- 1Department of Botany, Banaras Hindu University, Varanasi, India
- 2Institute of Environment and Sustainable Development, Banaras Hindu University, Varanasi, India
- 3Molecular Glycobiotechnology Group, Discipline of Biochemistry, School of Natural Sciences, National University of Ireland Galway, Galway, Ireland
Numerous plants and fungi produce mannitol, which may serve as an osmolyte or metabolic store; furthermore, mannitol also acts as a powerful quencher of reactive oxygen species (ROS). Some phytopathogenic fungi use mannitol to stifle ROS-mediated plant resistance. Mannitol is essential in pathogenesis to balance cell reinforcements produced by both plants and animals. Mannitol likewise serves as a source of reducing power, managing coenzymes, and controlling cytoplasmic pH by going about as a sink or hotspot for protons. The metabolic pathways for mannitol biosynthesis and catabolism have been characterized in filamentous fungi by direct diminishment of fructose-6-phosphate into mannitol-1-phosphate including a mannitol-1-phosphate phosphatase catalyst. In plants mannitol is integrated from mannose-6-phosphate to mannitol-1-phosphate, which then dephosphorylates to mannitol. The enzyme mannitol dehydrogenase plays a key role in host–pathogen interactions and must be co-localized with pathogen-secreted mannitol to resist the infection.
Introduction
Mannitol, a six carbon non-cyclic sugar liquor, is a polyol commonly found in plants and fungi. In plant species, mannitol appears to be, in every way, to be the most expansive, being found in more than 70 families (Lewis and Smith, 1967; Ruijter et al., 2003). In plants, mannitol is made despite sucrose and is a phloem-translocated photoassimilate. Mannitol has various capacities in the plants including serving as a carbon stockpiling compound (Lewis, 1984), as a store of reducing power (Stacey, 1974; Loescher, 1987; Stoop and Pharr, 1992), as a compatible osmolyte (Brown and Simpson, 1972; Yancey et al., 1982) and in osmoregulation (Hellebust, 1976). Mannitol has similarly been shown to be an oxygen radical quencher both in vitro (Smirnoff and Cumbes, 1989) and in vivo (Shen et al., 1997a,b). Mannitol is the essential translocated sugar when the sucrose pool is drained (Davis and Loescher, 1990). It may moreover be incorporated in the utilization of photochemical impulses (Jennings et al., 2002).
Mannitol is the most widely recognized polyol in fungi, where it is found in spores, fruiting bodies, and mycelia (Solomon et al., 2007), and is considered to be the abundant most of all dissolvable starches inside mycelia and fruiting bodies (Lewis and Smith, 1967; Horer et al., 2001; Dulermo et al., 2009). In fungi, mannitol is a storage or a translocated carbohydrate, and is essential in spore germination under starvation conditions (Horikoshi et al., 1965; Lewis and Smith, 1967; Dijkema et al., 1985; Witteveen and Visser, 1995). Mannitol additionally extinguishes reactive oxygen species (ROS; Smirnoff and Cumbes, 1989; Chaturvedi et al., 1997; Voegele et al., 2005), prompting the speculation that it can assume a cell reinforcement part in host–pathogen interactions. As a case in point, mannitol-deficient mutants of Cryptococcus neoformans are less harmful than the wild type strain, probably because of the way that mannitol ensures protection against oxidative executing by phagocytic cells (Chaturvedi et al., 1996a,b).
Mannitol production and secretion are required for the pathogenicity of several fungal pathogens of both animals and plants (Chaturvedi et al., 1996a,b). In infecting tobacco, Alternaria alternata secretes mannitol, which is induced by host leaf extracts (Jennings et al., 1998). This observation revealed that fungal pathogens secrete mannitol to quench the ROS that mediate plant defenses. In response, pathogen-induced mannitol dehydrogenase (MTD) in the plant catabolize the pathogen’s secreted mannitol, thus protecting the plants ROS-mediated defenses. Mannitol deficient mutants of A. alternata created by target gene disruption had reduced pathogenicity on tobacco (Vélëz et al., 2007, 2008), confirming that mannitol production and secretion was a significant factor for pathogenicity of this fungus.
Mannitol Biosynthesis in Fungi
The metabolic pathway for mannitol biosynthesis and catabolism is well described in filamentous fungi. Mannitol metabolism in fungus is cyclical process (Hult and Gatenbeck, 1978). Figure 1 depicts the pathways of mannitol synthesis in organisms. In this cycle, mannitol-1-phosphate 5-dehydrogenase (MPD; EC 1.1.1.17) was proposed to decrease Fructose-6-phosphate into mannitol-1-phosphate utilizing the NADH cofactor, processed by dephosphorylation by mannitol-1-phosphate phosphatase (MPP; EC 3.1.3.22), into an inorganic phosphate mannitol. Mannitol would then be oxidized to fructose by MTD (EC 1.1.1.138) utilizing the NADP+ cofactor. At last, fructose would be phosphorylated to fructose-6-phosphate by a hexokinase (HX; EC 2.7.1.1). Dephosphorylation of mannitol-1-phosphate into mannitol by means of MPP was portrayed as being irreversible. Thus, the proposed cycle would run in one direction with MPD as a catabolic enzyme system (Calmes et al., 2013).
The physiological functions of mannitol in fungi have been widely studied, and sometimes just as widely disputed. Mannitol serves as a store carbon source and as reservoir of reducing power (Hult et al., 1980), and other functions of mannitol have been proposed that incorporate stress resilience (Ruijter et al., 2003) and spore dispersal (Trail and Xu, 2002). The mannitol biosynthetic mutants served as a very useful model when in tobacco inoculation experiments, mutants with reduced mannitol production demonstrated an equally parallel reduction in disease symptoms (Vélëz et al., 2007).
A few fungal systems build stress resistance by collecting mannitol. The conidia of the cereal pathogen Gibberella zeae were promptly changed to chlamydospore-like structures (CLS) in cultures supplemented with high measures of mannitol (Reyneri, 2006; Son et al., 2012). These morphological changes in CLS, mannitol amassing may be specifically identified with expanded CLS stress resistance. Numerous parasitic species amass mannitol in their hyphae and spores, up to 10–15% of the dry weight (Witteveen and Visser, 1995). It can be hypothesized, based on information gained from model species, that this mannitol will be important for spore germination or hyphal protein during pathogenesis.
Mannitol Biosynthesis in Plants
In higher plants more than 13 polyols are confirmed (Bieleski, 1982; Lewis, 1984), in which polyol mannitol may be the most wide spread, being found in >100 types of plants in 70 families (Lewis and Smith, 1967; Jennings, 1984; Stoop et al., 1996). Table 1 lists plants that contain mannitol. In celery, mannitol assumes a huge part as an osmoprotectant and in addition interchange carbon and energy source (Loescher et al., 1995; Pharr et al., 1995a,b; Stoop et al., 1996; Nuccio et al., 1999; Loescher and Everard, 2000; Williamson et al., 2013). Mannitol is produced in higher plants from mannose-6-phosphate through the activity of a NADPH-mannose-6-phosphate reductase (M6PR). Mannose-6-phosphate is converted to mannitol-1-phosphate by the assistance of the M6PR enzyme, and then mannitol-1-phosphate is dephoshorylated by a phosphatase to mannitol (Rumpho et al., 1983; Loescher et al., 1992).
Figure 2 illustrates the pathways of mannitol metabolism in plants. In celery, mannitol synthesis occurs in mature leaves where M6PR is localized in the cytosol of green palisade and spongy parenchyma tissues and bundle-sheath cells (Everard et al., 1993; Loescher et al., 1995). M6PR activity is regulated by light and the development stage of the plant tissue. In mature leaves of celery, the M6PR activity is high but in sink tissues such as roots and unstressed, immature leaves, no M6PR activity is detected (Everard et al., 1993, 1997; Stoop and Pharr, 1994; Jennings et al., 2002).
