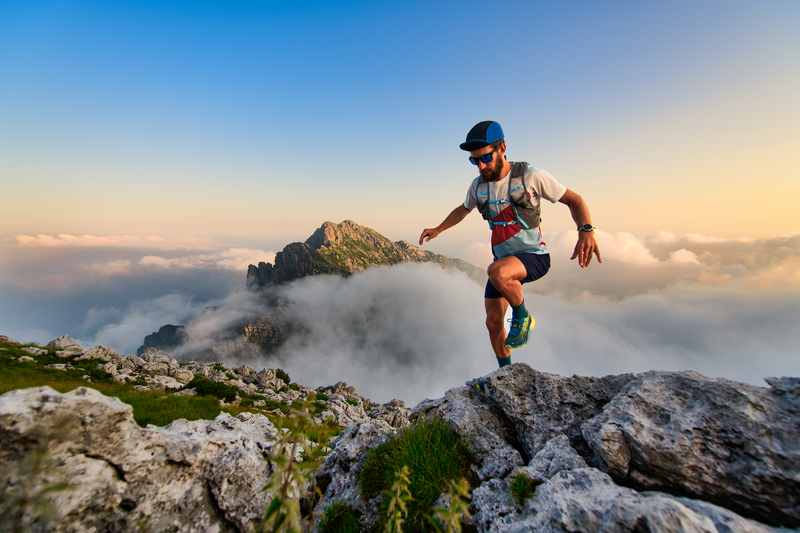
95% of researchers rate our articles as excellent or good
Learn more about the work of our research integrity team to safeguard the quality of each article we publish.
Find out more
REVIEW article
Front. Med. , 24 January 2025
Sec. Infectious Diseases: Pathogenesis and Therapy
Volume 12 - 2025 | https://doi.org/10.3389/fmed.2025.1536547
This article is part of the Research Topic Tuberculosis: Recent Updates in Basic Research, Drug Discovery and Treatment View all 6 articles
Bone and joint tuberculosis (BJTB), caused by Mycobacterium tuberculosis (MTB), is a prevalent form of extrapulmonary tuberculosis that poses significant challenges to global public health due to difficulties in early diagnosis, prolonged treatment cycles, and drug resistance. Recent advancements in nanotechnology have introduced novel solutions for the early detection and precise treatment of BJTB, leveraging unique physicochemical properties such as high specific surface area, targeted delivery capabilities, sustained drug release, and excellent biocompatibility. In diagnostic applications, nanomaterials markedly enhance the sensitivity and accuracy of detection methods while reducing testing time. These technologies are adaptable to resource-limited settings, enabling earlier patient intervention and mitigating disease progression risk. In therapeutic applications, nanomaterials prolong drug retention in bone tissue through targeted delivery, thereby decreasing medication frequency and minimizing toxic side effects, which significantly improves treatment efficacy. Despite substantial progress, further research is required to address long-term safety concerns, broaden clinical applicability, and evaluate performance under complex pathological conditions. This review summarizes recent advancements in nanomaterials for diagnosing and treating BJTB and identifies key areas for future research, laying the groundwork for advancing precision medicine and personalized treatments.
Tuberculosis (TB) is an infectious disease with a rich historical background, dating back to the identification of spinal tuberculosis in Egyptian mummies from 3,000 BC (1). Mycobacterium tuberculosis (Mtb) is the main pathogen of tuberculosis, which spreads through the air and is engulfed by macrophages after entering the lungs. Some pathogens escape from the immune system and spread to the whole body, causing extrapulmonary tuberculosis. Bone and joint tuberculosis are more common, mainly involving the spine and large joints, which can lead to dysfunction and decreased quality of life in severe cases. Despite significant advancements in modern medicine for TB prevention and treatment, the global incidence remains high, with over 10 million new infections reported annually (2). The occurrence of TB is closely associated with socioeconomic development levels, particularly affecting vulnerable populations such as the elderly and individuals living with AIDS who face a significantly higher risk of infection (3–5). Pulmonary tuberculosis remains the most prevalent form, whereas bone and joint tuberculosis ranks third among extrapulmonary cases, underscoring its clinical significance (6). The immunosuppressive effects of HIV substantially exacerbate the spread and progression of tuberculosis (7). Research data indicate that HIV-positive individuals have a 1.42-fold higher risk of developing TB compared to HIV-negative individuals (8). This association underscores the critical role of the immune system in defending against Mycobacterium tuberculosis (Mtb) and highlights the complexity and severity of HIV/TB co-infection on a global scale.
