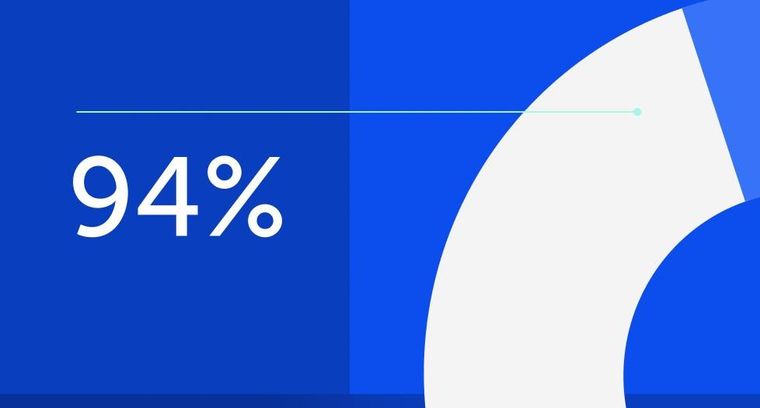
94% of researchers rate our articles as excellent or good
Learn more about the work of our research integrity team to safeguard the quality of each article we publish.
Find out more
ORIGINAL RESEARCH article
Front. Med., 03 April 2025
Sec. Translational Medicine
Volume 12 - 2025 | https://doi.org/10.3389/fmed.2025.1561732
This article is part of the Research TopicAdvancements in Translational Models: Bridging Basic Infection Research and Clinical ApplicationsView all articles
Chronic wounds, particularly pressure ulcers, pose significant healthcare challenges, especially in the elderly population. This study presents an experimental murine model of chronically infected pressure ulcers using a single cycle of magnet-induced ischemic injury combined with infection by bioluminescent Staphylococcus aureus. The model addresses previous limitations in studying pressure ulcer infection pathogenesis and evaluating treatment efficacy. By combining this model with in vivo imaging system (IVIS) technology, we achieved real-time, non-invasive monitoring of infection dynamics. This approach demonstrated persistent pressure ulcer wound infection and provided temporal and spatial data on infection status. To validate the model’s utility, we evaluated the antimicrobial efficacy of TCP-25, a synthetic host defense peptide, delivered in a topical gel formulation. Our findings highlight the potential of this model for investigating wound infection mechanisms, bacterial persistence, and therapeutic interventions. This innovative approach represents a significant advancement in pressure ulcer research, offering new opportunities for developing effective treatment strategies and improving patient outcomes.
Chronic wounds, including pressure ulcers, venous leg ulcers, and diabetic foot ulcers, mainly affect older adults and pose significant challenges to healthcare systems (1). Pressure ulcers, also known as bedsores or decubitus ulcers, represent a significant medical problem, particularly affecting immobilized or elderly patients. These wounds develop when prolonged pressure on the skin reduces blood flow, leading to tissue damage and necrosis (2). Pressure ulcers are highly susceptible to bacterial colonization and infection, with Staphylococcus aureus being one of the most prevalent pathogens isolated from these wounds (3, 4). S. aureus infections can significantly impair wound healing and lead to complications, including sepsis, highlighting the need for effective treatment strategies and reliable experimental models.
To better understand the pathogenesis of pressure ulcer infections and evaluate potential treatments, animal models that closely mimic human wound conditions are essential. Mouse models have been widely used in wound healing research due to their cost-effectiveness, ease of handling, and availability of genetically modified strains. Animal models of chronic wounds are limited and challenging to develop (5–7), with few studies demonstrating successful incorporation of exogenous wound pathogens in these models (7, 8). Moreover, developing a reliable murine model of chronically infected pressure ulcers has been challenging due to either self-limiting nature of infection or onset of sepsis (9, 10). This makes the study of pressure ulcer infection pathogenesis and potential treatments difficult.
Recent advances in pressure ulcer modeling have led to the development of more clinically relevant experimental mouse models. One such approach involves the use of magnets to create ischemia–reperfusion injuries that simulate the human conditions leading to pressure ulcer formation (11–13). This method offers several advantages, including controlled and reproducible injury, mimicking human pathophysiology, and being minimally invasive. Use of magnets to induce ischemic injury, presents a more accurate representation of pressure ulcers, enabling the study of complex interactions between ischemia–reperfusion, tissue damage, bacterial colonization, and the host immune response in a controlled setting (11–13).
Wound studies are often invasive and ethically challenging, raising concerns about animal welfare. Non-invasive longitudinal monitoring methods would facilitate unbiased evaluation of wound infection dynamics and treatment efficacy, reducing the need for frequent tissue sampling and animal sacrifice. By using strains engineered to express luciferase enzymes, bacterial growth and dissemination can be tracked in real-time using an in vivo imaging system (IVIS). The use of bioluminescent S. aureus strains has enhanced our ability to monitor infection progression in various mouse models (14–16). The use of IVIS technology for longitudinal infection imaging offers several significant advantages. It reduces animal usage by allowing repeated measurements on the same animals, enhances statistical power through longitudinal data collection, provides high temporal resolution of infection dynamics, and offers detailed spatial information on bacterial spread. Additionally, bioluminescent signals can be quantified, allowing for objective comparisons between treatment groups and over time. These advantages make the combination of bioluminescent S. aureus and IVIS technology a powerful tool for investigating wound infection pathogenesis and evaluating potential therapeutic interventions.