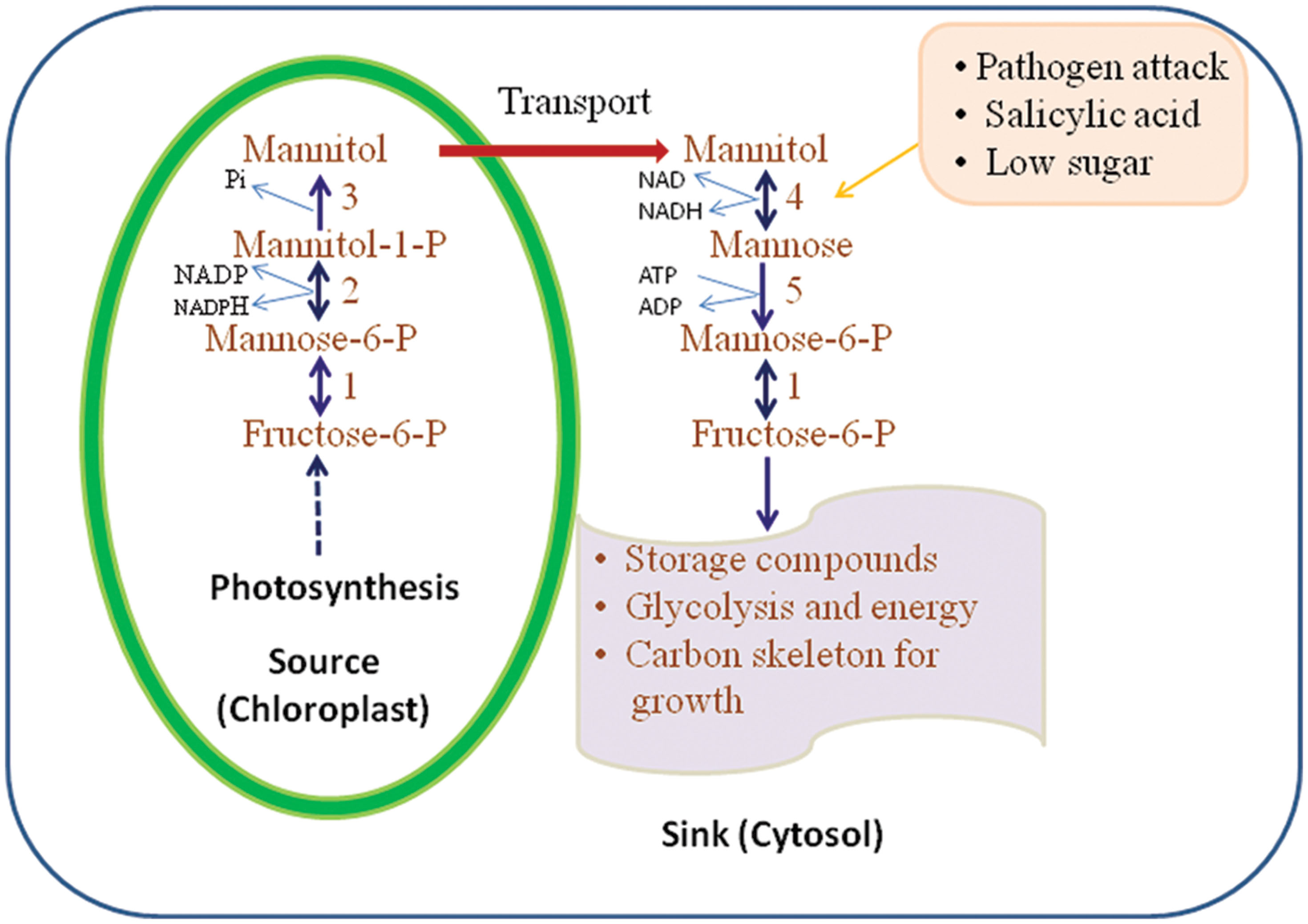
FIGURE 2. Mannitol biosynthesis and catabolism as proposed in higher plants. 1, mannose; 6-P isomerise; 2, mannose-6-P reductase; 3, mannitol-1-phosphate dehydrogenase (M1PDA); 4, mannitol-1-dehydrogenase; 5, hexokinase (HX; adapted and modified from Stoop et al., 1996).
Role of Mannitol and MTD during Plant–Pathogen Interactions
In fungi, mannitol plays a role in metabolism and a role in pathogenesis. In response to pathogen invasion, plants produce ROS in the extracellular space or apoplast for defense. In plants ROS are generated by a plasmalemma-embedded NADPH oxidase and/or a pH-dependent peroxidase (Bolwell and Wojtaszek, 1997). The ROS created in this “oxidative blast” serve different parts in the plant response. They serve as signals for the start of downstream resistance components, including the hypersensitive response (HR) and systemic acquired resistance (SAR). Some pathogens use strategies to circumvent these ROS-mediated defenses by the detoxification of ROS generated by host. It is reported that some plant and animal pathogenic fungi apparently use mannitol to detoxify ROS generated in the host environment.
The animal pathogen C. neoformans secretes large amounts of mannitol during the infection processes, and a mannitol low-producing mutant had reduced pathogenicity and oxidative stress tolerance (Chaturvedi et al., 1996a). The survival rate of C. neoformans was increased on addition of mannitol in vitro under the oxidative stress caused by ROS (Chaturvedi et al., 1996a,b). Virulent races of the tomato fungal pathogen Cladosporium fulvum produce and secrete mannitol during infection, while mutants unable to produce mannitol are non-pathogenic (Joosten et al., 1990). Mannitol secretion during the infection process is also reported in Uromyces fabae (a rust fungus), with mannitol accumulation in the apoplast paralleling high levels of fungal MTD activity (fungal mannitol biosynthesis) in the haustoria (Voegele et al., 2005). Fungal pathogen A. alternata also produces and secretes mannitol (Jennings et al., 1998) when treated with host plant (tobacco) extracts. Vélëz et al. (2008) reported that after treatment with the host extracts, the induction of genes for mannitol biosynthesis takes place and the pathogenicity was reduced in mannitol null mutants, which confirms that mannitol is a pathogenicity factor in fungi.
A key indication that mannitol may have a part in plant–pathogen communications came from the observation that the celery mannitol catabolic catalyst MTD was a pathogen-induced protein in celery (Figure 3; Williamson et al., 1995). Tobacco does not produce mannitol, MTD and corresponding protein and RNA accumulation is induced in fungus infected tobacco (Jennings et al., 1998).
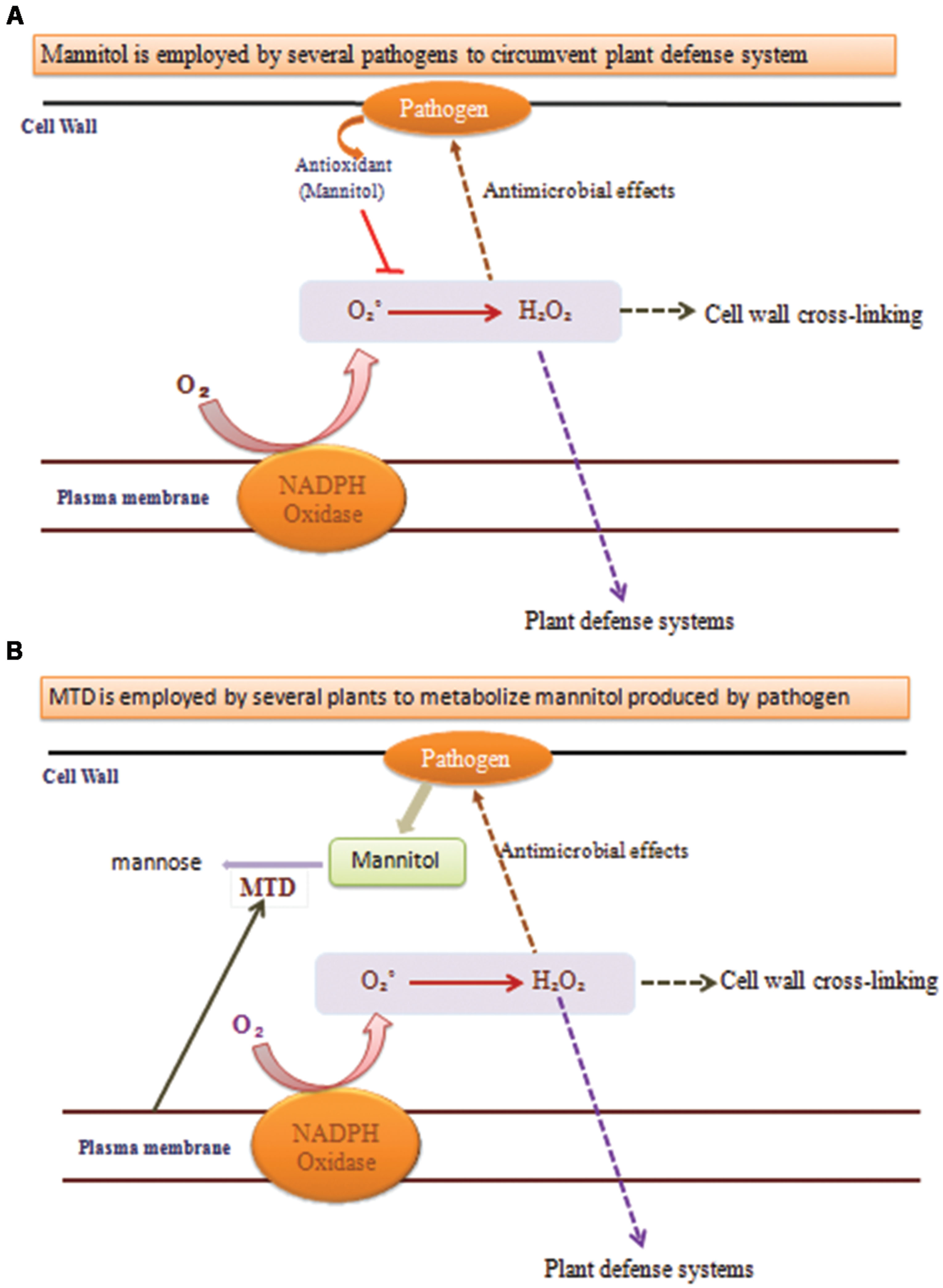
FIGURE 3. Functions of mannitol and mannitol dehydrogenase (MTD) during plant–pathogen interactions. (A) When pathogens uses mannitol to circumvent plant defense system it quenches the ROS production and the processes marked with dotted arrows do not occur, leading to pathogen replication. (B) When plants use MTD to metabolize mannitol produced by pathogens then the processes marked with dotted arrows occur and provide resistance against the pathogen.
