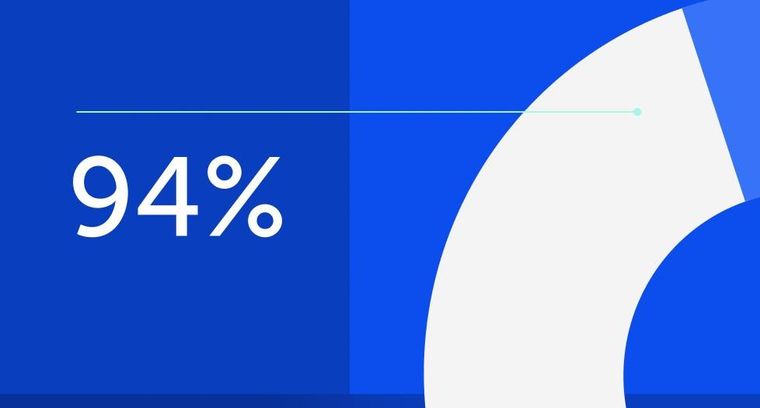
94% of researchers rate our articles as excellent or good
Learn more about the work of our research integrity team to safeguard the quality of each article we publish.
Find out more
SPECIALTY GRAND CHALLENGE article
Front. Membr. Sci. Technol., 28 October 2022
Sec. Membrane Applications - Energy
Volume 1 - 2022 | https://doi.org/10.3389/frmst.2022.1053646
Membranes have always been at the heart of discussions on energy storage and conversion devices such as batteries and fuel cells (Park et al., 2016; Lu et al., 2017; Jiao et al., 2021). This is because they provide the functionality to isolate the cathode and anode as well as to conduct charge-carriers to complete the internal circuit (Guiver, 2022). The membranes must cater to the needs of high chemical and mechanical stability, high selectivity and conductivity, and low cost (Lee et al., 2014; Xiong et al., 2021; Li et al., 2022), since they play a decisive role in a device’s performance and reliability.
Membranes for energy storage and conversion devices can be divided into two types according to the ion transport mechanism: ion exchange membranes (IEMs) based on an ion-exchange mechanism and porous membranes (PMs) based on an ion-sieving mechanism (Yuan et al., 2018; Xiong et al., 2021). The performance of IEMs is dependent on the ion exchange groups fixed to the polymer backbone, which can be classified as an anion-exchange membrane (AEM) holding the OH− or Cl− counter anion, cation-exchange membrane (CEM), and featuring the anion group, which is capable of delivering the cations (i.e., anionic groups holding K+, Na+ or H+ counter-cation) and amphoteric ion-exchange membrane (AIEM), whose surface net charge closely depends on the external solution.
Depending on requirements, the application of ion exchange membranes in energy storage and conversion devices is flexible and not restricted by their ion-exchange groups. For instance, the acidic vanadium flow battery can use an AEM to achieve a high coulombic efficiency, which benefits from the charge repulsion effect between positively charged vanadium ions and positively charged anion-exchange groups (Chen et al., 2013; Mai et al., 2013). CEM can have widespread applications in batteries or fuel cells that also involve an alkaline media (An et al., 2012; Lin et al., 2015; De Porcellinis et al., 2018). Different from ion exchange membranes, PMs, which are widely applied in batteries and flow batteries in particular (Yuan et al., 2016b; Costa et al., 2019; Tan et al., 2020), separate the active materials or fuels from charge carriers via pore size exclusion. Free of ion exchange groups, PMs normally feature the advantages of high stability, diverse structure, low cost, and easy fabrication process. With emphasis focused on promoting the performance of batteries, in recent decades we have witnessed the development of PMs, with significant advances accelerating the application of batteries for energy storage.