In this study, we have established a pressure ulcer wound mouse model using magnet-induced ischemic injury and infected it with bioluminescent S. aureus. The model shows persistent S. aureus infection and allows for the evaluation of antimicrobial treatments. We used bioluminescent S. aureus and achieved real-time monitoring of infection kinetics and spread. We tested our model to assess the antimicrobial efficacy of a topical gel containing TCP-25, a synthetic host defense peptide that has shown promising antimicrobial activity in vitro and in vivo (14, 17).
The persistence of S. aureus in pressure ulcers is a critical factor with respect to wound healing and infection management. Recent studies have shown that S. aureus can persist in pressure ulcers for extended periods, potentially adopting a colonizing role rather than acting as an acute pathogen (18, 19). This persistence may be associated with changes in bacterial virulence and adaptation to the wound environment. Understanding these dynamics is crucial for developing effective treatment strategies and improving patient outcomes.
In conclusion, the development of a pressure ulcer wound mouse model using magnet-induced ischemic injury and infection with bioluminescent S. aureus represents a significant advancement for wound research. This model, combined with IVIS technology, offers a unique opportunity to gain insights into the complex interactions between host tissue, bacterial pathogens, and potential therapeutic interventions in a clinically relevant setting. The successful evaluation of TCP-25 gel using this model demonstrates its potential for assessing novel antimicrobial strategies and advancing our understanding of pressure ulcer treatment options.
This study establishes and characterizes a murine model of chronically S. aureus-infected pressure ulcers. Balb/c mice underwent a single 16-h cycle of magnet-induced ischemic injury on the dorsal skin to induce pressure ulcers. Following ischemia–reperfusion, wounds were inoculated with bioluminescent S. aureus (SAP229). The wounds were then covered in HEC gel (for moisture) and a dressing. Infection dynamics were monitored non-invasively over 14 days using in vivo bioluminescent imaging (IVIS) and microbiological analysis (CFU counts from wound swabs and dressings). Wound pathology was assessed via histological analysis and cytokine profiling of wound fluid. Finally, the model’s utility for therapeutic evaluation was demonstrated by assessing the efficacy of a topical TCP-25-containing hydrogel in reducing bacterial burden. A control group, with pressure ulcer without S. aureus infection was also studied. Wounds were observed for macroscopic changes, bacterial load, longitudinal infection imaging, and cytokines. Data were analyzed using appropriate statistical methods to compare groups and assess the effects of treatment.
The peptide TCP-25 (GKYGFYTHVFRLKKWIQKVIDQFGE) was synthesized by Ambiopharm (North Augusta, USA). The purity (95%) of peptide was confirmed by mass spectral analysis (MALDI-ToF Voyager).
The bacterial strain used for the pressure ulcer wound infection was bioluminescent S. aureus SAP229, kindly provided by Dr. Roger D. Plaut (Division of Bacterial, Parasitic, and Allergenic Products, FDA, Bethesda, Maryland, United States). This bacterial strain has been widely used in our experimental wound infection studies and in vivo imaging (14–16).
Bacteria are routinely cultured on Todd Hewitt agar (THA). Using a 1 μL loop, a colony was inoculated in a tube with 5 mL of Todd Hewitt Broth (THB) and cultured overnight at 37°C in a shaking incubator. The following morning, to refresh the culture, 100 μL of the overnight culture was inoculated into a new tube containing 5 mL of THB and incubated at 37°C in a shaking incubator. The bacteria were grown until the optical density (OD) at 620 nm reached between 0.4 and 0.6. The bacteria culture was then centrifuged at 5,600 rpm for 10 min. The supernatant was discarded, and the pellet was washed in 5 mL Tris buffer (10 mM, pH 7.4) and centrifuged again at 5,600 rpm for 5 min. The supernatant was then discarded, and the bacteria were diluted in Tris buffer to a cell density of 2 × 109 CFU/mL. This final bacterial suspension was used to infect mouse pressure ulcer wounds.
A hydrogel was prepared using the polymer hydroxyethylcellulose (HEC) (Natrosol™ 250HX, Ashland Inc., UK) and formulated with or without the addition of TCP-25. The gel composition included 1.37% (w/v) Natrosol™ 250HX, 1.21 g/L Tris, and 25 g/L glycerol, adjusted to pH 7.0, with or without 8.6 mg/mL TCP-25.
Graphic illustrations and photographs from the experimental set-up and workflow of the S. aureus infected murine pressure ulcer model are presented in Figures 1, 2. Balb/c mice (8-12-week-old male; Janvier Labs, France) were used for the study. In a low-flow anesthesia system (SomnoSuite, Kent Scientific), mice were anaesthetized using isoflurane (Baxter; 4% for induction and 2% for maintenance). All procedures were performed under aseptic conditions. Hair from the dorsum were removed using a hair trimmer. For depilation, using a cotton swab, a depilatory cream was applied on the trimmed area and 2 min later, the area was cleaned with a gauze. Finally, to remove any traces of cream, the area was cleaned with prewet gauze and dried. Using a skin marker, midline and area of magnet placement were marked. Approximately 1 cm space was left in between two magnets. Pressure ulcers were induced by sandwiching the skin between two round ferrite magnets (12 × 5 mm; MAGNORDIC, Denmark), each with a pulling force of 0.3 kilogram. The mice were immediately moved to their cages. Mice with magnets showed normal behaviors and no signs of discomfort was observed. After 16 h, the magnets were removed, and the animals were allowed to rest for 6 h to facilitate skin reperfusion. Clear, round ischemic areas were observed at the place where magnets had been applied. Subsequently, the ischemic wounds were infected with 104 CFU of S. aureus suspension (10 μL). In a group of animals, control wounds were not infected with bacteria. To maintain moisture, 50 μL of HEC gel was applied to the wounds, followed by covering with a layer of primary dressing (Mepilex transfer; Mölnlycke Health Care) and a secondary dressing (Tegaderm film, 3 M). Finally, a layer of flexible self-adhesive bandage (Vet Flex, Kruuse, Denmark) was applied over the dressings and around the body. Under isoflurane anesthesia, dressing changes were performed on days 1, 2, 4, 6, 8, 10, and 12. On each dressing change, photography, wound swab sample collection, IVIS imaging was done, and new HEC gel was applied. The experiment was terminated on day 14, and wound skin tissue was collected in 10% neutral buffered formalin for further histological analysis. Wound dressing weights and pictures, as well as wound pictures, were recorded daily.