Mannitol and MTD assume noteworthy parts in photosynthesis and in salt and oxidative stress resilience. Studies likewise implicate mannitol and MTD in plant–pathogen safeguards. To discover MTD in mannitol-containing plants (e.g., celery, parsley, and snapdragon), three non-mannitol plants (tobacco, tomato, and Arabidopsis) have been found to contain pathogen-incited MTD, where mannitol might act as a signal molecule (Jennings et al., 1999; Chan et al., 2011; Wyatt et al., 2014). Moreover, expression of a celery MTD in tobacco confers resistance to the mannitol secreting fungus Alternaria. Thus, MTD appears to represent a new class of resistance gene with potential for introducing increased fungal resistance in plants (Jennings et al., 1999).
Plants use ROS both as antimicrobial operators and as signal molecules to start differing safeguard reactions. Pathogens have developed numerous modes to sidestep these barriers. Pathogens incorporate mannitol, a ROS quencher, whose creation is fundamental for pathogenicity by such differing types as the tomato pathogen Cladosporium and the human pathogen Cryptococcus. The pathogen mannitol acts in extinguishing ROS and intervening with the formation of host barriers that ought to be more impervious to assault by the plants. MTD assumes a part in host–pathogen cooperations, must be colocalized with pathogen-emitted mannitol. In early stages of plant infection, the separation of infection structures and fungal protection against extracellular ROS created by oxidative burst were related with mannitol aggregation in hyphae and conidia, separately. Amid tissue colonization, albeit the fast change of plant sugars into mannitol in response to hyphae intrusion may not be specifically connected to necrosis, the polyol likely is involved in fungal protection against intracellular isothiocyanate-induced oxidative stress (Calmes et al., 2013).
In plants, polyols (mannitol or sorbitol) play a major role in sugar transport. Polyol/monosaccharide transporters (PMTs) are involved in phloem loading and unloading (Dusotoit-Coucaud et al., 2010). Polyols being a major sugar in the metabolism of some fungi (Solomon et al., 2007), PMTs have also been recommended to assume a part in plant–fungal interactions.
Mannitol Accumulation and Metabolism in Fungi
Fungi aggregate large amounts of polyols interacellulary, up to a several hundred millimoles every litre (Ruijter et al., 2003). Aspergillus niger creates various diverse polyols, including glycerol, erythriol, and D-mannitol (Witteveen and Visser, 1995). The production of the individual polyols in A. niger relies on the growth conditions and developmental stage. It recommends that polyols have important functions in fungal physiology. The hexitol D-mannitol is collected in numerous fungal species (Jennings, 1984). In A. niger conidiospores, D-mannitol is the prevalent carbon-containing compound and makes up 10–15% of the dry weight (Witteveen and Visser, 1995; Ruijter et al., 2003). The high amassings of mannitol in conidia of A. niger (Witteveen and Visser, 1995; Ruijter et al., 2003) and a few other organisms, for example, Aspergillus oryzae (Horikoshi et al., 1965), and Aspergillus clavatus (Corina and Munday, 1971; Ruijter et al., 2003), support a role in the survival of spores. During spore germination in A. niger mannitol is quickly metabolized, suggesting that it assumes a part in the capacity of carbon or reducing power (Witteveen and Visser, 1995; Ruijter et al., 2003). Correspondingly, in the basidiomycete Agaricus bisporus fruiting bodies, mannitol contributes up to half of the dry weight and is accepted to be a fundamental source of carbon that preserves the mushroom after harvest (Hammond and Nichols, 1975; Ruijter et al., 2003).
Mannitol is crucial for the security of spores against cell harm which happens under high temperature, drying, or increasing stress conditions. Mannitol creation is to avoid oxidative harm, for example, mannitol generation within the host by the human pathogen C. neoformans (Chaturvedi et al., 1996b) and secretion of mannitol by the phytopathogenic fungus A. alternata during plant disease (Jennings et al., 1998; Trontin et al., 2014). Interestingly, the plant increases MTD activity in light of parasitic contamination, probably for the evacuation of mannitol to balance the fungal protection mechanism (Jennings et al., 1998). In A. niger, mannitol is included in conidial stress resistance, especially oxidative and high-temperature stresses (Ruijter et al., 2003), and in Stagonospora nodorum the vicinity of mannitol is needed for asexual sporulation (Solomon et al., 2006). Since A. niger is a saprophyte and is pathogenic only in immuno-deficient people, it likely does not utilize mannitol generation for barrier amid development yet rather utilizes mannitol to guarantee maximal resilience of spores to survive unfavorable conditions. Numerous parasitic species aggregate trehalose and/or mannitol in their propagules, despite the fact that the levels differ (Dijksterhuis and Samson, 2002). Case in point, the trehalose level in Aspergillus nidulans spores is high compared to that in A. niger (Fillinger et al., 2001; Ruijter et al., 2003). Evidently, there is a species-particular inclination for mannitol or trehalose accumulation in conidia. In A. nidulans conidia, trehalose is imperative for long term spore survival and spore germination, proposing as a part of capacity carbon (Fillinger et al., 2001). The high mannitol level in A. niger conidia is not needed for the viability of conidiospores amid delayed stockpiling or spore germination, since inactivation of mpdA gene had no antagonistic impacts (Ruijter et al., 2003).
Accumulation of Mannitol in Plants
Abiotic Stress-imposed Mannitol Accumulation in Plants
In higher plants alditols and mannitol are osmolytes and solutes that provide resistance against various abiotic stresses (Hema et al., 2014). In a few plants mannitol and sorbitol collect in response to stress, working as osmolytes or compatible cytoplasmic solutes (Loescher, 1987). Changed tobacco plants encoding a quality mannitol-1-phosphate dehydrogenase (mtlD) brought about mannitol gathering, these changed tobacco plants survived, while non-changed plants were severely injured or killed when presented to 250 mol m-3 NaCl, indicating a function of mannitol in salt resilience (Tarczynski et al., 1993). Askari and Pepoyan (2012) reported that transgenic potato plants transformed with mtlD showed enhanced tolerance against salinity owing to the mannitol production due to mtlD activity. Similarly, a mannitol-synthesizing transgenic peanut plant has been shown to have high level of tolerance against salinity and drought stresses attributing to the abiotic stress mitigating potentials of mannitol (Bhauso et al., 2014).
A positive connection between carbon apportioning into mannitol and salt anxiety was found in celery, which creates mannitol characteristically (Kann et al., 1993). Celery grown in hydroponic nutrient solution with salinity (equal to 30% sea water) showed dry weight gain equal to control plants grown at normal nutrient level. However, fresh weight gains under high salinity were reduced as compared to control. This indicates that water content of plants decreased as salinity increased but the total assimilatory ability was unaffected. Mannitol concentration is progressively increased when total salinity of the growth solution was increased. Mannitol enhances the development of transgenic wheat submerged and saltiness stress both at the callus and entire plant level (Tilahun et al., 2003). In tobacco, these finding are similar to use the mtlD gene (Tarczynski et al., 1992; Karakas et al., 1997) and same as Arabidopsis (Thomas et al., 1995). In transgenic wheat, the measure of mannitol collection was in the low end of the concentrations reported for tobacco and Arabidopsis.
Numerous plant species collect polyols and cyclitols in leaves because of water stress (Noiraud et al., 2000). Mannitol and sorbitol are the most ubiquitous polyols found in plants. Mannitol is synthesised in the cytoplasm from fructose-6-phosphate under drought conditions, and these polyols accumulate up to 80% of the total solutes involved in the osmotic adjustment process of some species, like peach and celery (Lo Bianco et al., 2000). Mannitol protects thiol-regulated enzymes (e.g., Phosphoribulokinase) against hydroxyl radicals, which are abundant during the oxidative stress process associated with water stress (Shen et al., 1997a). Under osmotic stress (salt- and water-stress driven) mannitol accumulation is attributable to a reduction in the catabolism of mannitol in green tissues (Stoop and Mooibroek, 1998). Mannitol production induced by water stress has been widely observed in plant species, e.g., tomatoes (Wang et al., 2000), sugarcane (Cha-um and Kirdmanee, 2008), rice, and sorghum (Cha-um et al., 2009).