The rising prevalence of multidrug-resistant tuberculosis (MDR-TB) presents significant challenges in the diagnosis and management of bone and joint tuberculosis, often resulting from incomplete or inadequate treatment regimens (9–11). Traditional diagnostic methods are limited by prolonged processing times, while treatment options are constrained by poor drug penetration into bone tissue and the extended duration required for therapy. Additionally, adverse effects associated with antituberculosis drugs can lead to reduced patient adherence to treatment protocols (12). These challenges have spurred researchers to investigate novel therapeutic strategies to enhance clinical outcomes for patients with bone and joint tuberculosis.
In this context, nanomaterials have introduced novel possibilities for the treatment of bone and joint tuberculosis. Nanomaterials possess distinctive characteristics, including enhanced targeted drug delivery, adjustable drug release rates, prolonged drug exposure, and improved drug absorption (13). They can serve as slow-release delivery systems (14, 15) for precise drug administration to infected bone tissue, thereby significantly enhancing drug permeability and therapeutic efficacy while effectively reducing toxicity (16, 95).
Considering the potential of nanomaterials in treating bone and joint tuberculosis and their profound impact on human health, it is crucial to explore their mechanisms of action and potential applications. This article comprehensively examines the pathogenesis, traditional diagnosis and treatment approaches for bone and joint tuberculosis while focusing on the advancements made in utilizing nanomaterials in this field. Furthermore, future research directions and challenges are anticipated.
Bone and joint tuberculosis is a widespread secondary extrapulmonary form of tuberculosis, primarily caused by local inflammation resulting from the hematogenous spread of Mycobacterium tuberculosis (Mtb) to bones or joints (17). The respiratory tract serves as the main route for Mtb transmission, wherein inhalation of droplets containing bacteria leads to colonization in the alveoli (18, 19). Macrophages attempt to eliminate invading Mtb by recruiting immune cells like monocytes, lymphocytes, and neutrophils (20). However, certain bacteria can evade clearance by the immune system, forming granulomas that restrict their proliferation and enter a dormant state (21). The effectiveness of host cell-mediated immune response during this stage plays a crucial role in determining disease control or progression (22). Subsequently, when host immunity declines significantly due to factors such as HIV infection or aging over subsequent years or even decades (23). There are two primary modes of transmission. Firstly, during the initial pulmonary infection, Mtb disseminates via the bloodstream to the bone and enters a dormant state. Under appropriate conditions, this latent infection within the bone tissue can be directly reactivated. Secondly, latent infections in the lungs may reactivate and subsequently spread to the bones through either the bloodstream or the lymphatic system (22). Regardless of the form, Mtb tends to invade the spine and large joints following its entry into bone tissue (24). Upon entering the joint cavity, Mtb typically initiates erosion from surrounding synovial tissues before gradually spreading into cartilage and bone tissues (Figure 1). This erosive process often persists for several months leading to severe disruption of local tissue architecture (25).
Figure 1. Pathogenesis of bone and joint tuberculosis. Created in BioRender. ding, y. (2025) https://BioRender.com/v66b991.
Given the aforementioned pathological process, early diagnosis and timely intervention play a pivotal role in managing bone and joint tuberculosis. By promptly identifying and treating the condition, disease progression can be effectively halted, mitigating irreversible tissue damage and ultimately enhancing patient prognosis and quality of life (26). Consequently, it is imperative to explore efficient and accurate early diagnostic technologies as well as optimize treatment plans to address the challenges posed by bone and joint tuberculosis (27).