Figure 1. Graphic illustration demonstrating the experimental set-up and workflow of the S. aureus infected murine pressure ulcer model. The model employs application of two circular magnets on skin fold on dorsum. (A,B) Illustration shows position of magnets on skin fold. (C) Pressure exerted by magnets create an ischemic region in the skin fold. (D) Upon removal of magnets, two ischemic wound regions can be observed on dorsal skin. (E) The wounds are inoculated with an S. aureus bacterial suspension. (F) Wound dressings are applied to protect the wounds.
Figure 2. Images showing murine pressure ulcer model during the various procedural steps. (A) Mouse is anaesthetized with isoflurane using a Low-Flow Anesthesia system. (B) Hair from the back is trimmed using a hair-trimmer. (C) Hair depilation is achieved using a depilatory cream. (D) Depilatory cream is removed, and the skin cleaned. (E) Using a skin marker, area is marked for positioning magnets. (F) Two magnets are placed on the skin fold. (G) After desired time, magnets are removed. Two ischemic regions can be seen after removal of magnets.
For the therapeutic evaluation of TCP-25 gel, treatments were initiated 30 min after bacterial inoculation. HEC gel (vehicle) or 8.6 mg/mL TCP-25 gel (50 μL) was applied to the wounds, followed application of dressings as described above. Dressing changes were performed on days 1, 2, 4, and 6. On each dressing change, photography, wound swab sample collection, IVIS imaging was performed, and new control gel or TCP-25 gel was applied. The selection of 8.6 mg/mL TCP-25 concentration was motivated by our previous study (14).
A DSLR camera (Canon EOS Rebel T7i, Japan) equipped with a 60 mm f/2.8 macro lens (Canon EF-S 60 mm, Japan) was used to capture high resolution photographs of the pressure ulcer wounds. A ring flash system (Canfield Twin Flash, United States) was used to ensure standardized lighting (20). A photography copy stand (Kaiser RS 2 XA) with a baseboard was used to keep standard camera distance and angle conditions. Wound photographs were taken immediately after dressing removal. A disposable single-use sticky ruler was attached on the mouse back below the wound area. Wounds pictures were taken including the ruler, to use it as reference during analyses with ImageJ (National Institute of Health). Upon opening an image with the program, the 1 cm distance represented by the ruler was converted to pixels by mean of the “Straight” command. Briefly, the two lines 1 cm far on the ruler, were connected by the “Straight line” command. The length of the generated straight line, representing 1 cm, was converted in pixel by the “Analyze”== > “Set Scale” command, setting “Known distance” as 1 and cm as “Unit of Length.” Then, each wound perimeter was manually followed using the “Freehand” command. The area included in the traced perimeter was then measured in mm2 by the “Analyze”== > “Set Scale” command. To have a precise measure for each wound, the procedures were repeated for every image.
Dressings and swabs from wounds were soaked in 500 μL of PBS and vortexed to release bacteria. Seven serial dilutions were performed, and the samples were plated on TH agar plates and incubated for 16 h at 37°C in a 5% humidified CO2 incubator. Then, the colonies were counted, and CFUs calculated.
For cytokine analysis, wound fluid was extracted from the primary dressing (Mepilex Transfer). Dressings from wounds were soaked in 500 μL of PBS in 1.5 mL tube and vortexed. The tubes were then centrifuged (600 × g at 4°C, 5 min), and the supernatant was collected for cytokine measurement. Cytokines were measured using the CBA Mouse Inflammation Kit (Becton Dickinson) according to the manufacturer’s instructions. In brief, the kit utilizes beads with different fluorescence intensities, each coated with antibodies against specific murine cytokines that can be quantified simultaneously after the addition of a PE-conjugated secondary antibody to the reaction mix.
Pressure ulcer wound infection was longitudinally evaluated by measuring bacterial bioluminescence using non-invasive imaging. An In vivo imaging system (IVIS spectrum, Perkin Elmer) coupled with Living Image 4.5.5 Software (PerkinElmer) was used. IVIS imaging was performed as described previously (21). In brief, mice were anesthetized in induction chamber using 4% isoflurane-mixed oxygen. Dressings were removed and mice were then transferred to the IVIS imaging chamber and anesthesia was maintained using 2% isoflurane. The mice were positioned in prone position. In Living Image software’s IVIS acquisition control panel, luminescent imaging mode and auto exposure was used for imaging. After image acquisition, a region of interest (ROI) was selected around the wound and the bioluminescence was measured using Living Image program. Heatmaps were created to visualize signals in produced images.
