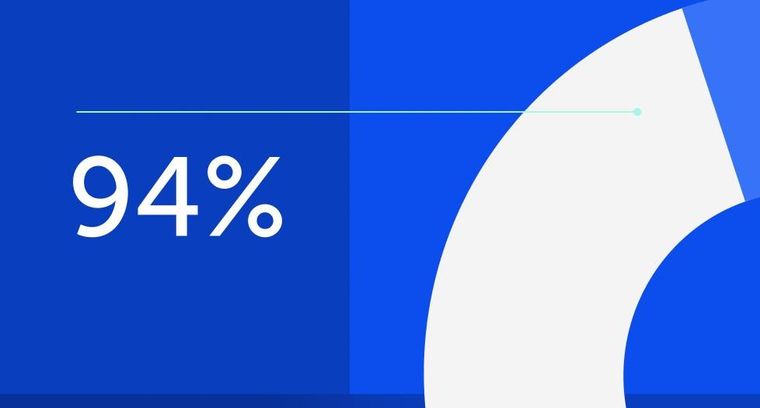
94% of researchers rate our articles as excellent or good
Learn more about the work of our research integrity team to safeguard the quality of each article we publish.
Find out more
REVIEW article
Front. Med., 26 March 2025
Sec. Geriatric Medicine
Volume 12 - 2025 | https://doi.org/10.3389/fmed.2025.1549658
This article is part of the Research TopicNew Insights into Oxidative Medicine: Unraveling the Complexity of Oxidative Stress in Health and DiseaseView all 4 articles
Community-acquired pneumonia (CAP) remains one of the leading respiratory diseases worldwide. With the aging of the global population, the morbidity, criticality and mortality rates of CAP in older adults remain high every year. Modulating the signaling pathways that cause the inflammatory response and improve the immune function of patients has become the focus of reducing inflammatory damage in the lungs, especially CAP in older adults. As an important factor that causes the inflammatory response of CAP and affects the immune status of the body, oxidative stress plays an important role in the occurrence, development and treatment of CAP. Furthermore, in older adults with CAP, oxidative stress is closely associated with immune senescence, sarcopenia, frailty, aging, multimorbidity, and polypharmacy. Therefore, multiple perspectives combined with the disease characteristics of older adults with CAP were reviewed to clarify the research progress and application value of modulating oxidative stress in older adults with CAP. Clearly, there is no doubt that targeted modulation of oxidative stress benefits CAP in older adults. However, many challenges and unknowns concerning how to modulate oxidative stress for further practical clinical applications exist, and more targeted research is needed. Moreover, the limitations and challenges of modulating oxidative stress are analyzed with the aim of providing references and ideas for future clinical treatment or further research in older adults with CAP.
Community-acquired pneumonia (CAP) refers to the infectious inflammation of the lung parenchyma (including the alveolar wall, i.e., pulmonary interstitium in general) acquired outside of hospitals, including pneumonia caused by pathogens with proven latency (1). CAP is one of the most common acute respiratory infections worldwide and can be caused by bacteria, viruses, fungi, or other pathogens alone or in combination. The common causative agents of CAP may vary from country to country, but the older adults and children have always been the primary groups of victims (2, 3). In particular, the global pandemic of coronavirus disease 2019 (COVID-19) has put unprecedented pressure on healthcare worldwide and incurred non-negligible medical costs (4). Oxidative stress, as one of the pathogenic mechanisms of CAP, has been widely demonstrated to be closely linked to inflammation and the immune response (5–7). In recent years, most studies related to the treatment of CAP have focused on reducing inflammation and oxidative damage in the lungs. However, immunosenescence, sarcopenia, frailty, multimorbidity, polypharmacy, and other aging-related disease risk factors are also present in older CAP patients. The impact of these factors on the development, treatment and prognosis of CAP in the older individuals and their relationship with oxidative stress are not sufficiently clear. In addition, the potential value and advantages of modulating oxidative stress compared with the traditional preventive and therapeutic modalities of CAP have rarely been mentioned. Therefore, in conjunction with the above unique disease manifestations present in the older population, this review on more links between oxidative stress and CAP in older adults aims to further clarify the possible benefits of modulating oxidative stress in older adults with CAP patients, with the goal of providing a reference for the treatment of CAP in older adults and related research in the future.
The lung is a complex microbial ecosystem. The microbes are in complex relationships with each other and with the host, in a state of mutual adaptation and dynamic interaction. Currently, it is recognized that most pathogens invade through the respiratory tract, and infection occurs when host defenses are compromised and/or when the host is exposed to highly virulent microorganisms or large amounts of inoculum. After entering the nasopharynx, pathogens escape recognition and clearance from host immune cells by mimicking the host molecular structure or altering their own antigens, escaping the mucus and adhering to the upper respiratory epithelium (8, 9).
The manner in which bacteria, viruses and fungi induce an immune response after invasion is somewhat different (Figure 1). When bacteria invade the alveoli and then multiply, they are sensed and recognized by polymorphonuclear leukocytes (neutrophils), which in turn elicits the body's innate immune response. Bacteria interact with alveolar cells, such as alveolar epithelial cells and macrophages, which secrete cytokines and neutrophil chemokines and subsequently recruit additional immune cells from the pulmonary circulation to the site of infection. These immune cells produce proteases, reactive oxygen species (ROS) and reactive nitrogen species (RNS) and act on infected cells to induce necrotic cell death (10). For viral antigens, the adaptive immune response is activated mainly by the encounter of viral particles with antigen-presenting cells or B-cell receptors and further induces a variety of immune cell interactions and the production of cytokines, chemokines, etc., to inhibit viral replication and transmission and protect the host from viral attack (11, 12). Certainly, viruses also evade immune cell recognition and antiviral responses by increasing their affinity for target cells, inhibiting the recognition of relevant receptors and disrupting immune signaling pathways. It can even provoke the immune system to attack its own tissues in the form of inducing autoimmune and autoinflammatory processes that allow it to survive in the host (13). For fungi, within the respiratory tree, inhaled fungal cells are opsonized with soluble pattern recognition receptors, surfactants, complement, and antibodies. Mainly cells such as neutrophils, monocytes, monocyte-derived dendritic cells, plasmacytoid dendritic cells (pDCs), and mediators such as cytokines, neutrophil chemotaxis, and interferon are involved in the whole immune process. Among these, pDCs do not bind conidia but enhance the oxidative burst to boost conidial killing in neutrophils (14).
Figure 1. Bacterial, viral, and fungal infections trigger immune and inflammatory responses and the process of ROS generation.
Certainly, there are many other disease-causing pathogens in CAP, but the same is true for the various immune responses of the organism to pathogens, which are usually beneficial to the host (15–17). However, some studies have noted that overzealous activation of the innate immune system can lead to immunopathology, which may result in poor outcomes and even contribute to secondary bacterial infections in the lower respiratory tract, which can lead to pneumonia (18–20). The occurrence of such an excessive immune response may be due to the uncontrolled production of inflammatory cytokines, which trigger the release of large amounts of inflammatory mediators and excessive production of ROS, which exacerbates or prolongs the stimulation and directly or indirectly leads to cell and tissue death and widespread lung injury, thus increasing the burden of CAP (21). Thus, there is still a delicate balance that needs to be regulated between appropriate immunoprotection and excessive pathologic immunity.
The new idea holds that the various microorganisms in the lungs have a complex relationship with the host and with each other that is analogous to an ‘adaptive island', where they maintain a dynamic equilibrium and constraints (22). On the basis of this theory, regardless of whether a new pathogen invades, disrupting the balance of existing microorganisms can lead to lung infections. The immune and inflammatory responses of an organism are measured to maintain this balance. Although the whole process is provoked by the pathogen, preinfectious susceptibility to pneumonia, the host's own characteristics, and host–pathogen interactions also play a catalytic role in the onset of CAP and the activation of the immune response (23, 24).
Compared with healthy young adults, it is commonly agreed that older adults have characteristic elevated serum levels of proinflammatory markers (25). During aging, the levels of inflammatory factors and serum proinflammatory markers increase significantly, even in older adults without chronic diseases (26). This persistent inflammation, also known as low-grade inflammation, drives the onset of age-related diseases and the exacerbation of chronic conditions such that older adults are more likely to have significant lung inflammation and lung tissue damage (27, 28). In short, multiple components, such as pathogenic microorganisms, inflammatory responses, and immune resistance, are combined to determine whether CAP occurs and how it progresses or subsides. And the impacts on older adult patients are particularly significant and complex.
Oxidation and antioxidation are two systems in the body that are interdependent but also mutually restrictive, and oxidative stress is the result of a disruption of the pro-oxidant–antioxidant balance and the consequent accumulation of ROS (29). ROS are highly reactive active molecules produced by the interaction of oxygen with certain elements; they hold at least one unpaired electron in their outer shell and are therefore extremely unstable (30). ROS can either originate from outside the body or be produced by certain oxidizing enzymes in the body. However, whether endogenous or exogenous, major cellular macromolecules such as nucleic acids, lipids and proteins can be oxidatively modified under the effect of ROS, which can lead to structural and functional alterations in cells (31). Therefore, ROS can be involved in both physiological apoptosis and pathological cell death.
Fortunately, the innate cellular antioxidant system also blocks overly intense inflammatory responses and prevents excessive ROS accumulation, thus limiting damage and inhibiting disease (32). Antioxidant defenses can be divided into non-enzymatic and enzymatic antioxidants. Non-enzymatic antioxidants include those naturally produced by cells, such as glutathione (GSH), coenzyme Q10 (CoQ10), and melatonin, and non-naturally produced antioxidants, such as vitamin C (VitC), vitamin E (VitE) and flavonoids (33). They are characterized by the ability to act directly and quickly inactivate free radicals and oxidants (34). GSH is an essential and important non-enzymatic antioxidant in mammalian cells. It is not only used directly as an antioxidant to protect cells from free radicals and pro-oxidants but also as a cofactor for antioxidant and detoxification enzymes such as glutathione peroxidase (GPX), glutathione S-transferase and glyoxalase (35). The main antioxidant enzymes include catalase (CAT), superoxide dismutase (SOD), GPX, etc. O2 is converted to H2O2 by SOD, which is then broken down by CAT into O2 and H2O, thereby preventing the generation of hydroxyl radicals. GPX converts peroxides and hydroxyl radicals to non-toxic forms by oxidizing GSH to glutathione disulfide, which is then reduced to GSH by glutathione reductase (36, 37).
The antioxidant response is modulated mainly by the Nrf2/Keap1 system (Figure 2). Nrf2 (NF-E2-related factor 2) is a transcription factor, whereas Keap1 (kelch-like ECH-associated protein 1) is an Nrf2 repressor. Under physiological conditions, Keap1 acts as a negative regulator of Nrf2, locking Nrf2 in the cytoplasm and preventing it from entering the nucleus. On the other hand, Keap1 mediates the ubiquitination-mediated degradation of Nrf2 to maintain intracellular Nrf2 homeostasis. When stimulated by ROS, the cysteine residues on Keap1 are modified by ROS, and Nrf2 dissociates from Keap1. The activated Nrf2 enters the nucleus and binds to the Maf protein to form a heterodimer. Binding to antioxidant response elements (AREs) induces the transcription of genes regulated by AREs, which initiates the expression of genes encoding cytoprotective enzymes, such as antioxidative stress proteins, especially antioxidant enzymes (38). However, upon exposure to oxidative stress, Keap1 loses the ability to ubiquitinate Nrf2, leading to disruption of the redox balance, which may induce damage to cellular mitochondria, proteins, DNA, etc. (39).
The antioxidant response is also modulated by signaling pathways mediated by adenosine 5'-monophosphate-activated protein kinase (AMPK). AMPK is a serine/threonine kinase expressed in all eukaryotic tissues and organs, playing a key role in adenosine triphosphate (ATP) synthesis (40). As a central cellular energy sensor, AMPK becomes activated during metabolic perturbations including oxidative stress and inflammatory conditions (40–42). Previous study has shown that AMPK plays cytoprotective roles in glucose and lipid metabolism and protein synthesis (43). AMPK activation also preserves mitochondrial function by stabilizing redox homeostasis and membrane potential, thereby suppressing apoptosis (43). Key AMPK-dependent transcription factors, including Forkhead box O3a (FoxO3a), Nrf2, and Sirt1, coordinate the expression of antioxidant defense genes (44–46). Under hypoxia, mitochondria induce hypoxia-inducible factor 1α (HIF-1α) expression, a process accompanied by ROS production (47). To modulate cellular energy homeostasis, AMPK enhances FoxO3a transcription (48). Induced FoxO3a mitigates increased ROS levels in hypoxic cells by decreasing HIF-1α accumulation, exerting anti-oxidative stress effects, and altering the cellular hypoxic response (49). AMPK activation directly causes Nrf2 to migrate and aggregate into the nucleus and indirectly increases nuclear Nrf2 levels, thereby promoting antioxidant enzyme gene expression to combat oxidative damage (32). Furthermore, AMPK increases Sirt1 activity in cells with oxidative stress-induced senescence (50, 51). The sirtuin protein family, particularly Sirt1, acts as a key epigenetic regulator of aging, exerting protective effects against sarcopenia and frailty in older adults and promoting longevity (52). Sirt1-mediated deacetylation of peroxisome proliferator-activated receptor γ coactivator-1α (PGC-1α) enhances mitochondrial stabilization in skeletal muscle, as shown in in vitro and in vivo models (53). Meanwhile, Sirt1 exerts anti-oxidative stress effects by enhancing the expression of antioxidants such as MnSOD, CAT, and GPX through deacetylation and activation of pathways like PGC-1α and FoxO3a (52). Conversely, Sirt1 inhibition increases ROS production (54). In conclusion, cellular defense against ROS overload involves coordinated mechanisms, with antioxidant enzyme systems playing a pivotal role in ROS scavenging. Furthermore, antioxidant enzymes have repair mechanisms for free radical-induced damage. It can repair damaged DNA and proteins, fight against oxidized lipids, stop chain propagation of peroxyl lipid radicals, and repair damaged cell membranes and molecules (55).
