act in part by controlling antigiardial IgA responses (Heyworth, 1989), while their functions are not related to classical Th1 or Th2 subsets, since their signature cytokines, IFN-γ, or IL-4, play no role in immune defense (Singer and Nash, 2000a). In contrast, IL-6 and IL-17 are important in Giardia clearance (Bienz et al., 2003; Zhou et al., 2003; Dreesen et al., 2014; Dann et al., 2015). IL-6 appears to act by promoting dendritic cell functions during infection (Kamda et al., 2012), although it has many other activities, including activation of neutrophils and monocytes, enhancement of follicular helper T cell responses, and stimulation of B cell proliferation and antibody production.
A human vaccine against giardiasis is not available. A crude veterinary vaccine (GiardiaVax), composed of total cell lysates of a mixture of sheep, dog and human isolates, reduces symptoms and duration of cyst output in cats and dogs (Olson et al., 2000). Interestingly, the vaccine has also been used as an immunotherapeutic agent in dogs with chronic giardiasis that had failed standard drug treatment (Olson et al., 2001), raising the intriguing possibility that a vaccine may be effective post-exposure.
Current Treatment Options
Several classes of antimicrobial drugs are available for the treatment of giardiasis. The most commonly utilized worldwide are members of the 5-nitroimidazole (5-NI) family such as metronidazole and tinidazole. However, this first line therapy fails in up to 20% of cases and cross-resistance between different agents can occur (Gardner and Hill, 2001), and resistance to all major antigiardial drugs has been reported (Ansell et al., 2015). Alternative agents exist for treatment failures and for special circumstances (e.g., pregnancy), but these are generally less effective than 5-NI drugs (Escobedo and Cimerman, 2007).
The oldest and most common 5-NI, metronidazole, is typically given in three divided daily 250 mg oral doses for 5–10 days, and has a reported efficacy of 80–95% (Gardner and Hill, 2001). More recently, tinidazole, first approved by the FDA in 2004, has become a good alternative for giardiasis because of its efficacy (85–90%), tolerability, and convenience (a single oral dose is recommended; Speelman, 1985). All 5-NI drugs are prodrugs whose microbial specificity is due to the requirement for reduction to toxic free radical intermediates by low redox potential reactions present only in the anaerobic target microbes (Upcroft and Upcroft, 2001). The metabolism of G. lamblia is fermentative, and electron transport proceeds in the absence of mitochondrial oxidative phosphorylation. However, the parasite is microaerotolerant and can reduce molecular oxygen and thus protect the highly oxygen-sensitive, central metabolic enzyme, pyruvate:ferredoxin oxidoreductase (PFOR), and iron-containing ferredoxins. PFOR decarboxylates pyruvate and donates electrons to ferredoxin, which in turn reduces other components in the electron transport chain and leads to ATP generation. Reduced ferredoxin can also reduce the critical nitro group of 5-NI prodrugs to toxic radicals which kill the parasite. Other reduction pathways, including nitroreductases and thioredoxin reductase, also activate 5-NI drugs in Giardia (Pal et al., 2009; Leitsch et al., 2011; Nillius et al., 2011), although their relative importance in reducing metronidazole and other nitro drugs remains to be established. The radicals that result from nitro drug reduction form covalently bonded adducts on microbial target molecules, leading to their inactivation. The specific molecular targets of 5-NI drugs have not been defined in G. lamblia. In spite of the general efficacy of 5-NI drugs, treatment failures in giardiasis are common (up to 20%), clinical resistance is proven, and in vitro resistance can be induced so that parasites grow in clinically relevant levels of metronidazole (Wright et al., 2003; Tejman-Yarden et al., 2011).
Nitazoxanide is a nitrothiazole with broad-spectrum activity against intestinal parasites. Like 5-NI drugs, it is a prodrug that must be reduced to form toxic radicals, which inactivate various microbial target molecules including PFOR (Hoffman et al., 2007). It is usually given in two daily 500 mg doses for 3 days, which is more convenient dosing than metronidazole, but has slightly lower efficacy (70–80%) than 5-NI drugs (Gardner and Hill, 2001) and is also impacted by metronidazole resistance (Tejman-Yarden et al., 2011). In a clinical trial involving children with diarrheal illness, nitazoxanide reduced symptom duration in those afflicted with giardiasis as well as in those without a microbiological diagnosis (Rossignol et al., 2012), further underlining its utility as a broad-spectrum antimicrobial agent.
Benzimidazoles, such as albendazole and mebendazole, are generally used to treat intestinal helminth infections. Albendazole is also effective in giardiasis (Gardner and Hill, 2001; Solaymani-Mohammadi et al., 2010), although its efficacy varies markedly (25–90%) depending on the dosing regimen. Albendazole can be taken once daily for 5 days, making it more convenient than three-times-a-day metronidazole, and its anti-helminth activity makes it an attractive agent for dual use purposes (Granados et al., 2012). However, results from a Bolivian cohort study (a region with endemic Giardia and helminth infections) found that treatment with mebendazole reduced hookworm infections but paradoxically led to an increase in giardiasis (Blackwell et al., 2013), suggesting an antagonistic relationship between the two parasites and complicating the prospect of multi-target therapies.
Quinacrine, an old malaria drug, is an acridine derivative with excellent efficacy against giardiasis (90%). A recent report describes the experience of a family of four with giardiasis, three of whom failed therapy with tinidazole but were cured with quinacrine (Requena-Mendez et al., 2014). In a randomized trial in children with giardiasis, chloroquine was equally effective as tinidazole and superior to albendazole (Escobedo et al., 2003). Despite its good efficacy, quinacrine has potentially severe adverse effects, including a number of psychiatric and dermatologic manifestations, and is no longer commercially available in the United States or Canada.
Finally, paromomycin is an aminoglycoside with antigiardial activity in vitro (Edlind, 1989) and in vivo (Geurden et al., 2006). However, it less effective among adults with metronidazole-refractory disease than other therapies and is rarely used in clinical practice.
Therapeutic strategies for treatment-refractory giardiasis include longer duration and/or higher doses of the original agent, switching to a different class of drug, or combination therapy. In a case series of 10 patients who failed nitroimidazole therapy, all were cured with one of the following combinations: metronidazole or tinidazole + paromomycin + albendazole in three cases, metronidazole + paromomycin in two cases, tinidazole + paromomycin in two cases, tinidazole + quinacrine in two cases, and metronidazole + quinacrine in one case (Morch et al., 2008). All the drugs were administered for 7 or 10 days except for tinidazole, which was given for 1–7 days. The combinations were well tolerated and had no serious adverse effects. In a case report, a HIV patient with giardiasis was unsuccessfully treated five times with metronidazole and albendazole, but was cured with nitazoxanide 500 mg b.i.d for 10 days and then 1 g b.i.d. for 15 days (Abboud et al., 2001). Susceptibility testing showed the strain to be resistant to metronidazole and albendazole, but susceptible to nitazoxanide (Abboud et al., 2001). Combination therapy generally decreases the risk of developing antimicrobial drug resistance, although it is presently not known how this concept applies to giardiasis.
Assays for Drug Development
Giardia lamblia trophozoites can be readily grown in vitro under axenic conditions, which greatly simplifies drug testing compared to the endosymbiont Cryptosporidium. Typical assays for Giardia involve serial drug dilutions in multi-well plates and parasite growth for 48 h under anaerobic conditions (Valdez et al., 2009; Miyamoto et al., 2013) (Figure 4). Cell numbers and viability can be assessed microscopically (Gut et al., 2011), or by determining ATP content in the cultures (Valdez et al., 2009) (Figure 2). A number of Assemblage A and B isolates are available, and resistant lines against all major antigiardial drugs have been generated in the laboratory (Upcroft et al., 1996a,b; Dunn et al., 2010; Tejman-Yarden et al., 2011). To date, drug-resistant axenic lines have not been generated from clinical isolates derived from patients who failed therapy, although short-term animal studies of such isolates have shown that resistance occurs in vivo (Lemee et al., 2000).
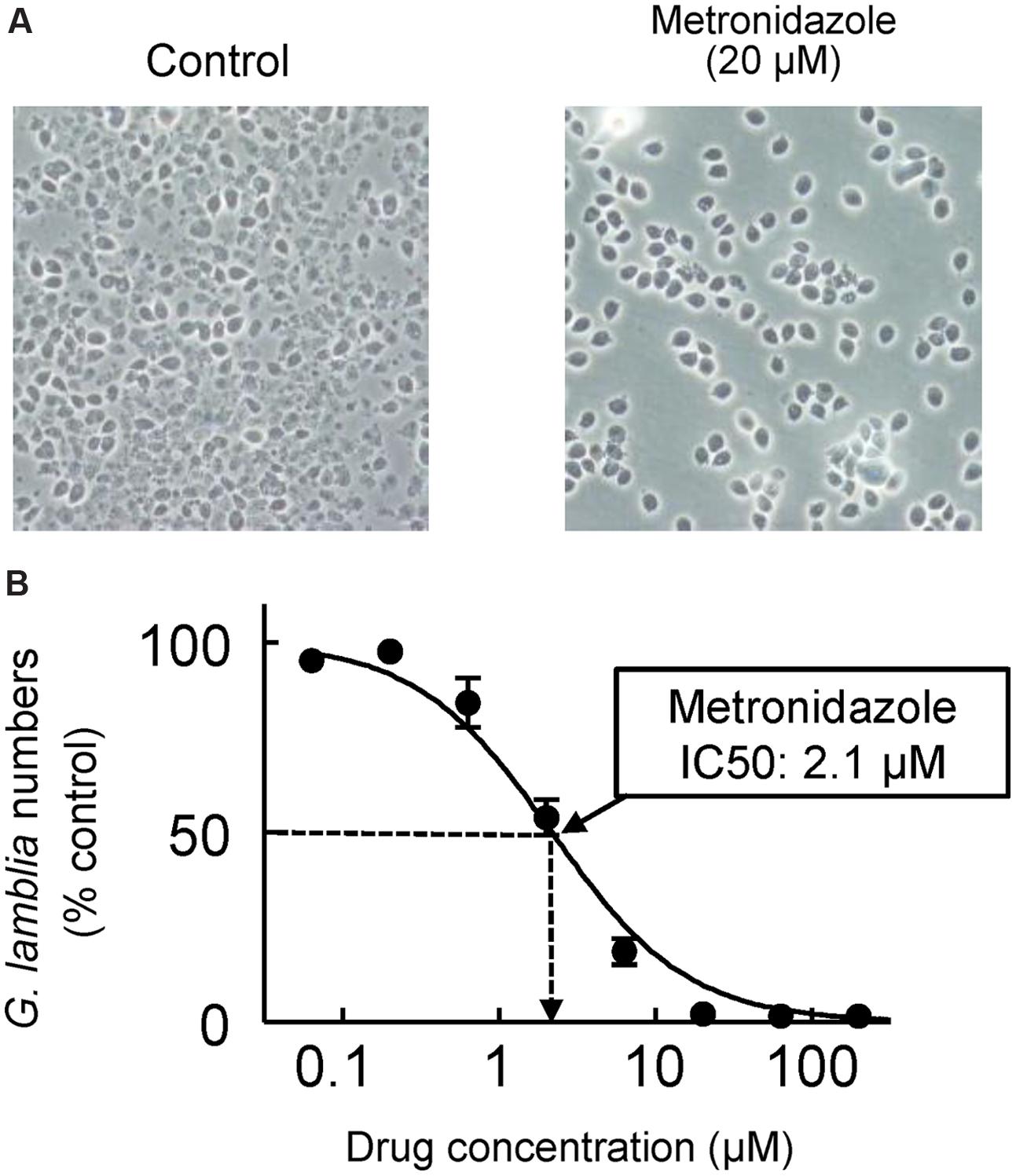
FIGURE 4. In vitro drug assay for G. lamblia. Serial dilutions of test compounds were made in 96-well plates, and trophozoites of G. lamblia WB were added to the wells (2 × 103/well). After 48 h incubation at 37°C under anaerobic conditions, cell growth and viability was determined with an ATP assay. (A) Representative phase-contrast images for controls without added drug (left) and cells exposed to 20 μM metronidazole (right). (B) A concentration-response curve for metronidazole. Data are mean ± SD of triplicate wells, and are expressed relative to the G. lamblia numbers in untreated controls. The IC50 for metronidazole was 2.1 μM in this experiment.