Currently, batteries can be divided into static cells (i.e., metal (lithium, sodium, potassium, zinc, aluminum, magnesium)-based batteries, lead-based batteries) and non-static cells (flow batteries). Among these batteries, static batteries and lithium-based and lead-based batteries are widely employed as a portable power supply in electronic devices and electric vehicles because of their high energy density advantage. Their large-scale applications in grid-scale storage still involve challenges, i.e., avoiding the membrane from being pierced by metal dendrite resulting from uneven deposition of metal during charging or overcharging of the battery (or avoiding short circuit and fire explosion that result from thermal runaway of the battery), efficient cascade utilization and green-recycling of the batteries. Compared to lithium-based batteries, flow batteries, which can be divided into inorganic-based flow batteries, all organic-based flow batteries (Janoschka et al., 2015; Huang et al., 2021), and inorganic/organic-based flow batteries (Lin et al., 2015; Zhang et al., 2019; Feng et al., 2021), are safer, and more flexible and adaptable for grid scale storage. Critically different from static batteries, the power (kW∼100 MW) and energy capacity (kWh∼100 MWh) of a flow battery can be designed flexibly. In September 2022, the world’s largest 100 MW/400 MWh flow battery plant reached the final stage of grid-connected debugging and is expected to be formally put into use in October 2022 (https://english.cas.cn/newsroom/research_news/chem/202205/t20220531_306054.shtml). Coupled with renewable energies such as solar and wind power, flow battery technology is expected to contribute to achieving carbon neutrality. Nevertheless, one major challenge for the widespread application of flow battery technology is the lack of high-performance and low-cost membranes. Although promising alternative membranes have been developed in recent decades, the bottleneck issues have not yet been resolved, i.e., breaking the “trade-off” of a membrane between ionic conductivity and selectivity via the controlling of ion movement in the battery, achieving a membrane with high stability, ease of processability and scalability.
Ion exchange membranes have found wide applications in various fields, e.g., chlor-alkali industry, water electrolysis (Li et al., 2021), fuel cells (Mustain et al., 2020), and flow batteries, where the low-cost, high-efficiency, and high-stability for these membranes are vital to realizing the successful deployment of the above technologies. Among numerous ion exchange membranes, the benchmark Nafion® membranes are the most widely used ion exchange membranes because of their ability to maintain excellent stability in critical conditions and easy availability. Nevertheless, these membranes suffer from high cost ($ 500–700 m−2), low ionic conductivity in neutral-or alkaline-based solutions (Hu et al., 2018), and high permeability for fuels or redox couples such as methyl alcohol and vanadium ions. Additionally, the by-products perfluoroalkyl and polyfluoroalkyl substances (PFAS) associated with the production of Nafion pose long-term threats to human health (Yuan et al., 2022). We urgently need innovative high-performance and low-cost membranes to replace benchmark Nafion membranes to address energy needs.
As promising alternatives to benchmark Nafion membranes, hydrocarbon-based ion exchange membranes based on poly (2,6-dimethyl phenylene oxide) (PPO), polyvinyl alcohol (PVA), polyethylene, polysulfone (PSF) or poly (ether ketone) polymer materials have been increasingly receiving attention in the past 30 years on account of their low cost, easy modification, and tunable properties, and demonstrate in fuel cells and batteries (Couture et al., 2011; Merle et al., 2011; Pan et al., 2013; De Porcellinis et al., 2018). However, one of the major bottlenecks for these membranes is their low stability (chemical degradation results in poor mechanical stability) in batteries (Chen and Hickner, 2013; Yuan et al., 2014) and fuel cells (Couture et al., 2011; Merle et al., 2011; Pan et al., 2013; De Porcellinis et al., 2018), which has been proven by the degradation of both polymer backbone and ion exchange groups. Based on the degradation mechanisms of hydrocarbon-based ion exchange membranes, tremendous efforts have concentrated on designing novel polymer materials to enable highly stable IEMs. For instance, strategies for polymer functionalization, polycondensation (Noh et al., 2019) or cationic structures design (Tao et al., 2021) require a highly stable membrane; nevertheless, their long-term stability in practical operating conditions has not yet been verified. Although there has been progress in developing innovative polymer materials with high stability [such as poly (aryl piperidinium)-based and poly (arylene piperidine)-based anion exchange membranes (Chen et al., 2021; Pan et al., 2021)], transformative alternative membranes as efficient as Nafion for energy storage and conversion devices are urgently needed. Another scientific challenge remains to be addressed, i.e., obtaining an ion exchange membrane that can satisfy the need for energy storage and conversion devices, including high ionic conductivity, outstanding selectivity, ease of processability, and high environmental sustainability. Normally, the ion exchange capacity is one of the most important parameters for evaluating the capacity of an IEM to transport charge carriers. A high IEC value can enable a high ionic conductivity, whereas a high IEC value can also lead to a high swelling of the membrane, which could in turn decrease the selectivity and mechanical stability of the membrane. Thus, how to control the IEC of IEM to achieve a balanced ionic conductivity and selectivity (as well as swelling) remains the challenge for the practical application of IEMs.