In Olea europaea, mannitol works as an antioxidant osmoprotectants against oxidative stress coming about because of salt/dry spell push and even sun oriented irradiance (Melgar et al., 2009; Cimato et al., 2010). Mannitol aggregation has as of late been proposed to ensure salt-treated leaves in full daylight from heat stress incited oxidative stress to a more noteworthy degree than leaves developing under incomplete shading (Cimato et al., 2010; Artur et al., 2011). Production of mannitol is useful for C. neoformans to oppose other environmental stresses like as heat and osmotic stresses and the mutant of C. neoformans performed that at minimum levels of mannitol was more vulnerable to heat and osmotic stress (Al-Fakih, 2014).
Biotic Stress-imposed Mannitol Accumulation in Plants
The polyol mannitol extinguishes reactive oxygen species (ROS) both in vitro and in vivo (Smirnoff and Cumbes, 1989; Chaturvedi et al., 1997; Voegele et al., 2005). Studies have recommended that mannitol may be imperative in pathogenesis to cell reinforcement barriers by both plants and animals (Chaturvedi et al., 1996a,b). Jennings et al. (1998) estimated that pathogens secrete mannitol to extinguish ROS amid contamination of tobacco plants, in light of the fact that tobacco (a non-producer of mannitol) communicates a mannitol-debasing compound (MTD) when tested with parasitic elicitors and inducers of plant safeguard reactions. MTD changes over the pathogen-prompted mannitol to mannose, that is permitting the ROS intervened plant protection reaction compelling against the fungi. Transgenic tobacco plants that constitutively express MTD have increased resistance against A. alternata (Jennings et al., 2002). Mannitol combination happens with either sucrose blend, as in celery or with raffinose saccharide amalgamation, as in olive.
MTD as a Pathogen-response (PR) Protein
Mannitol dehydrogenase is one catalyst in the catabolism of mannitol. Mannitol is not found in all plants, but is a photosynthetic product in over 100 species in a number of diverse families (Loescher and Everard, 2000). The regulation of its primary catabolic enzyme MTD is quite complex, responding to factors including salts and simple sugars (Williamson et al., 2002). MTD has high amino acid sequence similarity to several pathogen-response (PR) proteins of unidentified function, and its expression is regulated by the endogenous PR-proteins inducers salicylic acid (SA) and hydrogen peroxide (H2O2; Williamson et al., 1995; Zamski et al., 2001; Jennings et al., 2002). Fungal pathogens secrete mannitol to quench the ROS that mediate plant defense responses. In response, pathogen-induced MTD in the plant might then catabolize the pathogen-secreted mannitol, thus protecting the plant’s ROS-mediated defenses. MTD shows decreased activity in celery leaves exposed to high salinity, due to reduced amounts of MTD proteins (Stoop et al., 1995) and MTD transcripts (Williamson et al., 1995).
Mannitol is employed by the necrotrophic fungus Botrytis cinerea to overcome ROS toxicity induced during HR in plants thus making it to survive luxuriously on the necrotized dead tissue and lower plant growth and yield. Tomato plants overexpressing celery MTD owing to its PR-protein like activity exhibited enhanced resistance against B. cinerea (Patel et al., 2015). Also the secretion of MTD does not follow the established ER/Golgi pathway as for other PR proteins (Cheng et al., 2009; Cheng and Williamson, 2010).
Roles of Mannitol and Other Polyols
Osmoregulation
Polyol generation is a common feature during the growth of many organisms. In many filamentous fungi and particularly in yeasts, glycerol is the favored osmoprotectant. In the yeast Saccharomyces cerevisiae, glycerol contributes to the osmotic capability of the cell. Change of a S. cerevisiae mutant in glycerol combination with qualities for bacterial MPD and plant sorbitol-6-phosphate dehydrogenase brought about mannitol and sorbitol creation. On the other hand, strains with mutations in mannitol/sorbitol synthesis were more sensitive to salt stress than mutants changed with the gpd1 quality as demonstrated by Shen et al. (1999). They inferred that polyol collection has two capacities, encouraging osmotic alteration and supporting redox control. In A. nidulans, glycerol and erythritol are the major polyols included in osmoregulation. The levels of glycerol, arabinitol, and mannitol were likewise followed in C. fulvum-infected tomato plants under ordinary and prohibitive watering regimens for a time of eight days (Clark et al., 2003). Mannitol and malic corrosive parts in the regulation of diurnal leaf water relations were compared in “Biancolilla” (high-mannitol) and “Cerasuola” (low-mannitol) olive trees. Mannitol was the most abundant polyol distinguished at low osmotic weight, while arabinitol levels amassed at higher osmotic weight, with glycerol having a transitory accumulation (Lo Bianco and Avellone, 2014).
Mannitol is likewise one of the essential osmolytes needed for producing turgor required for ascospore release, and MTD action was seen in both asexual and sexual formative stages (Trail et al., 2002; Min et al., 2010). G. zeae amasses sugar alcohols (glycerol, erythritol, arabitol, and mannitol), especially against matric water stresses (Ramirez et al., 2004). Since matric potential is the significant segment of the aggregate water potential in soil and oat crop buildups, sugar alcohols, for example, mannitol may accumulate in cells cultivated under submerged conditions. Results showed that mannitol supplement in medium instigated the transformation of conidia to CLS in G. zeae, and a few qualities are included in this conidial adjustment. Expanded CLS imperviousness to external stresses may be gained from metabolic changes, including the accumulation of mannitol, glycogen, lipids, and chitin.
Quencher of ROS
Mannitol and likely other sugar alcohols may be utilized to protect against ROS. Mannitol has been indicated to extinguish ROS both in vivo and in vitro (Smirnoff and Cumbes, 1989; Chaturvedi et al., 1997; Hema et al., 2014). ROS assume a significant part in pathogen resistance for both plants and animals. In animals, ROS are created by phagocytic leukocytes (macrophages/neutrophils; Rotrosen and Gallin, 1987), while in plants ROS are delivered by a NADPH oxidase confined in the plasmalamella layer (Grant and Loake, 2000). In plants, ROS have an administrative part in activating plant safeguards (e.g., lignin creation, phytoalexin production, lipid peroxidation, and the touch reaction) and additionally having antimicrobial effects (Baker and Orlandi, 1995). More confirmation that fungi use mannitol, to anticipate oxidative damage can be found in A. alternata, a parasitic pathogen of tobacco (Nicotiana tabacum L.). At the point when A. alternata was grown in culture medium and amended with tobacco extracts, an increment in mannitol levels and discharge was observed (Jennings et al., 1998).
Mannitol, which is made by the tomato pathogen C. fulvum, was found in intercellular liquids of tomato leaves infected with harmful races of C. fulvum. However, no mannitol was discovered if avirulent races were utilized (Joosten et al., 1990). Glucose and fructose from the plant were metabolized to mannitol by the parasite. The mannitol could then be utilized to provide energy during sporulation or could be translocated to the spores specifically (Joosten et al., 1990). Since mannitol is traded or filtered inactively into the apoplast, it could likewise have a part in ROS extinguishing.
Storage Carbohydrate
Mannitol is implied to have a part in fungal systems as a stockpiling sugar (Lewis and Smith, 1967). Ballio et al. (1964) separated glycerol, erythritol, arabitol, mannitol, and trehalose from conidia of Penicillium chrysogenum. Mannitol is likewise concentrated in sclerotia of Sclerotinia sclerotiorum, Claviceps purpurea, Claviceps nigricans, and Sclerotinia curreyana (Cooke, 1969). Mannitol is found in spores of A. oryzae, Myrothecium verrucaria, Neurospora sitophila, Neurospora crassa, A. bisporus, Sterostratum corticoides, Puccinia coronata, Puccinia graminis f. sp. tritici, Erysiphe graminis f. sp. hordei, and A. clavatus (Lewis and Smith, 1967). Mannitol in the conidia of A. oryzae is metabolized at an early stage amid germination (Horikoshi et al., 1965). Horikoshi et al. (1965) concluded that mannitol was being utilized as the carbon hotspot for endogenous breath during the first ventures of germination, which was later managed by glucose.
In mycorrhiza, mannitol serves as sink for the translocation and storage of carbohydrate, which probably is not accessible to the host plant (Lewis and Smith, 1967; Koide et al., 2000; Calmes et al., 2013). This concept has also been applied to interaction between the fungus C. fulvum and tomato (Lycopersicon esculentum L.).
Regulating Cofactors
Hult and Gatenbeck (1978) proposed that the mannitol cycle could be utilized to control the coenzymes NADH and NADP+, that is, an approach to produce NADPH to the detriment of NADH and ATP with every turn of the cycle. However, studies with the parasite A. nidulans, gave no support to the operation of the mannitol cycle or for NADPH generation in this fungus (Singh et al., 1988). As indicated by Singh et al. (1988) A. nidulans cultivated on NO3 as a nitrogen source would build the interest for NADPH, and accordingly create an increment in the maximal particular exercises of the enzyme in the mannitol cycle.