Oxidative stress is an important component of the innate immune system and is part of the defense mechanism against pathogens (56). On the one hand, oxidative stress is considered to be one of the pathogenic mechanisms of CAP and is closely related to inflammation (57). Numerous studies have shown that oxidative stress is greater in patients suffering from CAP than in healthy volunteers (6, 58, 59). Indeed, after the onset of pneumonia, immune cells respond by releasing ROS and inflammatory factors at the site of infection. For example, macrophages are major contributors to the immune response. Upon activation, macrophage TXNIP protein induces ROS production, causing endoplasmic reticulum (ER) stress. This, in turn, triggers cellular signaling processes, including redox homeostasis, inflammation, and immune responses (60). During ER stress, stimulator of interferon genes (STING), a transmembrane protein predominantly located in the ER, responds to stress signals and promotes inflammasome activation, such as NLRP3, via the STING/TANK-binding kinase 1 (TBK1) pathway, leading to tissue inflammation (61). Neutrophils, another key cell type involved in killing bacteria and other microorganisms, activate NADPH oxidase (NOX2) on their cell membranes upon stimulation. This generates large amounts of superoxide and ROS, which react with pathogens in phagosomes and trigger an inflammatory response (62). In contrast, lung cells respond to oxidative responses by activating nuclear factor kappa-light-chain-enhancer of activated B cells (NF-κB) and activating protein-1 (AP-1), which inhibits oxidative stress while also stimulating the release of cytokines such as tumor necrosis factor-α (TNF-α) (57, 63). In this way, while maintaining the balance of the redox system, lung cells are able to generate a certain inflammatory response to trigger an optimal immune response. A review states that ROS generated by immune and inflammatory responses in tissues and organs act as potent killers of various pathogens, and controlled accumulation of ROS serves as an effective weapon against pathogen invasion (32). For example, in neutrophils, pathogens are captured and internalized via phagocytosis, forming membrane-enclosed phagosomes (64). Within these phagosomes, pathogens are exposed to bactericidal peptides, destructive enzymes, and ROS, leading to significant degradation (62). However, once uncontrolled oxidative stress occurs and cytokines are overproduced, not only can the inflammatory state of the lungs be exacerbated, but more ROS will continue to be produced, worsening the situation (Figure 3).
Figure 3. (a) Mechanistic models of the reciprocal modulation of the inflammatory response and oxidative stress. (b) Vicious cycle of uncontrolled oxidative stress and an uncontrolled inflammatory response and strategies to address this cycle.
Notably, if the homeostasis of the redox system is persistently disrupted, it also weakens the innate and acquired immune response, exacerbates bacterial and viral infections, causes cellular and tissue damage, and drives the progression of CAP. Specific modes of damage include affecting the CD4+/CD8+ T-cell ratio, impairing natural killer cell function and neutrophil migration/phagocytosis, and reducing the stability of the macrophage cytoskeleton to affect phagocytosis (32). At the molecular level, excess ROS can react rapidly and non-specifically with chemical groups in DNA, lipids and proteins, leading to cellular metabolic disorders, loss of function and abnormal apoptosis (65). If a vicious cycle of uncontrolled oxidative stress and an uncontrolled inflammatory response further develops, more trauma to cells and tissues can occur, leading to a poor CAP outcome. For example, when a respiratory viral infection is combined with invasive fungal disease, cytokines attract more immune cells, which in turn produce more cytokines, creating an inflammatory cycle that damages lung tissues and causes the formation of fibrin and scar tissue, which allows fluids to seep into and fill the lung cavities, leading to respiratory failure (66). However, causing oxidative stress is not always detrimental. An early review revealed that oxidative stress is associated with persistent bacterial infections and antibiotic resistance. Pathogenic bacteria may undergo physiological changes by adapting to oxidative stress, either by promoting bacterial survival during antibiotic exposure or by influencing the oxidative stress response mechanism, thereby reducing the effective intracellular concentration of the antibiotic and allowing the bacteria to survive (67).
Beyond genetically resistant bacteria, persisters, which are genetically susceptible cells that survive antibiotic doses that kill the rest of the clonal population (68). These persisters are non-growing (NG) bacteria that coexist with their growing counterparts but are transiently insensitive to antibiotics (69). They are thought to resume growth after antibiotic treatment ceases, leading to chronic infections, recurrences, and subsequent treatment failure (70, 71). Altered adaptation to oxidative stress is a major driver of antibiotic resistance in these persisters (72, 73). A recent research has shown that in vitro treatment of stationary-phase Staphylococcus aureus (S. aureus) with fluoroquinolones (FQ) enhances FQ lethality by stimulating ROS production in cells (74). Thus, enhancing oxidative stress might be valuable in cases of persistent bacterial infections and/or antibiotic resistance. However, the role of ROS in controlling bacterial infections and mediating bacterial responses to antibiotics is complex. Studies indicate that in the case of the major Gram-positive pathogen, S. aureus, ROS are inhibitory to antibiotic mediated killing and their generation by the host increases S. aureus antibiotic tolerance during infection (75, 76). Another study found that after engulfment by macrophages, sustained production of RNS by host cells locks persisters in a NG state via tricarboxylic acid-cycle intoxication, thereby poisoning bacterial respiration. Chemical inhibition of RNS production can stimulate persisters growth resumption, thereby resensitizing them to the action of bactericidal antibiotics and improve the effectiveness of treatment (73). Therefore, given that anti-infection therapy remains the core treatment for CAP, it is crucial to modulate oxidative stress to address the diverse bacterial responses to antibiotics.
Viral infections are associated with reduced GSH levels, which cause a cellular redox imbalance and activate a vicious cycle of reduced host defenses, leading to a severe imbalance in the intracellular microenvironment, and adjunctive antioxidant treatment may improve the outcomes of antiviral therapies (77). In fungal infections, an emerging view is that ROS production, regulation, and response are central axes of the host–pathogen interaction (78). Both the host and fungi produce ROS, use conserved mechanisms to detoxify ROS, and leverage ROS in their local environment to mediate defense mechanisms. In conclusion, oxidative stress is one of the important mechanisms in the development and progression of CAP, and the inflammatory response caused by lung lesions can exacerbate oxidative stress. On the basis of this situation, theoretically, exogenous supplementation with antioxidants is necessary along with the modulation of the immune and inflammatory responses. Moreover, an initial trial demonstrated that altered oxidative stress status is associated with the severity of CAP (5). Consequently, antioxidant therapy has been proposed as a supplementary treatment option.
Global aging is already a reality today, putting enormous pressure on every country's healthcare system and representing an enormous challenge to global public health. The number of intensive care unit hospitalizations due to CAP have been rising steadily in recent years, especially in the older population, where CAP is a common cause of hospitalization, readmission, and death (79). A survey indicated that nearly 1 million older people in the U.S. are hospitalized for CAP each year, and nearly one-third of them die within 1 year; the burden of CAP in the older population is enormous (80). Despite geographic differences, Streptococcus pneumoniae remains a major pathogen for all ages worldwide (81, 82). And older adults are also more vulnerable to the growing epidemic caused by viruses (83). Compared with younger patients, older patients have an increased risk of infection with other pneumonia pathogens, and infections with influenza virus and antibiotic-resistant pathogens should also be considered. However, the incidence of so-called atypical pathogens is relatively low, and Mycoplasma infections are particularly rare (84). Early and appropriate anti-infective therapy remains a core part of the CAP treatment regimen. However, inappropriate antibiotic use is common in older adults (>65 years old), and older adults are particularly vulnerable to severe antibiotic-related adverse effects (85). Moreover, bactericidal antibiotics (quinolones, β-lactams, and aminoglycosides) have been found to cause ROS overproduction and mitochondrial dysfunction in mammalian cells, leading to oxidative damage to membrane lipids, proteins, and DNA (86).
Additionally, the aging process is accompanied by chronic inflammation and immune senescence (87). It has already been made clear that CAP is closely related to the immune response and inflammation, and the presence of an inadequate immune response together with an inflammatory flare-up can lead to a worse outcome (88). Therefore, older patients are at greater risk. On the basis of these findings, clinical intervention involving immunomodulation and suppression of the inflammatory response in older adults with CAP, such as the use of immunoglobulins and cortisol, would theoretically be beneficial but requires careful evaluation before use and has limited applicability (89, 90). Attention should also be paid to the fact that inappropriate treatment of patients hospitalized with CAP prolongs hospitalization and increases costs and mortality rates (82). In addition, with respect to older age groups, the fact that the nutritional status of older age groups, multiple age-related diseases, and chronic underlying conditions all have an impact on the onset and progression of CAP in older adults cannot be ignored (84). The positive effects of modulating oxidative stress in sepsis, acute respiratory distress syndrome (ARDS) and acute lung injury have been clinically demonstrated. It improves oxygenation rates and GSH levels and strengthens the immune response, and it reduces mechanical ventilation time, the length of stay in the intensive care unit, multiple organ dysfunctions, the length of stay in the hospital and mortality rates in acute lung injury/ARDS patients (84).
In conclusion, oxidative stress is involved in several aspects of CAP, including immunity and inflammation. Given the special risk factors and manifestations of CAP in older individuals and the existing studies, modulating oxidative stress may have the following advantages and application value (Table 1).
Table 1. Summary of pilot studies on vitamins C and E, NAC, CoQ10, and melatonin discussed in this article.
Regardless of what disease you are facing, the first tool to consider should be prevention. Vaccines, as the only effective preventive medical treatment, can reduce the risk and burden of contracting diseases such as influenza and pneumococcal pneumonia (120). An analysis of national pneumococcal vaccination data in Korea revealed that previous pneumococcal vaccination in the older population effectively improved hospitalization rates and 30-day mortality among patients hospitalized for pneumonia (121). However, the outcomes of pneumococcal vaccine use in older adults remain controversial. A retrospective study in Japan concluded that the effectiveness of pneumococcal vaccines was significant in people aged 65–85 years but not in the older population (122). However, vaccine coverage is not satisfactory, and increasing vaccination rates remains a difficult challenge (123). Moreover, vaccines do not cover all pathogens and take a certain amount of time to develop. When faced with an outbreak of a worldwide disease such as COVID-19, the use of a vaccine can be caught off guard (124).
Oxidative stress is one of the important mechanisms in the development of CAP, and maintaining the balance of the oxidative state in the body may be able to produce a preventive effect through a different mode of action than vaccines do. Moreover, in the older population, there is a low-grade chronic inflammatory state formed by senescent cells stimulating the secretion of proinflammatory cytokines. Individuals with low-grade chronic inflammation present a dysregulated innate immune system, resulting in an increased risk of infection (125). Meanwhile, the subsequent inflammatory cascade further increases extracellular ROS concentrations and oxidative stress (126). From this perspective, modulating oxidative stress can also play a role in preventing the onset and controlling the progression of CAP in older individuals by controlling chronic inflammation and reducing the accumulation of ROS.
For example, vitamins C and E are the most common antioxidants. VitE is a fat-soluble antibiotic whose main role is to prevent lipid peroxidation, which minimizes the formation of secondary radicals. Alpha-tocopherol is the most potent antioxidant in the VitE composition and can react quickly with free radicals to generate relatively stable tocopheroxyl radicals, thus improving oxidative stress (33). An earlier prospective cohort study also confirmed that VitE supplements prevented rehospitalization in patients (>65 years old) who were first hospitalized for CAP (98). In another follow-up study, VitE (50 mg/d) was administered for 5–8 years to older male smokers (50–69 years old), which revealed that VitE administration reduced the incidence of pneumonia in older men in different subgroups (99). However, some studies have noted that the effect of VitE on the incidence of pneumonia does not seem to be completely uniform (127–129). An early clinical trial found that VitE (50 mg/day) did not affect pneumonia risk in participants with physically demanding jobs but reduced the risk by 50% in those engaging in moderate or heavy exercise during leisure time (129). Another study found that VitE reduced the risk of pneumonia by 69% in participants who smoked the least and exercised in their leisure time but increased the risk of pneumonia by 68% in participants who smoked the most and did not exercise (130). However, the study focused only on Finnish male smokers aged 50–69 years, and long-term high-frequency smoking is more directly and detrimentally associated with the development of pneumonia.
VitC can directly quench free radicals in the aqueous layer and also cooperate with VitE to regenerate α-tocopherol from α-tocopherol radicals in membranes and lipoproteins and increase GSH levels in the cell; thus, it plays an important role in protein thiol group protection against oxidation (33). One study reported that VitC can effectively kill bacteria, mycobacteria, HIV, and HCV because it can generate free radicals and H2O2 (131). As such, VitC plays a number of important roles in reducing oxidative stress caused by infection, balancing the immune system, and killing microorganisms by generating free radicals. However, the role of VitC in preventing pneumonia is not very certain and is still only a reliable speculation that lacks confirmation from more reliable and relevant experimental studies. In an earlier study, 674 Marine Corps recruits received 2 g/day of VitC for 8 weeks. The study found that VitC did not significantly alter the incidence or duration of colds, but it did reduce the severity of cold symptoms in the treatment group (91). A recent meta-analysis emphasized that the current evidence is insufficient to affirm the efficacy of VitC supplements in preventing pneumonia because of the small number of trials and the very low quality of the available results (132). However, a recent review confirmed the preventive role of VitC in viral pneumonia through a different lens. Owing to pneumonia, viral infections can reduce the level of ascorbic acid because they create oxygen (O) and nitrogen (N) species (133). Therefore, VitC can prevent pneumonia. In addition, an analysis of nationally representative data from more than 34,000 participants revealed that low serum levels of antioxidant vitamins were associated with increased respiratory morbidity and/or mortality in U.S. adults (134). These results underscore the importance of antioxidant vitamins in respiratory health.
There are many other important antioxidants, including the previously mentioned GSH, CoQ10, which is present in all membranes of the cell, and melatonin. The powerful antioxidant effects of GSH are not repeated. Increasing GSH levels for the prevention and inhibition of COVID-19 are also widely recognized (135). N-Acetyl-L-cysteine (NAC) is a precursor of reduced GSH. NAC has better oral and topical bioavailability than GSH, and has an excellent safety profile and is also a mucolytic agent (136). NAC is able to act as a potent antioxidant scavenger of free radicals directly but also reduces downregulated inflammation and lowers the level of oxidative stress through stimulation of Nrf2 and inhibition of the NFκB pathway (137). NAC, a pleiotropic drug, has been proposed for the treatment and/or prevention of a variety of diseases involving GSH depletion and altered redox status. For example, severe acute respiratory syndrome coronavirus 2 (SARS-CoV-2) infection impairs the metabolism of cellular GSH, and NAC can prevent such defects in vitro (107).
CoQ10 has potent antioxidant effects on the mitochondrial membrane, as well as other membranes in the cell and in the plasma and cytoplasm. Its most important and relevant role is the ability to switch between the redox forms (ubiquinone, semiubiquinone, and ubiquinol) and maintain a balance between the redox forms outside the mitochondria through enzymatic action (138). A molecular review revealed that CoQ10, which reduces important inflammatory cytokines and prevents organ damage due to massive oxidative stress, has been tested for the prevention of a wide range of diseases, especially those with an inflammatory pathogenesis. CoQ10 supplementation could prevent COVID-19-induced morbidities and potentially has a protective role against the deleterious consequences of the disease (139).