Current research has demonstrated that the introduction of ion exchange groups triggers the degradation of IEMs. PMs overcome the stability restriction caused by ion exchange groups from IEMs and inspire the development of high-performance and cost-effective membranes for energy storage and conversion devices (Tan et al., 2020). Commercial PMs, such as the Celgard (microporous polyolefin membranes), Daramic (porous polypropylene (PP) membranes) and cellulose-based dialysis membranes have been widely applied in power batteries (such as lithium sulfur battery and lithium (sodium)-based batteries), flow batteries, and electrolyzers. Challenges for these membranes, e.g., the relatively low thermal stability of Celgard and low selectivity toward polysulfide of Celgard, and the relatively low selectivity of Daramic toward redox couples in a flow battery, still need to be overcome. In addition to commercial PMs, other kinds of PMs such as porous polybenzimidazole (PBI) membranes (Yuan et al., 2016a), porous polyether imide (PEI) membranes (Shi et al., 2015), porous poly (ether sulfone) (PES) membranes (Junoh et al., 2021) are also used, which are mainly applied in batteries and fuel cells at the laboratory scale. The application of these laboratory-scale porous membranes in energy storage and conversion devices is a relatively new domain, which may inspire the development of new membranes to enable high device performance. Most porous membranes are prepared by phase separation, where a dense and thick skin layer could be formed. Normally, a denser and thicker skin layer is favored to endow the porous membrane with a high ion selectivity, whereas the ion conductivity of the membrane would be decreased (Dai et al., 2020). Thus, how to break the trade-off between ion conductivity and ion selectivity has been identified as one of the primary challenges that need to be figured out for their practical application. Additionally, the transport mechanism of charge carriers through a porous membrane is not as unambiguous as that in an ion exchange membrane since the structure of the porous membrane is diverse and complex. This is not favored in the design and development of high-performance porous membranes. Although vehicular mechanism and Grotthuss mechanisms can be found and simultaneously exist in porous membranes (Hu et al., 2021), the quantitative contribution of these two mechanisms to the ion conductivity of a porous membrane remains a critical challenge. Different from the fabrication process of IEMs that can be roll-to-roll or melt extrusion manufactured, the phase inversion method used to prepare porous membranes normally requires relatively sophisticated processing, especially in the process of pilot-scale production. Furthermore, how to guarantee the uniformity of porous membranes in this process is also confronted by challenges.
In the past 30 years, significant progress has been achieved in developing the membranes applied in energy storage and conversion devices such as fuel cells, batteries, and electrolyzers. Innovative membranes have significantly enhanced the performance of these devices, e.g., the development of membranes from the perspective of materials synthesis and structure design, increasing the current density from 50 mA cm−2–300 mA cm−2 for vanadium flow battery. Nevertheless, scientific challenges remain to be addressed and further applications explored, i.e., obtaining a membrane that meets the requirements of high ionic conductivity, high selectivity, high stability including high chemical and mechanical stability, inexpensive polymer materials, and ease of manufacture, as well as developing environmentally friendly approaches. The scientific understanding of the degradation mechanism of the membrane, the transport behavior of charge carriers in the membrane, and the relationship between membrane structure and performance mean that we need to develop membranes that meet these demands, which requires new perspectives on polymer materials as well as membrane design and their utilization in energy storage and conversion devices. In this section on “Energy” of the journal Frontiers in Membrane Science and Technology, high-quality original research articles and review articles are preferred and welcomed. They are expected to contribute to membrane development in this field and further bridge the gap between laboratory-scale development of membranes and industrial-scale manufacturing.
This article was drafted by ZY, and revised and finalized by XL.