Appropriate animal infection models are a key requirement for antimicrobial drug development. A number of mammals, including different rodents, dogs and cats, can be experimentally infected with G. lamblia. In cats and dogs, giardiasis has veterinary relevance (Bouzid et al., 2015), so drug development has veterinary objectives in these species. For drug testing studies against human G. lamblia, the most common models are mice and Mongolian gerbils. Adult mice are not readily infectable with most human G. lamblia strains, with the sole exception of the Assemblage B strain, GS/M (Byrd et al., 1994). The strain was shown to cause diarrheal disease upon experimental inoculation in humans (Nash et al., 1987), so it is a clinically relevant, pathogenic strain, yet the reasons for its seemingly unique ability to colonize adult mice remain unclear. However, following the observation that resistance to infection could be conferred by co-housing of animals, it was shown that conditioning of mice with a cocktail of suitable antibiotics (typically vancomycin + gentamicin or neomycin + ampicillin or penicillin; none of these kill G. lamblia) in the drinking water sensitizes mice to infection with a range of different G. lamblia strains of both A and B assemblages (Singer and Nash, 2000b; Solaymani-Mohammadi and Singer, 2011). This conditioning regimen is now commonly used, although the impact on drug testing has not been explored. As an alternative, gerbils can be infected with a wide range of human G. lamblia strains without antibiotic conditioning (Tejman-Yarden et al., 2011, 2013; Benere et al., 2012). They have proven valuable for pathophysiologic and immunological studies, but have not been widely used for drug testing.
New Drugs
The discovery of antibiotics revolutionized medicine and represents a turning point in human history. Unfortunately, enthusiasm for these miracle drugs was soon tempered by the emergence of resistant strains. As in bacteria, protozoa like Giardia can develop resistance that make widely used agents ineffective. While still uncommon, antibiotic resistance (especially against 5-NI drugs) is increasing in G. lamblia and contributes to treatment failures (Lemee et al., 2000). Elucidating the complex resistance mechanisms is an active area of investigation and important insights have been gained over the last decade. Recent studies indicate that drug resistance in G. lamblia is caused by one of several different cellular adaptations. Of these, the best described mechanism is the loss of the parasite’s ability to activate nitro prodrugs to toxic radicals by reduction, thus effectively allowing the parasite to avoid suicidal drug activation. For example, suppression of PFOR leads to metronidazole resistance (Dan et al., 2000) and certain strains of metronidazole-resistant G. lamblia have reduced levels of this critical enzyme required for ferredoxin-dependent drug activation (Leitsch et al., 2011). Other drug activation pathways have been identified, and they can also be impacted in resistant cells (Pal et al., 2009; Leitsch et al., 2011; Nillius et al., 2011). Another mechanism of drug resistance to both metronidazole and nitazoxanide is altered expression of genes involved in stress responses, including heat-shock proteins and expression of nitazoxanide-binding proteins (Muller et al., 2008). Fortunately, metronidazole-resistant giardiasis is not yet common in clinical practice, perhaps because of the detrimental effects of metronidazole resistance on attachment and infectivity in a subset of organisms, although others with resistance can infect normally (Tejman-Yarden et al., 2011). Resistance to other antigiardial drugs also exists. For example, resistance to benzimidazoles is thought to occur through mutations in the molecular target, β-tubulin (Aguayo-Ortiz et al., 2013). Overall, the studies on emerging drug resistance and the underlying mechanisms underline that the development of new antigiardial agents to broaden the therapeutic armamentarium remains an important challenge.
The goals of new drug development against G. lamblia and Cryptosporidium are somewhat different. For Cryptosporidium, only one moderately effective drug (nitazoxanide) is available, so new classes of more effective drugs are a high priority. By comparison, for G. lamblia, several classes of drugs with good efficacy exist, but dosing regimens are suboptimal or emerging resistance begins to threaten clinical utility. Consequently, improvements in potency and dosing, and the ability to overcome existing and prevent new forms of drug resistance are priorities in antigiardial drug development. A number of strategies have been employed for the development of new drugs against G. lamblia, which include, as for Cryptosporidium, both activity- and target-centered approaches. In addition, optimization within existing drug classes, analogous to the development of next-generation β-lactam antibiotics, has played a much more important role in drug development against G. lamblia compared to Cryptosporidium.
Prime examples for the potential of optimization within an existing drug class are the 5-NI compounds. The most common 5-NI, metronidazole, is composed of a 5-membered nitroimidazole core carrying simple hydroxyethyl and methyl side chains in the 1′ and 2′ position, respectively (Figure 5). Modifications of these side chains have been a productive strategy for developing new and improved agents. For example, substitution of side chains in the 1′, 2′, or 4′ positions of the imidazole ring with larger and more complex functional groups has led to compounds with enhanced (up to 500-fold) antigiardial activity (Upcroft et al., 2006; Valdez et al., 2009; Miyamoto et al., 2013) (Figure 5). Importantly, many of the new 5-NI compounds were able to overcome existing forms of metronidazole resistance (Miyamoto et al., 2013). The underlying mechanisms for improved activity remain to be determined but may relate to differential drug activation by one or more reductases (Leitsch et al., 2011; Nillius et al., 2011). For other metronidazole derivatives it was shown that they were more active than the parent compound in altering the morphology and ultrastructure of G. lamblia by affecting vesicle trafficking and differentiation into cysts (Busatti et al., 2013). Similar advances have been demonstrated for the 5-nitrothiazole, nitazoxanide, where placement of a benzene ring between the nitro group and the thiazole ring was used to create new benzologues (Navarrete-Vazquez et al., 2011). The best compounds were five-times more active than nitazoxanide and 18-times more active than metronidazole against G. lamblia and showed minimal toxicity in mammalian cells (Navarrete-Vazquez et al., 2011). In another series of nitrothiazole derivatives, substitutions at 2′ position with a range of acylamino side chains yielded compounds with >10-fold greater activity against G. lamblia than the reference compound nitazoxanide (Nava-Zuazo et al., 2014). Collectively, these studies demonstrate that systematic structural modifications of existing nitro drugs are likely to yield vastly improved antimicrobials that can serve as “next-generation” nitro drugs for the treatment of giardiasis and potentially other infections with clinically important anaerobic pathogens (Miyamoto et al., 2013).
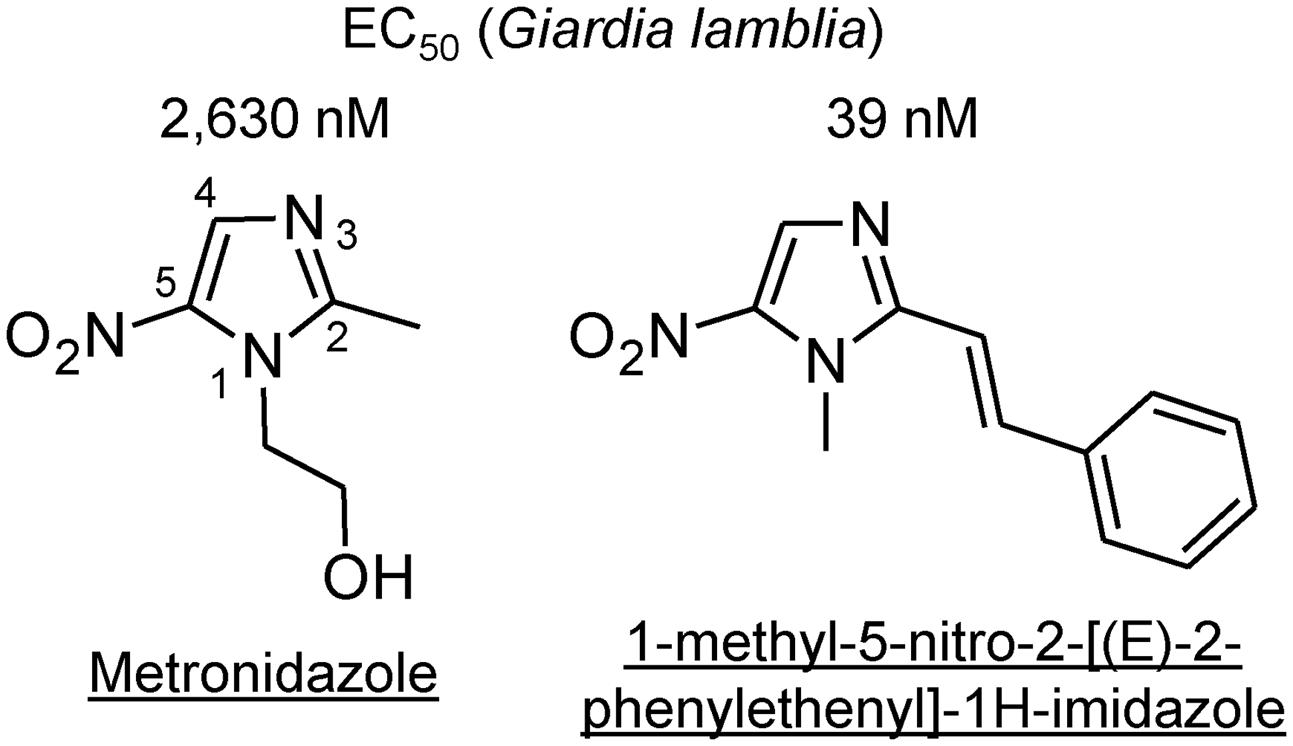
FIGURE 5. Structures of 5-nitro antimicrobial drugs. The structures of metronidazole (left) and a 2-position derivative are depicted, along with their respective in vitro EC50 values against a representative strain of G. lamblia (Valdez et al., 2009). The numbers along the core imidazole of metronidazole designate the ring positions.