MTDs of the several bacterial and fungal pathogens are mannitol 2-oxidoreductases which produce fructose in the forward reaction of mannitol cycle by using either NAD+ (EC 1.1.1.67) or NADP+ (EC 1.1.1.138) as a cofactor (Voegele et al., 2005). In Basidiomycetes (Hult et al., 1980), mannitol-1-P dehydrogenase seems to be absent, so mannitol is possibly formed by direct reduction of fructose through a MTD (EC 1.1.1.67 or EC 1.1.1.138). NAD+-dependent MTD activity is reported in P. graminis f. sp. tritici axenic cultures (Maclean, 1990), and Clancy and Coffey (1980) have also shown NAD+- and NADP+- dependent MTD activity in Melampsora lini axenic cultures and uredospores (Voegele et al., 2005).
Regulation of pH
Jennings (1984) suggested that polyols could be utilized by fungus to control their pH. Polyols could achieve this by serving as sinks or hotspots for protons, as the polyols are made (decreased) or oxidized into different starches. Ribitol accumulation occurred after mannitol consumption and during unsaturated fatty-acid degradation when a hydrogen-acceptor may be needed. At the point when the salt stress is discharged, the convergance of Cl- in vivo diminishes (Mostaert et al., 1995), and that of fructose 6-P would be expanded by the mannitol-catabolizing pathway with MTD and HX (Karsten et al., 1997a,b; Iwamoto et al., 2003). Regulation of mannitol turn over may allow the quick reaction to a change in the natural salt situation. Since fructose 6-P is metabolically at a limb point along the mannitol pathway including glycolysis and reductive and oxidative pentose phosphate cycles, the biosynthesis of mannitol by means of M1P could likewise be controlled by the supply of F6P (Iwamoto et al., 2003). F6P could hence work as a key control component for mannitol metabolism, in addition to the regulation of M1PDH action by NaCl.
Morphogenesis and Conidiation
Mannitol is needed for vegetative sporulation in S. nodorum, both in planta and in vitro (Solomon et al., 2006, 2007). Utilizing mutant strains that are not able to metabolize mannitol, the study found that subculturing of these strains on media without the polyol brought about the suspension of asexual sporulation.
The requirement of mannitol for completion of the life cycle of ascomycetes and morphogenesis in basidiomycetes is also thought to be dependent on the metabolism of mannitol, as indicated by an increase in both MTD and fructose 6-phosphate dehydrogenase activity during fruiting body development in A. bisporus (Stoop and Mooibroek, 1998). Mannitol is also accumulated in the unsporulated oocysts in the avian parasite Eimeria tenella. Mannitol enables sporulation of the oocysts outside of the host by functioning as the endogenous carbon and energy source (Allocco et al., 1999). Mannitol has been shown to be a vital factor against heat and oxidative stress in Neosartorya fischeri for ascospore development as well as stress resistance of conidia (Wyatt et al., 2014).
Future Prospects
It is clear that mannitol can play an important role in plant growth and responses to diverse biotic and abiotic stresses. Possible future of mannitol for osmoprotection, efficient growth and resistance to pathogens, the engineering of plants with mannitol metabolism is a desirable goal. There is a discriminating issue, as mannitol use in sink tissues is spatially divided from its union in experienced photosynthetic tissues. There is little knowledge about the cellular and sub-cellular location of MTD or the control of MTD expression in response to environmental and metabolic factors. It is crucial to study the mannitol transport, enzyme localization and transcriptional and post-transcriptional regulation of MTD expression. Mannitol and the regulation of its production and degradation study in plants, animals and fungi is a vast topic for future research.
Conflict of Interest Statement
The authors declare that the research was conducted in the absence of any commercial or financial relationships that could be construed as a potential conflict of interest.
Acknowledgments
The work was supported in part by a Rajiv Gandhi National Fellowship granted by the University Grant Commission, New Delhi, India, to MM. The authors are grateful to Dr. Alexander Idnurm, School of Biosciences University of Melbourne, Australia for his valuable suggestions and English corrections.
References
Al-Fakih, A. A. (2014). Overview on the fungal metabolites involved in mycopathy. Open J. Med. Microbiol. 4, 38–63. doi: 10.4236/ojmm.2014.41006
Allocco, J. J., Profous-Juchelka, H., Myers, R. W., Nare, B., and Schmatz, D. M. (1999). Biosynthesis and catabolism of mannitol is developmentally regulated in the protozoan parasite Eimeria tenella. J. Parasitol. 85, 167–173. doi: 10.2307/3285614
Artur, C., Paulo, S., Alice, A., Carlos, C., and Hernani, G. (2011). Mannitol transport and mannitol dehydrogenase activities are coordinated in Olea europaea under salt and osmotic stresses. Plant Cell Physiol. 52, 1766–1775. doi: 10.1093/pcp/pcr121
Askari, A., and Pepoyan, A. (2012). Overexpression of mtlD gene in potato (Solanum tuberosum L.), cv. Arinda improves salt tolerance. Adv. Environ. Biol. 6, 2646–2653.
Baker, C. J., and Orlandi, E. W. (1995). Active oxygen in plant pathogenesis. Annu. Rev. Phytopathol. 33, 299–321. doi: 10.1146/annurev.py.33.090195.001503
Ballio, A., Di Vittorio, V., and Russi, S. (1964). The isolation of trehalose and polyols from the conidia of Penicillium chrysogenium Thom. Arch. Biochem. Biophys. 107, 177–183. doi: 10.1016/0003-9861(64)90319-4
Barker, S. A. (1955). “Acyclic sugar alcohols,” in Modern Methods of Plant Analysis, Vol. 2, eds K. Peach and M. V. Tracey (Berlin: Springer-Verlag), 55–63.
Bhauso, T. D., Radhakrishnan, T., Kumar, A., Mishra, G. P., Dobaria, J. R., Patel, K. K., et al. (2014). Overexpression of bacterial mtlD gene in peanut improves drought tolerance through accumulation of mannitol. ScientificWorldJournal 2014, 125967. doi: 10.1155/2014/125967
Bieleski, R. L. (1982). “Sugar alcohols,” in Encyclopedia of Plant Physiology, Vol. 13A, eds F. A. Loewus and W. Tanner (New York, NY: Springer-Verlag), 158–192.
Bolwell, G. P., and Wojtaszek, P. (1997). Mechanisms for the generation of reactive oxygen species in plant defense– a broad perspective. Physiol. Mol. Plant Pathol. 51, 347–366. doi: 10.1006/pmpp.1997.0129
Bourne, E. J. (1958). “The polyhydric alcohols. Acyclic polyhydric alcohols,” in Encyclopedia of Plant Physiology: Formation, Storage, Mobilization, and Transformation of Carbohydrates, Vol. 6, ed. W. Ruhland (Berlin: Springer Verlag), 345–361.
Brown, A. D., and Simpson, J. R. (1972). Water relations of sugar-tolerant yeasts: the role of intracellular polyols. J. Gen. Microbiol. 72, 589–591. doi: 10.1099/00221287-72-3-589
Calmes, B., Guillemette, T., Teyssier, L., Siegler, B., Pigné, S., Landreau, A., et al. (2013). Role of mannitol metabolism in the pathogenicity of the necrotrophic fungus Alternaria brassicicola. Front. Plant Sci. 4:131. doi: 10.3389/fpls.2013.00131
Chan, Z., Grumet, R., and Loescher, W. (2011). Global gene expression analysis of transgenic, mannitol-producing, and salt-tolerant Arabidopsis thaliana indicates widespread changes in abiotic and biotic stress-related genes. J. Exp. Bot. 62, 4787–4803. doi: 10.1093/jxb/err130
Chaturvedi, V., Bartiss, A., and Wong, B. (1997). Expression of bacterial mtlD in Saccharomyces cerevisiae results in mannitol synthesis and protects a glycerol-defective mutant from high-salt and oxidative stress. J. Bacteriol. 179, 157–162.
Chaturvedi, V., Flynn, T., Niehaus, W. G., and Wong, B. (1996a). Stress tolerance and pathogenic potential of a mannitol mutant of Cryptococcus neoformans. Microbiology 142, 937–943. doi: 10.1099/00221287-142-4-937
Chaturvedi, V., Wong, B., and Newman, S. L. (1996b). Oxidative killing of Cryptococcus neoformans by human neutrophils: evidence that fungal mannitol protects by scavenging reactive oxygen intermediates. J. Immunol. 156, 3836–3840.
Cha-um, S., and Kirdmanee, C. (2008). Effect of osmotic stress on proline accumulation, photosynthetic abilities and growth of sugarcane plantlets (Saccharum officinarum L.). Pak. J. Bot. 40, 2541–2552.