The authors gratefully acknowledge financial support from the Free Exploring Basic Research Project of Liaoning (2022JH6/100100005), and the “Transformational Technologies for Clean Energy and Demonstration” Strategic Priority Research Program of the Chinese Academy of Sciences (Grant No. XDA21000000 and XDA21070500).
The authors acknowledge Professor Michael D. Guiver, who provided kind suggestions that improved this article.
The authors declare that the research was conducted in the absence of any commercial or financial relationships that could be construed as a potential conflict of interest.
All claims expressed in this article are solely those of the authors and do not necessarily represent those of their affiliated organizations, or those of the publisher, the editors and the reviewers. Any product that may be evaluated in this article, or claim that may be made by its manufacturer, is not guaranteed or endorsed by the publisher.
An, L., Zhao, T. S., Li, Y., and Wu, Q. (2012). Charge carriers in alkaline direct oxidation fuel cells. Energy Environ. Sci. 5 (6), 7536–7538. doi:10.1039/C2EE21734A
Chen, D., Hickner, M. A., Agar, E., and Kumbur, E. C. (2013). Anion exchange membranes for vanadium redox flow batteries. ECS Trans. 53 (7), 83–89. doi:10.1149/05307.0083ecst
Chen, D., and Hickner, M. A. (2013). V5+ degradation of sulfonated Radel membranes for vanadium redox flow batteries. Phys. Chem. Chem. Phys. 15 (27), 11299–11305. doi:10.1039/c3cp52035h
Chen, N., Jin, Y., Liu, H., Hu, C., Wu, B., Xu, S., et al. (2021). Insight into the alkaline stability of N-heterocyclic ammonium groups for anion-exchange polyelectrolytes. Angew. Chem. Int. Ed. Engl. 60 (35), 19272–19280. doi:10.1002/anie.202105231
Costa, C. M., Lee, Y.-H., Kim, J.-H., Lee, S.-Y., and Lanceros-Méndez, S. (2019). Recent advances on separator membranes for lithium-ion battery applications: From porous membranes to solid electrolytes. Energy Storage Mater. 22, 346–375. doi:10.1016/j.ensm.2019.07.024
Couture, G., Alaaeddine, A., Boschet, F., and Ameduri, B. (2011). Polymeric materials as anion-exchange membranes for alkaline fuel cells. Prog. Polym. Sci. 36 (11), 1521–1557. doi:10.1016/j.progpolymsci.2011.04.004
Dai, Q., Liu, Z., Huang, L., Wang, C., Zhao, Y., Fu, Q., et al. (2020). Publisher Correction: Thin-film composite membrane breaking the trade-off between conductivity and selectivity for a flow battery. Nat. Commun. 11 (1), 2609. doi:10.1038/s41467-020-16360-z
De Porcellinis, D., Mecheri, B., D'Epifanio, A., Licoccia, S., Granados-Focil, S., and Aziz, M. J. (2018). Communication—sulfonated poly (ether ether ketone) as cation exchange membrane for alkaline redox flow batteries. J. Electrochem. Soc. 165 (5), A1137–A1139. doi:10.1149/2.1291805jes
Feng, R., Zhang, X., Murugesan, V., Hollas, A., Chen, Y., Shao, Y., et al. (2021). Reversible ketone hydrogenation and dehydrogenation for aqueous organic redox flow batteries. Science 372(6544), 836–840. doi:10.1126/science.abd9795
Guiver, M. D. (2022). Field grand challenge for membrane science and technology. Front. Membr. Sci. Technol. 1. doi:10.3389/frmst.2022.878879
Hu, J., Tang, X., Dai, Q., Liu, Z., Zhang, H., Zheng, A., et al. (2021). Layered double hydroxide membrane with high hydroxide conductivity and ion selectivity for energy storage device. Nat. Commun. 12 (1), 3409. doi:10.1038/s41467-021-23721-9
Hu, J., Zhang, H., Xu, W., Yuan, Z., and Li, X. (2018). Mechanism and transfer behavior of ions in Nafion membranes under alkaline media. J. Membr. Sci. 566, 8–14. doi:10.1016/j.memsci.2018.08.057