Similar modification strategies have been applied to benzimidazoles, whose prototype compound, albendazole, has been used as an alternative to 5-NI drugs against giardiasis. A number of novel derivatives have been created, including an alkylthiol group at the 2′ position and an ethoxy group at the 5′ position of the benzimidazole ring, resulting in highly potent compounds with antigiardial activity in the 10–50 nM range (Perez-Villanueva et al., 2013). Using reverse-phase high performance liquid chromatography and permeability assays with cultured epithelial cells, it was observed that the antigiardial activity of a series of benzimidazole derivatives was influenced by their lipophilicity, hydrogen bond donors, and molecular volume, but not by their apparent permeability across epithelial cell monolayers (Hernandez-Covarrubias et al., 2012). A computational analysis of the structure-activity relationships of multiple benzimidazoles showed complex activity landscapes for antigiardial activity, with substitution at position 2 on the benzimidazole moiety playing an important role in increasing potency and substitutions at positions 4–7 influencing selectivity against G. lamblia over other protozoan parasites (Perez-Villanueva et al., 2010). These findings offer hope that quantitative models may help to identify structural properties associated with enhanced antigiardial activity in future drug design, at least for compounds that have well-defined molecular targets such as β-tubulin for benzimidazoles (Aguayo-Ortiz et al., 2013). Similar structure activity analyses have been conducted for 5-NI derivatives (Miyamoto et al., 2013), although the current lack of understanding of the specific reductases involved in prodrug activation or the molecular targets of activated drugs confounds these analyses and complicates their exploitation in drug development.
Besides compound optimization within existing drug classes, activity-centered screens of drug libraries have also been applied to G. lamblia. For example, a screen of 1,520 compounds from the BIOMOL ICCB Known Bioactives 2 and NINDS Custom Collection 2 libraries for growth-inhibitory activity against G. lamblia yielded 48 initial hits, some of which, such as indirubin, were not known to kill G. lamblia, although many others had known antigiardial agents or were broad inhibitors of cellular functions (e.g., actinomycin D; Bonilla-Santiago et al., 2008). Such toxic compounds are not likely to be of clinical utility. By comparison, screening of libraries of FDA-approved drugs has the distinct advantage that preclinical safety testing has already been done, which can accelerate the progression to clinical trials of efficacy. For instance, screening of a library of 746 compounds available for human use found that auranofin, which is employed in the treatment of rheumatoid arthritis, inhibited the growth and survival of multiple different G. lamblia isolates (Tejman-Yarden et al., 2013). Importantly, auranofin completely overcame metronidazole resistance (Tejman-Yarden et al., 2013). The action mechanism involved inhibition of thioredoxin oxidoreductase, a critical enzyme involved in maintaining normal protein function and combating oxidative damage in G. lamblia and other parasites including Entamoeba histolytica and Schistosoma mansoni (Kuntz et al., 2007; Debnath et al., 2012; Tejman-Yarden et al., 2013). Given that its pharmacokinetic properties and toxicology are well established (Blocka et al., 1986), auranofin may be a promising new candidate for the treatment of drug-resistant giardiasis. In another screen, a total of 2,816 compounds from the Library of Pharmaceutical Active Compounds (LOPAC1280) and the NIH Chemical Genomics Center Pharmaceutical collection library (NPC) were tested for activity against G. lamblia (Chen et al., 2011). Detailed follow-up studies of 28 of the most active compounds identified three new drugs, fumagillin, carbadox, and tioxidazole, with strong activity against metronidazole-sensitive and -resistant G. lamblia isolates (Kulakova et al., 2014). Furthermore, fumagillin, a complex biomolecule derived from Aspergillus fumigatus with known antimicrobial activities against Entamoeba and microsporidia, was efficacious in a mouse giardiasis model (Kulakova et al., 2014).
An abbreviated form of activity-centered drug discovery is the retesting of compounds that have shown activity in related microbes. Screening of an NIH Chemical Genomics Center Pharmaceutical Collection containing >2,000 compounds identified 32 highly active compounds against Plasmodium falciparum (Yuan et al., 2011). One of these, tetrahydrolipstatin (orlistat), a lipase inhibitor approved for the treatment of obesity, was then tested for growth inhibition of G. lamblia, based on the general rationale that efficient lipid metabolism is important for a fast growing parasite such as Giardia. Orlistat showed a potent and dose-dependent inhibition of G. lamblia replication, with a half maximal inhibitory concentration significantly lower than that of metronidazole (Hahn et al., 2013). Notably, orlistat is poorly absorbed from the intestinal tract and remains highly active in the lumen until excreted in the stool, raising the possibility of essentially topical therapy of giardiasis. Disadvantages of orlistat are the potential malabsorption of lipids and other lipophilic drugs, as well as cost issues. Another example is miltefosine, a potent oral treatment for human visceral leishmaniasis due to Leishmania donovani. The drug had previously been shown to kill this parasite by inducing a cell death process with numerous cytoplasmic, nuclear, and membrane features of metazoan apoptosis (Paris et al., 2004). Subsequent testing of miltefosine against G. lamblia revealed good activity in vitro and efficacy in a mouse model in vivo (Eissa and Amer, 2012). As with orlistat, trials to ascertain the effectiveness of miltefosine in humans with giardiasis will be needed. More broadly, repurposing of existing human drugs can be a powerful and resource-efficient strategy for rapid progress in drug development, but the finite number of existing compounds (with only ∼1,500 currently FDA-approved drugs) ultimately limits this discovery path in the ongoing struggle for new antimicrobial agents.
One way out of the closed loop of drug repurposing is the screening of unknown chemical entities. Plants and their extracts have historically been rich sources for new antimicrobial agents. One of these is the naturally occurring weed Oxalis corniculata, long used in India for treating dysentery and diarrhea. Systematic fractionation of extracts was used to isolate a novel galacto-glycerolipid compound with antigiardial activity comparable to metronidazole (Manna et al., 2010). The compound had no toxicity in human cells (Manna et al., 2010). Extracts of sandalwood, Osyris alba, have been used by traditional healers in Jordan to ameliorate diarrheal diseases. Phytochemical investigation identified a new pyrrolizidine alkaloid, osyrisine, which had significant activity against Giardia but was non-toxic to human cells (Al-Jaber et al., 2010). These examples demonstrate that crude plant extracts, or perhaps in the future other rich biological sources of structurally diverse compounds, can be the source of new antigiardial compounds, although their medicinal characterization, synthesis, and optimization are likely to pose challenges not encountered with the exploration of libraries of chemically defined compounds with drug-like properties.
The third drug development strategy, exploitation of molecularly defined targets, has been less prominent for Giardia compared to Cryptosporidium. Nonetheless, a small number of G. lamblia targets have been explored for druggability. The metabolic enzyme arginine deiminase (ADI) catalyzes the first step in a major pathway for generating ATP in the parasite (Knodler et al., 1998). Knock-down of ADI by RNA interference resulted in non-viable trophozoites, indicating that ADI is important for trophozoite survival (Li et al., 2009). Furthermore, ADI may also serve as a virulence factor by depleting arginine in the host, which may enable Giardia to evade the host immune response (Eckmann et al., 2000; Banik et al., 2013). Humans lack an analogous enzyme to ADI, making it an attractive target for drug development. Another enzyme target is carbamate kinase, which catalyzes the final step in the arginine dihydrolase pathway converting ADP and carbamoyl phosphate to ATP and carbamate. It is essential in Giardia metabolism, but has no equivalent in humans (Minotto et al., 1999). A luminescent enzyme activity assay was employed in a high throughput screen of over 4,000 compounds to identify 30 hits with activity in the low mM range (Chen et al., 2012). Although the detected activities are low at this point, the hits could be starting points for the development of more potent and selective inhibitors of carbamate kinase in G. lamblia and ultimately a novel class of antigiardial drugs. A third attractive target enzyme in Giardia is fructose 1,6-bisphosphate aldolase (FBPA), which catalyzes the reversible cleavage of D-fructose-1,6-bisphosphate to dihydroxyacetone phosphate and glyceraldehyde 3-phosphate, a key step in the Embden–Meyerhof–Parnas glycolytic pathway used by amitochondriate protozoa as a main source of energy (Li et al., 2011). FBPA is critical for Giardia survival and had unique structural and functional features that distinguish it from human analogs (Galkin et al., 2007). Inhibitors of the enzyme have antigiardial activity in the 100 nM range and very low mammalian cytotoxicity (Navarrete-Vazquez et al., 2015).
Outlook
Several treatment options currently exist for giardiasis, but important therapeutic challenges remain. One major objective is improvements in dosing, ideally in form of a single, highly effective oral dose, which is the ultimate goal for most if not all tropical infection diseases as it allows diagnosis and definitive treatment on a single clinic visit. Another objective is the ability to overcome and prevent drug resistance, which is not yet common for G. lamblia, but constitutes a continuous threat that should not be taken lightly. Current studies suggest that these objectives can be achieved within existing classes of drugs or with new classes of drugs and molecular targets. However, as for cryptosporidiosis, a major challenge are the economic incentives for new drug development against giardiasis, which is clinically important throughout the world but is typically considered a Neglected Disease with primary health impact in low-development countries (Escobedo et al., 2010).
Funding
This work was supported by NIH grant AI114671.
Conflict of Interest Statement
The authors declare that the research was conducted in the absence of any commercial or financial relationships that could be construed as a potential conflict of interest.
References
Abboud, P., Lemee, V., Gargala, G., Brasseur, P., Ballet, J. J., Borsa-Lebas, F., et al. (2001). Successful treatment of metronidazole- and albendazole-resistant giardiasis with nitazoxanide in a patient with acquired immunodeficiency syndrome. Clin. Infect. Dis. 32, 1792–1794. doi: 10.1086/320751
Abrahamsen, M. S., Templeton, T. J., Enomoto, S., Abrahante, J. E., Zhu, G., Lancto, C. A., et al. (2004). Complete genome sequence of the apicomplexan, Cryptosporidium parvum. Science 304, 441–445. doi: 10.1126/science.1094786
Abubakar, I., Aliyu, S. H., Arumugam, C., Hunter, P. R., and Usman, N. K. (2007). Prevention and treatment of cryptosporidiosis in immunocompromised patients. Cochrane Database Syst. Rev. 24:CD004932. doi: 10.1002/14651858.CD004932.pub2
Adell, A. D., Miller, W. A., Harvey, D. J., Vanwormer, E., Wuertz, S., and Conrad, P. A. (2013). Individual subject meta-analysis of parameters for Cryptosporidium parvum shedding and diarrhoea in animal experimental models. Epidemiol. Infect. 141, 1662–1678. doi: 10.1017/S0950268812002294
Aguayo-Ortiz, R., Mendez-Lucio, O., Romo-Mancillas, A., Castillo, R., Yepez-Mulia, L., Medina-Franco, J. L., et al. (2013). Molecular basis for benzimidazole resistance from a novel beta-tubulin binding site model. J. Mol. Graph. Model. 45, 26–37. doi: 10.1016/j.jmgm.2013.07.008
Aguirre, S. A., Mason, P. H., and Perryman, L. E. (1994). Susceptibility of major histocompatibility complex (MHC) class I- and MHC class II-deficient mice to Cryptosporidium parvum infection. Infect. Immun. 62, 697–699.