Melatonin is secreted mainly by the pineal gland of the brain, and its secretion is extremely high in infants and adolescents and much lower in older individuals. Melatonin scavenges free radicals directly and also stimulates a variety of antioxidant enzymes, including SOD, GPX, glutathione reductase, and CAT, inhibits pro-oxidant enzymes and increases intracellular GSH levels. There is also evidence that melatonin stabilizes cell membranes, which may help fight oxidative damage (33). A review summarizes the potent bacteriostatic effects of melatonin, such as resistance to pathogenic bacterial infections in vivo via various pathways, including NF-κB and ROS; antimicrobial activity against classical gram-negative and gram-positive bacteria, even members of other bacterial groups such as Mycobacterium tuberculosis; and antiviral effects (140). Although there is no experimental confirmation of the effectiveness of the antioxidant effects of melatonin in the prevention of CAP. However, melatonin has demonstrated satisfactory results in terms of being a possible prophylactic measure for COVID-19 infection (141). This may be related to its ability to reduce mitochondrial oxidative stress in posterior lung cells after COVID-19 infection while conferring a general antioxidant effect (142). A retrospective cross-sectional study analyzed data from a closed population of 110 adult patients treated with melatonin for a variety of sleep disorders until the onset of the COVID-19 pandemic (143). The patients in general were mostly older adults treated with a mean dose of melatonin >40 mg/day for various sleep disorders, mainly for complaints of insomnia, for more than 12 months. COVID-19 infection was recorded in 15 patients (13.5%) requiring hospitalization, 5 of whom were infected, only one with severe pneumonia and no deaths due to COVID-19. These results are consistent with a possible preventive effect of melatonin in the context of the COVID-19 pandemic.
The previously mentioned representative antioxidants, while playing an antioxidant role, are also nutrients needed by humans and can indirectly improve the state of malnutrition in the older population, further affecting immune function and playing a preventive role (65). Certainly, the modulation of oxidative stress works in an indirect, slow, and gentle manner as opposed to the direct stimulation of the immune response by vaccines. In addition, some vaccines produce effects by exerting antioxidative stress effects (144). Theoretically, vaccines are synergistic with antioxidative stress, and the significance and value of modulating oxidative stress in preventing CAP in older individuals may be better realized on the basis of the low-grade chronic inflammatory state and the chronic accumulation of ROS in older individuals. However, because we have only just experienced COVID-19, there will be a relatively greater number and depth of articles on prophylactic research related to viral CAPs such as pneumonia with COVID-19. This enthusiasm should be maintained for research on other pathogens, including bacteria and fungi. The more we understand about biology, the better we are equipped to meet sudden public health challenges such as the COVID-19 pandemic (145).
Inflammation and oxidation are apparently interrelated processes, as excessive or uncontrolled free radical production induces an inflammatory response, while free radicals are inflammatory effectors. In fact, both oxidation and inflammation occur when the immune system responds to pathogen invasion (146). An analysis of endogenous oxidative damage markers and their associations with pulmonary involvement severity in patients with SARS-CoV-2 pneumonia indicated that SARS-CoV-2 pneumonia is significantly associated with increased endogenous oxidative damage. Oxidative damage seems to be associated with the severity of pulmonary involvement (147). Many compounds with antioxidant effects, such as those mentioned above, that protect lung tissue from CAP by attenuating oxidative stress-induced damage and inhibiting inflammatory responses have been identified.
According to the results of an animal study in rats, the oral administration of antioxidant vitamins such as VitE and VitC may help inhibit the development of ARDS (104). An early randomized, double-blind trial of 57 older adult patients admitted to the hospital for acute respiratory infections (bronchitis and bronchopneumonia) using a clinical scoring system based on major symptoms of the respiratory condition revealed that VitC-supplemented patients fared significantly better than placebo-supplemented patients did (92). An in vitro assay confirmed that VitC reduced ROS and DNA damage in peripheral blood mononuclear cells and decreased TNF-α and IL-6 levels in whole blood cells from patients with severe CAP (93). A research has found that COVID-19 patients have an average plasma VitC concentration nearly five times lower than that of healthy volunteers. Therefore, it is recommended that COVID-19 patients receive VitC supplementation at a dose of 100 mg/kg per day (94). NAC is a safe, tolerable, available and affordable drug which seems like a promising adjuvant therapeutic agent for COVID-19 (108). In animal experiments, NAC was found to attenuate lipopolysaccharide-induced inflammation in bovine embryonic tracheal cells and lung injury in mice (109). In the clinical trial, the group of COVID-19 patients treated with NAC had a greater risk at baseline, such as being older (average age over 70) and suffering from more comorbidities such as hypertension, diabetes, and COPD. However, patients who received NAC had a significantly lower mortality rate than did COVID-19 patients who did not receive NAC, which was attributed to the antioxidative stress effect of NAC (110). Treatment with NAC in critically ill patients with COVID-19 (mean age >60 years in both experimental and control patients) improved patients' PaO2/FiO2 indices, and it was hypothesized that NAC prevented the reduction in GSH and thus affected clinical outcomes (111). In mouse experiments, CoQ10 significantly inhibited Pseudomonas aeruginosa-induced cell death; thus, antioxidants such as CoQ10 have been proposed for the treatment of pneumonia caused by Pseudomonas aeruginosa infection (112). CoQ10 treatment in older adults with CAP accelerated symptom resolution, shortened the duration of antibiotic therapy, and also performed well in the subgroup of patients with severe pneumonia (113). In cellular experiments, melatonin can upregulate telomerase activity in macrophages by decreasing ROS levels, increasing cellular energy production, and inhibiting NLRP3 inflammatory vesicle activation in macrophages, suggesting that melatonin has therapeutic potential for pneumonia (114). In clinical trials, adjunctive treatment with melatonin has demonstrated efficacy in patients with mild, moderate, and severe COVID-19 (115, 116). Melatonin can be used as a medicinal adjuvant with an anti-inflammatory mechanism to reduce and control inflammatory cytokines by regulating the expression of T helper 1 (Th1) and Th2 regulatory genes in patients with COVID-19 (117). The results of a recent clinical trial revealed that melatonin restores redox homeostasis, which is altered in COVID-19 patients and can be used as an adjuvant therapy for SARS-CoV-2 infection (118).
Indeed, modulating oxidative stress plays a role primarily in controlling the inflammatory response component of CAP. A range of lung damage caused by inflammation and oxidative stress can be reduced, which can improve clinical symptoms in older patients. Previous studies have theoretically and experimentally demonstrated the positive therapeutic effects of modulating oxidation in viral CAPs, such as COVID-19, but there is a relative lack of more relevant clinical studies to further clarify the clinical efficacy in CAP infection caused by other pathogens.
With respect to older adults, the topic of aging, especially immune aging, cannot be avoided. The immune system is widely believed to undergo quantitative and qualitative changes during the process of aging (148). This age-related decline in immune function, called immune senescence, leads to alterations in the cytokine microenvironment as well as impaired innate and adaptive immunity and is closely related to oxidative stress (149). According to existing studies, immune senescence is characterized mainly by major features, such as thymic degeneration, changes in T-cell populations, senescent secretory phenotypes, dysregulation of immune responses, and metabolic and epigenetic changes, which affect cardiovascular diseases, neurodegenerative diseases, COVID-19, autoimmune diseases, and cancer[(150–154), Figure 4].
Figure 4. Main features of immunosenescence and the major cells and diseases affected. Thymic degeneration: The thymus gland is essential for T-cell development, but it gradually shrinks and degenerates with aging, leading to a decrease in the production of new T cells. T-cell population changes: An increase in memory T cells and a decrease in initial T cells weaken the ability to respond to new antigens. Senescence-associated secretory phenotype (SASP): This phenotype promotes cellular senescence, including immune cells, and promotes the secretion of proinflammatory cytokines, chemokines, and proteases that lead to chronic inflammation (inflammatory aging). Immune response dysregulation: Decreased immune surveillance, poor response to vaccines, increased susceptibility to infections, and increased incidence of autoimmune diseases and cancers. Metabolic and epigenetic changes: Alterations in metabolic and epigenetic pathways significantly affect immune system aging and T-cell senescence.
In general, all immune cells are affected by aging (155). Compromised immune cells lose their ability to modulate their own redox and inflammatory homeostasis, which is characterized by mononuclear immune cells infiltrating different tissues and producing excessive amounts of ROS and proinflammatory mediators (156, 157). High levels of ROS in cells are considered the driving force behind the deleterious effects of the aging process (153). This may lead to impaired phagocytosis of macrophages, decreased production of lymphocyte factors and antibodies, dysregulation of inflammatory processes, and an impaired immune response (87). If these affected immune cells are not well modulated, they further increase oxidative and inflammatory stress, creating a perpetual cycle that increases the rate of aging (158). These immune senescence changes further reduce the ability of the lungs to respond to infection and repair damage, increasing vulnerability to respiratory disease and slowing recovery from illness (159).
In addition, one of the hallmarks of immune senescence is “inflammation,” which refers to an age-related systemic state of sterile, chronic and low-grade inflammation characterized by the upregulation of blood markers of inflammation and is considered to be a consequence of increasing chronological age, as well as a hallmark of biological senescence, multimorbidity and mortality risk (87, 153). And oxidative stress plays an important role in the development and maintenance of low-grade inflammation in age-related diseases. The ROS generated by oxidative stress activate the NF-κB signaling pathway, which drives the expression of many proinflammatory cytokine genes as transcription factors, leading to chronic sterile inflammation and significantly affecting the aging process (160). In fact, unstimulated neutrophils and lymphocytes isolated from older adults patients accumulate greater amounts of ROS, present decreased SOD activity, and are less resistant to cell death compared to those cells obtained from young individuals (161). In contrast, the feedback loop between ROS and NF-κB exacerbates oxidative damage and inflammation, leading to immune cell senescence and a decrease in tissue function. Together, oxidative stress and immune senescence form a vicious cycle in which impaired immune function increases susceptibility to oxidative damage, and oxidative stress further weakens the immune response (162).
In conclusion, the relationships among immune senescence, chronic inflammation and oxidative stress are intertwined and complex. While the links have not been fully elucidated, reducing systemic oxidative stress likely reduces the risk of stress-induced cellular senescence and associated inflammation (87). Notably, immune senescence is particularly detrimental to the respiratory system of older adults, who are easy targets for exogenous infections (162). Furthermore, due to immune senescence, older patients have an inadequate inflammatory response to infection, which may lead to an underestimation of the severity of pneumonia (163).
Some existing studies have also confirmed the effectiveness of counteracting immune senescence from the perspective of modulating oxidative stress. For example, flavonoids, which are natural antioxidants present in various foods, can act as antioxidants through signaling pathways such as the NF-κB pathway (164). Quercetin, a type of flavonoid, has been widely reported for its antioxidant activity (165). In an in vitro cellular assay, aged peripheral blood mononuclear cells treated with quercetin presented a strong proliferative response comparable to that of young cells and increased GSH antioxidant defense levels, significantly reducing the expression of immune senescence markers (166). Another botanical bioflavonoid composed of Scutellaria baicalensis and Acacia catechu improved innate and adaptive immunity through antioxidant effects in a rat model of immune senescence and has great potential for the treatment of respiratory disorders due to immune stress and aging (162). In immunosenescent mice, although not showing any statistical difference, the administration of VitE increased the ratio of naïve to effector-memory T cells, suggesting that VitE may be effective in restoring oxidative stress-induced immunosenescence (101). There are also studies confirming that melatonin supplementation can prevent or delay the deterioration of immune system function that accompanies aging and may restore it to a “youthful” state (149).
Indeed, chronic oxidative stress is only one of the many factors that contributes to immune senescence in the older population. Basic experiments have demonstrated that modulating stress can improve or even reverse immune senescence. However, this is destined to be a long process, and long-term clinical studies are needed to fully substantiate the value of antioxidant therapy.
In the case of older adults with CAP, to seek effective treatment strategies, it is necessary to consider not only pathogen infection but also functional decline. Cough is the most common and primary manifestation of CAP and is important for clearing secretions from the airways. The strength of the cough is regulated by the respiratory muscles, and a weakened cough reflex can lead to the development of pneumonia, especially aspiration pneumonia, in older individuals (167). Therefore, respiratory muscle weakness has also been shown to be a risk factor for the development of pneumonia and for the recurrence of pneumonia in older individuals (168). In addition, the role of the swallowing reflex is also important. It is another protective airway reflex, such as coughing, and its strength is related to the swallowing muscles (169). Both impaired reflexes are recognized as major causes of aspiration pneumonia in older individuals (170). A high-quality review confirmed that aging weakens respiratory and swallowing muscles and causes sarcopenia in multiple ways; thus, older adults with CAP may be more challenging (171). Sarcopenia is an aging-related change in which skeletal muscle mass decreases, skeletal muscle strength decreases, and physical function decreases with age (172). The results of a meta-analysis indicate that sarcopenia is highly prevalent in individuals with respiratory disease (173). The onset of sarcopenia is closely linked to inflammation and immune senescence and eventually leads to frailty (Figure 5).
Figure 5. Mechanistic models of sarcopenia and frailty induced by inflammation and immunosenescence. Inflammation induces the production of proinflammatory cytokines and activates scalpains and caspase-3 to cleave myofibrillar proteins. The ubiquitin–proteasome system degrades cleaved proteins. Autophagy is another pathway of muscle atrophy caused by inflammation. Chronic low-grade inflammation causes immunosenescence, leading to aberrantly migrating neutrophils that cause secondary damage to healthy muscle. Sarcopenia can develop further into frailty if it is not promptly corrected.
Inflammation leads to muscle proteolysis by affecting elevated levels of TNF-α, IL-6, IL-1, and CRP and inhibits protein synthesis through activation of the NF-κB and ubiquitin–proteasome pathways, which affects the balance between anabolic and catabolic processes in muscle, resulting in sarcopenia (174). A study has postulated that muscle injury in an older person combined with immune aging would cause secondary damage to healthy muscle from aberrantly migrating neutrophils, resulting in myocyte damage and apoptosis and loss of muscle fibers, which could result in functional loss and physical weakness associated with frailty (175). Frailty is an extremely relevant geriatric condition in an aging society, and its occurrence increases with age (176). It can be defined simply as the clinical state in which an individual increases vulnerability to the development of negative health-related events when exposed to endogenous or exogenous stressors (177). It can be viewed as a progressive age-related decline in physiological systems, leading to a reduction in intrinsic capacity reserves, making people highly vulnerable to stress and increasing the risk of a range of adverse health outcomes (178). Sarcopenia is a high risk factor for frailty, leading to increased mortality (172). Frailty syndromes might result in the following: poorer response to somatic and/or psychiatric stressors, increased risk of hospitalization, adverse disease outcomes, hospitalization, and premature death (179). Hence, both sarcopenia and frailty are extremely challenging for older adults with CAP. Previous studies have revealed relationships among oxidative stress, sarcopenia and frailty (52, 180). In addition to the previously mentioned immune senescence- and inflammation-related mechanisms, sarcopenia is also associated with oxidative stress in skeletal muscle. An animal study found that mice deficient in Cu/ZnSOD exhibited sarcopenia (181). Oxidative stress promotes muscle atrophy, so skeletal muscle atrophy can be treated by targeting antioxidative stress (182). For example, an early animal study noted that VitE and C supplementation reduced oxidative stress and improved antioxidant enzymes and positive muscle work in chronically loaded muscles of aged rats (105). There are also studies summarizing the preventive and therapeutic potential of a particular type of VitE, tocotrienol, whose superior antioxidant capacity improves antioxidant defense mechanisms in muscle-forming cells, enhances musculoskeletal health and addresses age-related musculoskeletal problems (102, 183). According to a recent study, melatonin targets mitochondria, scavenges free radicals and reduces oxidative damage, with a potential and promising therapeutic role in limiting muscle degeneration (mainly mitochondrial function) and sarcopenia (184). This conclusion has also been confirmed in a recent animal study. Following melatonin treatment, histological damage was alleviated, and the cross-sectional area of muscle fibers in the gastrocnemius tissues of middle-aged mice increased. Furthermore, the percentage and size of normal mitochondria increased, while the levels of MDA and ROS decreased in these tissues (119).