The clinical manifestations of bone and joint tuberculosis are intricate and diverse, with spinal tuberculosis being the most prevalent, followed by tuberculous arthritis and extracorporeal tuberculous osteomyelitis (28). Currently, the diagnosis of bone and joint tuberculosis primarily relies on comprehensive approaches such as medical history collection, imaging examination, and hematologic analysis (29). However, these methods have certain limitations at different disease stages, which can impact the accuracy and timeliness of diagnosis. In the early stages of the disease, patients often present with localized dull pain and swelling alone, potentially accompanied by constitutional symptoms like fever, night sweats, and anemia that are associated with tuberculosis (30). Nevertheless, these symptoms lack specificity and can be easily mistaken for other conditions. Furthermore, patients with bone or joint tuberculosis frequently exhibit pathological changes such as caseous necrosis that hinder prompt detection through techniques like smears or bacterial culture—although considered diagnostic gold standards—due to their time-consuming nature often taking weeks or longer (31, 32).
Therefore, the early diagnosis of bone and joint tuberculosis poses not only significant challenges but also a high rate of misdiagnosis. Untimely identification and treatment of bone and joint tuberculosis can result in severe consequences. For instance, when lesions affect the nervous system, patients may experience irreversible neurological damage leading to functional impairment (33, 34). In advanced stages of the disease, patients commonly present with pronounced joint pain, deformity, dislocation, and substantial limitations in daily activities. Imaging techniques such as X-rays often reveal marked reduction in joint space accompanied by destructive changes (35). Progression of the disease may even lead to fibrous ankylosis of the joint resulting in complete loss of joint function (36).
In conclusion, multiple factors limit early-stage diagnosis of bone and joint tuberculosis while advanced stages significantly impact patient quality of life and functional prognosis. Henceforth, optimizing existing diagnostic methods and exploring novel techniques hold great significance for enhancing clinical outcomes.
Currently, the management of bone and joint tuberculosis primarily involves first-line anti-tuberculosis drugs, second-line anti-tuberculosis drugs, and surgical intervention when necessary. The foundation of routine therapy consists of first-line agents such as isoniazid (INH), pyrazinamide (PZA), rifampin (RIF), and ethambutol (EMB) (37). Second-line drugs are employed in cases where initial treatment fails or resistance develops. These include injectable agents (streptomycin, kanamycin, amikacin, capreomycin, and viomycin), fluoroquinolones (e.g., ofloxacin, levofloxacin, gatifloxacin, and moxifloxacin), as well as other oral agents (e.g., ethionamide, prothionamide, cycloserine, terizidone, and p-aminosalicylic acid) (38, 39). According to current standards, treatment duration for bone and joint tuberculosis is prolonged. Typically, the minimum duration for antituberculosis drug therapy is 8 months; however, a complete course of chemotherapy requires at least 20 months (40). Nevertheless, this extended treatment period poses a significant challenge to patient adherence. Many patients may discontinue treatment due to stress, resulting in the emergence and dissemination of drug-resistant strains. This has become one of the major challenges in global tuberculosis management (41).
There are various routes of administration for anti-tuberculosis drugs, each with its own set of advantages and disadvantages (42, 43). Oral administration is the most commonly employed method; however, it exhibits slow efficacy and low bioavailability. High oral doses not only contribute to the proliferation of drug-resistant bacteria but also lead to significant toxic side effects such as hepatotoxicity and gastrointestinal discomfort (44). In contrast, parenteral administration offers improved absorption and effectiveness but necessitates the presence of a healthcare professional, often causing discomfort and pain during the procedure. Surgical intervention is recommended in selected cases, particularly for complex situations like deformity correction or neurological impairment, as it can promptly alleviate pain and restore function. Nevertheless, surgical treatment is not universally suitable and typically limited to conditions where clear indications exist, such as established deformities or neurologic impairments (45, 46).
In conclusion, current treatment options partially control bone and joint tuberculosis; however, their limitations are evident. Prolonged treatment duration coupled with drug toxicity leads to poor patient compliance while drug resistance exacerbates treatment challenges. Although surgery may serve as an adjunctive therapy under specific circumstances, it does not apply universally to all patients (Table 1). Therefore, future research should focus on developing more efficient and tolerable treatment strategies along with optimizing existing therapeutic approaches.
With the rapid advancement of science and technology, nanotechnology has emerged as a frontier in modern medicine with great potential and application value (47, 48). Due to their unique physicochemical properties such as high specific surface area, good biocompatibility, targeting ability, and controlled drug release capability, nanomaterials play a crucial role in the diagnosis and treatment of bone and joint tuberculosis (49, 50). Nanomaterials can be classified into two categories: organic and inorganic (51). Organic nanomaterials like liposomes have gained significant attention due to their excellent biosafety and compatibility (52); however, inorganic nanomaterials such as mesoporous silica are more prominent for bone tissue targeted therapy because of their high stability and drug delivery efficiency (53).