Alcantara Warren, C., Destura, R. V., Sevilleja, J. E., Barroso, L. F., Carvalho, H., Barrett, L. J., et al. (2008). Detection of epithelial-cell injury, and quantification of infection, in the HCT-8 organoid model of cryptosporidiosis. J. Infect. Dis. 198, 143–149. doi: 10.1086/588819
Al-Jaber, H. I., Mosleh, I. M., Mallouh, A., Abu Salim, O. M., and Abu Zarga, M. H. (2010). Chemical constituents of Osyris alba and their antiparasitic activities. J. Asian. Nat. Prod. Res. 12, 814–820. doi: 10.1080/10286020.2010.502892
Al-Mekhlafi, H. M., Al-Maktari, M. T., Jani, R., Ahmed, A., Anuar, T. S., Moktar, N., et al. (2013). Burden of Giardia duodenalis infection and its adverse effects on growth of schoolchildren in rural Malaysia. PLoS Negl. Trop. Dis. 7:e2516. doi: 10.1371/journal.pntd.0002516
Amadi, B., Mwiya, M., Musuku, J., Watuka, A., Sianongo, S., Ayoub, A., et al. (2002). Effect of nitazoxanide on morbidity and mortality in Zambian children with cryptosporidiosis: a randomised controlled trial. Lancet 360, 1375–1380. doi: 10.1016/S0140-6736(02)11401-2
Andersen, Y. S., Gillin, F. D., and Eckmann, L. (2006). Adaptive immunity-dependent intestinal hypermotility contributes to host defense against Giardia spp. Infect. Immun. 74, 2473–2476. doi: 10.1128/IAI.74.4.2473-2476.2006
Ankarklev, J., Svard, S. G., and Lebbad, M. (2012). Allelic sequence heterozygosity in single Giardia parasites. BMC Microbiol. 12:65. doi: 10.1186/1471-2180-12-65
Ansell, B. R., McConville, M. J., Ma’ayeh, S. Y., Dagley, M. J., Gasser, R. B., Svard, S. G., et al. (2015). Drug resistance in Giardia duodenalis. Biotechnol. Adv. 33(Pt 1), 888–901. doi: 10.1016/j.biotechadv.2015.04.009
Argenzio, R. A., Armstrong, M., and Rhoads, J. M. (1996). Role of the enteric nervous system in piglet cryptosporidiosis. J. Pharmacol. Exp. Ther. 279, 1109–1115.
Argenzio, R. A., Lecce, J., and Powell, D. W. (1993). Prostanoids inhibit intestinal NaCl absorption in experimental porcine cryptosporidiosis. Gastroenterology 104, 440–447.
Argenzio, R. A., Liacos, J. A., Levy, M. L., Meuten, D. J., Lecce, J. G., and Powell, D. W. (1990). Villous atrophy, crypt hyperplasia, cellular infiltration, and impaired glucose-Na absorption in enteric cryptosporidiosis of pigs. Gastroenterology 98, 1129–1140. doi: 10.1016/0016-5085(90)90325-U
Baldursson, S., and Karanis, P. (2011). Waterborne transmission of protozoan parasites: review of worldwide outbreaks - an update 2004–2010. Water Res. 45, 6603–6614. doi: 10.1016/j.watres.2011.10.013
Banik, S., Renner Viveros, P., Seeber, F., Klotz, C., Ignatius, R., and Aebischer, T. (2013). Giardia duodenalis arginine deiminase modulates the phenotype and cytokine secretion of human dendritic cells by depletion of arginine and formation of ammonia. Infect. Immun. 81, 2309–2317. doi: 10.1128/IAI.00004-13
Barry, M. A., Weatherhead, J. E., Hotez, P. J., and Woc-Colburn, L. (2013). Childhood parasitic infections endemic to the United States. Pediatr. Clin. North Am. 60, 471–485. doi: 10.1016/j.pcl.2012.12.011
Bartelt, L. A., Roche, J., Kolling, G., Bolick, D., Noronha, F., Naylor, C., et al. (2013). Persistent G. lamblia impairs growth in a murine malnutrition model. J. Clin. Invest. 123, 2672–2684. doi: 10.1172/JCI67294
Benere, E., Van Assche, T., Van Ginneken, C., Peulen, O., Cos, P., and Maes, L. (2012). Intestinal growth and pathology of Giardia duodenalis assemblage subtype A(I), A(II), B and E in the gerbil model. Parasitology 139, 424–433. doi: 10.1017/S0031182011002137
Berkman, D. S., Lescano, A. G., Gilman, R. H., Lopez, S. L., and Black, M. M. (2002). Effects of stunting, diarrhoeal disease, and parasitic infection during infancy on cognition in late childhood: a follow-up study. Lancet 359, 564–571. doi: 10.1016/S0140-6736(02)07744-9
Bernander, R., Palm, J. E., and Svard, S. G. (2001). Genome ploidy in different stages of the Giardia lamblia life cycle. Cell Microbiol. 3, 55–62. doi: 10.1046/j.1462-5822.2001.00094.x
Bessoff, K., Sateriale, A., Lee, K. K., and Huston, C. D. (2013). Drug repurposing screen reveals FDA-approved inhibitors of human HMG-CoA reductase and isoprenoid synthesis that block Cryptosporidium parvum growth. Antimicrob. Agents Chemother. 57, 1804–1814. doi: 10.1128/AAC.02460-12
Bessoff, K., Spangenberg, T., Foderaro, J. E., Jumani, R. S., Ward, G. E., and Huston, C. D. (2014). Identification of Cryptosporidium parvum active chemical series by Repurposing the open access malaria box. Antimicrob. Agents Chemother. 58, 2731–2739. doi: 10.1128/AAC.02641-13
Betancourt, W. Q., and Rose, J. B. (2004). Drinking water treatment processes for removal of Cryptosporidium and Giardia. Vet. Parasitol. 126, 219–234. doi: 10.1016/j.vetpar.2004.09.002
Bienz, M., Dai, W. J., Welle, M., Gottstein, B., and Muller, N. (2003). Interleukin-6-deficient mice are highly susceptible to Giardia lamblia infection but exhibit normal intestinal immunoglobulin A responses against the parasite. Infect. Immun. 71, 1569–1573. doi: 10.1128/IAI.71.3.1569-1573.2003
Bissuel, F., Cotte, L., Rabodonirina, M., Rougier, P., Piens, M. A., and Trepo, C. (1994). Paromomycin: an effective treatment for cryptosporidial diarrhea in patients with AIDS. Clin. Infect. Dis. 18, 447–449. doi: 10.1093/clinids/18.3.447
Blackwell, A. D., Martin, M., Kaplan, H., and Gurven, M. (2013). Antagonism between two intestinal parasites in humans: the importance of co-infection for infection risk and recovery dynamics. Proc. Biol. Sci. 280, 20131671. doi: 10.1098/rspb.2013.1671
Blanshard, C., Shanson, D. C., and Gazzard, B. G. (1997). Pilot studies of azithromycin, letrazuril and paromomycin in the treatment of cryptosporidiosis. Int. J. STD AIDS 8, 124–129. doi: 10.1258/0956462971919543
Blocka, K. L., Paulus, H. E., and Furst, D. E. (1986). Clinical pharmacokinetics of oral and injectable gold compounds. Clin. Pharmacokinet. 11, 133–143. doi: 10.2165/00003088-198611020-00003
Bonilla-Santiago, R., Wu, Z., Zhang, L., and Widmer, G. (2008). Identification of growth inhibiting compounds in a Giardia lamblia high-throughput screen. Mol. Biochem. Parasitol. 162, 149–154. doi: 10.1016/j.molbiopara.2008.08.005
Boucher, S. E., and Gillin, F. D. (1990). Excystation of in vitro-derived Giardia lamblia cysts. Infect. Immun. 58, 3516–3522.
Bouzid, M., Halai, K., Jeffreys, D., and Hunter, P. R. (2015). The prevalence of Giardia infection in dogs and cats, a systematic review and meta-analysis of prevalence studies from stool samples. Vet. Parasitol. 207, 181–202. doi: 10.1016/j.vetpar.2014.12.011
Busatti, H. G., Alves, R. J., Santana-Anjos, K. G., Gil, F. F., Cury, M. C., Vannier-Santos, M. A., et al. (2013). Effects of metronidazole analogues on Giardia lamblia: experimental infection and cell organization. Diagn. Microbiol. Infect. Dis. 75, 160–164. doi: 10.1016/j.diagmicrobio.2012.11.001
Byrd, L. G., Conrad, J. T., and Nash, T. E. (1994). Giardia lamblia infections in adult mice. Infect. Immun. 62, 3583–3585.
Campbell, L. D., Stewart, J. N., and Mead, J. R. (2002). Susceptibility to Cryptosporidium parvum infections in cytokine- and chemokine-receptor knockout mice. J. Parasitol. 88, 1014–1016. doi: 10.1645/0022-3395(2002)088[1014:STCPII]2.0.CO;2
Carreno, R. A., Martin, D. S., and Barta, J. R. (1999). Cryptosporidium is more closely related to the gregarines than to coccidia as shown by phylogenetic analysis of apicomplexan parasites inferred using small-subunit ribosomal RNA gene sequences. Parasitol. Res. 85, 899–904. doi: 10.1007/s004360050655
Castellanos-Gonzalez, A., Cabada, M. M., Nichols, J., Gomez, G., and White, A. C. Jr., (2013a). Human primary intestinal epithelial cells as an improved in vitro model for Cryptosporidium parvum infection. Infect. Immun. 81, 1996–2001. doi: 10.1128/IAI.01131-12
Castellanos-Gonzalez, A., White, A. C. Jr., Ojo, K. K., Vidadala, R. S., Zhang, Z., Reid, M. C., et al. (2013b). A novel calcium-dependent protein kinase inhibitor as a lead compound for treating cryptosporidiosis. J. Infect. Dis. 208, 1342–1348. doi: 10.1093/infdis/jit327
Checkley, W., White, A. C. Jr., Jaganath, D., Arrowood, M. J., Chalmers, R. M., Chen, X. M., et al. (2015). A review of the global burden, novel diagnostics, therapeutics, and vaccine targets for Cryptosporidium. Lancet Infect. Dis. 15, 85–94. doi: 10.1016/S1473-3099(14)70772-8
Chen, C. Z., Kulakova, L., Southall, N., Marugan, J. J., Galkin, A., Austin, C. P., et al. (2011). High-throughput Giardia lamblia viability assay using bioluminescent ATP content measurements. Antimicrob. Agents Chemother. 55, 667–675. doi: 10.1128/AAC.00618-10
Chen, C. Z., Southall, N., Galkin, A., Lim, K., Marugan, J. J., Kulakova, L., et al. (2012). A homogenous luminescence assay reveals novel inhibitors for Giardia lamblia carbamate kinase. Curr. Chem. Genomics 6, 93–102. doi: 10.2174/1875397301206010093
Chen, X. M., Keithly, J. S., Paya, C. V., and LaRusso, N. F. (2002). Cryptosporidiosis. N. Engl. J. Med. 346, 1723–1731. doi: 10.1056/NEJMra013170
Cosyns, M., Tsirkin, S., Jones, M., Flavell, R., Kikutani, H., and Hayward, A. R. (1998). Requirement of CD40-CD40 ligand interaction for elimination of Cryptosporidium parvum from mice. Infect. Immun. 66, 603–607.