Cha-um, S., Thadavong, S., and Kirdmanee, C. (2009). Effect of mannitol-induced osmotic stress on proline accumulation, pigment degradation, photosynthetic abilities and growth characters in C3 rice and C4 sorghum. Front. Agric. China 3:266–273. doi: 10.1007/s11703-009-0063-5
Cheng, F.-Y., and Williamson, J. D. (2010). Is there leaderless protein secretion in plants? Plant Signal. Behav. 5, 129–131. doi: 10.4161/psb.5.2.10304
Cheng, F.-Y., Zamski, E., Guo, W.-W., Pharr, D. M., and Williamson, J. D. (2009). Salicylic acid stimulates secretion of the normally symplastic enzyme mannitol dehydrogenase (MTD): a possible defense against mannitol secreting fungal pathogens. Planta 230, 1093–1103. doi: 10.1007/s00425-009-1006-3
Cimato, A., Castelli, S., Tattini, M., and Traversi, M. L. (2010). An ecophysiological analysis of salinity tolerance in olive. Environ. Exp. Bot. 68, 214–221. doi: 10.1016/j.envexpbot.2009.12.006
Clancy, F. G., and Coffey, M. D. (1980). Polyol dehydrogenases in the rust fungus, Melampsora lini (Ehrenb.). Lev. J. Gen. Microbiol. 120, 85–88.
Clark, A. J., Blissett, K. J., and Oliver, R. P. (2003). Investigating the role of polyols in Cladosporium fulvum during growth under hyper-osmotic stress and in planta. Planta 216, 614–619.
Cooke, R. C. (1969). Changes in soluble carbohydrates during sclerotium formation by Sclerotinia sclerotiorum and Sclerotinia trifoliorum. Trans. Brit. Mycol. Soc. 58, 77–86. doi: 10.1016/S0007-1536(69)80009-4
Corina, D. L., and Munday, K. A. (1971). Studies on polyol function in Aspergillus clavatus: a role for mannitol and ribitol. J. Gen. Microbiol. 69, 221–227. doi: 10.1099/00221287-69-2-221
Davis, J. M., and Loescher, W. H. (1990). [14C]-Assimilate translocation in the light and dark in celery (Apium graveolens) leaves of different ages. Plant Physiol. 79, 656–662. doi: 10.1111/j.1399-3054.1990.tb00040.x
Dijkema, C., Kester, H. C. M., and Visser, J. (1985). 13C NMR studies of carbon metabolism in the hyphal fungus Aspergillus nidulans. Proc. Natl. Acad. Sci. U.S.A. 82, 14–18. doi: 10.1073/pnas.82.1.14
Dijksterhuis, J., and Samson, R. A. (2002). “Food and crop spoilage on storage,” in The Mycota: Agricultural Applications, Vol. XI, ed. F. Kempken (Berlin: Springer-Verlag KG), 39–52.
Dulermo, T., Rascle, C., Chinnici, G., Gout, E., Bligny, R., and Cotton, P. (2009). Dynamic carbon transfer during pathogenesis of sunflower by the necrotrophic fungus Botrytis cinerea: from plant hexoses to mannitol. New Phytol. 183, 1149–1162. doi: 10.1111/j.1469-8137.2009.02890.x
Dusotoit-Coucaud, A., Porcheron, B., Brunel, N., Kongsawadworakul, P., Franchel, J., Viboonjun, U., et al. (2010). Cloning and characterization of a new polyol transporter (HbPLT2) in Hevea brasiliensis. Plant Cell Physiol. 51, 1878–1888. doi: 10.1093/pcp/pcq151
Everard, J. D., Cantini, C., Grumet, R., Plummer, J., and Loescher, W. H. (1997). Molecular cloning of mannose 6-phosphate reductase and its developmental expression in celery. Plant Physiol. 113, 1427–1435. doi: 10.1104/pp.113.4.1427
Everard, J. D., Franceschi, V. R., and Loescher, W. H. (1993). Mannose-6-phosphate reductase, a key enzyme in photoassimilate partitioning, is abundant and located in the cytosol of photosynthetically active cells of celery (Apium graveolens L.) source leaves. Plant Physiol. 102, 345–356.
Fillinger, S., Chaveroche, M. K., Van Dijck, P., De Vries, R., Ruijter, G., Thevelein, J., et al. (2001). Trehalose is required for the acquisition of tolerance to a variety of stresses in the filamentous fungus Aspergillus nidulans. Microbiology 147, 1851–1862. doi: 10.1099/00221287-147-7-1851
Grant, J. J., and Loake, G. J. (2000). Role of reactive oxygen intermediates and cognate redox signaling in disease resistance. Plant Physiol. 124, 21–29. doi: 10.1104/pp.124.1.21
Hammond, J. B. W., and Nichols, R. (1975). Changes in respiration and soluble carbohydrates during the post-harvest storage of mushrooms (Agaricus bisporus). J. Sci. Food Agric. 26, 835–842. doi: 10.1002/jsfa.2740260615
Hellebust, J. A. (1976). Osmoregulation. Annu. Rev. Plant Physiol. 27, 485–505. doi: 10.1146/annurev.pp.27.060176.002413
Hema, R., Vemanna, R. S., Sreeramulu, S., Reddy, C. P., Senthil-Kumar, M., and Udayakumar, M. (2014). Stable expression of mtlD gene imparts multiple stress tolerance in Finger millet. PLoS ONE 9:e99110. doi: 10.1371/journal.pone.0099110
Horer, S., Stoop, J., Mooibroek, H., Baumann, U., and Sassoon, J. (2001). The crystallographic structure of the mannitol 2-dehydrogenase NADP+ binary complex from Agaricus bisporus. J. Biol. Chem. 276, 27555–27561. doi: 10.1074/jbc.M102850200
Horikoshi, K., Iida, S., and Ikeda, Y. (1965). Mannitol and mannitol dehydrogenase in conidia of Aspergillus oryzae. J. Bacteriol. 89, 326–330.
Hult, K., and Gatenbeck, S. (1978). Production of NADPH in the mannitol cycle and its relation to polyketide formation in Alternaria alternata. Eur. J. Biochem. 88, 607–612. doi: 10.1111/j.1432-1033.1978.tb12487.x
Hult, K., Veide, A., and Gatenbeck, S. (1980). The distribution of the NADPH regenerating mannitol cycle among fungal species. Arch. Microbiol. 128, 253–255. doi: 10.1007/BF00406168
Iwamoto, K., Kawanobe, H., Ikawa, T., and Shiraiwa, Y. (2003). Characterization of salt-regulated mannitol-1-phosphate dehydrogenase in the red alga Caloglossa continua. Plant Physiol. 133, 893–900. doi: 10.1104/pp.103.026906
Jennings, D. B., Daub, M. E., Pharr, D. M., and Williamson, J. D. (2002). Constitutive expression of a celery mannitol dehydrogenase in tobacco enhances resistance to the mannitol-secreting fungal pathogen Alternaria alternata. J. Plant 32, 41–49. doi: 10.1046/j.1365-313X.2001.01399.x
Jennings, D. B., Ehrenshaft, M., Pharr, D. M., and Williamson, J. D. (1998). Roles for mannitol and mannitol dehydrogenase in active oxygen-mediated plant defense. Proc. Natl. Acad. Sci. U.S.A. 95, 15129–15133. doi: 10.1073/pnas.95.25.15129
Jennings, D. B., Paul, L. C., Daub, M. E., Pharr, D. M., and Williamson, J. D. (1999). Analyses of mannitol dehydrogenases from mannitol- vs. non-mannitol-producing plants: implications of plant–pathogen interactions. Plant Physiol. Abstract 632.
Jennings, D. H. (1984). Polyol metabolism in fungi. Adv. Microb. Physiol. 25, 149–193. doi: 10.1016/S0065-2911(08)60292-1
Joosten, M. H. A. J., Hendrickx, L. J. M., and De Witt, P. G. J. M. (1990). Carbohydrate composition of apoplastic fluids isolated from tomato leaves inoculated with virulent or a virulent races of Cladosporium fulvum (syn. Fulvia fulva). Neth. J. Pl. Path. 96, 103–112. doi: 10.1007/BF02005134
Kann, S. C., Everard, J. D., and Loescher, W. H. (1993). Growth, salt tolerance, and mannitol accumulation in celery. HortScience 28, 486–486.
Karakas, B., Ozias-Akins, P., Stushnoff, C., Suefferheld, M., and Rieger, M. (1997). Salinity and drought tolerance in mannitol-accumulating transgenic tobacco. Plant Cell Environ. 20, 609–616. doi: 10.1111/j.1365-3040.1997.00132.x
Karsten, U., Barrow, K. D., West, J. A., and King, R. J. (1997a). Mannitol metabolism in the intertidal mangrove red alga Caloglossa leprieurii: salinity effects on enzymatic activity. Phycologia 36, 150–156. doi: 10.2216/i0031-8884-36-2-150.1
Karsten, U., Barrow, K. D., Nixdorf, O., West, J. A., and King, R. J. (1997b). Characterization of mannitol metabolism in the mangrove red alga Caloglossa leprieurii (Montagne) J.Agardh. Planta 201, 173–178. doi: 10.1007/BF01007701
Koide, R. T., Shumway, D. L., and Stevens, C. M. (2000). Soluble carbohydrates of red pine (Pinus resinosa) mycorrhizas and mycorrhizal fungi. Mycol. Res. 104, 834–840. doi: 10.1017/S0953756299002166
Lewis, D. H. (1984). “Physiology and metabolism of alditols,” in Storage Carbohydrates in Vascular Plants, ed. D. H. Lewis (Cambridge: Cambridge University Press), 157–179.