Skin tissue harvested from the pressure ulcer wounds was placed on absorbent paper to prevent curling and fixed overnight in 10% neutral buffered formalin. After serial dehydration, the tissue was embedded in paraffin blocks, sectioned at 5 μm, and stained with hematoxylin and eosin (H&E). Samples were imaged with bright field microscopy (Axioplan2, Zeiss, Germany) under 100× and 200× magnifications. From each H&E-stained sections, 4–5 microscopic views (100×) were scored which covered most of the wound area. Scoring was done on a scale of 0–5 (where 0 is worse and 5 is best score) (14). The histology scoring was based on epithelization, granulation tissue, inflammatory cells, abscesses and tissue architecture.
Differences between groups were statistically analyzed using Student’s t-test for normally distributed data and the Mann–Whitney U test for non-normally distributed data. For grouped analysis, means of more than two groups were compared using two-way ANOVA followed by Sidak’s multiple comparison test. Data are presented as means ± SEM. Details of statistical analysis are indicated in each figure legend and GraphPad Prism software v10 was used. P-values <0.05 were statistically significant.
This model utilizes application of two circular magnets over a skin fold, followed by inoculation of the wound area with bioluminescent S. aureus (Figures 1, 2). Briefly, mice were anaesthetized, and dorsal skin area was prepared by depilation and cleaning. To induce ischemia, the dorsal skin fold was compressed between two circular magnets for 16 h. After magnet removal, the ischemic wound area was infected with luminescent S. aureus and a neutral hydroxyethyl cellulose (HEC) gel was applied to keep the wound area moist. Wounds were covered with a primary polyurethane (PU) foam dressing and secured with Tegaderm film dressing. Subsequently, the wounds were analyzed for macroscopic changes, bacterial load, longitudinal infection imaging, and cytokines.
One ischemia–reperfusion cycle using a single application of magnets for 16 h was sufficient to produce visible circular ischemic regions on the skin (Figure 3A). After removal of magnets at day 0, pale circular wounds were clearly observed. Within pale areas, some petechiae can be observed which might be due to the subcutaneous hemorrhage due to the pressure exerted by the magnets. At day 0, the wounds were infected with S. aureus, a common pressure ulcer wound pathogen (3, 22). At day 2, the pale ischemic wound area started to appear yellowish as a sign of infection establishment. Wounds remained yellowish until day 10 and purulent discharge could be seen upon dressing changes. In this model, as expected, severe contraction was observed as wound size reduced over time and reached to almost 25% of the initial wound area at day 14 (Figure 3B). In contrast to the S. aureus-infected wounds, the control group with non-infected pressure ulcers showed no purulent discharge (Supplementary Figure 1A). However, the degree of wound contraction observed in the non-infected control group was somewhat similar to that of the infected wounds (Supplementary Figure 1B). This suggests that the wound contraction may be primarily driven by the initial ischemic injury and subsequent healing processes, rather than being solely dependent on the presence of infection.
Figure 3. Characteristics of wound infection and tissue pathology in S. aureus infected murine pressure ulcer model. (A) Photographs show macroscopic appearance of pressure ulcer wounds during 14 days of experimental protocol. (B) The wound area was measured and represented here as percent wound area. (C) H&E staining was used to study wound tissue histology. (D) Bar-chart shows histology scoring. Uninfected pressure ulcer wounds and normal unwounded skin was used for the comparison. Data are represented as the mean ± SEM (n = 6). P-values were determined using an unpaired t-test. Significant results are denoted as ***p < 0.001. Arrow, immune cell infiltration; filled arrowhead, epidermis; hollow arrowhead, distinct area of proliferative phase characterized by high cellular activity.
Hematoxylin and eosin (H&E) staining of wound biopsies collected at day 14 showed significant tissue changes. Compared to the normal unwounded skin, no visible epidermis, no distinct dermis and basement membrane were observed in the pressure ulcer wounds (Figure 3C). Skin tissue was significantly infiltrated with immune cells and normal skin tissue architecture was completely absent. Histology scoring showed significantly poorer score for the pressure ulcer wound tissue (Figure 3D). The non-infected pressure ulcer wounds exhibited distinct areas of proliferative phase characterized by high cellular activity and re-epithelialization. While the skin tissue architecture in non-infected wounds was significantly better than in infected wounds, it still showed impairment compared to normal skin tissue. Notably, fewer inflammatory cells were observed in non-infected wounds compared to their infected counterparts. The histology score of non-infected wounds was significantly better than infected wounds but poorer than normal tissue, providing a clear demonstration of the impact of bacterial infection on wound healing and tissue integrity. Taken together, results show that this experimental method produces reproducible pressure ulcer wounds with significant tissue changes over a 14-day period.
We next investigated whether S. aureus could establish a persistent infection within the pressure ulcer wounds created by our model. Ischemic wounds were infected with a low inoculum of S. aureus (104 CFUs). Wound dressings were changed at days 1, 2, 4, 6, 8, 10, 12, and 14. During dressing changes, wound swab samples were taken, and primary dressing material was collected for microbiological analysis. A significant wound bacterial load was observed over the 14-day study period (Figure 4A). Wound swab CFU counts showed an increasing trend until day 8 after which it appeared to be stable until day 14. The CFU count from the dressings was higher than the swab CFU count but showed a similar trend (Figure 4B). No mice showed signs of sepsis or mortality during this period. The results showed a persistent infection in these pressure ulcer wounds.
Figure 4. Colony Forming Unit (CFU) analysis of S. aureus infected murine pressure ulcer wounds. (A) Wound swab samples were taken at days 1, 2, 4, 6, 8, 10, 12, and 14 and CFUs were determined. (B) In addition, wound dressings were collected and analyzed for CFU. Data are presented as the mean ± SEM (n = 8). Red data points overlaid on the bar chart represent the individual Colony Forming Unit (CFU) counts obtained from each wound.