In the field of diagnostics, several novel nanotechnologies have significantly enhanced the sensitivity and specificity of tests (Figure 2). Within the therapeutic domain, nanomaterials present patients with more efficient and safer treatment alternatives by optimizing targeted delivery systems (54). In recent years, extensive exploration and development of various nanoformulations have led to their clinical application in bone and joint tuberculosis management (55, 56). These nanomaterials not only augment the therapeutic efficacy of antituberculosis drugs within bone tissue but also substantially reduce drug frequency and dosage, thereby mitigating the risk of drug resistance and toxicity (57, 58). Furthermore, integration of nanomaterials into scaffolds provides porous carriers that enhance biocompatibility while promoting bone tissue regeneration (59). These groundbreaking innovations hold immense potential for early detection and effective treatment of bone and joint tuberculosis, ultimately improving patient prognosis and quality of life.
Figure 2. Application of nanomaterials in the diagnosis of bone and joint tuberculosis. Created in BioRender. ding, y. (2025) https://BioRender.com/f06p848.
Palladium-platinum bimetallic nanoparticles (Pd@Pt NPs) have garnered considerable attention as an emerging nanotechnology due to their exceptional performance in catalysis and biosensing applications. The incorporation of palladium into platinum, forming a bimetallic structure, endows Pd@Pt NPs with peroxidase-like properties and significantly enhances the reactive surface area when interacting with substrates such as 3,3′,5,5′-tetramethylbenzidine (TMB) (60). Compared to alternative materials, Pd@Pt NPs exhibit several distinct advantages, such as superior sensitivity, rapid detection capabilities, cost-effectiveness, portability, and visualization features (61). Its porous structure not only offers a high specific surface area but also demonstrates superior catalytic activity and efficiency, thereby significantly enhancing detection performance (62, 63). Furthermore, serving as nanozymes enables them to exhibit long-term storage stability and withstand extreme environments effectively expanding their range of applications (64). Although Pd@Pt NPs have shown great potential in the fields of catalysis and biosensing, the complex preparation process and strict conditions limit their feasibility in large-scale applications.
In Cy et al.’s study (65), Pd@Pt nanoparticles were integrated into a multilayered paper-based assay device and DNA hybridization assay system for the detection of Mycobacterium tuberculosis (Mtb). The results demonstrated that the Pd@Pt nanoparticles exhibited superior catalytic efficiency and enabled target DNA detection within 15 min compared to conventional methods. This diagnostic approach holds significant potential in resource-limited medical settings, providing an efficient and convenient tool for detecting tuberculosis in remote or developing areas. Early diagnosis of bone and joint tuberculosis can reduce bone destruction and improve patient prognosis.
Gold nanoparticles (AuNPs) have emerged as a superior alternative to traditional fluorescence and isotope technology for nucleic acid detection in recent years due to their exceptional stability, efficient nucleic acid hybridization ability, straightforward operational process, making them a rapid and reliable tool (66). Compared to conventional techniques, AuNPs can provide results within approximately 30 min and have been extensively employed for pathogen nucleic acid detection including Mtb (67). However, due to the high cost associated with Au as a precious metal, the widespread adoption of AuNPs in large-scale clinical diagnostics is constrained. In a recent study, Pedrosa et al. (68) simultaneously amplified the rpoB531 and inhA C-15T genes of Mtb using multiplex PCR and utilized gold nanoparticle probe chips for detecting drug resistance gene mutations. The findings demonstrated that this method exhibited a detection consistency rate of up to 100% for rifampin-resistant and sensitive samples while achieving an accuracy rate of 84% for isoniazid-resistant samples, thereby highlighting the potential clinical application value of gold nanoparticle probes in rapidly identifying gene mutations in Mtb.