Previous studies have suggested that oxidative stress may be associated with frailty by affecting RNA oxidation, absolute telomere length shortening, increased markers of oxidative stress, and mitochondrial dysfunction and it was also found that the deleterious effects of both oxidative stress and frailty increase linearly with aging (185, 186). Therefore, it may be feasible to intervene in frailty by modulating oxidative stress. An early clinical trial involving 827 older adults revealed an association between low circulating levels of VitE, one of the most important components of the human antioxidant system, and the presence of frailty. Participants with the highest VitE levels were less likely to be frail than those with the lowest VitE levels were (103). The results from a 12-year Framingham Heart Follow-Up Study suggest that higher dietary quercetin intake is particularly strongly associated with lower odds of frailty onset in adults (187).
Surely, the onset and development of sarcopenia and frailty are not determined by only oxidative stress, and oxidative stress is only one of the important mechanisms. However, relevant studies have been able to affirm, to some extent, the positive significance of modulating oxidative stress in sarcopenia and frailty, which could be instructive for the future of older CAP patients with related diseases.
Aging is characterized by a progressive loss of tissue and organ function caused by genetic and environmental factors, nutrition and lifestyle. Oxidative stress is one of the most important mechanisms of increased cellular senescence and frailty, leading to a wide range of diseases that are common in the older population, including chronic lung disease, cardiovascular disease, chronic kidney disease, skeletal muscle dysfunction and cancer (188, 189). In such cases, a public health problem arises—multimorbidity—in which individuals develop two or more chronic pathologies. It is estimated that more than half of the population over the age of 60 is affected (190). The requirement of medications as first-line treatment for these chronic conditions increases the potential cost of treatment, leaving older adults in a polypharmacy dilemma and causing many negative effects. It is also directly correlated with the development of frailty, which may further compromise the antioxidant defense system (191). Risk factors such as malnutrition, multimorbidity and polypharmacy also increase the risk of severe illness and death in older CAP patients (192). In cases where oxidative stress is the pathophysiological basis, some natural antioxidant products work through different mechanisms than conventional drugs do to enhance therapeutic efficacy, avoiding the need to add new drugs to basal therapies, thus achieving therapeutic goals at lower doses of standard therapies (191). One study revealed that the use of vitamins C and/or E in men taking daily metformin improved insulin resistance and fasting blood glucose levels, perhaps without the need to add another hypoglycemic agent (106). A meta-analysis revealed that VitC supplementation in patients with chronic obstructive pulmonary disease (COPD) increased serum antioxidant levels (VitC and GSH) and improved lung function [FEV1% and FEV1/FVC; (193)]. Another review discussed the beneficial health effects of VitE in the prevention or treatment of hyperlipidemia, diabetes, osteoporosis, and cancer (194). Quercetin can be a potential treatment agent for a variety of inflammatory conditions, and it can also lower blood pressure, lower cholesterol levels, improve endothelial function, and even exhibit anticancer effects by inhibiting cancer cell proliferation and inducing apoptosis (195). Some reviews affirmed the importance of the antioxidant effects of melatonin in ameliorating chronic kidney disease and diabetic chronic kidney disease and proposed that endogenous antioxidants have fewer side effects than do exogenous antioxidants (196, 197). CoQ10 supplementation has a positive effect on mitochondrial deficiency syndrome and some of the symptoms of aging, whereas cardiovascular disease and inflammation are mitigated by the antioxidant effects of CoQ10 (198).
Additionally, some studies have indicated that certain medications for underlying conditions, commonly used in older adults, can lead to nutrient deficiencies. For example, individuals with hyperlipidemia treated with statins exhibit significant reductions in CoQ10, α-tocopherol, and other important antioxidants. These reductions are thought to drive statin-induced myopathy (199). Coadministration of CoQ10 may ameliorate these effects, but additional studies are needed to confirm this, particularly in older adults experiencing or at risk of polypharmacy (200). Long-term, high-dose aspirin use has been associated with decreased VitC levels, which can lead to gastric mucosal thinning, gastritis, peptic ulcer disease, nausea, anorexia, and malnutrition (199, 201). However, the doses used for primary and secondary cardiovascular disease prophylaxis are much lower. Moreover, there is currently no evidence suggesting a similar reduction in VitC levels or a need for VitC supplementation in chronic low-dose aspirin users (200).
In fact, patients with CAP cannot avoid the risk of multimorbidity and polypharmacy, and some chronic diseases are even detected during the treatment of CAP. Owing to various changes in the modern environment, diet and life structure, this situation can hardly be solved at the source and plagues the treatment of many diseases, not only CAP. Multiple medications may interact with each other and, to some extent, may be detrimental to the treatment of CAP or even affect the treatment plan for CAP. However, oxidative stress has been linked to many diseases in older individuals, and it might be a good attempt to intervene in chronic diseases from the perspective of modulating oxidative stress. In particular, many natural antioxidant compounds have relatively safe compositions and a wide therapeutic range, and we are confident in their further development and use in the future.
Since the worldwide outbreak of SARS-CoV-2, oxidative stress has attracted a great deal of attention, and there has been a proliferation of scientific studies on the theory and practice of its relationship with pneumonia (202, 203). As more is known about the disease and a vaccine has been developed, the threat of COVID-19 is diminishing, but some patients experience persistent symptoms or sequelae even after recovering from COVID-19 (204, 205). The estimated overall global prevalence of COVID-19 sequelae is approximately 43%, with major clinical manifestations, including pulmonary, cardiovascular, renal, neuropsychiatric, and other related symptoms (206). Moreover, COVID-19 appears to have long-term health effects, which may put strain on the health care system. This long-term COVID-19 affects a large proportion of people in all age groups, and older adults are characterized by age-specific manifestations and are more likely to have persistent symptoms or sequelae, increasing susceptibility to the disease and affecting quality of life (207). A Korean study revealed that COVID-19 negatively affects physical activity, quality of life, and memory in older adults (> 55 years old), leading to mental diseases such as insomnia and depression (208). Therefore, after the onset of COVID-19, older CAP patients deserve more attention, and providing more comprehensive therapeutic measures and modulating the oxidative stress state may be a good choice.
For example, melatonin reduces the long-term inflammatory and oxidative effects of viruses, stimulates the suppressed immune system, and has the potential to ameliorate inflammation and inhibit the cytokine storm induced by SARS-CoV-2 (209). Treatment with antioxidant supplements such as NAC, VitC, VitE, and melatonin in COVID-19 patients reduces biomarkers of oxidative stress and inflammation, such as total systemic antioxidant capacity, CRP, IL-6, and lipid peroxidation, and improves the survival score (210). However, a recent study of markers of oxidative stress in older adults with SARS-CoV-2 infection revealed that oxidative stress was associated with fatal events as well as near-term prognosis in older adults with SARS-CoV-2 infection but was less important than other predictors (e.g., cardiovascular factors) of long-term prognosis at 2 years (211). Notably, the unique active ingredients in various natural traditional Chinese medicine (TCM) herbs also play important roles in the prevention and treatment of COVID-19 through their anti-inflammatory, antioxidant and antiapoptotic effects (212). In fact, the respiratory tract and lungs bear the brunt of this worldwide epidemic. Even if most older adults are clinically cured, the respiratory damage caused by COVID-19 should not be ignored. In conjunction with existing research, modulating oxidative stress homeostasis can indeed benefit older adults to some extent and increase their resistance to future threats of challenges such as COVID-19.
Oxidative stress has been demonstrated to be associated with CAP in older individuals through various theories and practices. Free radicals act as physiological signaling messengers to oxidatively modify biologically essential molecules to maintain normal cellular physiology and pathology. The current concern is that excessive or inappropriate antioxidant interventions in aging CAP instead disrupt normal cell signaling pathways and inhibit the beneficial effects of ROS and lipid peroxidation products on disease, such as by mediating the physiological apoptosis of immune cells (213). This, in turn, deviates from the original intent of antioxidant therapy and negatively affects inflammation, the immune response, etc., driving the progression of CAP and increasing the risk of CAP. In particular, older people also have chronic low-grade inflammation, so antioxidants should be carefully applied. Moreover, not all conditions are suitable for antioxidative stress treatment. Researches have indicated that some pathogens respond to host oxidative stress by producing GSH to survive in the environment and the host. This mechanism involves most gram-negative bacteria and a few gram-positive bacteria and is more prevalent and essential in eukaryotic cells (214). Experiments have also demonstrated that GSH biosynthesis-deficient Pseudomonas aeruginosa mutants are less virulent in an acute pneumonia infection model (215). Therefore, analyzing from the other side, increasing the oxidative stress response of the cells can serve to deplete part of the GSH defense produced by the pathogen, thus reducing its virulence. It has also been suggested that the development of chronic bacterial infections and antibiotic resistance is associated with altered adaptation of pathogens to oxidative stress, suggesting that chronic infections and antibiotic resistance can be counteracted by increasing oxidative stress in cells (67). However, controlling the degree of enhancement to inhibit the pathogen while avoiding excessive oxidative stress in the organism is still a current challenge.
Studies show that antioxidants can have dichotomous roles in ROS production. They are easily oxidized and can act as oxidants to induce damage at high concentrations (100). An early animal study demonstrated that VitC primarily acts as an antioxidant at low doses, whereas pro-oxidant effects predominate at high doses. Thus, its use at pharmacological doses should be approached with caution (95). Such differences are also observed in clinical trials. For example, the effects of different high doses of VitC are not uniform in patients with severe COVID-19 (96). Administering 3 g/day of VitC to moderate and severe COVID-19 ICU patients reduced inflammation and mortality without adverse effects. However, a dose of 6 g/day has no significant impact on ICU length of stay or mortality (97). Earlier studies have shown that in healthy volunteers, daily doses of VitC exceeding 500 mg tend to decrease its bioavailability. Safe daily doses are generally less than 1000 mg, and doses above 400 mg daily offer no additional benefit (216). However, the optimal dose of VitC that is both safe and effective for antioxidant activity in disease states remains unclear. In addition, studies have shown that NAC can protect melanocytes from oxidative stress and damage and delay the onset of ultraviolet-induced melanoma in mice. However, high doses may increase Nrf2 nuclear translocation, potentially promoting metastasis (217, 218).
In summary, we advocate the healthy modulation of oxidative stress in older CAP patients to achieve the desired therapeutic assistance rather than simply inhibiting and scavenging free radicals. However, although common antioxidants such as vitamins, NAC, and Co Q10 are commonly used as drugs in the clinic, the emphasis is still on their primary pharmacological effects, and their antioxidant effects remain at the research stage. The wide variety of antioxidants that have been identified are constantly being depleted, as they work with free radicals, and different antioxidants are often accompanied by other biological effects. Therefore, the choice of antioxidants to be used to treat older adults with CAP, the dosage and timing of their use, and the optimal mode of administration are still not very clear, and more targeted studies are needed.
Given existing studies, we propose focusing research on adjunctive treatments for CAP with combined antibiotics to alleviate clinical symptoms while considering the unique characteristics of older adult patients. Most studies have focused on COVID-19 patients, with fewer addressing CAP caused by other pathogens, limiting generalizability. To avoid drug interactions, the study population should initially consist of older CAP patients without comorbidities, then expand to include those with one or more comorbidities, malnutrition, or immunodeficiencies. Drug selection should prioritize single-agent therapies with high safety profiles, clear mechanisms of action, high bioavailability, and suitability for CAP treatment, such as NAC. Determining drug dosage, timing, and mode of administration should begin with in vitro experiments, reference clinically established safe dosages, and integrate drug toxicology. Oxidative stress involves multiple signaling molecules and reactive enzymes; thus, detection indices should be both experimentally valuable and universally applicable. Incorporating the effects of pathogenic microorganisms, inflammation, and immune responses into the study design would further enrich the experimental content.
Global aging has resulted in an enormous increase in the population of older people and an enormous challenge to global public health. The impact of CAP, one of the most common respiratory system diseases, on the older population is obvious. The onset and development of CAP are closely related to several pathophysiological aspects, including pathogenic microorganisms, inflammation, immunity, and oxidative stress. In older CAP patients, there are also multiple age-related risk factors, such as immune senescence, sarcopenia, and polypharmacy, that influence the clinical presentation, development, and prognosis. Anti-infective therapy is still the primary treatment for CAP, but inappropriate use of antibiotics is common in older individuals, and older individuals are particularly susceptible to antibiotic-associated adverse effects. Modulating the signaling pathways that cause the inflammatory response and improve the immune function of patients has become the focus of reducing inflammatory damage in the lungs, especially in older adults with CAP. Oxidative stress, as an important mechanism of CAP, has been widely demonstrated to be related to inflammation and immunity. Especially after the occurrence of COVID-19, a number of studies related to oxidative stress have been conducted, but they tend to be limited to inflammation and immunity only, ignoring the possible additional links with the older population, who are the most infected with CAP. Therefore, we attempted to further clarify the link between oxidative stress and age-related risk factors such as immune senescence, sarcopenia, frailty, aging, and multimorbidity in the context of older adults CAP patients, as well as the greater potential value and prospects of modulating oxidative stress in treating older adults with CAP.
Clearly, there is no doubt that targeted modulation of oxidative stress benefits older adults CAP patients. However, many challenges and unknowns concerning how to modulate oxidative stress for further practical clinical applications exist, and more targeted research is needed. This review is limited in length, and we mainly discuss toward the characteristics of older adults CAP, emphasizing the value of modulating oxidative stress; thus, we only briefly describe the various immune, inflammatory, and aging mechanisms and only cover the study of common antioxidants, such as GSH, NAC, vitamins, CoQ10, and melatonin. If readers need further information, please refer to the outstanding articles cited in the text for relevant content.
WM: Conceptualization, Data curation, Formal analysis, Investigation, Methodology, Resources, Software, Writing – original draft, Writing – review & editing. XL: Conceptualization, Data curation, Resources, Software, Writing – original draft, Writing – review & editing. SF: Conceptualization, Formal analysis, Investigation, Resources, Supervision, Visualization, Writing – review & editing. RZ: Data curation, Investigation, Methodology, Supervision, Validation, Writing – review & editing. ML: Conceptualization, Data curation, Investigation, Writing – review & editing. SX: Conceptualization, Data curation, Formal analysis, Funding acquisition, Methodology, Project administration, Resources, Supervision, Validation, Visualization, Writing – review & editing.
The author(s) declare that financial support was received for the research and/or publication of this article. Receipt of the following financial support for the research and/or publication of this article: the Natural Science Foundation of Chongqing Yongchuan (2022YC-JCKX20045).
The authors declare that the research was conducted in the absence of any commercial or financial relationships that could be construed as a potential conflict of interest.
The author(s) declare that no Gen AI was used in the creation of this manuscript.