Current, W. L., and Reese, N. C. (1986). A comparison of endogenous development of three isolates of Cryptosporidium in suckling mice. J. Protozool. 33, 98–108. doi: 10.1111/j.1550-7408.1986.tb05567.x
Dan, M., Wang, A. L., and Wang, C. C. (2000). Inhibition of pyruvate-ferredoxin oxidoreductase gene expression in Giardia lamblia by a virus-mediated hammerhead ribozyme. Mol. Microbiol. 36, 447–456. doi: 10.1046/j.1365-2958.2000.01863.x
Dann, S. M., Manthey, C. F., Le, C., Miyamoto, Y., Gima, L., Abrahim, A., et al. (2015). IL-17A promotes protective IgA responses and expression of other potential effectors against the lumen-dwelling enteric parasite Giardia. Exp. Parasitol. 156, 68–78. doi: 10.1016/j.exppara.2015.06.003
Dash, M., Padhi, S., Panda, P., and Parida, B. (2013). Intestinal protozoans in adults with diarrhea. N. Am. J. Med. Sci. 5, 707–712. doi: 10.4103/1947-2714.123261
Davids, B. J., Palm, J. E., Housley, M. P., Smith, J. R., Andersen, Y. S., Martin, M. G., et al. (2006). Polymeric immunoglobulin receptor in intestinal immune defense against the lumen-dwelling protozoan parasite Giardia. J. Immunol. 177, 6281–6290. doi: 10.4049/jimmunol.177.9.6281
Debnath, A., Parsonage, D., Andrade, R. M., He, C., Cobo, E. R., Hirata, K., et al. (2012). A high-throughput drug screen for Entamoeba histolytica identifies a new lead and target. Nat. Med. 18, 956–960. doi: 10.1038/nm.2758
Dreesen, L., De Bosscher, K., Grit, G., Staels, B., Lubberts, E., Bauge, E., et al. (2014). Giardia muris infection in mice is associated with a protective interleukin 17A response and induction of peroxisome proliferator-activated receptor alpha. Infect. Immun. 82, 3333–3340. doi: 10.1128/IAI.01536-14
Dunn, L. A., Burgess, A. G., Krauer, K. G., Eckmann, L., Vanelle, P., Crozet, M. D., et al. (2010). A new-generation 5-nitroimidazole can induce highly metronidazole-resistant Giardia lamblia in vitro. Int. J. Antimicrob. Agents 36, 37–42. doi: 10.1016/j.ijantimicag.2010.03.004
Eckmann, L., Laurent, F., Langford, T. D., Hetsko, M. L., Smith, J. R., Kagnoff, M. F., et al. (2000). Nitric oxide production by human intestinal epithelial cells and competition for arginine as potential determinants of host defense against the lumen-dwelling pathogen Giardia lamblia. J. Immunol. 164, 1478–1487. doi: 10.4049/jimmunol.164.3.1478
Edlind, T. D. (1989). Susceptibility of Giardia lamblia to aminoglycoside protein synthesis inhibitors: correlation with rRNA structure. Antimicrob. Agents Chemother. 33, 484–488. doi: 10.1128/AAC.33.4.484
Eissa, M. M., and Amer, E. I. (2012). Giardia lamblia: a new target for miltefosine. Int. J. Parasitol. 42, 443–452. doi: 10.1016/j.ijpara.2012.02.015
Erickson, M. C., and Ortega, Y. R. (2006). Inactivation of protozoan parasites in food, water, and environmental systems. J. Food Prot. 69, 2786–2808.
Escobedo, A. A., Almirall, P., Robertson, L. J., Franco, R. M., Hanevik, K., Morch, K., et al. (2010). Giardiasis: the ever-present threat of a neglected disease. Infect. Disord. Drug Targets 10, 329–348. doi: 10.2174/187152610793180821
Escobedo, A. A., and Cimerman, S. (2007). Giardiasis: a pharmacotherapy review. Expert Opin. Pharmacother. 8, 1885–1902. doi: 10.1517/14656566.8.12.1885
Escobedo, A. A., Nunez, F. A., Moreira, I., Vega, E., Pareja, A., and Almirall, P. (2003). Comparison of chloroquine, albendazole and tinidazole in the treatment of children with giardiasis. Ann. Trop. Med. Parasitol. 97, 367–371. doi: 10.1179/000349803235002290
Faubert, G. (2000). Immune response to Giardia duodenalis. Clin. Microbiol. Rev. 13, 35–54. doi: 10.1128/CMR.13.1.35-54.2000
Flanigan, T., Whalen, C., Turner, J., Soave, R., Toerner, J., Havlir, D., et al. (1992). Cryptosporidium infection and CD4 counts. Ann. Intern. Med. 116, 840–842. doi: 10.7326/0003-4819-116-10-840
Franzen, O., Jerlstrom-Hultqvist, J., Castro, E., Sherwood, E., Ankarklev, J., Reiner, D. S., et al. (2009). Draft genome sequencing of Giardia intestinalis assemblage B isolate GS: is human giardiasis caused by two different species? PLoS Pathog. 5:e1000560. doi: 10.1371/journal.ppat.1000560
Furness, B. W., Beach, M. J., and Roberts, J. M. (2000). Giardiasis surveillance–United States, 1992–1997. MMWR CDC Surveill. Summ. 49, 1–13.
Galkin, A., Kulakova, L., Melamud, E., Li, L., Wu, C., Mariano, P., et al. (2007). Characterization, kinetics, and crystal structures of fructose-1,6-bisphosphate aldolase from the human parasite, Giardia lamblia. J. Biol. Chem. 282, 4859–4867. doi: 10.1074/jbc.M609534200
Gardner, T. B., and Hill, D. R. (2001). Treatment of giardiasis. Clin. Microbiol. Rev. 14, 114–128. doi: 10.1128/CMR.14.1.114-128.2001
Geurden, T., Claerebout, E., Dursin, L., Deflandre, A., Bernay, F., Kaltsatos, V., et al. (2006). The efficacy of an oral treatment with paromomycin against an experimental infection with Giardia in calves. Vet. Parasitol. 135, 241–247. doi: 10.1016/j.vetpar.2005.09.006
Gookin, J. L., Foster, D. M., Coccaro, M. R., and Stauffer, S. H. (2008). Oral delivery of L-arginine stimulates prostaglandin-dependent secretory diarrhea in Cryptosporidium parvum-infected neonatal piglets. J. Pediatr. Gastroenterol. Nutr. 46, 139–146. doi: 10.1097/MPG.0b013e31815c0480
Gorla, S. K., Kavitha, M., Zhang, M., Chin, J. E., Liu, X., Striepen, B., et al. (2013). Optimization of benzoxazole-based inhibitors of Cryptosporidium parvum inosine 5’-monophosphate dehydrogenase. J. Med. Chem. 56, 4028–4043. doi: 10.1021/jm400241j
Gorla, S. K., Kavitha, M., Zhang, M., Liu, X., Sharling, L., Gollapalli, D. R., et al. (2012). Selective and potent urea inhibitors of Cryptosporidium parvum inosine 5’-monophosphate dehydrogenase. J. Med. Chem. 55, 7759–7771. doi: 10.1021/jm3007917
Gorla, S. K., McNair, N. N., Yang, G., Gao, S., Hu, M., Jala, V. R., et al. (2014). Validation of IMP dehydrogenase inhibitors in a mouse model of cryptosporidiosis. Antimicrob. Agents Chemother. 58, 1603–1614. doi: 10.1128/AAC.02075-13
Granados, C. E., Reveiz, L., Uribe, L. G., and Criollo, C. P. (2012). Drugs for treating giardiasis. Cochrane Database. Syst. Rev. 12, CD007787. doi: 10.1002/14651858.CD007787.pub2
Greenberg, P. D., and Cello, J. P. (1996). Treatment of severe diarrhea caused by Cryptosporidium parvum with oral bovine immunoglobulin concentrate in patients with AIDS. J. Acquir. Immune. Defic. Syndr. Hum. Retrovirol. 13, 348–354. doi: 10.1097/00042560-199612010-00008
Griffiths, J. K., Moore, R., Dooley, S., Keusch, G. T., and Tzipori, S. (1994). Cryptosporidium parvum infection of Caco-2 cell monolayers induces an apical monolayer defect, selectively increases transmonolayer permeability, and causes epithelial cell death. Infect. Immun. 62, 4506–4514.