Lewis, D. H., and Smith, D. C. (1967). Sugar alcohols (polyols) in fungi and green plants. I. Distribution, physiology and metabolism. New Phytol. 66, 143–184. doi: 10.1111/j.1469-8137.1967.tb05997.x
Lo Bianco, R., and Avellone, G. (2014). Diurnal regulation of leaf water status in high- and low-mannitol olive cultivars. Plants 3, 196–208.
Lo Bianco, R., Rieger, M., and Sung, S. J. S. (2000). Effect of drought on sorbitol and sucrose metabolism in sinks and sources of peach. Physiol. Plant. 108, 71–78. doi: 10.1034/j.1399-3054.2000.108001071.x
Loescher, W. H. (1987). Physiology and metabolism of sugar alcohols in higher plants. Plant Physiol. 70, 553–557. doi: 10.1111/j.1399-3054.1987.tb02857.x
Loescher, W. H., and Everard, J. D. (2000). “Regulation of sugar alcohol biosynthesis,” in Photosynthesis: Physiology and Metabolism, eds R. C. Leegood, T. D. Sharkey, and S. Von Caemmerer (Dordrecht: Kluwer Academic Publishers), 275–299.
Loescher, W. H., Everard, J. D., Cantini, C., and Grumet, R. (1995). “Sugar, alcohol metabolism in source leaves,” in Carbon Partitioning and Source-Sink Interactions in Plants: Current Topics in Plant Physiology, Vol. 13, eds M. A. Madore and W. J. Lucas (Rockville, MD: American Society of Plant Physiologists), 170–179.
Loescher, W. H., Tyson, R. H., Everard, J. D., Redgwell, R. J., and Bieleski, R. L. (1992). Mannitol synthesis in higher plants. Evidence for the role and characterization of a NADPH-dependent mannose-6-phosphate reductase. Plant Physiol. 98, 1396–1402. doi: 10.1104/pp.98.4.1396
Maclean, D. J. (1990). Polyol dehydrogenases in axenic mycelia of the wheat stem rust fungus Puccinia graminis f. sp. tritici. J. Gen. Microbiol. 136, 2275–2281. doi: 10.1099/00221287-136-11-2275
Melgar, J. C., Guidi, L., Remorini, D., Agati, G., Degl Innocenti, E., Castelli, S., et al. (2009). Antioxidant defenses and oxidative damage in salt-treated olive plants under contrasting sunlight irradiance. Tree Physiol. 29, 1187–1198. doi: 10.1093/treephys/tpp047
Min, K., Lee, J., Kim, J. C., Kim, S. G., Kim, Y. H., Vogel, S., et al. (2010). A novel gene, ROA, is required for proper morphogenesis and discharge of ascospores in Gibberella zeae. Eukaryot. Cell 9, 1495–1503. doi: 10.1128/EC.00083-10
Mostaert, A. S., Karsten, U., and King, R. J. (1995). Inorganic ions and mannitol in the red alga Caloglossa leprieurii (Ceramiales, Rhodophyta): response to salinity change. Phycologia 34, 501–507. doi: 10.2216/i0031-8884-34-6-501.1
Noiraud, N., Delrot, S., and Lemoine, R. (2000). The sucrose transporter of celery: identification and expression during salt stress. Plant Physiol. 122, 1447–1456. doi: 10.1104/pp.122.4.1447
Nuccio, M. L., Rhodes, D., McNeil, S. D., and Hanson, A. D. (1999). Metabolic engineering of plants for osmotic stress resistanc. Curr. Opin. Plant Biol. 2, 128–134. doi: 10.1016/S1369-5266(99)80026-0
Obaton, M. F. (1929). Evolution della mannite (mannitol) chez les vegetaux. Rev. Gen. Bot. 41, 622–633.
Patel, T. K., Krasnyanski, S. F., Allen, G. C., Louws, F. J., Panthee, D. R., and Williamson, J. D. (2015). Tomato plants overexpressing a celery mannitol dehydrogenase (MTD) have decreased susceptibility to Botrytis cinerea. Am. J. Plant Sci. 6, 1116–1125. doi: 10.4236/ajps.2015.68116
Patkowska, E., and Konopinski, M. (2008). Pathogenicity of selected soil-borne microorganisms for scorzonera seedlings (Scorzonera hispanica L.). Folia Hortic. 20, 31–42.
Pedersen, P., Charlotte, H. G., Steven, J. W., and Soren, R. J. (2007). Chemical markers in Veronica sect. Hebe. II. Biochem. Syst. Ecol. 35, 614–620. doi: 10.1016/j.bse.2007.04.010
Pharr, D. M., Stoop, J. M. H., Studer-Feusi, M. E., Williamson, J. D., Massel, M. O., and Conkling, M. A. (1995a). “Mannitol catabolism in plant sink tissues,” in Carbon Partitioning and Source-Sink Interactions in Plants, Current Topics in Plant Physiology, Vol. 13, eds M. A. Madore and W. J. Lucas (Rockville, MD: American Society of Plant Physiologists), 180–194.
Pharr, D. M., Stoop, J. M. H., Williamson, J. D., Studer-Feusi, M. E., Massel, M. O., and Conkling, M. A. (1995b). The dual role of mannitol as osmoprotectant and photoassimilate in celery. J. Am. Soc. Hortic. Sci. 30, 1182–1188.
Pharr, M. D., and Stoop, M. H. (1993). Mannitol Oxidoreductase Isolated from Vascular Plants. U.S. Patent No. 5268288. Washington, DC: United States patent and trademark office.
Ramirez, M. L., Chulze, S. N., and Magan, N. (2004). Impact of osmotic and matric water stress on germination, growth, mycelial water potentials and endogenous accumulation of sugars and sugar alcohols in Fusarium graminearum. Mycologia 96, 470–478. doi: 10.2307/3762167
Reyneri, A. (2006). The role of climatic condition on micotoxin production in cereal. Vet. Res. Commun. 30, 87–92. doi: 10.1007/s11259-006-0018-8
Rotrosen, D., and Gallin, J. I. (1987). Disorders of phagocyte function. Annu. Rev. Immunol. 5, 127–150. doi: 10.1146/annurev.iy.05.040187.001015
Ruijter, G. J. G., Bax, M., Patel, H., Flitter, S. J., Van de Vondervoort, P. J. I., De Vries, R. P., et al. (2003). Mannitol is required for stress tolerance in Aspergillus niger conidiospores. Eukaryot. Cell 2, 690–698. doi: 10.1128/EC.2.4.690-698.2003
Rumpho, M. E., Edwards, G. E., and Loescher, W. H. (1983). A pathway for photosynthetic carbon flow to mannitol in celery leaves: activity and localization of key enzymes. Plant Physiol. 73, 869–873. doi: 10.1104/pp.73.4.869
Salmon, S., Lemoine, R., Jamai, A., Bouche-Pilon, S., and Fromont, J. C. (1995). Study of sucrose and mannitol transport in plasma-membrane vesicles from phloem and non-phloem tissues of celery (Apium graveolens L.) petioles. Planta 197, 76–83. doi: 10.1007/BF00239942
Shen, B., Hohmann, S., Jensen, R. G., and Bohnert, H. J. (1999). Roles of sugar alcohols in osmotic stress adaptation, Replacement of glycerol by mannitol and sorbitol in yeast. Plant Physiol. 121, 45–52. doi: 10.1104/pp.121.1.45
Shen, B., Jensen, R. G., and Bohnert, H. J. (1997a). Increased resistance to oxidative stress in transgenic plants by targeting mannitol biosynthesis to the chloroplast. Plant Physiol. 113, 1177–1183. doi: 10.1104/pp.113.4.1177
Shen, B., Jensen, R. G., and Bohnert, H. J. (1997b). Mannitol protects against oxidation by hydroxyl radicals. Plant Physiol. 115, 527–532.
Singh, M., Scrutton, M. S., and Scrutton, M. C. (1988). NADPH generation in Aspergillus nidulans: is the mannitol cycle involved? J. Gen. Microbiol. 134, 643–654.