All claims expressed in this article are solely those of the authors and do not necessarily represent those of their affiliated organizations, or those of the publisher, the editors and the reviewers. Any product that may be evaluated in this article, or claim that may be made by its manufacturer, is not guaranteed or endorsed by the publisher.
CAP, community-acquired pneumonia; COVID-19, coronavirus disease 2019; ROS, reactive oxygen species; RNS, reactive nitrogen species; GSH, glutathione; CoQ10, coenzyme Q10; VitC, vitamin C; VitE, vitamin E; GPX, glutathione peroxidase; CAT, catalase; SOD, superoxide dismutase; Nrf2, NF-E2-related factor 2; Keap1, kelch-like ECH-associated protein 1; AREs, antioxidant response elements; AMPK, adenosine 5'-monophosphate-activated protein kinase; ATP, adenosine triphosphate; FoxO3a, Forkhead box O3a; HIF-1α, hypoxia-inducible factor 1α; PGC-1α, peroxisome proliferator-activated receptor γ coactivator-1α; ER, endoplasmic reticulum; STING, stimulator of interferon genes; TBK1, TANK-binding kinase 1; NOX2, NADPH oxidase; NF-κB, nuclear factor kappa-light-chain-enhancer of activated B cells; AP-1, activating protein 1; TNF-α, tumor necrosis factor-α; NG, non-growing; S. aureus, Staphylococcus aureus; FQ, fluoroquinolones; ARDS, acute respiratory distress syndrome; NAC, N-Acetyl-L-cysteine; SARS-CoV-2, severe acute respiratory syndrome coronavirus 2; Th1, T helper 1.
1. Cao B, Huang Y, She DY, Cheng QJ, Fan H, Tian XL, et al. Diagnosis and treatment of community-acquired pneumonia in adults: 2016 clinical practice guidelines by the Chinese thoracic society, Chinese medical association. Clin Respir J. (2018) 12:1320–60. doi: 10.1111/crj.12674
2. Torres A, Cilloniz C, Niederman MS, Menéndez R, Chalmers JD, Wunderink RG, et al. Pneumonia. Nat Rev Dis Primers. (2021) 7:25. doi: 10.1038/s41572-021-00259-0
3. Sun Y, Li H, Pei Z, Wang S, Feng J, Xu L, et al. Incidence of community-acquired pneumonia in urban China: a national population-based study. Vaccine. (2020) 38:8362–70. doi: 10.1016/j.vaccine.2020.11.004
4. Vig SL, Goyal P, Saini S, Singh M, Prasad J, Parashar L. Economic hardships in managing COVID-19 patients in the intensive care unit – a retrospective observational study at a tertiary care hospital in North India. Cureus. (2024) 16:e54588. doi: 10.7759/cureus.54588
5. Castillo RL, Carrasco RA, Alvarez PI, Ruiz M, Luchsinger V, Zunino E, et al. Relationship between severity of adult community-acquired pneumonia and impairment of the antioxidant defense system. Biol Res. (2013) 46:207–13. doi: 10.4067/S0716-97602013000200013
6. Muravlyova L, Molotov-Luchankiy V, Bakirova R, Klyuyev D, Demidchik L, Lee V. Characteristic of the oxidative stress in blood of patients in dependence of community-acquired pneumonia severity. Open Access Maced J Med Sci. (2016) 4:122–7. doi: 10.3889/oamjms.2016.040
7. Noonong K, Chatatikun M, Surinkaew S, Kotepui M, Hossain R, Bunluepuech K, et al. Mitochondrial oxidative stress, mitochondrial ROS storms in long COVID pathogenesis. Front Immunol. (2023) 14:1275001. doi: 10.3389/fimmu.2023.1275001
8. Grousd JA, Rich HE, Alcorn JF. Host-pathogen interactions in gram-positive bacterial pneumonia. Clin Microbiol Rev. (2019) 32:e00107–18. doi: 10.1128/CMR.00107-18
9. Siegel SJ, Weiser JN. Mechanisms of bacterial colonization of the respiratory tract. Annu Rev Microbiol. (2015) 69:425–44. doi: 10.1146/annurev-micro-091014-104209
10. Craig A, Mai J, Cai S, Jeyaseelan S. Neutrophil recruitment to the lungs during bacterial pneumonia. Infect Immun. (2009) 77:568–75. doi: 10.1128/IAI.00832-08
11. Primorac D, Vrdoljak K, Brlek P, Pavelić E, Molnar V, Matišić V, et al. Adaptive immune responses and immunity to SARS-CoV-2. Front Immunol. (2022) 13:848582. doi: 10.3389/fimmu.2022.848582
12. Clementi N, Ghosh S, De Santis M, Castelli M, Criscuolo E, Zanoni I, et al. Viral respiratory pathogens and lung injury. Clin Microbiol Rev. (2021) 34:e00103–20. doi: 10.1128/CMR.00103-20
13. Gusev E, Sarapultsev A, Solomatina L, Chereshnev V. SARS-CoV-2-specific immune response and the pathogenesis of COVID-19. Int J Mol Sci. (2022) 23:1716. doi: 10.3390/ijms23031716
14. Heung LJ, Wiesner DL, Wang K, Rivera A, Hohl TM. Immunity to fungi in the lung. Semin Immunol. (2023) 66:101728. doi: 10.1016/j.smim.2023.101728
15. Ji Y, Karbaschi M, Cooke MS. Mycoplasma infection of cultured cells induces oxidative stress and attenuates cellular base excision repair activity. Mutat Res Genet Toxicol Environ Mutagen. (2019) 845:403054. doi: 10.1016/j.mrgentox.2019.05.010
16. Wang M, Ren R, Xu Y, Wang T, Liang X, Li S. Oxidative stress in the alveolar lavage fluid of children with Mycoplasma pneumoniae pneumonia. Pediatr Pulmonol. (2024) 59:2772–82. doi: 10.1002/ppul.27120
17. Di Pietro M, Filardo S, De Santis F, Mastromarino P, Sessa R. Chlamydia pneumoniae and oxidative stress in cardiovascular disease: state of the art and prevention strategies. Int J Mol Sci. (2014) 16:724–35. doi: 10.3390/ijms16010724
18. Mifsud EJ, Kuba M, Barr IG. Innate immune responses to influenza virus infections in the upper respiratory tract. Viruses. (2021) 13:2090. doi: 10.3390/v13102090
19. Mattoo SU, Kim SJ, Ahn DG, Myoung J. Escape and over-activation of innate immune responses by SARS-CoV-2: two faces of a coin. Viruses. (2022) 14:530. doi: 10.3390/v14030530
20. Chan L, Pinedo K, Stabile MA, Hamlin RE, Pienkos SM, Ratnasiri K, et al. Prior vaccination prevents overactivation of innate immune responses during COVID-19 breakthrough infection. Sci Transl Med. (2025) 17:eadq1086. doi: 10.1126/scitranslmed.adq1086
21. Siljan WW, Holter JC, Nymo SH, Husebye E, Ueland T, Aukrust P, et al. Cytokine responses, microbial aetiology and short-term outcome in community-acquired pneumonia. Eur J Clin Invest. (2018) 48:e12865. doi: 10.1111/eci.12865
22. Jones B, Waterer G. Advances in community-acquired pneumonia. Ther Adv Infect Dis. (2020) 7:2049936120969607. doi: 10.1177/2049936120969607
23. Dela Cruz CS, Wunderink RG, Christiani DC, Cormier SA, Crothers K, Doerschuk CM, et al. Future research directions in pneumonia. NHLBI working group report. Am J Respir Crit Care Med. (2018) 198:256–63. doi: 10.1164/rccm.201801-0139WS
24. Thibeault C, Suttorp N, Opitz B. The microbiota in pneumonia: from protection to predisposition. Sci Transl Med. (2021) 13:eaba0501. doi: 10.1126/scitranslmed.aba0501
25. Hammami S, Ghzaiel I, Hammouda S, Sakly N, Hammami M, Zarrouk A. Evaluation of pro-inflammatory cytokines in frail Tunisian older adults. PLoS ONE. (2020) 15:e0242152. doi: 10.1371/journal.pone.0242152
26. Bian AL, Hu HY, Rong YD, Wang J, Wang JX, Zhou XZ, et al. A study on relationship between elderly sarcopenia and inflammatory factors IL-6 and TNF-α. Eur J Med Res. (2017) 22:25. doi: 10.1186/s40001-017-0266-9
27. Ferrucci L, Fabbri E. Inflammageing: chronic inflammation in ageing, cardiovascular disease, and frailty. Nat Rev Cardiol. (2018) 15:505–22. doi: 10.1038/s41569-018-0064-2
28. Singh T, Newman AB. Inflammatory markers in population studies of aging. Ageing Res Rev. (2011) 10:319–29. doi: 10.1016/j.arr.2010.11.002
29. Soysal P, Isik AT, Carvalho AF, Fernandes BS, Solmi M, Schofield P, et al. Oxidative stress and frailty: a systematic review and synthesis of the best evidence. Maturitas. (2017) 99:66–72. doi: 10.1016/j.maturitas.2017.01.006
30. Chandrasekaran A, Idelchik M, Melendez JA. Redox control of senescence and age-related disease. Redox Biol. (2017) 11:91–102. doi: 10.1016/j.redox.2016.11.005
31. Shaito A, Aramouni K, Assaf R, Parenti A, Orekhov A, Yazbi AE, et al. Oxidative stress-induced endothelial dysfunction in cardiovascular diseases. Front Biosci. (2022) 27:105. doi: 10.31083/j.fbl2703105
32. Xu W, Zhao T, Xiao H. The implication of oxidative stress and AMPK-Nrf2 antioxidative signaling in pneumonia pathogenesis. Front Endocrinol. (2020) 11:400. doi: 10.3389/fendo.2020.00400
33. Kurutas EB. The importance of antioxidants which play the role in cellular response against oxidative/nitrosative stress: current state. Nutr J. (2016) 15:71. doi: 10.1186/s12937-016-0186-5
34. Mirończuk-Chodakowska I, Witkowska AM, Zujko ME. Endogenous non-enzymatic antioxidants in the human body. Adv Med Sci. (2018) 63:68–78. doi: 10.1016/j.advms.2017.05.005
35. Averill-Bates DA. The antioxidant glutathione. Vitam Horm. (2023) 121:109–41. doi: 10.1016/bs.vh.2022.09.002
36. Liguori I, Russo G, Curcio F, Bulli G, Aran L, Della-Morte D, et al. Oxidative stress, aging, and diseases. Clin Interv Aging. (2018) 13:757–72. doi: 10.2147/CIA.S158513
37. Birben E, Sahiner UM, Sackesen C, Erzurum S, Kalayci O. Oxidative stress and antioxidant defense. World Allergy Organ J. (2012) 5:9–19. doi: 10.1097/WOX.0b013e3182439613
38. Baird L, Yamamoto M. The molecular mechanisms regulating the KEAP1-NRF2 pathway. Mol Cell Biol. (2020) 40:e00099–20. doi: 10.1128/MCB.00099-20
39. Suzuki T, Yamamoto M. Stress-sensing mechanisms and the physiological roles of the Keap1-Nrf2 system during cellular stress. J Biol Chem. (2017) 292:16817–24. doi: 10.1074/jbc.R117.800169
40. Gowans GJ, Hawley SA, Ross FA, Hardie DG. AMP is a true physiological regulator of AMP-activated protein kinase by both allosteric activation and enhancing net phosphorylation. Cell Metab. (2013) 18:556–66. doi: 10.1016/j.cmet.2013.08.019
41. Tanaka M, Inoue H, Takahashi N, Uehara M. AMPK negatively regulates RANKL-induced osteoclast differentiation by controlling oxidative stress. Free Radic Biol Med. (2023) 205:107–15. doi: 10.1016/j.freeradbiomed.2023.05.033
42. Oakhill JS, Steel R, Chen ZP, Scott JW, Ling N, Tam S, et al. AMPK is a direct adenylate charge-regulated protein kinase. Science. (2011) 332:1433–5. doi: 10.1126/science.1200094
43. Fan C, Feng J, Tang C, Zhang Z, Feng Y, Duan W, et al. Melatonin suppresses ER stress-dependent proapoptotic effects via AMPK in bone mesenchymal stem cells during mitochondrial oxidative damage. Stem Cell Res Ther. (2020) 11:442. doi: 10.1186/s13287-020-01948-5
44. Salminen A, Hyttinen JM, Kaarniranta K. AMP-activated protein kinase inhibits NF-κB signaling and inflammation: impact on healthspan and lifespan. J Mol Med. (2011) 89:667–76. doi: 10.1007/s00109-011-0748-0
45. Matzinger M, Fischhuber K, Pölöske D, Mechtler K, Heiss EH. AMPK leads to phosphorylation of the transcription factor Nrf2, tuning transactivation of selected target genes. Redox Biol. (2020) 29:101393. doi: 10.1016/j.redox.2019.101393
46. Lin CH, Cheng YC, Nicol CJ, Lin KH, Yen CH, Chiang MC. Activation of AMPK is neuroprotective in the oxidative stress by advanced glycosylation end products in human neural stem cells. Exp Cell Res. (2017) 359:367–73. doi: 10.1016/j.yexcr.2017.08.019
47. Lee HJ, Jung YH, Choi GE, Ko SH, Lee SJ, Lee SH, et al. BNIP3 induction by hypoxia stimulates FASN-dependent free fatty acid production enhancing therapeutic potential of umbilical cord blood-derived human mesenchymal stem cells. Redox Biol. (2017) 13:426–43. doi: 10.1016/j.redox.2017.07.004
48. Herzig S, Shaw RJ. AMPK: guardian of metabolism and mitochondrial homeostasis. Nat Rev Mol Cell Biol. (2018) 19:121–35. doi: 10.1038/nrm.2017.95
49. Zhong S, Chen W, Wang B, Gao C, Liu X, Song Y, et al. Energy stress modulation of AMPK/FoxO3 signaling inhibits mitochondria-associated ferroptosis. Redox Biol. (2023) 63:102760. doi: 10.1016/j.redox.2023.102760
50. Han X, Tai H, Wang X, Wang Z, Zhou J, Wei X, et al. AMPK activation protects cells from oxidative stress-induced senescence via autophagic flux restoration and intracellular NAD(+) elevation. Aging Cell. (2016) 15:416–27. doi: 10.1111/acel.12446
51. Zheng Z, Bian Y, Zhang Y, Ren G, Li G. Metformin activates AMPK/SIRT1/NF-κB pathway and induces mitochondrial dysfunction to drive caspase3/GSDME-mediated cancer cell pyroptosis. Cell Cycle. (2020) 19:1089–104. doi: 10.1080/15384101.2020.1743911
52. Anwar M, Pradhan R, Dey S, Kumar R. The role of sirtuins in sarcopenia and frailty. Aging Dis. (2023) 14:25–32. doi: 10.14336/AD.2022.0622
53. Nemoto S, Fergusson MM, Finkel T. SIRT1 functionally interacts with the metabolic regulator and transcriptional coactivator PGC-1α. J Biol Chem. (2005) 280:16456–60. doi: 10.1074/jbc.M501485200