Guarino, A., Canani, R. B., Casola, A., Pozio, E., Russo, R., Bruzzese, E., et al. (1995). Human intestinal cryptosporidiosis: secretory diarrhea and enterotoxic activity in Caco-2 cells. J. Infect. Dis. 171, 976–983. doi: 10.1093/infdis/171.4.976
Guo, F., Zhang, H., Fritzler, J. M., Rider, S. D. Jr., Xiang, L., McNair, N. N., et al. (2014). Amelioration of Cryptosporidium parvum infection in vitro and in vivo by targeting parasite fatty acyl-coenzyme A synthetases. J. Infect. Dis. 209, 1279–1287. doi: 10.1093/infdis/jit645
Gut, J., Ang, K. K., Legac, J., Arkin, M. R., Rosenthal, P. J., and McKerrow, J. H. (2011). An image-based assay for high throughput screening of Giardia lamblia. J. Microbiol. Methods 84, 398–405. doi: 10.1016/j.mimet.2010.12.026
Hahn, J., Seeber, F., Kolodziej, H., Ignatius, R., Laue, M., Aebischer, T., et al. (2013). High sensitivity of Giardia duodenalis to tetrahydrolipstatin (orlistat) in vitro. PLoS ONE 8:e71597. doi: 10.1371/journal.pone.0071597
Hanevik, K., Hausken, T., Morken, M. H., Strand, E. A., Morch, K., Coll, P., et al. (2007). Persisting symptoms and duodenal inflammation related to Giardia duodenalis infection. J. Infect. 55, 524–530. doi: 10.1016/j.jinf.2007.09.004
Hanevik, K., Wensaas, K. A., Rortveit, G., Eide, G. E., Morch, K., and Langeland, N. (2014). Irritable bowel syndrome and chronic fatigue 6 years after Giardia infection: a controlled prospective cohort study. Clin. Infect. Dis. 59, 1394–1400. doi: 10.1093/cid/ciu629
Hayward, A. R., Cosyns, M., Jones, M., and Ponnuraj, E. M. (2001). Marrow-derived CD40-positive cells are required for mice to clear Cryptosporidium parvum infection. Infect. Immun. 69, 1630–1634. doi: 10.1128/IAI.69.3.1630-1634.2001
Hernandez-Covarrubias, C., Vilchis-Reyes, M. A., Yepez-Mulia, L., Sanchez-Diaz, R., Navarrete-Vazquez, G., Hernandez-Campos, A., et al. (2012). Exploring the interplay of physicochemical properties, membrane permeability and giardicidal activity of some benzimidazole derivatives. Eur. J. Med. Chem. 52, 193–204. doi: 10.1016/j.ejmech.2012.03.014
Hewitt, R. G., Yiannoutsos, C. T., Higgs, E. S., Carey, J. T., Geiseler, P. J., Soave, R., et al. (2000). Paromomycin: no more effective than placebo for treatment of cryptosporidiosis in patients with advanced human immunodeficiency virus infection. AIDS Clin. Trial. Group. Clin. Infect. Dis. 31, 1084–1092. doi: 10.1086/318155
Heyworth, M. F. (1989). Intestinal IgA responses to Giardia muris in mice depleted of helper T lymphocytes and in immunocompetent mice. J. Parasitol. 75, 246–251. doi: 10.2307/3282773
Hijjawi, N. S., Meloni, B. P., Ryan, U. M., Olson, M. E., and Thompson, R. C. (2002). Successful in vitro cultivation of Cryptosporidium andersoni: evidence for the existence of novel extracellular stages in the life cycle and implications for the classification of Cryptosporidium. Int. J. Parasitol. 32, 1719–1726. doi: 10.1016/S0020-7519(02)00199-6
Hoffman, P. S., Sisson, G., Croxen, M. A., Welch, K., Harman, W. D., Cremades, N., et al. (2007). Antiparasitic drug nitazoxanide inhibits the pyruvate oxidoreductases of Helicobacter pylori, selected anaerobic bacteria and parasites, and Campylobacter jejuni. Antimicrob. Agents Chemother. 51, 868–876. doi: 10.1128/AAC.01159-06
House, S. A., Richter, D. J., Pham, J. K., and Dawson, S. C. (2011). Giardia flagellar motility is not directly required to maintain attachment to surfaces. PLoS Pathog. 7:e1002167. doi: 10.1371/journal.ppat.1002167
Hunter, P. R., and Nichols, G. (2002). Epidemiology and clinical features of Cryptosporidium infection in immunocompromised patients. Clin. Microbiol. Rev. 15, 145–154. doi: 10.1128/CMR.15.1.145-154.2002
Hussien, S. M., Abdella, O. H., Abu-Hashim, A. H., Aboshiesha, G. A., Taha, M. A., El-Shemy, A. S., et al. (2013). Comparative study between the effect of nitazoxanide and paromomycine in treatment of cryptosporidiosis in hospitalized children. J. Egypt Soc. Parasitol. 43, 463–470. doi: 10.12816/0006403
Jefferies, R., Yang, R., Woh, C. K., Weldt, T., Milech, N., Estcourt, A., et al. (2015). Target validation of the inosine monophosphate dehydrogenase (IMPDH) gene in Cryptosporidium using Phylomer(®) peptides. Exp. Parasitol. 148, 40–48. doi: 10.1016/j.exppara.2014.11.003
Jerlstrom-Hultqvist, J., Ankarklev, J., and Svard, S. G. (2010). Is human giardiasis caused by two different Giardia species? Gut. Microbes 1, 379–382. doi: 10.4161/gmic.1.6.13608
Kamda, J. D., Nash, T. E., and Singer, S. M. (2012). Giardia duodenalis: dendritic cell defects in IL-6 deficient mice contribute to susceptibility to intestinal infection. Exp. Parasitol. 130, 288–291. doi: 10.1016/j.exppara.2012.01.003
Kang, J. M., Ju, H. L., Yu, J. R., Sohn, W. M., and Na, B. K. (2012). Cryptostatin, a chagasin-family cysteine protease inhibitor of Cryptosporidium parvum. Parasitology 139, 1029–1037. doi: 10.1017/S0031182012000297
Kapel, N., Huneau, J. F., Magne, D., Tome, D., and Gobert, J. G. (1997). Cryptosporidiosis-induced impairment of ion transport and Na+-glucose absorption in adult immunocompromised mice. J. Infect. Dis. 176, 834–837. doi: 10.1086/517316
Karanis, P., Kourenti, C., and Smith, H. (2007). Waterborne transmission of protozoan parasites: a worldwide review of outbreaks and lessons learnt. J. Water Health 5, 1–38. doi: 10.2166/wh.2006.002
Knodler, L. A., Sekyere, E. O., Stewart, T. S., Schofield, P. J., and Edwards, M. R. (1998). Cloning and expression of a prokaryotic enzyme, arginine deiminase, from a primitive eukaryote Giardia intestinalis. J. Biol. Chem. 273, 4470–4477. doi: 10.1074/jbc.273.8.4470
Kotloff, K. L., Nataro, J. P., Blackwelder, W. C., Nasrin, D., Farag, T. H., Panchalingam, S., et al. (2013). Burden and aetiology of diarrhoeal disease in infants and young children in developing countries (the Global Enteric Multicenter Study, GEMS): a prospective, case-control study. Lancet 382, 209–222. doi: 10.1016/S0140-6736(13)60844-2
Kuhls, T. L., Greenfield, R. A., Mosier, D. A., Crawford, D. L., and Joyce, W. A. (1992). Cryptosporidiosis in adult and neonatal mice with severe combined immunodeficiency. J. Comp. Pathol. 106, 399–410. doi: 10.1016/0021-9975(92)90024-O
Kulakova, L., Galkin, A., Chen, C. Z., Southall, N., Marugan, J. J., Zheng, W., et al. (2014). Discovery of novel antigiardiasis drug candidates. Antimicrob. Agents Chemother. 58, 7303–7311. doi: 10.1128/AAC.03834-14
Kuntz, A. N., Davioud-Charvet, E., Sayed, A. A., Califf, L. L., Dessolin, J., Arner, E. S., et al. (2007). Thioredoxin glutathione reductase from Schistosoma mansoni: an essential parasite enzyme and a key drug target. PLoS Med 4:e206. doi: 10.1371/journal.pmed.0040206
Lal, A., and Hales, S. (2015). Heterogeneity in hotspots: spatio-temporal patterns in neglected parasitic diseases. Epidemiol. Infect. 143, 631–639. doi: 10.1017/S0950268814001101
Langford, T. D., Housley, M. P., Boes, M., Chen, J., Kagnoff, M. F., Gillin, F. D., et al. (2002). Central importance of immunoglobulin A in host defense against Giardia spp. Infect. Immun. 70, 11–18. doi: 10.1128/IAI.70.1.11-18.2002
Laurent, F., Eckmann, L., Savidge, T. C., Morgan, G., Theodos, C., Naciri, M., et al. (1997). Cryptosporidium parvum infection of human intestinal epithelial cells induces the polarized secretion of C-X-C chemokines. Infect. Immun. 65, 5067–5073.
Laurent, F., Kagnoff, M. F., Savidge, T. C., Naciri, M., and Eckmann, L. (1998). Human intestinal epithelial cells respond to Cryptosporidium parvum infection with increased prostaglandin H synthase 2 expression and prostaglandin E2 and F2alpha production. Infect. Immun. 66, 1787–1790.