Smirnoff, N., and Cumbes, Q. (1989). Hydroxyl radical scavenging activity of compatible solutes. Phytochemistry 28, 1057–1060. doi: 10.1016/0031-9422(89)80182-7
Solomon, P. S., Waters, O. D., Jorgens, C. I., Lowe, R. G., Rechberger, J., Trengove, R. D., et al. (2006). Mannitol is required for asexual sporulation in the wheat pathogen Stagonospora nodorum (glume blotch). J. Biochem. 399, 231–239. doi: 10.1042/BJ20060891
Solomon, P. S., Waters, O. D. C., and Oliver, R. P. (2007). Decoding the mannitol enigma in filamentous fungi. Trends Microbiol. 15, 257–262. doi: 10.1016/j.tim.2007.04.002
Son, H., Lee, J., and Lee, Y. W. (2012). Mannitol induces the conversion of conidia to chlamydospore-like structures that confer enhanced tolerance to heat, drought, and UV in Gibberella zeae. Microbiol. Res. 167, 608–615. doi: 10.1016/j.micres.2012.04.001
Stacey, B. E. (1974). “Plant polyols,” in Plant Carbohydrate Biochemistry, ed. J. B. Pridham (New York, NY: Academic Press), 47–59.
Stoop, J. M., and Mooibroek, H. (1998). Cloning and characterization of NADP-mannitol dehydrogenase cDNA from the button mushroom, Agaricus bisporus, and its expression in response to NaCl stress. Appl. Environ. Microbiol. 64, 4689–4696.
Stoop, J. M. H., and Pharr, D. M. (1992). Partial purification and characterization of mannitol: mannose 1-oxidoreductase from celeriac (Apium graveolens var. rapaceum) roots. Arch. Biochem. Biophys. 298, 612–619. doi: 10.1016/0003-9861(92)90456-7
Stoop, J. M. H., and Pharr, D. M. (1994). Mannitol metabolism in celery stressed by excess macronutrients. Plant Physiol. 106, 503–511.
Stoop, J. M. H., Williamson, J. D., Conkling, M. A., and Pharr, D. M. (1995). Purification of NAD-dependent mannitol dehydrogenase from celery suspension cultures. Plant Physiol. 108, 1219–1225. doi: 10.1104/pp.108.3.1219
Stoop, J. M. H., Williamson, J. D., and Pharr, D. M. (1996). Mannitol metabolism in plants: a method for coping with stress. Trends Plant Sci. 1, 139–144. doi: 10.1016/S1360-1385(96)80048-3
Tarczynski, M. C., Jensen, R. G., and Bohnert, H. J. (1992). Expression of a bacterial mtlD gene in transgenic tobacco leads to production and accumulation of mannitol. Proc. Natl. Acad. Sci. U.S.A. 89, 2600–2604. doi: 10.1073/pnas.89.7.2600
Tarczynski, M. C., Jensen, R. G., and Bohnert, H. J. (1993). Stress protection of transgenic tobacco by production of the osmolyte mannitol. Science 259, 508–510. doi: 10.1126/science.259.5094.508
Thomas, J. C., Sepahi, M., Arendall, B., and Bohnert, H. J. (1995). Enhancement of seed germination in high salinity by engineering mannitol expression in Arabidopsis thaliana. Plant Cell Environ. 18, 801–806. doi: 10.1111/j.1365-3040.1995.tb00584.x
Tilahun, A., Arron, C. G., Bjorn, M., and John, C. C. (2003). Tolerance of mannitol-accumulating transgenic wheat to water stress and salinity. Plant Physiol. 131, 1748–1755. doi: 10.1104/pp.102.003616
Trail, F., and Xu, H. (2002). Purification and characterization of mannitol dehydrogenase and identification of the corresponding cDNA from the head blight fungus, Gibberella zeae (Fusarium graminearum). Phytochemistry 61, 791–796. doi: 10.1016/S0031-9422(02)00430-2
Trail, F., Xu, H., Loranger, R., and Gadoury, D. (2002). Physiological and environmental aspects of ascospore discharge in Gibberella zeae (anamorph Fusarium graminearum). Mycologia 94, 181–189. doi: 10.2307/3761794
Trip, P., Nelson, C. D., and Krotkov, G. (1965). Selective and preferential translocation of 14C-labeled sugars in white ash and lilac. Plant Physiol. 40, 470–477. doi: 10.1104/pp.40.4.740
Trontin, C., Kianis, S., Corwin, J. A., Hématy, K., Yansouni, J., Kliebenstein, D. J., et al. (2014). A pair of receptor-like kinases is responsible for natural variation in shoot growth response to mannitol treatment in Arabidopsis thaliana. Plant J. 78, 121–133. doi: 10.1111/tpj.12454
Vélëz, H., Glassbrook, N. J., and Daub, M. E. (2007). Mannitol metabolism in the phytopathogenic fungus Alternaria alternata. Fungal Genet. Biol. 44, 258–268. doi: 10.1016/j.fgb.2006.09.008
Vélëz, H., Glassbrook, N. J., and Daub, M. E. (2008). Mannitol biosynthesis is required for plant pathogenicity by Alternaria alternata. FEMS Microbiol. Lett. 285, 122–129. doi: 10.1111/j.1574-6968.2008.01224.x
Voegele, R. T., Hahn, M., Lohaus, G., Link, T., Heiser, I., and Mendgen, K. (2005). Possible roles for mannitol and mannitol dehydrogenase in the biotrophic plant pathogen Uromyces fabae1. Plant Physiol. 137, 190–198. doi: 10.1104/pp.104.051839
Wang, H. Z., Huang, D. N., Lu, R. F., Liu, J. J., Qian, Q., and Peng, X. X. (2000). Salt tolerance of transgenic rice (Oryza sativa L.) with mtlD gene and gutD gene. Chin. Sci. Bull. 45, 1685–1690. doi: 10.1007/BF02898987
Williamson, J. D., Desai, A., Krasnyanski, S. F., Ding, F., Guo, W., Nguyen, T., et al. (2013). Overexpression of mannitol dehydrogenase in zonal geranium confers increased resistance to the mannitol secreting fungal pathogen Botrytis cinerea. Plant Cell Tiss. Organ Cult. 115, 367–375. doi: 10.1007/s11240-013-0368-1
Williamson, J. D., Jennings, D. B., Guo, W. W., Pharr, D. M., and Ehrenshaft, M. (2002). Sugar alcohols, salt stress, and fungal resistance: polyols – multifunctional plant protection? J. Am. Soc. Hortc. Sci. 127, 467–473.
Williamson, J. D., Stoop, J. M. H., Massel, M. O., Conkling, M. A., and Pharr, D. M. (1995). Sequence analysis of a mannitol dehydrogenase cDNA from plants reveals a function for the pathogenesis related protein ELI3. Proc. Natl. Acad. Sci. U.S.A. 92, 7148–7152. doi: 10.1073/pnas.92.16.7148
Witteveen, C. F. B., and Visser, J. (1995). Polyol pools in Aspergillus niger. FEMS Microbiol. Lett. 134, 57–62. doi: 10.1111/j.1574-6968.1995.tb07914.x
Wyatt, T. T., Van Leeuwen, M. R., Wösten, H. A. B., and Dijksterhuis, J. (2014). Mannitol is essential for the development of stress-resistant ascospores in Neosartorya fischeri (Aspergillus fischeri). Fungal Genet. Biol. 64, 11–24. doi: 10.1016/j.fgb.2013.12.010
Yancey, P. H., Clark, M. E., Hand, S. C., Bowles, R. D., and Somero, G. N. (1982). Living with water stress: evolution of osmolyte systems. Science 217, 1214–1222. doi: 10.1126/science.7112124
Zamski, E., Guo, W. W., Yamamoto, Y. T., Pharr, D. M., and Williamson, J. D. (2001). Analysis of celery (Apium graveolens) mannitol dehydrogenase (Mtd) promoter regulation in Arabidopsis suggests roles for MTD in key environmental and metabolic responses. Plant Mol. Biol. 47, 621–631. doi: 10.1023/A:1012395121920
Keywords: Mannitol, reactive oxygen species (ROS), mannitol dehydrogenase (MTD), mannitol-1-phosphate-5-dehydrogenase (MPD), polyols
Citation: Meena M, Prasad V, Zehra A, Gupta VK and Upadhyay RS (2015) Mannitol metabolism during pathogenic fungal–host interactions under stressed conditions. Front. Microbiol. 6:1019. doi: 10.3389/fmicb.2015.01019
Received: 06 August 2015; Accepted: 08 September 2015;
Published: 24 September 2015.
Edited by:
Simone Ferrari, Sapienza Università di Roma, ItalyReviewed by:
Anthonia O’Donovan, Technology Centre Biofuel and Biorefinery, IrelandHesham Ali El-Enshasy, Universiti Teknologi Malaysia, Malaysia
Copyright © 2015 Meena, Prasad, Zehra, Gupta and Upadhyay. This is an open-access article distributed under the terms of the Creative Commons Attribution License (CC BY). The use, distribution or reproduction in other forums is permitted, provided the original author(s) or licensor are credited and that the original publication in this journal is cited, in accordance with accepted academic practice. No use, distribution or reproduction is permitted which does not comply with these terms.
*Correspondence: Ram S. Upadhyay, Department of Botany, Banaras Hindu University, Varanasi 221 005, India,dXBhZGh5YXlfYmh1QHlhaG9vLmNvLnVr;dXBhZGh5YXkuYm90QGdtYWlsLmNvbQ==