54. Zarzuelo MJ, López-Sepúlveda R, Sánchez M, Romero M, Gómez-Guzmán M, Ungvary Z, et al. SIRT1 inhibits NADPH oxidase activation and protects endothelial function in the rat aorta: implications for vascular aging. Biochem Pharmacol. (2013) 85:1288–96. doi: 10.1016/j.bcp.2013.02.015
55. Muftuoglu M, Mori MP, de Souza-Pinto NC. Formation and repair of oxidative damage in the mitochondrial DNA. Mitochondrion. (2014) 17:164–81. doi: 10.1016/j.mito.2014.03.007
56. Valko M, Leibfritz D, Moncol J, Cronin MT, Mazur M, Telser J. Free radicals and antioxidants in normal physiological functions and human disease. Int J Biochem Cell Biol. (2007) 39:44–84. doi: 10.1016/j.biocel.2006.07.001
57. Zhang Q, Ju Y, Ma Y, Wang T. N-acetylcysteine improves oxidative stress and inflammatory response in patients with community acquired pneumonia: a randomized controlled trial. Medicine. (2018) 97:e13087. doi: 10.1097/MD.0000000000013087
58. Trefler S, Rodríguez A, Martín-Loeches I, Sanchez V, Marín J, Llauradó M, et al. Oxidative stress in immunocompetent patients with severe community-acquired pneumonia. A pilot study. Med Intensiva. (2014) 38:73–82. doi: 10.1016/j.medin.2013.01.004
59. Škesters A, Lece A, Kustovs D, Zolovs M. Selenium status and oxidative stress in SARS-CoV-2 patients. Medicina. (2023) 59:527. doi: 10.3390/medicina59030527
60. Zhang K, Kaufman RJ. From endoplasmic-reticulum stress to the inflammatory response. Nature. (2008) 454:455–62. doi: 10.1038/nature07203
61. Zhan Y, Xu D, Tian Y, Qu X, Sheng M, Lin Y, et al. Novel role of macrophage TXNIP-mediated CYLD-NRF2-OASL1 axis in stress-induced liver inflammation and cell death. JHEP Rep. (2022) 4:100532. doi: 10.1016/j.jhepr.2022.100532
62. Winterbourn CC, Kettle AJ, Hampton MB. Reactive oxygen species and neutrophil function. Annu Rev Biochem. (2016) 85:765–92. doi: 10.1146/annurev-biochem-060815-014442
63. Cazzola M, Page CP, Wedzicha JA, Celli BR, Anzueto A, Matera MG. Use of thiols and implications for the use of inhaled corticosteroids in the presence of oxidative stress in COPD. Respir Res. (2023) 24:194. doi: 10.1186/s12931-023-02500-8
64. Nauseef WM, Borregaard N. Neutrophils at work. Nat Immunol. (2014) 15:602–11. doi: 10.1038/ni.2921
65. Iddir M, Brito A, Dingeo G, Fernandez Del Campo SS, Samouda H, La Frano MR, et al. Strengthening the immune system and reducing inflammation and oxidative stress through diet and nutrition: considerations during the COVID-19 crisis. Nutrients. (2020) 12:1562. doi: 10.3390/nu12061562
66. Salazar F, Bignell E, Brown GD, Cook PC, Warris A. Pathogenesis of respiratory viral and fungal coinfections. Clin Microbiol Rev. (2022) 35:e0009421. doi: 10.1128/CMR.00094-21
67. Grant SS, Hung DT. Persistent bacterial infections, antibiotic tolerance, and the oxidative stress response. Virulence. (2013) 4:273–83. doi: 10.4161/viru.23987
68. Balaban NQ, Helaine S, Lewis K, Ackermann M, Aldridge B, Andersson DI, et al. Definitions and guidelines for research on antibiotic persistence. Nat Rev Microbiol. (2019) 17:441–8. doi: 10.1038/s41579-019-0196-3
69. Michaux C, Ronneau S, Giorgio RT, Helaine S. Antibiotic tolerance and persistence have distinct fitness trade-offs. PLoS Pathog. (2022) 18:e1010963. doi: 10.1371/journal.ppat.1010963
70. Lewis K. Persister cells, dormancy and infectious disease. Nat Rev Microbiol. (2007) 5:48–56. doi: 10.1038/nrmicro1557
71. Fauvart M, De Groote VN, Michiels J. Role of persister cells in chronic infections: clinical relevance and perspectives on anti-persister therapies. J Med Microbiol. (2011) 60:699–709. doi: 10.1099/jmm.0.030932-0
72. Hernandez-Morfa M, Reinoso-Vizcaíno NM, Olivero NB, Zappia VE, Cortes PR, Jaime A, et al. Host cell oxidative stress promotes intracellular fluoroquinolone persisters of Streptococcus pneumoniae. Microbiol Spectr. (2022) 10:e0436422. doi: 10.1128/spectrum.04364-22
73. Ronneau S, Michaux C, Helaine S. Decline in nitrosative stress drives antibiotic persister regrowth during infection. Cell Host Microbe. (2023) 31:993–1006.e6. doi: 10.1016/j.chom.2023.05.002
74. Batchelder JI, Taylor AJ, Mok WWK. Metabolites augment oxidative stress to sensitize antibiotic-tolerant Staphylococcus aureus to fluoroquinolones. MBio. (2024) 15:e0271424. doi: 10.1128/mbio.02714-24
75. Ranganathan N, Johnson R, Edwards AM. The general stress response of Staphylococcus aureus promotes tolerance of antibiotics and survival in whole human blood. Microbiology. (2020) 166:1088–94. doi: 10.1099/mic.0.000983
76. Rowe SE, Wagner NJ, Li L, Beam JE, Wilkinson AD, Radlinski LC, et al. Reactive oxygen species induce antibiotic tolerance during systemic Staphylococcus aureus infection. Nat Microbiol. (2020) 5:282–90. doi: 10.1038/s41564-019-0627-y
77. Santus P, Danzo F, Zuffi A, Pini S, Saad M, Visconti A, et al. Oxidative stress and viral Infections: rationale, experiences, and perspectives on N-acetylcysteine. Eur Rev Med Pharmacol Sci. (2022) 26:8582–90. doi: 10.26355/eurrev_202211_30395
78. Warris A, Ballou ER. Oxidative responses and fungal infection biology. Semin Cell Dev Biol. (2019) 89:34–46. doi: 10.1016/j.semcdb.2018.03.004
79. Surme S, Balkan II, Bayramlar OF, Kara Ali R, Mete B, Tabak F, et al. Predictors of long-term outcomes in the older adults with community-acquired pneumonia. J Infect Dev Ctries. (2021) 15:1910–6. doi: 10.3855/jidc.14881
80. Arnold FW, Reyes Vega AM, Salunkhe V, Furmanek S, Furman C, Morton L, et al. Older adults hospitalized for pneumonia in the United States: incidence, epidemiology, and outcomes. J Am Geriatr Soc. (2020) 68:1007–14. doi: 10.1111/jgs.16327
81. Li ZJ, Zhang HY, Ren LL, Lu QB, Ren X, Zhang CH, et al. Etiological and epidemiological features of acute respiratory infections in China. Nat Commun. (2021) 12:5026. doi: 10.1038/s41467-021-25120-6
82. Eshwara VK, Mukhopadhyay C, Rello J. Community-acquired bacterial pneumonia in adults: an update. Indian J Med Res. (2020) 151:287–302. doi: 10.4103/ijmr.IJMR_1678_19
83. Furman CD, Leinenbach A, Usher R, Elikkottil J, Arnold FW. Pneumonia in older adults. Curr Opin Infect Dis. (2021) 34:135–41. doi: 10.1097/QCO.0000000000000718
84. Schöll N, Rohde GGU. [Community-acquired pneumonia in the elderly]. Pneumologie. (2019) 73:605–16. doi: 10.1055/a-0835-1943
85. Pulia MS, Keller SC, Crnich CJ, Jump RLP, Yoshikawa TT. Antibiotic stewardship for older adults in ambulatory care settings: addressing an unmet challenge. J Am Geriatr Soc. (2020) 68:244–9. doi: 10.1111/jgs.16256
86. Kalghatgi S, Spina CS, Costello JC, Liesa M, Morones-Ramirez JR, Slomovic S, et al. Bactericidal antibiotics induce mitochondrial dysfunction and oxidative damage in mammalian cells. Sci Transl Med. (2013) 5:192ra85. doi: 10.1126/scitranslmed.3006055
87. Teissier T, Boulanger E, Cox LS. Interconnections between inflammageing and immunosenescence during ageing. Cells. (2022) 11:359. doi: 10.3390/cells11030359
88. Prina E, Ceccato A, Torres A. New aspects in the management of pneumonia. Crit Care. (2016) 20:267. doi: 10.1186/s13054-016-1442-y
89. Zhu T, Xu A, Bai X, He Y, Zhang H. [Effect of convalescent plasma and immunoglobulin on patients with severe acute respiratory syndrome: a systematic review]. Zhonghua Wei Zhong Bing Ji Jiu Yi Xue. (2020) 32:435–8. doi: 10.3760/cma.j.cn121430-20200326-00240
90. Liu X, Cao W, Li T. High-Dose intravenous immunoglobulins in the treatment of severe acute viral pneumonia: the known mechanisms and clinical effects. Front Immunol. (2020) 11:1660. doi: 10.3389/fimmu.2020.01660
91. Pitt HA, Costrini AM. Vitamin C prophylaxis in marine recruits. JAMA. (1979) 241:908–11. doi: 10.1001/jama.1979.03290350028016
92. Hunt C, Chakravorty NK, Annan G, Habibzadeh N, Schorah CJ. The clinical effects of vitamin C supplementation in elderly hospitalised patients with acute respiratory infections. Int J Vitam Nutr Res. (1994) 64:212–9.
93. Chen Y, Luo G, Yuan J, Wang Y, Yang X, Wang X, et al. Vitamin C mitigates oxidative stress and tumor necrosis factor-alpha in severe community-acquired pneumonia and LPS-induced macrophages. Mediators Inflamm. (2014) 2014:426740. doi: 10.1155/2014/426740
94. Xing Y, Zhao B, Yin L, Guo M, Shi H, Zhu Z, et al. Vitamin C supplementation is necessary for patients with coronavirus disease: an ultra-high-performance liquid chromatography-tandem mass spectrometry finding. J Pharm Biomed Anal. (2021) 196:113927. doi: 10.1016/j.jpba.2021.113927
95. Seo MY, Lee SM. Protective effect of low dose of ascorbic acid on hepatobiliary function in hepatic ischemia/reperfusion in rats. J Hepatol. (2002) 36:72–7. doi: 10.1016/S0168-8278(01)00236-7
96. Kumar V, Bhushan D, Supriya S, Ganapule AA, Lohani P, Shyama, et al. Efficacy of intravenous vitamin C in management of moderate and severe COVID-19: A double blind randomized placebo controlled trial. J Family Med Prim Care. (2022) 11:4758–65. doi: 10.4103/jfmpc.jfmpc_2437_21
97. JamaliMoghadamSiahkali S, Zarezade B, Koolaji S, SeyedAlinaghi S, Zendehdel A, Tabarestani M, et al. Safety and effectiveness of high-dose vitamin C in patients with COVID-19: a randomized open-label clinical trial. Eur J Med Res. (2021) 26:20. doi: 10.1186/s40001-021-00490-1
98. Neupane B, Walter SD, Krueger P, Marrie T, Loeb M. Predictors of inhospital mortality and re-hospitalization in older adults with community-acquired pneumonia: a prospective cohort study. BMC Geriatr. (2010) 10:22. doi: 10.1186/1471-2318-10-22
99. Hemilä H. Vitamin E administration may decrease the incidence of pneumonia in elderly males. Clin Interv Aging. (2016) 11:1379–85. doi: 10.2147/CIA.S114515
100. Milisav I, Ribarič S, Poljsak B. Antioxidant vitamins and ageing. Subcell Biochem. (2018) 90:1–23. doi: 10.1007/978-981-13-2835-0_1
101. Lee JY, Paik IY, Kim JY. Voluntary exercise reverses immune aging induced by oxidative stress in aging mice. Exp Gerontol. (2019) 115:148–54. doi: 10.1016/j.exger.2018.08.009
102. Khor SC, Wan Ngah WZ, Mohd Yusof YA, Abdul Karim N, Makpol S. Tocotrienol-rich fraction ameliorates antioxidant defense mechanisms and improves replicative senescence-associated oxidative stress in human myoblasts. Oxid Med Cell Longev. (2017) 2017:3868305. doi: 10.1155/2017/3868305
103. Ble A, Cherubini A, Volpato S, Bartali B, Walston JD, Windham BG, et al. Lower plasma vitamin E levels are associated with the frailty syndrome: the InCHIANTI study. J Gerontol A Biol Sci Med Sci. (2006) 61:278–83. doi: 10.1093/gerona/61.3.278
104. Erol N, Saglam L, Saglam YS, Erol HS, Altun S, Aktas MS, et al. The protection potential of antioxidant vitamins against acute respiratory distress syndrome: a rat trial. Inflammation. (2019) 42:1585–94. doi: 10.1007/s10753-019-01020-2
105. Ryan MJ, Dudash HJ, Docherty M, Geronilla KB, Baker BA, Haff GG, et al. Vitamin E and C supplementation reduces oxidative stress, improves antioxidant enzymes and positive muscle work in chronically loaded muscles of aged rats. Exp Gerontol. (2010) 45:882–95. doi: 10.1016/j.exger.2010.08.002
106. El-Aal AA, El-Ghffar EAA, Ghali AA, Zughbur MR, Sirdah MM. The effect of vitamin C and/or E supplementations on type 2 diabetic adult males under metformin treatment: a single-blinded randomized controlled clinical trial. Diabetes Metab Syndr. (2018) 12:483–9. doi: 10.1016/j.dsx.2018.03.013
107. Bartolini D, Stabile AM, Bastianelli S, Giustarini D, Pierucci S, Busti C, et al. SARS-CoV2 infection impairs the metabolism and redox function of cellular glutathione. Redox Biol. (2021) 45:102041. doi: 10.1016/j.redox.2021.102041
108. Afaghi S, Moghimi N, Malekpour Alamdari N, Rahimi FS, Irilouzadian R, Esmaeili Tarki F, et al. N-acetylcysteine as adjuvant therapy for hospitalized COVID-19 patients: a single-center prospective cohort study. Casp J Intern Med. (2023) 14:543–52. doi: 10.22088/cjim.14.3.553
109. Chen H, Ma N, Song X, Wei G, Zhang H, Liu J, et al. Protective effects of N-acetylcysteine on lipopolysaccharide-induced respiratory inflammation and oxidative stress. Antioxidants. (2022) 11:879. doi: 10.3390/antiox11050879
110. Izquierdo JL, Soriano JB, González Y, Lumbreras S, Ancochea J, Echeverry C, et al. Use of N-acetylcysteine at high doses as an oral treatment for patients hospitalized with COVID-19. Sci Prog. (2022) 105:368504221074574. doi: 10.1177/00368504221074574
111. Gamarra-Morales Y, Herrera-Quintana L, Molina-López J, Vázquez-Lorente H, Machado-Casas JF, Castaño-Pérez J, et al. Response to intravenous N-acetylcysteine supplementation in critically ill patients with COVID-19. Nutrients. (2023) 15:2235. doi: 10.3390/nu15092235