Laurent, F., McCole, D., Eckmann, L., and Kagnoff, M. F. (1999). Pathogenesis of Cryptosporidium parvum infection. Microbes Infect. 1, 141–148. doi: 10.1016/S1286-4579(99)80005-7
Lauwaet, T., Andersen, Y., Van de Ven, L., Eckmann, L., and Gillin, F. D. (2010). Rapid detachment of Giardia lamblia trophozoites as a mechanism of antimicrobial action of the isoflavone formononetin. J. Antimicrob. Chemother. 65, 531–534. doi: 10.1093/jac/dkp501
Leitsch, D., Burgess, A. G., Dunn, L. A., Krauer, K. G., Tan, K., Duchene, M., et al. (2011). Pyruvate:ferredoxin oxidoreductase and thioredoxin reductase are involved in 5-nitroimidazole activation while flavin metabolism is linked to 5-nitroimidazole resistance in Giardia lamblia. J. Antimicrob. Chemother. 66, 1756–1765. doi: 10.1093/jac/dkr192
Lemee, V., Zaharia, I., Nevez, G., Rabodonirina, M., Brasseur, P., Ballet, J. J., et al. (2000). Metronidazole and albendazole susceptibility of 11 clinical isolates of Giardia duodenalis from France. J. Antimicrob. Chemother. 46, 819–821. doi: 10.1093/jac/46.5.819
Li, E., Zhou, P., and Singer, S. M. (2006). Neuronal nitric oxide synthase is necessary for elimination of Giardia lamblia infections in mice. J. Immunol. 176, 516–521. doi: 10.4049/jimmunol.176.1.516
Li, Z., Kulakova, L., Li, L., Galkin, A., Zhao, Z., Nash, T. E., et al. (2009). Mechanisms of catalysis and inhibition operative in the arginine deiminase from the human pathogen Giardia lamblia. Bioorg. Chem. 37, 149–161. doi: 10.1016/j.bioorg.2009.06.001
Li, Z., Liu, Z., Cho, D. W., Zou, J., Gong, M., Breece, R. M., et al. (2011). Rational design, synthesis and evaluation of first generation inhibitors of the Giardia lamblia fructose-1,6-biphosphate aldolase. J. Inorg. Biochem. 105, 509–517. doi: 10.1016/j.jinorgbio.2010.12.012
Liu, J., Kabir, F., Manneh, J., Lertsethtakarn, P., Begum, S., Gratz, J., et al. (2014). Development and assessment of molecular diagnostic tests for 15 enteropathogens causing childhood diarrhoea: a multicentre study. Lancet Infect. Dis. 14, 716–724. doi: 10.1016/S1473-3099(14)70808-4
Manna, D., Dutta, P. K., Achari, B., and Lohia, A. (2010). A novel galacto-glycerolipid from Oxalis corniculata kills Entamoeba histolytica and Giardia lamblia. Antimicrob. Agents Chemother. 54, 4825–4832. doi: 10.1128/AAC.00546-10
Mathur, M. K., Verma, A. K., Makwana, G. E., and Sinha, M. (2013). Study of opportunistic intestinal parasitic infections in human immunodeficiency virus/acquired immunodeficiency syndrome patients. J. Glob. Infect. Dis. 5, 164–167. doi: 10.4103/0974-777X.122012
Minotto, L., Tutticci, E. A., Bagnara, A. S., Schofield, P. J., and Edwards, M. R. (1999). Characterisation and expression of the carbamate kinase gene from Giardia intestinalis. Mol. Biochem. Parasitol. 98, 43–51. doi: 10.1016/S0166-6851(98)00141-8
Miyamoto, Y., Kalisiak, J., Korthals, K., Lauwaet, T., Cheung, D. Y., Lozano, R., et al. (2013). Expanded therapeutic potential in activity space of next-generation 5-nitroimidazole antimicrobials with broad structural diversity. Proc. Natl. Acad. Sci. U.S.A. 110, 17564–17569. doi: 10.1073/pnas.1302664110
Morch, K., Hanevik, K., Robertson, L. J., Strand, E. A., and Langeland, N. (2008). Treatment-ladder and genetic characterisation of parasites in refractory giardiasis after an outbreak in Norway. J. Infect. 56, 268–273. doi: 10.1016/j.jinf.2008.01.013
Morrison, H. G., McArthur, A. G., Gillin, F. D., Aley, S. B., Adam, R. D., Olsen, G. J., et al. (2007). Genomic minimalism in the early diverging intestinal parasite Giardia lamblia. Science 317, 1921–1926. doi: 10.1126/science.1143837
Muller, J., Ley, S., Felger, I., Hemphill, A., and Muller, N. (2008). Identification of differentially expressed genes in a Giardia lamblia WB C6 clone resistant to nitazoxanide and metronidazole. J. Antimicrob. Chemother. 62, 72–82. doi: 10.1093/jac/dkn142
Murphy, R. C., Ojo, K. K., Larson, E. T., Castellanos-Gonzalez, A., Perera, B. G., Keyloun, K. R., et al. (2010). Discovery of potent and selective inhibitors of calcium-dependent protein kinase 1 (CDPK1) from C. parvum and T. gondii. ACS Med. Chem. Lett. 1, 331–335. doi: 10.1021/ml100096t
Nash, T. E., Herrington, D. A., Losonsky, G. A., and Levine, M. M. (1987). Experimental human infections with Giardia lamblia. J. Infect. Dis. 156, 974–984. doi: 10.1093/infdis/156.6.974
Navarrete-Vazquez, G., Chavez-Silva, F., Argotte-Ramos, R., Rodriguez-Gutierrez Mdel, C., Chan-Bacab, M. J., Cedillo-Rivera, R., et al. (2011). Synthesis of benzologues of Nitazoxanide and Tizoxanide: a comparative study of their in vitro broad-spectrum antiprotozoal activity. Bioorg. Med. Chem. Lett. 21, 3168–3171. doi: 10.1016/j.bmcl.2011.02.100
Navarrete-Vazquez, G., Chavez-Silva, F., Colin-Lozano, B., Estrada-Soto, S., Hidalgo-Figueroa, S., Guerrero-Alvarez, J., et al. (2015). Synthesis of nitro(benzo)thiazole acetamides and in vitro antiprotozoal effect against amitochondriate parasites Giardia intestinalis and Trichomonas vaginalis. Bioorg. Med. Chem. 23, 2204–2210. doi: 10.1016/j.bmc.2015.02.059
Nava-Zuazo, C., Chavez-Silva, F., Moo-Puc, R., Chan-Bacab, M. J., Ortega-Morales, B. O., Moreno-Diaz, H., et al. (2014). 2-acylamino-5-nitro-1,3-thiazoles: preparation and in vitro bioevaluation against four neglected protozoan parasites. Bioorg. Med. Chem. 22, 1626–1633. doi: 10.1016/j.bmc.2014.01.029
Ndao, M., Nath-Chowdhury, M., Sajid, M., Marcus, V., Mashiyama, S. T., Sakanari, J., et al. (2013). A cysteine protease inhibitor rescues mice from a lethal Cryptosporidium parvum infection. Antimicrob. Agents Chemother. 57, 6063–6073. doi: 10.1128/AAC.00734-13
Nematian, J., Gholamrezanezhad, A., and Nematian, E. (2008). Giardiasis and other intestinal parasitic infections in relation to anthropometric indicators of malnutrition: a large, population-based survey of schoolchildren in Tehran. Ann. Trop. Med. Parasitol. 102, 209–214. doi: 10.1179/136485908X267876
Nesterenko, M. V., Tilley, M., and Upton, S. J. (1995). A metallo-dependent cysteine proteinase of Cryptosporidium parvum associated with the surface of sporozoites. Microbios 83, 77–88.
Nillius, D., Muller, J., and Muller, N. (2011). Nitroreductase (GlNR1) increases susceptibility of Giardia lamblia and Escherichia coli to nitro drugs. J. Antimicrob. Chemother. 66, 1029–1035. doi: 10.1093/jac/dkr029
Oberhuber, G., Kastner, N., and Stolte, M. (1997). Giardiasis: a histologic analysis of 567 cases. Scand. J. Gastroenterol. 32, 48–51. doi: 10.3109/00365529709025062
Ojo, K. K., Larson, E. T., Keyloun, K. R., Castaneda, L. J., Derocher, A. E., Inampudi, K. K., et al. (2010). Toxoplasma gondii calcium-dependent protein kinase 1 is a target for selective kinase inhibitors. Nat. Struct. Mol. Biol. 17, 602–607. doi: 10.1038/nsmb.1818
Olson, M. E., Ceri, H., and Morck, D. W. (2000). Giardia vaccination. Parasitol. Today 16, 213–217. doi: 10.1016/S0169-4758(99)01623-3
Olson, M. E., Hannigan, C. J., Gaviller, P. F., and Fulton, L. A. (2001). The use of a Giardia vaccine as an immunotherapeutic agent in dogs. Can. Vet. J. 42, 865–868.
Operario, D. J., Bristol, L. S., Liotta, J., Nydam, D. V., and Houpt, E. R. (2015). Correlation between diarrhea severity and oocyst count via quantitative PCR or fluorescence microscopy in experimental cryptosporidiosis in calves. Am. J. Trop. Med. Hyg. 92, 45–49. doi: 10.4269/ajtmh.14-0488
Overturf, G. D. (1994). Endemic giardiasis in the United States–role of the daycare center. Clin. Infect. Dis. 18, 764–765. doi: 10.1093/clinids/18.5.764
Painter, J. E., Hlavsa, M. C., Collier, S. A., Xiao, L., and Yoder, J. S. (2015). Cryptosporidiosis surveillance - United States, 2011-2012. MMWR Surveill. Summ. 64(Suppl. 3), 1–14.
Pal, D., Banerjee, S., Cui, J., Schwartz, A., Ghosh, S. K., and Samuelson, J. (2009). Giardia, Entamoeba, and Trichomonas enzymes activate metronidazole (nitroreductases) and inactivate metronidazole (nitroimidazole reductases). Antimicrob. Agents Chemother. 53, 458–464. doi: 10.1128/AAC.00909-08
Paris, C., Loiseau, P. M., Bories, C., and Breard, J. (2004). Miltefosine induces apoptosis-like death in Leishmania donovani promastigotes. Antimicrob. Agents Chemother. 48, 852–859. doi: 10.1128/AAC.48.3.852-859.2004
Perez-Cordon, G., Nie, W., Schmidt, D., Tzipori, S., and Feng, H. (2011). Involvement of host calpain in the invasion of Cryptosporidium parvum. Microbes Infect. 13, 103–107. doi: 10.1016/j.micinf.2010.10.007
Perez-Villanueva, J., Hernandez-Campos, A., Yepez-Mulia, L., Mendez-Cuesta, C., Mendez-Lucio, O., Hernandez-Luis, F., et al. (2013). Synthesis and antiprotozoal activity of novel 2-{[2-(1H-imidazol-1-yl)ethyl]sulfanyl}-1H-benzimidazole derivatives. Bioorg. Med. Chem. Lett. 23, 4221–4224. doi: 10.1016/j.bmcl.2013.05.012
Perez-Villanueva, J., Santos, R., Hernandez-Campos, A., Giulianotti, M. A., Castillo, R., and Medina-Franco, J. L. (2010). Towards a systematic characterization of the antiprotozoal activity landscape of benzimidazole derivatives. Bioorg. Med. Chem. 18, 7380–7391. doi: 10.1016/j.bmc.2010.09.019
Requena-Mendez, A., Goni, P., Lobez, S., Oliveira, I., Aldasoro, E., Valls, M. E., et al. (2014). A family cluster of giardiasis with variable treatment responses: refractory giardiasis in a family after a trip to India. Clin. Microbiol. Infect. 20, O135–O138. doi: 10.1111/1469-0691.12327
Rossignol, J. F., Ayoub, A., and Ayers, M. S. (2001). Treatment of diarrhea caused by Cryptosporidium parvum: a prospective randomized, double-blind, placebo-controlled study of Nitazoxanide. J. Infect. Dis. 184, 103–106. doi: 10.1086/321008
Rossignol, J. F., Hidalgo, H., Feregrino, M., Higuera, F., Gomez, W. H., Romero, J. L., et al. (1998). A double-‘blind’ placebo-controlled study of nitazoxanide in the treatment of cryptosporidial diarrhoea in AIDS patients in Mexico. Trans. R. Soc. Trop. Med. Hyg. 92, 663–666. doi: 10.1016/S0035-9203(98)90804-5
Rossignol, J. F., Lopez-Chegne, N., Julcamoro, L. M., Carrion, M. E., and Bardin, M. C. (2012). Nitazoxanide for the empiric treatment of pediatric infectious diarrhea. Trans. R. Soc. Trop. Med. Hyg. 106, 167–173. doi: 10.1016/j.trstmh.2011.11.007
Roxstrom-Lindquist, K., Palm, D., Reiner, D., Ringqvist, E., and Svard, S. G. (2006). Giardia immunity–an update. Trends Parasitol. 22, 26–31. doi: 10.1016/j.pt.2005.11.005
Ryan, U., and Hijjawi, N. (2015). New developments in Cryptosporidium research. Int. J. Parasitol. 45, 367–373. doi: 10.1016/j.ijpara.2015.01.009
Sharp, T. W., Thornton, S. A., Wallace, M. R., Defraites, R. F., Sanchez, J. L., Batchelor, R. A., et al. (1995). Diarrheal disease among military personnel during Operation Restore Hope, Somalia, 1992–1993. Am. J. Trop. Med. Hyg. 52, 188–193.