Enhanced diagnostic methods for bone and joint tuberculosis are urgently needed to overcome the limitations of low sensitivity in traditional approaches. Despite the potential of antigen-detection techniques, there remain significant challenges in isolating and preserving highly specific monoclonal antibodies (69). By integrating nanomaterials into aptamer sensors, these issues can be effectively addressed, leading to a substantial improvement in antigen detection efficiency (70).
In Huang et al.‘s (71) study, researchers successfully developed a dual-signal output aptamer sensor based on MXene/C60NPs/Au@Pt nanocomposites for ultrasensitive detection of ESAT-6 antigen. Leveraging its exceptional REDOX activity and catalytic properties, this nanocomposite exhibited superior specificity and accuracy in distinguishing healthy donors from patients with other lung diseases as well as TB patients. Experimental results demonstrated an impressive system sensitivity of 97.5% and specificity of 96.7%, surpassing that achieved by conventional diagnostic methods. This innovative sensor represents a valuable tool for efficient and reliable clinical diagnosis of bone and joint tuberculosis while also holding great promise for early screening, precision diagnosis, and treatment strategies. However, there are still some potential problems that need to be solved in the practical application of this nanocomposite. For example, its biocompatibility and safety for long-term use have not been fully studied, and there may be a risk of cytotoxicity or environmental effects.
Enhanced ultrasensitive fluorescence quantification through synergistic amplification of nanomaterials enables dual targeting for improved diagnostic performance in tuberculosis (TB). Traditional diagnostic methods, such as Mtb culture, CT imaging studies, tuberculin skin tests (TST), and interferon-γ release assays (IGRAs), have inherent limitations (72). To overcome these limitations and enhance sensitivity and specificity for TB infection detection, additional biomarkers have been integrated into IGRAs. Notably, the upregulation of IFN-γ-inducible protein 10 (IP-10) in response to Mtb stimulation offers a promising avenue for higher accuracy. The combined detection of IFN-γ and IP-10 further enhances the ability to differentiate active TB cases from healthy individuals (73).
In Shi et al.‘s (74) study, a dual-target strategy was implemented to simultaneously detect IP-10 and IFN-γ in patient blood samples using selective recognition of fluorescent nanomaterials and enzyme-free nucleic acid amplification. Compared to the conventional IGRA method, this approach reduces detection time by at least 12 h and significantly enhances diagnostic efficiency through the specific recognition of aptamers. This methodology presents a novel avenue for rapid diagnosis and clinical decision-making in cases of bone and joint tuberculosis. However, compared to traditional methods, this strategy may necessitate more advanced technical and equipment support, which presents additional challenges for implementation in primary care settings.
Chitosan, a naturally-derived biopolymer obtained through N-deacetylation of chitin, can be extracted from crustaceans and aquatic microorganisms (75). Chemically, chitosan is a linear binary heteropolysaccharide composed of β-1,4-linked D-glucosamine (deacetyl unit) and N-acetyl-D-glucosamine (acetyl unit) (76). Due to its exceptional antibacterial properties, chitosan exhibits significant activity against Gram-negative bacteria, Gram-positive bacteria, and fungi (77, 78). Moreover, the drug carrier potential of chitosan is greatly enhanced by its pH-dependent transport capacity, providing it with a notable advantage in pharmaceutical applications (79). The natural glycosaminoglycan structure of chitosan contributes to its excellent biocompatibility as it facilitates easy breakdown and absorption within the human body (80, 81).
The delivery of rifampin was targeted using a chitosan nanoparticle system in a study (82), wherein the conjugation of mannose as a ligand to chitosan significantly enhanced the uptake efficiency of particles by macrophages. By combining this system with an in situ gel prepared by Poloxamer 407 and HPMC K4M, the drug release time was extended to 40 h. In a simulated synovial fluid model, the system exhibited preferential uptake by macrophages and efficient release of rifampicin, resulting in Mycobacterium tuberculosis (Mtb) eradication. Compared to conventional drug administration, chitosan nanoparticles demonstrate superior efficacy and reduced toxicity, exemplifying successful integration of materials science and pharmacy for bone and joint tuberculosis treatment. Nevertheless, the application of chitosan still faces several significant challenges. Firstly, given that chitosan is primarily derived from crustaceans, its potential to induce allergic reactions warrants careful consideration. Additionally, its long-term biocompatibility and potential toxicity have not been thoroughly and systematically evaluated. In particular, high-dose or prolonged use may pose hidden risks of cytotoxicity or environmental impact.