112. Ousingsawat J, Schreiber R, Gulbins E, Kamler M, Kunzelmann K. P. aeruginosa induced lipid peroxidation causes ferroptotic cell death in airways. Cell Physiol Biochem. (2021) 55:590–604. doi: 10.33594/000000437
113. Farazi A, Sofian M, Jabbariasl M, Nayebzadeh B. Coenzyme q10 administration in community-acquired pneumonia in the elderly. Iran Red Crescent Med J. (2014) 16:e18852. doi: 10.5812/ircmj.18852
114. Jiang W, Liu J, Zhao X, Yang W. Melatonin-induced upregulation of telomerase activity interferes with macrophage mitochondrial metabolism and suppresses NLRP3 inflammasome activation in the treatment of Pneumonia. Heliyon. (2024) 10:e29681. doi: 10.1016/j.heliyon.2024.e29681
115. Farnoosh G, Akbariqomi M, Badri T, Bagheri M, Izadi M, Saeedi-Boroujeni A, et al. Efficacy of a low dose of melatonin as an adjunctive therapy in hospitalized patients with COVID-19: a randomized, double-blind clinical trial. Arch Med Res. (2022) 53:79–85. doi: 10.1016/j.arcmed.2021.06.006
116. Ameri A, Frouz Asadi M, Ziaei A, Vatankhah M, Safa O, Kamali M, et al. Efficacy and safety of oral melatonin in patients with severe COVID-19: a randomized controlled trial. Inflammopharmacology. (2023) 31:265–74. doi: 10.1007/s10787-022-01096-7
117. Hosseini A, Esmaeili Gouvarchin Ghaleh H, Aghamollaei H, Fasihi Ramandi M, Alishiri G, Shahriary A, et al. Evaluation of Th1 and Th2 mediated cellular and humoral immunity in patients with COVID-19 following the use of melatonin as an adjunctive treatment. Eur J Pharmacol. (2021) 904:174193. doi: 10.1016/j.ejphar.2021.174193
118. Soto ME, Pérez-Torres I, Manzano-Pech L, Palacios-Chavarría A, Valdez-Vázquez RR, Guarner-Lans V, et al. Redox homeostasis alteration is restored through melatonin treatment in COVID-19 patients: a preliminary study. Int J Mol Sci. (2024) 25:4543. doi: 10.3390/ijms25084543
119. Fang F, Yu P, Sun X, Shen Z, Zhang F, Sun J. Melatonin protects against sarcopenia in middle-aged mice. Histol Histopathol. (2024) 13:18814. doi: 10.14670/HH-18-814
120. van Werkhoven CH, Huijts SM. Vaccines to prevent pneumococcal community-acquired pneumonia. Clin Chest Med. (2018) 39:733–52. doi: 10.1016/j.ccm.2018.07.007
121. Kim S, Kim MJ, Myong JP, Lee YH, Kim BY, Hwang A, et al. Prior pneumococcal vaccination improves in-hospital mortality among elderly population hospitalized due to community-acquired pneumonia. BMC Pulm Med. (2024) 24:168. doi: 10.1186/s12890-024-02928-8
122. Yamana H, Ono S, Michihata N, Uemura K, Jo T, Yasunaga H. Effect of the 23-valent pneumococcal polysaccharide vaccine on the incidence of hospitalization with pneumonia in adults aged ≥65 years: retrospective cohort study using a population-based database in Japan. Clin Microbiol Infect. (2023) 29:904–10. doi: 10.1016/j.cmi.2023.04.006
123. Candemir I, Turk S, Ergun P, Kaymaz D. Influenza and pneumonia vaccination rates in patients hospitalized with acute respiratory failure. Hum Vaccin Immunother. (2019) 15:2606–11. doi: 10.1080/21645515.2019.1613128
124. Barbari A. COVID-19 vaccine concerns: fact or fiction? Exp Clin Transplant. (2021) 19:627–34. doi: 10.6002/ect.2021.0056
125. Goldberg EL, Shaw AC, Montgomery RR. How Inflammation Blunts Innate Immunity in aging. Interdiscip Top Gerontol Geriatr. (2020) 43:1–17. doi: 10.1159/000504480
126. Leyane TS, Jere SW, Houreld NN. Oxidative stress in ageing and chronic degenerative pathologies: molecular mechanisms involved in counteracting oxidative stress and chronic inflammation. Int J Mol Sci. (2022) 23:7273. doi: 10.3390/ijms23137273
127. Hemilä H. Vitamin E and the risk of pneumonia: using the I 2 statistic to quantify heterogeneity within a controlled trial. Br J Nutr. (2016) 116:1530–6. doi: 10.1017/S0007114516003408
128. Hemilä H, Virtamo J, Albanes D, Kaprio J. Vitamin E and beta-carotene supplementation and hospital-treated pneumonia incidence in male smokers. Chest. (2004) 125:557–65. doi: 10.1378/chest.125.2.557
129. Hemilä H, Kaprio J, Albanes D, Virtamo J. Physical activity and the risk of pneumonia in male smokers administered vitamin E and β-carotene. Int J Sports Med. (2006) 27:336–41. doi: 10.1055/s-2005-865670
130. Lugg ST, Scott A, Parekh D, Naidu B, Thickett DR. Cigarette smoke exposure and alveolar macrophages: mechanisms for lung disease. Thorax. (2022) 77:94–101. doi: 10.1136/thoraxjnl-2020-216296
131. Lee SI, Lim CM, Koh Y, Huh JW, Lee JS, Hong SB. The effectiveness of vitamin C for patients with severe viral pneumonia in respiratory failure. J Thorac Dis. (2021) 13:632–41. doi: 10.21037/jtd-20-1306
132. Padhani ZA, Moazzam Z, Ashraf A, Bilal H, Salam RA, Das JK, et al. Vitamin C supplementation for prevention and treatment of pneumonia. Cochrane Database Syst Rev. (2020) 4:Cd013134. doi: 10.1002/14651858.CD013134.pub2
133. Komal, Kumar J, Sen A. The role of vitamin C: from prevention of pneumonia to treatment of COVID-19. Mater Today Proc. (2022). doi: 10.1016/j.matpr.2022.11.213
134. Salo PM, Mendy A, Wilkerson J, Molsberry SA, Feinstein L, London SJ, et al. Serum antioxidant vitamins and respiratory morbidity and mortality: a pooled analysis. Respir Res. (2022) 23:150. doi: 10.1186/s12931-022-02059-w
135. Silvagno F, Vernone A, Pescarmona GP. The role of glutathione in protecting against the severe inflammatory response triggered by COVID-19. Antioxidants. (2020) 9:624. doi: 10.3390/antiox9070624
136. Ershad M, Naji A, Patel P, Vearrier D. N-Acetylcysteine. StatPearls. Treasure Island (FL) ineligible companies. StatPearls Publishing LLC (2024).
137. De Flora S, Balansky R, La Maestra S. Rationale for the use of N-acetylcysteine in both prevention and adjuvant therapy of COVID-19. FASEB J. (2020) 34:13185–93. doi: 10.1096/fj.202001807
138. Gutierrez-Mariscal FM, Arenas-de Larriva AP, Limia-Perez L, Romero-Cabrera JL, Yubero-Serrano EM, López-Miranda J. Coenzyme Q10 supplementation for the reduction of oxidative stress: clinical implications in the treatment of chronic diseases. Int J Mol Sci. (2020) 21:7870. doi: 10.3390/ijms21217870
139. Fakhrolmobasheri M, Hosseini MS, Shahrokh SG, Mohammadi Z, Kahlani MJ, Majidi SE, et al. Coenzyme Q10 and its therapeutic potencies against COVID-19 and other similar infections: a molecular review. Adv Pharm Bull. (2023) 13:233–43. doi: 10.34172/apb.2023.026
140. He F, Wu X, Zhang Q, Li Y, Ye Y, Li P, et al. Bacteriostatic potential of melatonin: therapeutic standing and mechanistic insights. Front Immunol. (2021) 12:683879. doi: 10.3389/fimmu.2021.683879
141. Azzeh FS, Kamfar WW, Ghaith MM, Alsafi RT, Shamlan G, Ghabashi MA, et al. Unlocking the health benefits of melatonin supplementation: a promising preventative and therapeutic strategy. Medicine. (2024) 103:e39657. doi: 10.1097/MD.0000000000039657
142. Corrao S, Mallaci Bocchio R, Lo Monaco M, Natoli G, Cavezzi A, Troiani E, et al. Does evidence exist to blunt inflammatory response by nutraceutical supplementation during COVID-19 pandemic? An overview of systematic reviews of vitamin D, vitamin C, melatonin, and zinc. Nutrients. (2021) 13:1261. doi: 10.3390/nu13041261
143. Valiensi SM, Folgueira A, Vera VA, González Cardozo A, Cardinali DP, Rugiero M. [Pre-pandemic melatonin treatment for sleep disorders and COVID-19 infection. A retrospective cross-sectional study]. Vertex. (2022) Xxxiii:13–24. doi: 10.53680/vertex.v33i155.132
144. Wakayama K, Shimamura M, Suzuki JI, Watanabe R, Koriyama H, Akazawa H, et al. Angiotensin II peptide vaccine protects ischemic brain through reducing oxidative stress. Stroke. (2017) 48:1362–8. doi: 10.1161/STROKEAHA.116.016269
146. Sousa LP, Pinho V, Teixeira MM. Harnessing inflammation resolving-based therapeutic agents to treat pulmonary viral infections: what can the future offer to COVID-19? Br J Pharmacol. (2020) 177:3898–904. doi: 10.1111/bph.15164
147. Yildiz H, Alp HH, Ekin S, Arisoy A, Gunbatar H, Asker S, et al. Analysis of endogenous oxidative damage markers and association with pulmonary involvement severity in patients with SARS-CoV-2 pneumonia. Infect Dis Now. (2021) 51:429–34. doi: 10.1016/j.idnow.2021.06.302
148. Michaud M, Balardy L, Moulis G, Gaudin C, Peyrot C, Vellas B, et al. Proinflammatory cytokines, aging, and age-related diseases. J Am Med Dir Assoc. (2013) 14:877–82. doi: 10.1016/j.jamda.2013.05.009
149. Espino J, Pariente JA, Rodríguez AB. Oxidative stress and immunosenescence: therapeutic effects of melatonin. Oxid Med Cell Longev. (2012) 2012:670294. doi: 10.1155/2012/670294
150. Lian J, Yue Y, Yu W, Zhang Y. Immunosenescence: a key player in cancer development. J Hematol Oncol. (2020) 13:151. doi: 10.1186/s13045-020-00986-z
151. Ray D, Yung R. Immune senescence, epigenetics and autoimmunity. Clin Immunol. (2018) 196:59–63. doi: 10.1016/j.clim.2018.04.002
152. Shive C, Pandiyan P. Inflammation, immune senescence, and dysregulated immune regulation in the elderly. Front Aging. (2022) 3:840827. doi: 10.3389/fragi.2022.840827
153. Liu Z, Liang Q, Ren Y, Guo C, Ge X, Wang L, et al. Immunosenescence: molecular mechanisms and diseases. Signal Transduct Target Ther. (2023) 8:200. doi: 10.1038/s41392-023-01451-2
154. Barbé-Tuana F, Funchal G, Schmitz CRR, Maurmann RM, Bauer ME. The interplay between immunosenescence and age-related diseases. Semin Immunopathol. (2020) 42:545–57. doi: 10.1007/s00281-020-00806-z
155. Guo J, Huang X, Dou L, Yan M, Shen T, Tang W, et al. Aging and aging-related diseases: from molecular mechanisms to interventions and treatments. Signal Transduct Target Ther. (2022) 7:391. doi: 10.1038/s41392-022-01251-0
156. Martínez de Toda I, Ceprián N, Díaz-Del Cerro E, De la Fuente M. The role of immune cells in oxi-inflamm-aging. Cells. (2021) 10:2974. doi: 10.3390/cells10112974
157. Virág L, Jaén RI, Regdon Z, Boscá L, Prieto P. Self-defense of macrophages against oxidative injury: fighting for their own survival. Redox Biol. (2019) 26:101261. doi: 10.1016/j.redox.2019.101261
158. Bauer ME, Fuente Mde L. The role of oxidative and inflammatory stress and persistent viral infections in immunosenescence. Mech Ageing Dev. (2016) 158:27–37. doi: 10.1016/j.mad.2016.01.001
159. Wessels I, Jansen J, Rink L, Uciechowski P. Immunosenescence of polymorphonuclear neutrophils. Sci World J. (2010) 10:145–60. doi: 10.1100/tsw.2010.14
160. Galli F, Marcantonini G, Giustarini D, Albertini MC, Migni A, Zatini L, et al. How aging and oxidative stress influence the cytopathic and inflammatory effects of SARS-CoV-2 infection: the role of cellular glutathione and cysteine metabolism. Antioxidants. (2022) 11:1366. doi: 10.3390/antiox11071366
161. Espino J, Bejarano I, Paredes SD, González D, Barriga C, Reiter RJ, et al. Melatonin counteracts alterations in oxidative metabolism and cell viability induced by intracellular calcium overload in human leucocytes: changes with age. Basic Clin Pharmacol Toxicol. (2010) 107:590–7. doi: 10.1111/j.1742-7843.2010.00546.x
162. Yimam M, Horm T, O'Neal A, Chua P, Jiao P, Hong M, et al. Botanical bioflavonoid composition from Scutellaria baicalensis- and Acacia catechu-protected mice against D-galactose-induced immunosenescence, and cyclophosphamide induced immune suppression. Nutrients. (2024) 16:3144. doi: 10.3390/nu16183144
163. Cillóniz C, Rodríguez-Hurtado D, Torres A. Characteristics and management of community-acquired pneumonia in the era of global aging. Med Sci. (2018) 6:35. doi: 10.3390/medsci6020035
164. Li G, Ding K, Qiao Y, Zhang L, Zheng L, Pan T, et al. Flavonoids regulate inflammation and oxidative stress in cancer. Molecules. (2020) 25:5628. doi: 10.3390/molecules25235628
165. Zhou Y, Qian C, Tang Y, Song M, Zhang T, Dong G, et al. Advance in the pharmacological effects of quercetin in modulating oxidative stress and inflammation related disorders. Phytother Res. (2023) 37:4999–5016. doi: 10.1002/ptr.7966
166. Bouamama S, Bouamama A. Quercetin handles cellular oxidant/antioxidant systems and mitigates immunosenescence hallmarks in human PBMCs: an in vitro study. J Biochem Mol Toxicol. (2023) 37:e23354. doi: 10.1002/jbt.23354
167. Won HK, Yoon SJ, Song WJ. The double-sidedness of cough in the elderly. Respir Physiol Neurobiol. (2018) 257:65–9. doi: 10.1016/j.resp.2018.01.009
168. Okazaki T, Suzukamo Y, Miyatake M, Komatsu R, Yaekashiwa M, Nihei M, et al. Respiratory muscle weakness as a risk factor for pneumonia in older people. Gerontology. (2021) 67:581–90. doi: 10.1159/000514007