Longitudinal in vivo imaging was conducted to track infection kinetics and verify that the persistent infection was specifically due to S. aureus. Bioluminescent bacterial strains allow monitoring of bacterial growth and spread in real-time through bioimaging with an in vivo imaging system (IVIS), facilitating non-invasive longitudinal studies of infection dynamics. This approach offers an advantage, as microbiological analysis of wounds with agar-based methods do not rule out the possibility of wound contamination by other host bacteria from the mice. Therefore, we used luminescent S. aureus in combination with IVIS imaging.
Mice were imaged at days 1, 2, 4, 6, 8, and 14. In agreement with the CFU data, the pressure ulcer wounds showed significant infection with S. aureus which increased until day 6 and then appeared to stabilize (Figures 5A,B). Superficially, infection appeared to spread outside the wound margins, however most of the bacterial load appeared to be within the pressure ulcer wounds. As evidenced by the intense bioluminescent signal, S. aureus was localized within the pressure ulcer wounds, and no luminescent emission was observed from other parts of the body indicating that the experimental model did not lead to bacterial spread and sepsis. In conclusion, the results showed that persistent infection is indeed due to S. aureus and moreover, that the infection is limited to the pressure ulcer wound area and surroundings.
Figure 5. Longitudinal in vivo infection imaging and cytokine analysis in the mouse model of S. aureus infected murine pressure ulcer wounds. (A) To achieve imaging of bacterial infection, pressure ulcer wounds were infected with bioluminescent S. aureus bacteria and were non-invasively analyzed using the IVIS bioimaging system. Representative heat-map images show bacterial luminescence at days 1, 2, 4, 6, 8, and 14. (B) The line graph shows measured bioluminescence intensity emitted by the bacteria. Data are presented as the mean ± SEM (n = 8). (C) Analysis of wound fluid cytokines collected on days 1, 2, 4, 6 and 8. Data are presented as the mean ± SEM (n = 6).
We next examined whether tissue changes in the pressure ulcers were associated with altered levels of pro-inflammatory cytokines in the wound fluid. Cytokines were measured in wound fluid extracted from dressing materials on days 1, 2, 4, 6 and 8. TNF-α, IL-6 and MCP-1 were detected, with notably higher levels observed during the initial days (Figure 5C).
A disease model’s utility depends on its ability to accurately differentiate therapeutic responses, thereby bridging the gap between preclinical research and clinical outcomes. Therefore, we wanted to test if the here described S. aureus infected pressure ulcer model can be utilized for treatment evaluation. We have previously shown that a host defense peptide, TCP-25, formulated in a hydrogel is effective against S. aureus wound infection in a porcine partial thickness wound model (14). In a proof-of-concept study, S. aureus infected pressure ulcer wounds were treated with 8.6 mg/mL TCP-25 gel. Wound dressings were changed on days 1, 2, 3, 4, and 6 and new gel was applied. An identical gel without TCP-25 was used as control. Non-invasive IVIS imaging was performed on days 2, 4 and 6. Results showed a significant reduction in bacterial infection in the wounds treated with TCP-25 gel (Figures 6A,B). Reduced bacterial spread was also observed in the TCP-25-gel treated wounds. Consistent with the IVIS imaging results, swab CFU count showed a significant reduction in CFUs in the wounds treated with TCP-25 gel (Figure 6C). Wound photographs show reduced wound exudate in TCP-25 treated wounds, suggesting a reduction in inflammation and bacterial load, and a cleaner, less inflamed appearance compared to control wounds, although macroscopic differences are subtle (Supplementary Figure 2). Taken together, these findings show that the S. aureus infected pressure ulcer model presented in this work can be used to evaluate efficacy of antimicrobial therapies.
Figure 6. Evaluation of therapeutic effects of TCP-25, a host defense peptide, in S. aureus infected murine pressure ulcer wounds. S. aureus infected murine pressure ulcer wounds were treated daily with a TCP-25-containing hydrogel. Control wounds were treated with only hydrogel. (A) Longitudinal in vivo infection imaging using IVIS. Representative heat-map images show bacterial luminescence at days 2, 4, and 6. (B) The bar-chart shows measured bioluminescence intensity emitted by the bacteria. Data are presented as the mean ± SEM (n = 6). (C) Microbiological analysis of TCP-25 gel treated pressure ulcer wounds. Wound swab samples were taken at days 2, 4, and 6 and CFUs were determined. Data are presented as the mean ± SEM (n = 6). P-values were determined using a two-way ANOVA. *p ≤ 0.05; **p ≤ 0.01; ns, not significant.
Various published models vary in their approach in mimicking the clinical conditions leading to pressure ulcers, with some focusing on ischemia–reperfusion injury, others on continuous pressure, and some combining multiple factors like Spinal cord injury (SCI). The choice of model depends on the specific research questions and the aspects of pressure ulcer pathophysiology being studied. Stadler et al. developed a model using two magnets to sandwich a pinch of skin, creating ischemia–reperfusion cycles by removing and reapplying the magnets (11). Similar approach was used by other studies where two round ceramic magnetic plates generating 50 mmHg compressive pressure and three 12-h ischemia/12-h reperfusion cycles were applied (11, 12, 23, 24). A model using a modified compression device delivering 150 mmHg pressure to human full-thickness skin grafts on mice was also developed (25). Three cycles of 8-h compression and 16-h release are applied. Another study reported a model involving implantation of one magnet under the skin and placing another externally, sandwiching the skin for 7 consecutive days (26). A model combining complete spinal cord transection with magnetic disc compression of a skin fold for 12 h, mimicking pressure ulcers in SCI patients has also been described (27). However, these studies did not address establishment of infection. Most clinical cases of pressure ulcer show presence of one or more bacterial species. Hence, in this study, focus was to establish a chronically infected pressure ulcer wound model so that dynamics and pathophysiology of infection and treatment efficacy could be studied.