l Bovine serum albumin (BSA) is an acidic protein that is widely distributed in the body fluids and tissues of mammals (83). It consists of a single polypeptide chain with three distinct domains containing multiple drug-binding sites, rendering it an optimal vehicle for drug delivery (84). Due to their ready availability, biocompatibility, lack of immunogenicity, and high drug-binding capacity, BSA nanoparticles (BSA NPs) are extensively employed for drug delivery purposes (85, 86). Traditional oral anti-tuberculosis drugs face challenges in effectively reaching bone tissue due to their rapid degradation rate; however, BSA NPs can specifically target liver and spleen tissues, prolonging the residence time of drugs and significantly enhancing their utilization (87).
In a 12-week controlled trial conducted in a New Zealand white rabbit model of spinal tuberculosis (88), the study demonstrated that BSA NPs significantly outperformed conventional treatments in drug delivery efficiency. Pathological examinations revealed a substantial increase in drug concentration in both bone tissue and blood when delivered via BSA NPs. After 12 weeks of treatment, imaging results indicated complete disappearance of broken bone tissue at the lesion site, with surrounding abscesses and necrotic tissues being fully replaced by normal bone tissue, leading to complete lesion resolution. In contrast, the conventional treatment group exhibited markedly inadequate efficacy. The control group showed incomplete repair of the vertebral body and persistent pathological necrotic nodules in the paravertebral area, underscoring the limited effectiveness of traditional treatment regimens for spinal tuberculosis. These findings highlight the significant advantages of BSA NPs in enhancing drug accumulation at the lesion site and improving tissue repair, thereby offering new insights and potential for more effective treatment of spinal tuberculosis. Nevertheless, certain limitations remain in the clinical application of BSA NPs. For instance, under extreme pH or elevated temperature conditions, their structural integrity may be compromised, thereby impacting the stability of drug delivery and therapeutic efficacy.
Mesoporous silica (MSN) is a versatile material characterized by its porous structure, which exhibits a large pore volume (up to 2.5 cmł/g), tunable pore size ranging from 1.3 to 50 nm, high specific surface area (>1,000 m2/g), and exceptional chemical and biological stability (52, 89). These remarkable properties of MSNs enable efficient loading of therapeutic agents, including small molecules, genes, peptides, and proteins, for targeted delivery through electrostatic adsorption or chemical bonding (90, 91).
In a recent study (59), an innovative MSN-based bioscaffold hydrogel system was developed for the controlled release of rifampin and levofloxacin. The results demonstrated that the hydrophobic interaction between the drugs and mesoporous silica nanoparticles (MSNs) significantly extended the drug release duration to approximately 60 days, while effectively mitigating drug resistance and suppressing pathogen proliferation. This strategy exemplifies successful integration of biomaterials with nanotechnology and provides a promising solution for treating bone and joint tuberculosis. However, mesoporous silica nanoparticles (MSNs) exhibit limited biodegradability, and their long-term accumulation in the body may pose toxicity risks, which represent significant challenges that must be addressed prior to clinical application.
Tetracycline (TC) has emerged as an optimal antibacterial agent for targeted bone tissue therapy owing to its strong affinity with hydroxyapatite. It exhibits significant antimicrobial activity against Gram-positive and Gram-negative bacteria, as well as other pathogens, by inhibiting bacterial protein synthesis (92). The distinctive characteristic of tetracycline lies in its selective accumulation in bone tissue, which is further enhanced when combined with nanoparticles, thereby improving the efficiency of targeted drug delivery (93, 94). Polylactic-co-glycolic acid (PLGA) is a hydrophobic synthetic polymer that has gained widespread use in the medical field owing to its superior biodegradability and biocompatibility. The degradation products of PLGA can be safely eliminated via metabolic pathways, rendering it an ideal material for drug delivery. TC-PLGA nanoparticles (TC-PLGA NPs) were synthesized via esterification between the hydroxyl group of tetracycline (TC) and the carboxyl group of PLGA. This system integrates the bone-targeting properties of tetracycline with the advantageous characteristics of PLGA, thereby demonstrating significant potential in the treatment of bone-related diseases (52).