Huang, J., Hu, S., Yuan, X., Xiang, Z., Huang, M., Wan, K., et al. (2021). Radical stabilization of a tripyridinium–triazine molecule enables reversible storage of multiple electrons. Angew. Chem. Int. Ed. Engl. 60 (38), 20921–20925. doi:10.1002/anie.202107216
Janoschka, T., Martin, N., Martin, U., Friebe, C., Morgenstern, S., Hiller, H., et al. (2015). An aqueous, polymer-based redox-flow battery using non-corrosive, safe, and low-cost materials. Nature 527 (7576), 78–81. doi:10.1038/nature15746
Jiao, K., Xuan, J., Du, Q., Bao, Z., Xie, B., Wang, B., et al. (2021). Designing the next generation of proton-exchange membrane fuel cells. Nature 595 (7867), 361–369. doi:10.1038/s41586-021-03482-7
Junoh, H., Jaafar, J., Nordin, N A H M, Ismail, A. F., Othman, M. H. D., Rahman, M.A., et al. (2021). Porous polyether sulfone for direct methanol fuel cell applications: Structural analysis. Int. J. Energy Res. 45 (2), 2277–2291. doi:10.1002/er.5921
Lee, H., Yanilmaz, M., Toprakci, O., Fu, K., and Zhang, X. (2014). A review of recent developments in membrane separators for rechargeable lithium-ion batteries. Energy Environ. Sci. 7 (12), 3857–3886. doi:10.1039/C4EE01432D
Li, D., Motz, A. R., Bae, C., Fujimoto, C., Yang, G., Zhang, F.-Y., et al. (2021). Durability of anion exchange membrane water electrolyzers. Energy Environ. Sci. 14 (6), 3393–3419. doi:10.1039/D0EE04086J
Li, X., Jiang, H., Liu, Y., Guo, X., He, G., Chu, Z., et al. (2022). Hierarchically porous membranes for lithium rechargeable batteries: Recent progress and opportunities. EcoMat 4 (1), e12162. doi:10.1002/eom2.12162
Lin, K., Chen, Q., Gerhardt, M. R., Tong, L., Kim, S. B., Eisenach, L., et al. (2015). Alkaline quinone flow battery. Science 349 (6255), 1529–1532. doi:10.1126/science.aab3033
Lu, W. J., Yuan, Z. Z., Zhao, Y. Y., Zhang, H. Z., Zhang, H. M., and Li, X. F. (2017). Porous membranes in secondary battery technologies. Chem. Soc. Rev. 46 (8), 2199–2236. doi:10.1039/c6cs00823b
Mai, Z., Zhang, H., Zhang, H., Xu, W., Wei, W., Na, H., et al. (2013). Anion-conductive membranes with ultralow vanadium permeability and excellent performance in vanadium flow batteries. ChemSusChem 6 (2), 328–335. doi:10.1002/cssc.201200561
Merle, G., Wessling, M., and Nijmeijer, K. (2011). Anion exchange membranes for alkaline fuel cells: A review. J. Membr. Sci. 377 (1), 1–35. doi:10.1016/j.memsci.2011.04.043
Mustain, W. E., Chatenet, M., Page, M., and Kim, Y. S. (2020). Durability challenges of anion exchange membrane fuel cells. Energy Environ. Sci. 13 (9), 2805–2838. doi:10.1039/D0EE01133A
Noh, S., Jeon, J. Y., Adhikari, S., Kim, Y. S., and Bae, C. (2019). Molecular engineering of hydroxide conducting polymers for anion exchange membranes in electrochemical energy conversion technology. Acc. Chem. Res. 52 (9), 2745–2755. doi:10.1021/acs.accounts.9b00355
Pan, D., Pham, T. H., and Jannasch, P. (2021). Poly(arylene piperidine) anion exchange membranes with tunable N-alicyclic quaternary ammonium side chains. ACS Appl. Energy Mat. 4 (10), 11652–11665. doi:10.1021/acsaem.1c02389
Pan, J., Li, Y., Han, J., Li, G., Tan, L., Chen, C., et al. (2013). A strategy for disentangling the conductivity–stability dilemma in alkaline polymer electrolytes. Energy Environ. Sci. 6 (10), 2912–2915. doi:10.1039/C3EE41968A
Park, C. H., Lee, S. Y., Hwang, D. S., Shin, D. W., Cho, D. H., Lee, K. H., et al. (2016). Nanocrack-regulated self-humidifying membranes. Nature 532 (7600), 480–483. doi:10.1038/nature17634