Singer, S. M., and Nash, T. E. (2000a). T-cell-dependent control of acute Giardia lamblia infections in mice. Infect. Immun. 68, 170–175. doi: 10.1128/IAI.68.1.170-175.2000
Singer, S. M., and Nash, T. E. (2000b). The role of normal flora in Giardia lamblia infections in mice. J. Infect. Dis. 181, 1510–1512. doi: 10.1086/315409
Smith, H. V., Nichols, R. A., and Grimason, A. M. (2005). Cryptosporidium excystation and invasion: getting to the guts of the matter. Trends Parasitol. 21, 133–142. doi: 10.1016/j.pt.2005.01.007
Solaymani-Mohammadi, S., Genkinger, J. M., Loffredo, C. A., and Singer, S. M. (2010). A meta-analysis of the effectiveness of albendazole compared with metronidazole as treatments for infections with Giardia duodenalis. PLoS Negl. Trop. Dis. 4:e682. doi: 10.1371/journal.pntd.0000682
Solaymani-Mohammadi, S., and Singer, S. M. (2010). Giardia duodenalis: the double-edged sword of immune responses in giardiasis. Exp. Parasitol. 126, 292–297. doi: 10.1016/j.exppara.2010.06.014
Solaymani-Mohammadi, S., and Singer, S. M. (2011). Host immunity and pathogen strain contribute to intestinal disaccharidase impairment following gut infection. J. Immunol. 187, 3769–3775. doi: 10.4049/jimmunol.1100606
Speelman, P. (1985). Single-dose tinidazole for the treatment of giardiasis. Antimicrob. Agents Chemother. 27, 227–229. doi: 10.1128/AAC.27.2.227
Striepen, B. (2013). Parasitic infections: time to tackle cryptosporidiosis. Nature 503, 189–191. doi: 10.1038/503189a
Striepen, B., Pruijssers, A. J., Huang, J., Li, C., Gubbels, M. J., Umejiego, N. N., et al. (2004). Gene transfer in the evolution of parasite nucleotide biosynthesis. Proc. Natl. Acad. Sci. U.S.A. 101, 3154–3159. doi: 10.1073/pnas.0304686101
Sun, Z., Khan, J., Makowska-Grzyska, M., Zhang, M., Cho, J. H., Suebsuwong, C., et al. (2014). Synthesis, in vitro evaluation and cocrystal structure of 4-oxo-[1]benzopyrano[4,3-c]pyrazole Cryptosporidium parvum inosine 5’-monophosphate dehydrogenase (CpIMPDH) inhibitors. J. Med. Chem. 57, 10544–10550. doi: 10.1021/jm501527z
Tako, E. A., Hassimi, M. F., Li, E., and Singer, S. M. (2013). Transcriptomic analysis of the host response to Giardia duodenalis infection reveals redundant mechanisms for parasite control. MBio 4:e00660. doi: 10.1128/mBio.00660-13
Tejman-Yarden, N., Millman, M., Lauwaet, T., Davids, B. J., Gillin, F. D., Dunn, L., et al. (2011). Impaired parasite attachment as fitness cost of metronidazole resistance in Giardia lamblia. Antimicrob. Agents Chemother. 55, 4643–4651. doi: 10.1128/AAC.00384-11
Tejman-Yarden, N., Miyamoto, Y., Leitsch, D., Santini, J., Debnath, A., Gut, J., et al. (2013). A reprofiled drug, auranofin, is effective against metronidazole-resistant Giardia lamblia. Antimicrob. Agents Chemother. 57, 2029–2035. doi: 10.1128/AAC.01675-12
Theodos, C. M., Sullivan, K. L., Griffiths, J. K., and Tzipori, S. (1997). Profiles of healing and nonhealing Cryptosporidium parvum infection in C57BL/6 mice with functional B and T lymphocytes: the extent of gamma interferon modulation determines the outcome of infection. Infect. Immun. 65, 4761–4769.
Thomas Iv, L. J., Zweig, A. P., and Tosh, A. K. (2014). An adolescent with chronic giardiasis mimicking anorexia nervosa. Int. J. Adolesc. Med. Health 26, 293–295. doi: 10.1515/ijamh-2013-0506
Tzipori, S., and Griffiths, J. K. (1998). Natural history and biology of Cryptosporidium parvum. Adv. Parasitol. 40, 5–36. doi: 10.1016/S0065-308X(08)60116-5
Tzipori, S., McCartney, E., Lawson, G. H., Rowland, A. C., and Campbell, I. (1981). Experimental infection of piglets with Cryptosporidium. Res. Vet. Sci. 31, 358–368.
Tzipori, S., Smith, M., Halpin, C., Angus, K. W., Sherwood, D., and Campbell, I. (1983). Experimental cryptosporidiosis in calves: clinical manifestations and pathological findings. Vet. Rec. 112, 116–120. doi: 10.1136/vr.112.6.116
Umejiego, N. N., Gollapalli, D., Sharling, L., Volftsun, A., Lu, J., Benjamin, N. N., et al. (2008). Targeting a prokaryotic protein in a eukaryotic pathogen: identification of lead compounds against cryptosporidiosis. Chem. Biol. 15, 70–77. doi: 10.1016/j.chembiol.2007.12.010
Umejiego, N. N., Li, C., Riera, T., Hedstrom, L., and Striepen, B. (2004). Cryptosporidium parvum IMP dehydrogenase: identification of functional, structural, and dynamic properties that can be exploited for drug design. J. Biol. Chem. 279, 40320–40327. doi: 10.1074/jbc.M407121200
Upcroft, J. A., Campbell, R. W., and Upcroft, P. (1996a). Quinacrine-resistant Giardia duodenalis. Parasitology 112(Pt 3), 309–313. doi: 10.1017/S0031182000065823
Upcroft, J., Mitchell, R., Chen, N., and Upcroft, P. (1996b). Albendazole resistance in Giardia is correlated with cytoskeletal changes but not with a mutation at amino acid 200 in beta-tubulin. Microb. Drug Resist. 2, 303–308. doi: 10.1089/mdr.1996.2.303
Upcroft, J. A., Dunn, L. A., Wright, J. M., Benakli, K., Upcroft, P., and Vanelle, P. (2006). 5-Nitroimidazole drugs effective against metronidazole-resistant Trichomonas vaginalis and Giardia duodenalis. Antimicrob. Agents Chemother. 50, 344–347. doi: 10.1128/AAC.50.1.344-347.2006
Upcroft, P., and Upcroft, J. A. (2001). Drug targets and mechanisms of resistance in the anaerobic protozoa. Clin. Microbiol. Rev. 14, 150–164. doi: 10.1128/CMR.14.1.150-164.2001
Upton, S. J., Tilley, M., and Brillhart, D. B. (1994). Comparative development of Cryptosporidium parvum (Apicomplexa) in 11 continuous host cell lines. FEMS Microbiol. Lett. 118, 233–236. doi: 10.1111/j.1574-6968.1994.tb06833.x
Upton, S. J., Tilley, M., and Brillhart, D. B. (1995). Effects of select medium supplements on in vitro development of Cryptosporidium parvum in HCT-8 cells. J. Clin. Microbiol. 33, 371–375.
Urban, J. F. Jr., Fayer, R., Chen, S. J., Gause, W. C., Gately, M. K., Finkelman, F. D., et al. (1996). IL-12 protects immunocompetent and immunodeficient neonatal mice against infection with Cryptosporidium parvum. J. Immunol. 156, 263–268.
Valdez, C. A., Tripp, J. C., Miyamoto, Y., Kalisiak, J., Hruz, P., Andersen, Y. S., et al. (2009). Synthesis and electrochemistry of 2-ethenyl and 2-ethanyl derivatives of 5-nitroimidazole and antimicrobial activity against Giardia lamblia. J. Med. Chem. 52, 4038–4053. doi: 10.1021/jm900356n
Vandenberg, O., Robberecht, F., Dauby, N., Moens, C., Talabani, H., Dupont, E., et al. (2012). Management of a Cryptosporidium hominis outbreak in a day-care center. Pediatr. Infect. Dis. J. 31, 10–15. doi: 10.1097/INF.0b013e318235ab64
von Oettingen, J., Nath-Chowdhury, M., Ward, B. J., Rodloff, A. C., Arrowood, M. J., and Ndao, M. (2008). High-yield amplification of Cryptosporidium parvum in interferon gamma receptor knockout mice. Parasitology 135, 1151–1156. doi: 10.1017/S0031182008004757
Watt, P. M. (2009). Phenotypic screening of phylomer peptide libraries derived from genome fragments to identify and validate new targets and therapeutics. Future Med. Chem. 1, 257–265. doi: 10.4155/fmc.09.28
Widerstrom, M., Schonning, C., Lilja, M., Lebbad, M., Ljung, T., Allestam, G., et al. (2014). Large outbreak of Cryptosporidium hominis infection transmitted through the public water supply, Sweden. Emerg. Infect. Dis. 20, 581–589. doi: 10.3201/eid2004.121415
Woessner, D. J., and Dawson, S. C. (2012). The Giardia median body protein is a ventral disc protein that is critical for maintaining a domed disc conformation during attachment. Eukaryot. Cell 11, 292–301. doi: 10.1128/EC.05262-11
Wright, J. M., Dunn, L. A., Upcroft, P., and Upcroft, J. A. (2003). Efficacy of antigiardial drugs. Expert Opin. Drug Saf. 2, 529–541. doi: 10.1517/14740338.2.6.529
Xu, P., Widmer, G., Wang, Y., Ozaki, L. S., Alves, J. M., Serrano, M. G., et al. (2004). The genome of Cryptosporidium hominis. Nature 431, 1107–1112. doi: 10.1038/nature02977
Yuan, J., Cheng, K. C., Johnson, R. L., Huang, R., Pattaradilokrat, S., Liu, A., et al. (2011). Chemical genomic profiling for antimalarial therapies, response signatures, and molecular targets. Science 333, 724–729. doi: 10.1126/science.1205216
Zhang, Y., Lee, B., Thompson, M., Glass, R., Cama, R. I., Figueroa, D., et al. (2000). Lactulose-mannitol intestinal permeability test in children with diarrhea caused by rotavirus and Cryptosporidium. Diarrhea Working Group, Peru. J. Pediatr. Gastroenterol. Nutr. 31, 16–21. doi: 10.1097/00005176-200007000-00006
Keywords: diarrhea, neglected diseases, protozoan parasites, cryptosporidiosis, giardiasis, antimicrobial drugs, drug development
Citation: Miyamoto Y and Eckmann L (2015) Drug Development Against the Major Diarrhea-Causing Parasites of the Small Intestine, Cryptosporidium and Giardia. Front. Microbiol. 6:1208. doi: 10.3389/fmicb.2015.01208
Received: 26 June 2015; Accepted: 16 October 2015;
Published: 19 November 2015.
Edited by:
Tzi Bun Ng, The Chinese University of Hong Kong, ChinaReviewed by:
Atte Von Wright, University of Eastern Finland, FinlandGuan Zhu, University of Georgia, USA
Copyright © 2015 Miyamoto and Eckmann. This is an open-access article distributed under the terms of the Creative Commons Attribution License (CC BY). The use, distribution or reproduction in other forums is permitted, provided the original author(s) or licensor are credited and that the original publication in this journal is cited, in accordance with accepted academic practice. No use, distribution or reproduction is permitted which does not comply with these terms.
*Correspondence: Lars Eckmann, bGVja21hbm5AdWNzZC5lZHU=