Our approach, using magnet-induced ischemic injury combined with bioluminescent S. aureus successfully addresses previous limitations in modeling pressure ulcer infections. Most other studies have used more than one cycles of ischemia reperfusion (11, 13). However, the model described here uses a single ischemia perfusion cycle, minimizing animal discomfort and reducing time requirements.
Infection establishment requires ambient and favorable conditions for bacterial colonization, and they are difficult to create specially when a single species of bacteria is exogenously inoculated to the wound. Among others, one important factor is maintenance of moisture at the site of inoculation. Using HEC gel to keep wounds moist, we have previously shown successful bacterial colonization of porcine partial thickness wounds (14). In the mouse model, pressure ulcers wounds are not open and comparatively dry during first few days. After bacterial inoculation and at each dressing change, we used HEC gel which promoted bacterial colonization despite a low inoculum size. The bacterial numbers increased during the first week and then stabilized. The sustained bacterial colonization observed over 14 days without causing sepsis closely mimics clinical pressure ulcer infections. The use of bioluminescent S. aureus with IVIS imaging enabled real-time tracking of infection dynamics, providing detailed spatial and temporal information about bacterial spread and treatment efficacy. In addition, this approach confirmed that bacterial colonization observed in imaging is indeed due to S. aureus. The IVIS system specifically detects light emitted by engineered S. aureus strain, providing real-time monitoring of infection dynamics. The localized and intense IVIS signals within the wounds strongly indicate that S. aureus is the predominant, and likely causative, agent of infection. While we cannot rule out the presence of other host bacteria at low levels, their contribution to the overall infection is likely minor given the IVIS findings. The persistent presence of S. aureus in pressure ulcer wounds points to a chronic infection that likely disrupts normal wound healing processes through sustained inflammation and tissue damage.
The histological changes and proinflammatory cytokine profiles observed in our model reflect the complex pathophysiology of human pressure ulcers. The presence of TNF-α, IL-6, and MCP-1 in wound fluid, particularly elevated in the early phase, indicates an active inflammatory response typical of chronic wounds. The use of wound fluid extracted from the primary dressing material enabled us to perform longitudinal cytokine analysis non-invasively. Wound histology at 14 days showed tissue necrosis, abnormal tissue architecture, severe inflammation and immune cell infiltration.
The utility of an animal model is determined by its ability to not only address fundamental biological research questions but also to effectively evaluate the efficacy of potential therapeutic interventions. We selected TCP-25 due to its unique combination of antimicrobial and anti-inflammatory properties, making it an ideal candidate for wound treatment. This thrombin-derived peptide demonstrates effective antibacterial activity against both Gram-positive and Gram-negative bacteria, including S. aureus and P. aeruginosa (14). Importantly, TCP-25 also scavenges pathogen-associated molecular patterns (PAMPs) like lipopolysaccharide (LPS), preventing CD14 interaction and Toll-like receptor dimerization, thus reducing downstream immune activation (28). This dual-action mechanism allows TCP-25 to address both infection and inflammation simultaneously. Its usability is demonstrated by its ability to be incorporated into hydrogel formulations (14) and dressings (16), allowing for various application methods in wound treatment. TCP-25 has shown therapeutic potential in experimental models of bacterial sepsis, endotoxin shock, and wound infections, and exhibits broad-spectrum activity against multiple bacterial species while modulating responses to various microbe-derived agonists (14, 15, 17). These comprehensive attributes make TCP-25 a promising candidate for developing novel wound treatments that effectively target both bacterial infection and inflammation. Our successful evaluation of TCP-25 gel demonstrates the model’s utility for testing novel antimicrobial formulation. The ability to quantitatively assess treatment outcomes through both IVIS imaging and traditional microbiological methods provides robust validation capabilities. While IVIS offers the distinct advantages of specific pathogen detection, repeated measurements on the same animal, high temporal resolution, and detailed spatial information, alternative methods can indeed be used to assess S. aureus infection in our model. For example, selective media like mannitol salt agar can effectively isolate S. aureus. Furthermore, 16S rRNA gene sequencing provides a comprehensive assessment of the wound microbiome, while qPCR offers a means of quantifying S. aureus DNA. We also recently demonstrated a simple filter paper-based method for spatial analysis of wound infection (20). There are several limitations in our study. First, our model focuses on a single bacterial species (S. aureus), whereas clinical pressure ulcers often involve polymicrobial infections. Second, while murine models offer practical advantages, they do not fully replicate human wound healing. In addition, our cytokine analysis, based on wound fluid, provides a localized assessment that may not reflect systemic responses.
In conclusion, this model offers a reliable platform for investigating new therapeutic strategies and understanding the mechanisms of bacterial persistence in pressure ulcers. The non-invasive monitoring approach reduces animal usage while providing comprehensive data on infection dynamics and treatment responses. Future studies using this model could explore host-pathogen interactions, bacterial adaptation mechanisms, and novel treatment combinations, ultimately contributing to improved clinical management of pressure ulcer infections.