Shi, J., Xia, Y., Yuan, Z., Hu, H., Li, X., Zhang, H., et al. (2015). Porous membrane with high curvature, three-dimensional heat-resistance skeleton: A new and practical separator candidate for high safety lithium ion battery. Sci. Rep. 5 (1), 8255. doi:10.1038/srep08255
Tan, R., Wang, A., Malpass-Evans, R., Williams, R., Zhao, E. W., Liu, T., et al. (2020). Hydrophilic microporous membranes for selective ion separation and flow-battery energy storage. Nat. Mat. 19 (2), 195–202. doi:10.1038/s41563-019-0536-8
Tao, Z., Wang, C., Zhao, X., Li, J., and Guiver, M. D. (2021). Progress in high-performance anion exchange membranes based on the design of stable cations for alkaline fuel cells. Adv. Mat. Technol. 6 (5), 2001220. doi:10.1002/admt.202001220
Xiong, P., Zhang, L., Chen, Y., Peng, S., and Yu, G. (2021). A chemistry and microstructure perspective on ion-conducting membranes for redox flow batteries. Angew. Chem. Int. Ed. Engl. 60 (47), 24770–24798. doi:10.1002/anie.202105619
Yuan, Z., Li, X., Hu, J., Xu, W., Cao, J., and Zhang, H. (2014). Degradation mechanism of sulfonated poly (ether ether ketone)(SPEEK) ion exchange membranes under vanadium flow battery medium. Phys. Chem. Chem. Phys. 16 (37), 19841–19847. doi:10.1039/c4cp03329a
Yuan, Z., Liang, L., Dai, Q., Li, T., Song, Q., Zhang, H., et al. (2022). Low-cost hydrocarbon membrane enables commercial-scale flow batteries for long-duration energy storage. Joule 6 (4), 884–905. doi:10.1016/j.joule.2022.02.016
Yuan, Z., Zhang, H., and Li, X. (2018). Ion conducting membranes for aqueous flow battery systems. Chem. Commun. 54 (55), 7570–7588. doi:10.1039/c8cc03058h
Yuan, Z., Duan, Y., Zhang, H., Li, X., Zhang, H., and Vankelecom, I. (2016a). Advanced porous membranes with ultra-high selectivity and stability for vanadium flow batteries. Energy Environ. Sci. 9 (2), 441–447. doi:10.1039/c5ee02896e
Yuan, Z., Zhu, X., Li, M., Lu, W., Li, X., and Zhang, H. (2016b). A highly ion-selective zeolite flake layer on porous membranes for flow battery applications. Angew. Chem. Int. Ed. Engl. 55 (9), 3058–3062. doi:10.1002/anie.201510849
Keywords: energy storage and conversion, battery, porous membrane, ion-exchange membrane, ion transport mechanism, upscaling and application
Citation: Yuan Z and Li X (2022) Grand challenges in membrane applications—Energy. Front. Membr. Sci. Technol. 1:1053646. doi: 10.3389/frmst.2022.1053646
Received: 26 September 2022; Accepted: 17 October 2022;
Published: 28 October 2022.
Edited and reviewed by:
Michael D. Guiver, Tianjin University, ChinaCopyright © 2022 Yuan and Li. This is an open-access article distributed under the terms of the Creative Commons Attribution License (CC BY). The use, distribution or reproduction in other forums is permitted, provided the original author(s) and the copyright owner(s) are credited and that the original publication in this journal is cited, in accordance with accepted academic practice. No use, distribution or reproduction is permitted which does not comply with these terms.
*Correspondence: Xianfeng Li, bGl4aWFuZmVuZ0BkaWNwLmFjLmNu
Disclaimer: All claims expressed in this article are solely those of the authors and do not necessarily represent those of their affiliated organizations, or those of the publisher, the editors and the reviewers. Any product that may be evaluated in this article or claim that may be made by its manufacturer is not guaranteed or endorsed by the publisher.
Research integrity at Frontiers
Learn more about the work of our research integrity team to safeguard the quality of each article we publish.