169. Fujishima I, Fujiu-Kurachi M, Arai H, Hyodo M, Kagaya H, Maeda K, et al. Sarcopenia and dysphagia: position paper by four professional organizations. Geriatr Gerontol Int. (2019) 19:91–7. doi: 10.1111/ggi.13591
170. Sakai Y, Ohira M, Yokokawa Y. Cough strength is an indicator of aspiration risk when restarting food intake in elderly subjects with community-acquired pneumonia. Respir Care. (2020) 65:169–76. doi: 10.4187/respcare.07067
171. Okazaki T, Ebihara S, Mori T, Izumi S, Ebihara T. Association between sarcopenia and pneumonia in older people. Geriatr Gerontol Int. (2020) 20:7–13. doi: 10.1111/ggi.13839
172. Sasaki KI, Fukumoto Y. Sarcopenia as a comorbidity of cardiovascular disease. J Cardiol. (2022) 79:596–604. doi: 10.1016/j.jjcc.2021.10.013
173. Pacifico J, Geerlings MAJ, Reijnierse EM, Phassouliotis C, Lim WK, Maier AB. Prevalence of sarcopenia as a comorbid disease: a systematic review and meta-analysis. Exp Gerontol. (2020) 131:110801. doi: 10.1016/j.exger.2019.110801
174. Franceschi C, Garagnani P, Morsiani C, Conte M, Santoro A, Grignolio A, et al. The continuum of aging and age-related diseases: common mechanisms but different rates. Front Med. (2018) 5:61. doi: 10.3389/fmed.2018.00061
175. Wilson D, Jackson T, Sapey E, Lord JM. Frailty and sarcopenia: the potential role of an aged immune system. Ageing Res Rev. (2017) 36:1–10. doi: 10.1016/j.arr.2017.01.006
176. Clegg A, Young J, Iliffe S, Rikkert MO, Rockwood K. Frailty in elderly people. Lancet. (2013) 381:752–62. doi: 10.1016/S0140-6736(12)62167-9
177. Morley JE, Vellas B, van Kan GA, Anker SD, Bauer JM, Bernabei R, et al. Frailty consensus: a call to action. J Am Med Dir Assoc. (2013) 14:392–7. doi: 10.1016/j.jamda.2013.03.022
178. Cesari M, Calvani R, Marzetti E. Frailty in older persons. Clin Geriatr Med. (2017) 33:293–303. doi: 10.1016/j.cger.2017.02.002
179. Buchmann N, Spira D, König M, Demuth I, Steinhagen-Thiessen E. Frailty and the metabolic syndrome - results of the berlin aging study II (BASE-II). J Frailty Aging. (2019) 8:169–75. doi: 10.14283/jfa.2019.15
180. Bernabeu-Wittel M, Gómez-Díaz R, González-Molina Á, Vidal-Serrano S, Díez-Manglano J, Salgado F, et al. Oxidative stress, telomere shortening, and apoptosis associated to sarcopenia and frailty in patients with multimorbidity. J Clin Med. (2020) 9:2669. doi: 10.3390/jcm9082669
181. Deepa SS, Van Remmen H, Brooks SV, Faulkner JA, Larkin L, McArdle A, et al. Accelerated sarcopenia in Cu/Zn superoxide dismutase knockout mice. Free Radic Biol Med. (2019) 132:19–23. doi: 10.1016/j.freeradbiomed.2018.06.032
182. Zhang H, Qi G, Wang K, Yang J, Shen Y, Yang X, et al. Oxidative stress: roles in skeletal muscle atrophy. Biochem Pharmacol. (2023) 214:115664. doi: 10.1016/j.bcp.2023.115664
183. Saud Gany SL, Chin KY, Tan JK, Aminuddin A, Makpol S. Preventative and therapeutic potential of tocotrienols on musculoskeletal diseases in ageing. Front Pharmacol. (2023) 14:1290721. doi: 10.3389/fphar.2023.1290721
184. Stacchiotti A, Favero G, Rodella LF. Impact of melatonin on skeletal muscle and exercise. Cells. (2020) 9:288. doi: 10.3390/cells9020288
185. Dziegielewska-Gesiak S, Muc-Wierzgoń M. Inflammation and oxidative stress in frailty and metabolic syndromes-two sides of the same coin. Metabolites. (2023) 13:475. doi: 10.3390/metabo13040475
186. Soysal P, Arik F, Smith L, Jackson SE, Isik AT. Inflammation, frailty and cardiovascular disease. Adv Exp Med Biol. (2020) 1216:55–64. doi: 10.1007/978-3-030-33330-0_7
187. Oei S, Millar CL, Nguyen Lily TN, Mukamal KJ, Kiel DP, Lipsitz LA, et al. Higher intake of dietary flavonols, specifically dietary quercetin, is associated with lower odds of frailty onset over 12 years of follow-up among adults in the Framingham heart study. Am J Clin Nutr. (2023) 118:27–33. doi: 10.1016/j.ajcnut.2023.04.013
188. Martemucci G, Portincasa P, Di Ciaula A, Mariano M, Centonze V, D'Alessandro AG. Oxidative stress, aging, antioxidant supplementation and their impact on human health: an overview. Mech Ageing Dev. (2022) 206:111707. doi: 10.1016/j.mad.2022.111707
189. Mustafa YF. Harmful free radicals in aging: a narrative review of their detrimental effects on health. Indian J Clin Biochem. (2024) 39:154–67. doi: 10.1007/s12291-023-01147-y
190. Chowdhury SR, Chandra Das D, Sunna TC, Beyene J, Hossain A. Global and regional prevalence of multimorbidity in the adult population in community settings: a systematic review and meta-analysis. EClinicalMedicine. (2023) 57:101860. doi: 10.1016/j.eclinm.2023.101860
191. Rojas-Solé C, Pinilla-González V, Lillo-Moya J, González-Fernández T, Saso L, Rodrigo R. Integrated approach to reducing polypharmacy in older people: exploring the role of oxidative stress and antioxidant potential therapy. Redox Rep. (2024) 29:2289740. doi: 10.1080/13510002.2023.2289740
192. Delara M, Murray L, Jafari B, Bahji A, Goodarzi Z, Kirkham J, et al. Prevalence and factors associated with polypharmacy: a systematic review and meta-analysis. BMC Geriatr. (2022) 22:601. doi: 10.1186/s12877-022-03279-x
193. Lei T, Lu T, Yu H, Su X, Zhang C, Zhu L, et al. Efficacy of vitamin C supplementation on chronic obstructive pulmonary disease (COPD): a systematic review and meta-analysis. Int J Chron Obstruct Pulmon Dis. (2022) 17:2201–16. doi: 10.2147/COPD.S368645
194. Chin KY, Pang KL, Soelaiman IN. Tocotrienol and its role in chronic diseases. Adv Exp Med Biol. (2016) 928:97–130. doi: 10.1007/978-3-319-41334-1_5
195. Aghababaei F, Hadidi M. Recent advances in potential health benefits of quercetin. Pharmaceuticals. (2023) 16:1020. doi: 10.3390/ph16071020
196. Sadeghi S, Hakemi MS, Pourrezagholie F, Naeini F, Imani H, Mohammadi H. Effects of melatonin supplementation on metabolic parameters, oxidative stress, and inflammatory biomarkers in diabetic patients with chronic kidney disease: study protocol for a double-blind, randomized controlled trial. Trials. (2024) 25:757. doi: 10.1186/s13063-024-08584-x
197. Ohashi N, Ishigaki S, Isobe S. The pivotal role of melatonin in ameliorating chronic kidney disease by suppression of the renin-angiotensin system in the kidney. Hypertens Res. (2019) 42:761–8. doi: 10.1038/s41440-018-0186-2
198. Aaseth J, Alexander J, Alehagen U. Coenzyme Q(10) supplementation - in ageing and disease. Mech Ageing Dev. (2021) 197:111521. doi: 10.1016/j.mad.2021.111521
199. Fenton R, Brook-Barclay L, Delaney CL, Spark JI, Miller MD. Do medications commonly prescribed to patients with peripheral arterial disease have an effect on nutritional status? A review of the literature. Ann Vasc Surg. (2016) 32:145–75. doi: 10.1016/j.avsg.2015.10.036
200. Little MO. Updates in nutrition and polypharmacy. Curr Opin Clin Nutr Metab Care. (2018) 21:4–9. doi: 10.1097/MCO.0000000000000425
201. Błeszyńska E, Wierucki Ł, Zdrojewski T, Renke M. Pharmacological interactions in the elderly. Medicina. (2020) 56:320. doi: 10.3390/medicina56070320
202. Wieczfinska J, Kleniewska P, Pawliczak R. Oxidative stress-related mechanisms in SARS-CoV-2 infections. Oxid Med Cell Longev. (2022) 2022:5589089. doi: 10.1155/2022/5589089
203. Georgieva E, Ananiev J, Yovchev Y, Arabadzhiev G, Abrashev H, Abrasheva D, et al. COVID-19 complications: oxidative stress, inflammation, and mitochondrial and endothelial dysfunction. Int J Mol Sci. (2023) 24:14876. doi: 10.3390/ijms241914876
204. Xiong Q, Xu M, Li J, Liu Y, Zhang J, Xu Y, et al. Clinical sequelae of COVID-19 survivors in Wuhan, China: a single-centre longitudinal study. Clin Microbiol Infect. (2021) 27:89–95. doi: 10.1016/j.cmi.2020.09.023
205. Kamal M, Abo Omirah M, Hussein A, Saeed H. Assessment and characterisation of post-COVID-19 manifestations. Int J Clin Pract. (2021) 75:e13746. doi: 10.1111/ijcp.13746
206. Chen C, Haupert SR, Zimmermann L, Shi X, Fritsche LG, Mukherjee B. Global prevalence of post-coronavirus disease 2019 (COVID-19) condition or long COVID: a meta-analysis and systematic review. J Infect Dis. (2022) 226:1593–607. doi: 10.1093/infdis/jiac136
207. Tosato M, Ciciarello F, Zazzara MB, Pais C, Savera G, Picca A, et al. Nutraceuticals and dietary supplements for older adults with long COVID-19. Clin Geriatr Med. (2022) 38:565–91. doi: 10.1016/j.cger.2022.04.004
208. Jung JH, Park JH, Park KH. Comparison of lifestyle, cognitive function, mental health, and quality of life between hospitalized older adults with COVID-19 and non-COVID-19 in South Korea: a cross-sectional study. BMC Geriatr. (2024) 24:306. doi: 10.1186/s12877-023-04646-y
209. Yiang GT, Wu CC, Lu CL, Hu WC, Tsai YJ, Huang YM, et al. Endoplasmic reticulum stress in elderly patients with COVID-19: potential of melatonin treatment. Viruses. (2023) 15:156. doi: 10.3390/v15010156
210. Chavarría AP, Vázquez RRV, Cherit JGD, Bello HH, Suastegui HC, Moreno-Castañeda L, et al. Antioxidants and pentoxifylline as coadjuvant measures to standard therapy to improve prognosis of patients with pneumonia by COVID-19. Comput Struct Biotechnol J. (2021) 19:1379–90. doi: 10.1016/j.csbj.2021.02.009
211. Vazquez-Agra N, Marques-Afonso AT, Cruces-Sande A, Novo-Veleiro I, Pose-Reino A, Mendez-Alvarez E, et al. Assessment of oxidative stress markers in elderly patients with SARS-CoV-2 infection and potential prognostic implications in the medium and long term. PLoS ONE. (2022) 17:e0268871. doi: 10.1371/journal.pone.0268871
212. Li L, Wang X, Guo X, Li Y, Song Q, Li A. Network pharmacology and computer-aided drug design to explored potential targets of Lianhua Qingwen and Qingfei Paidu decoction for COVID-19. Front Pharmacol. (2022) 13:1013428. doi: 10.3389/fphar.2022.1013428
213. Niki E. Do antioxidants impair signaling by reactive oxygen species and lipid oxidation products? FEBS Lett. (2012) 586:3767–70. doi: 10.1016/j.febslet.2012.09.025
214. Ku JW, Gan YH. Modulation of bacterial virulence and fitness by host glutathione. Curr Opin Microbiol. (2019) 47:8–13. doi: 10.1016/j.mib.2018.10.004
215. Michie KL, Dees JL, Fleming D, Moustafa DA, Goldberg JB, Rumbaugh KP, et al. Role of Pseudomonas aeruginosa glutathione biosynthesis in lung and soft tissue infection. Infect Immun. (2020) 88:e00116–20. doi: 10.1128/IAI.00116-20
216. Levine M, Conry-Cantilena C, Wang Y, Welch RW, Washko PW, Dhariwal KR, et al. Vitamin C pharmacokinetics in healthy volunteers: evidence for a recommended dietary allowance. Proc Natl Acad Sci U S A. (1996) 93:3704–9. doi: 10.1073/pnas.93.8.3704
217. Cotter MA, Thomas J, Cassidy P, Robinette K, Jenkins N, Florell SR, et al. N-acetylcysteine protects melanocytes against oxidative stress/damage and delays onset of ultraviolet-induced melanoma in mice. Clin Cancer Res. (2007) 13:5952–8. doi: 10.1158/1078-0432.CCR-07-1187
Keywords: community-acquired pneumonia, older adults, oxidative stress, inflammation, aging
Citation: Mao W, Liu X, Fan S, Zhang R, Liu M and Xiao S (2025) Modulating oxidative stress: a reliable strategy for coping with community-acquired pneumonia in older adults. Front. Med. 12:1549658. doi: 10.3389/fmed.2025.1549658
Received: 21 December 2024; Accepted: 11 March 2025;
Published: 26 March 2025.
Edited by:
Matteo Becatti, University of Firenze, ItalyReviewed by:
Laura Vitiello, IRCCS San Raffaele Roma, ItalyCopyright © 2025 Mao, Liu, Fan, Zhang, Liu and Xiao. This is an open-access article distributed under the terms of the Creative Commons Attribution License (CC BY). The use, distribution or reproduction in other forums is permitted, provided the original author(s) and the copyright owner(s) are credited and that the original publication in this journal is cited, in accordance with accepted academic practice. No use, distribution or reproduction is permitted which does not comply with these terms.
*Correspondence: Shunqiong Xiao, bWUxb2RyYW1hQDE2My5jb20=
†These authors have contributed equally to this work
Disclaimer: All claims expressed in this article are solely those of the authors and do not necessarily represent those of their affiliated organizations, or those of the publisher, the editors and the reviewers. Any product that may be evaluated in this article or claim that may be made by its manufacturer is not guaranteed or endorsed by the publisher.
Research integrity at Frontiers
Learn more about the work of our research integrity team to safeguard the quality of each article we publish.