The raw data supporting the conclusions of this article will be made available by the authors, without undue reservation.
All animal experiments were performed according to Swedish Animal Welfare Act SFS 1988:534 and were approved by the Animal Ethics Committee of Malmö/Lund, Sweden. The study was conducted in accordance with the local legislation and institutional requirements.
MT: Writing – review & editing. SF: Writing – review & editing. XW: Writing – review & editing. GP: Writing – review & editing. MP: Writing – original draft, Writing – review & editing. AS: Writing – review & editing.
The author(s) declare that financial support was received for the research and/or publication of this article. This work was supported by grants from the United States Department of Defense (No. W81XWH-22-1-0783), Swedish Research Council (project 2017–02341, 2020–02016, 2021–06388), Edvard Welanders Stiftelse and Finsenstiftelsen (Hudfonden), the Royal Physiographic Society, Österlund and Mats Paulsson Foundations, Clas Groschinskys minnesfond, Vinnova, and the Swedish Government Funds for Clinical Research (ALF).
The authors thank Elzbieta Eriksson for assistance with animal care and Congyu Luo for excellent help with microbiological assays.
AS is a co-founder and shareholder of Xinnate AB, a company developing TCP-25-based therapies for wound indications. GP was employed part-time (20%) by Xinnate AB.
The remaining authors declare that the research was conducted in the absence of any commercial or financial relationships that could be construed as a potential conflict of interest.
The authors declare that no Gen AI was used in the creation of this manuscript.
All claims expressed in this article are solely those of the authors and do not necessarily represent those of their affiliated organizations, or those of the publisher, the editors and the reviewers. Any product that may be evaluated in this article, or claim that may be made by its manufacturer, is not guaranteed or endorsed by the publisher.
The Supplementary Material for this article can be found online at: https://www.frontiersin.org/articles/10.3389/fmed.2025.1561732/full#supplementary-material
1. Gould, L, Abadir, P, Brem, H, Carter, M, Conner-Kerr, T, Davidson, J, et al. Chronic wound repair and healing in older adults: current status and future research. Wound Repair Regen. (2015) 23:1–13. doi: 10.1111/wrr.12245
2. Boyko, TV, Longaker, MT, and Yang, GP. Review of the current Management of Pressure Ulcers. Adv Wound Care. (2018) 7:57–67. doi: 10.1089/wound.2016.0697
3. Dana, AN, and Bauman, WA. Bacteriology of pressure ulcers in individuals with spinal cord injury: what we know and what we should know. J Spinal Cord Med. (2015) 38:147–60. doi: 10.1179/2045772314Y.0000000234
4. Dunyach-Remy, C, Salipante, F, Lavigne, JP, Brunaud, M, Demattei, C, Yahiaoui-Martinez, A, et al. Pressure ulcers microbiota dynamics and wound evolution. Sci Rep. (2021) 11:18506. doi: 10.1038/s41598-021-98073-x
5. Nunan, R, Harding, KG, and Martin, P. Clinical challenges of chronic wounds: searching for an optimal animal model to recapitulate their complexity. Dis Model Mech. (2014) 7:1205–13. doi: 10.1242/dmm.016782
6. Saeed, S, and Martins-Green, M. Assessing animal models to study impaired and chronic wounds. Int J Mol Sci. (2024) 25:3837. doi: 10.3390/ijms25073837
7. Pignet, AL, Schellnegger, M, Hecker, A, Kamolz, LP, and Kotzbeck, P. Modeling wound chronicity in vivo: the translational challenge to capture the complexity of chronic wounds. J Invest Dermatol. (2024) 144:1454–70. doi: 10.1016/j.jid.2023.11.024
8. Mohammad, H, Abutaleb, NS, and Seleem, MN. Auranofin rapidly eradicates methicillin-resistant Staphylococcus Aureus (Mrsa) in an infected pressure ulcer mouse model. Sci Rep. (2020) 10:7251. doi: 10.1038/s41598-020-64352-2
9. Fila, G, Kasimova, K, Arenas, Y, Nakonieczna, J, Grinholc, M, Bielawski, KP, et al. Murine model imitating chronic wound infections for evaluation of antimicrobial photodynamic therapy efficacy. Front Microbiol. (2016) 7:1258. doi: 10.3389/fmicb.2016.01258
10. Kraft, WG, Johnson, PT, David, BC, and Morgan, DR. Cutaneous infection in Normal and immunocompromised mice. Infect Immun. (1986) 52:707–13. doi: 10.1128/iai.52.3.707-713.1986
11. Stadler, I, Zhang, RY, Oskoui, P, Whittaker, MS, and Lanzafame, RJ. Development of a simple, noninvasive, clinically relevant model of pressure ulcers in the mouse. J Investig Surg. (2004) 17:221–7. doi: 10.1080/08941930490472046
12. Reid, RR, Sull, AC, Mogford, JE, Roy, N, and Mustoe, TA. A novel murine model of cyclical cutaneous ischemia-reperfusion injury. J Surg Res. (2004) 116:172–80. doi: 10.1016/s0022-4804(03)00227-0
13. Strong, AL, Bowles, AC, Mac Crimmon, CP, Lee, SJ, Frazier, TP, Katz, AJ, et al. Characterization of a murine pressure ulcer model to assess efficacy of adipose-derived stromal cells. Plast Reconstr Surg Glob Open. (2015) 3:e334. doi: 10.1097/GOX.0000000000000260
14. Puthia, M, Butrym, M, Petrlova, J, Stromdahl, AC, Andersson, MA, Kjellstrom, S, et al. A dual-action peptide-containing hydrogel targets wound infection and inflammation. Sci Transl Med. (2020) 12:eaax6601. doi: 10.1126/scitranslmed.aax6601
15. Puthia, M, Petrlova, J, Petruk, G, Butrym, M, Samsudin, F, Andersson, MA, et al. Bioactive suture with added innate defense functionality for the reduction of bacterial infection and inflammation. Adv Healthc Mater. (2023) 12:e2300987. doi: 10.1002/adhm.202300987
16. Stromdahl, AC, Ignatowicz, L, Petruk, G, Butrym, M, Wasserstrom, S, Schmidtchen, A, et al. Peptide-coated polyurethane material reduces wound infection and inflammation. Acta Biomater. (2021) 128:314–31. doi: 10.1016/j.actbio.2021.04.045
17. Papareddy, P, Rydengard, V, Pasupuleti, M, Walse, B, Morgelin, M, Chalupka, A, et al. Proteolysis of human thrombin generates novel host defense peptides. PLoS Pathog. (2010) 6:e1000857. doi: 10.1371/journal.ppat.1000857
18. Fayolle, M, Morsli, M, Gelis, A, Chateauraynaud, M, Yahiaoui-Martinez, A, Sotto, A, et al. The persistence of Staphylococcus Aureus in pressure ulcers: a Colonising role. Genes (Basel). (2021) 12:1883. doi: 10.3390/genes12121883
19. Braga, IA, Brito, CS, Filho, AD, Filho, PP, and Ribas, RM. Pressure ulcer as a reservoir of multiresistant gram-negative Bacilli: risk factors for colonization and development of bacteremia. Braz J Infect Dis. (2017) 21:171–5. doi: 10.1016/j.bjid.2016.11.007
20. Wallblom, K, Lundgren, S, Saleh, K, Schmidtchen, A, and Puthia, M. Image-based non-invasive assessment of suction blister wounds for clinical safety and efficacy. Wound Repair Regen. (2024) 32:343–59. doi: 10.1111/wrr.13172
21. Schmidtchen, A, and Puthia, M. Rapid in vitro and in vivo evaluation of antimicrobial formulations using bioluminescent pathogenic Bacteria. Bio Protoc. (2022) 12:e 4302. doi: 10.21769/BioProtoc.4302
22. Livesley, NJ, and Chow, AW. Infected pressure ulcers in elderly individuals. Clin Infect Dis. (2002) 35:1390–6. doi: 10.1086/344059
23. de la Garza-Rodea, AS, Knaan-Shanzer, S, and van Bekkum, DW. Pressure ulcers: description of a new model and use of mesenchymal stem cells for repair. Dermatology. (2011) 223:266–84. doi: 10.1159/000334628
24. Wassermann, E, van Griensven, M, Gstaltner, K, Oehlinger, W, Schrei, K, and Redl, H. A chronic pressure ulcer model in the nude mouse. Wound Repair Regen. (2009) 17:480–4. doi: 10.1111/j.1524-475X.2009.00502.x
25. Maldonado, AA, Cristobal, L, Martin-Lopez, J, Mallen, M, Garcia-Honduvilla, N, and Bujan, J. A novel model of human skin pressure ulcers in mice. PLoS One. (2014) 9:e109003. doi: 10.1371/journal.pone.0109003
26. Takeuchi, Y, Ueno, K, Mizoguchi, T, Samura, M, Harada, T, Oga, A, et al. Development of novel mouse model of ulcers induced by implantation of magnets. Sci Rep. (2017) 7:4843. doi: 10.1038/s41598-017-05250-y
27. Kumar, S, Tan, Y, Yarmush, ML, Dash, BC, Hsia, HC, and Berthiaume, F. Mouse model of pressure ulcers after spinal cord injury. J Vis Exp. (2019):145. doi: 10.3791/58188
Keywords: pressure ulcer, Staphylococcus aureus , TCP-25, wound infection, bioimaging
Citation: Tavecchio M, Fanni S, Wu X, Petruk G, Puthia M and Schmidtchen A (2025) A murine pressure ulcer model for evaluating persistence and treatment of Staphylococcus aureus infection. Front. Med. 12:1561732. doi: 10.3389/fmed.2025.1561732
Received: 16 January 2025; Accepted: 19 March 2025;
Published: 03 April 2025.
Edited by:
Elisa Belluzzi, University of Padua, ItalyReviewed by:
Sueli Fumie Yamada-Ogatta, State University of Londrina, BrazilCopyright © 2025 Tavecchio, Fanni, Wu, Petruk, Puthia and Schmidtchen. This is an open-access article distributed under the terms of the Creative Commons Attribution License (CC BY). The use, distribution or reproduction in other forums is permitted, provided the original author(s) and the copyright owner(s) are credited and that the original publication in this journal is cited, in accordance with accepted academic practice. No use, distribution or reproduction is permitted which does not comply with these terms.
*Correspondence: Manoj Puthia, bWFub2oucHV0aGlhQG1lZC5sdS5zZQ==
†These authors have contributed equally to this work
‡These authors share last authorship
Disclaimer: All claims expressed in this article are solely those of the authors and do not necessarily represent those of their affiliated organizations, or those of the publisher, the editors and the reviewers. Any product that may be evaluated in this article or claim that may be made by its manufacturer is not guaranteed or endorsed by the publisher.
Research integrity at Frontiers
Learn more about the work of our research integrity team to safeguard the quality of each article we publish.