In a study conducted by Liang (95), tetracycline-modified nanoparticles were synthesized using DCC/NHS technology, while rifampicin was employed as the loading drug for control experiments. The experimental results demonstrated that rifapentine-loaded NPs exhibited sustained drug release characteristics for approximately 100 h, in contrast to free rifapentine. The results demonstrated that this nanoparticle system could preferentially accumulate in bone tissue without inducing apparent toxicity and significantly enhance the therapeutic efficacy of the drug. Compared to conventional anti-tuberculosis treatment approaches, this system reduces both the frequency and dosage of drugs administered while minimizing the risk of toxicity, thus providing a more efficient option for treating bone and joint tuberculosis. However, this technique is not without limitations. Firstly, the synthesis process of TC-PLGA nanoparticles is relatively intricate and demands stringent preparation conditions, potentially complicating large-scale production. Secondly, while preliminary experiments have demonstrated low short-term toxicity, further research is necessary to investigate long-term biocompatibility and metabolic characteristics to ensure safety for extended use.
Liposomes are biocompatible delivery systems composed of one or more amphoteric phospholipids, and their unique bilayer structure enables the efficient delivery of both hydrophilic and hydrophobic drugs (96–98). Liposomes possess the ability to encapsulate not only hydrophilic substances within the vesicle core but also hydrophobic materials within a lipid bilayer (99). This exceptional characteristic renders liposomes an outstanding carrier for drug delivery (97) as well as an excellent sustained-release system (100), thereby enhancing drug availability by preventing premature decomposition (101).
In Liu et al.’s (102) studies, a liposome-hydrogel nanoparticle-based sustained-release system was developed and its efficacy was evaluated in New Zealand white rabbits and SD rats. By incorporating liposomes into the hydrogel matrix, this system achieves prolonged and controlled release of isoniazid derivatives while maintaining localized drug concentrations. In vivo drug release experiments demonstrated that the drug concentration in the control group rapidly declined to below 0.3 μg/mL within 24 h, whereas the drug concentration in the liposome-hydrogel system was sustained between 0.48 and 0.61 μg/mL, indicating markedly superior stability. The experimental findings demonstrated that this strategy significantly enhances sustained drug release performance and effectively reduces the frequency of drug administration without altering the properties of the hydrogel. Consequently, this approach offers additional therapeutic options for bone and joint tuberculosis treatment, particularly for patients requiring long-term medication management. Although the liposome-hydrogel system demonstrates superior drug delivery performance, the mechanical strength and degradation rate of the hydrogels can vary across different physiological environments. Further optimization is essential to ensure their reliability under diverse pathological conditions. Different nanoparticles used for bone and joint tuberculosis treatment was summarized in Table 2.
Although nanotechnology has demonstrated significant potential in the diagnosis and treatment of bone and joint tuberculosis, its widespread application still encounters numerous challenges (103). The short experimental duration hinders the complete verification of nanomaterials’ efficacy in long-term treatment, as well as the clarification of their potential toxicity and long-term safety. These uncertainties impede the full clinical implementation of this technique. Therefore, further systematic and long-term experiments are necessary to explore biocompatibility, metabolic pathways, and performance of nanomaterials in complex physiological environments comprehensively. Additionally, to achieve clinical translation of this technology, optimization of the nano-drug delivery system is essential not only for enhancing targeting ability and stability but also for developing cost-effective solutions that are convenient and suitable for low-resource areas.
With its versatility and interdisciplinary characteristics, nanotechnology has opened up a new avenue for precise diagnosis and treatment of bone and joint tuberculosis. From chitosan and bovine serum albumin to mesoporous silica and liposome hydrogels, various nanomaterials have demonstrated significant clinical potential through continuous optimization. These materials not only exhibit notable advantages in drug delivery systems, such as targeted delivery, prolonged drug retention time, and reduced toxic side effects but also facilitate bone tissue regeneration via nanoscaffolds, thereby providing a robust complement to address the limitations of conventional treatment methods (Figure 3).
Figure 3. Various types of nanoparticles utilized in the treatment of bone and joint tuberculosis. Created in BioRender. ding, y. (2025) https://BioRender.com/n85l599.
The development direction of nanomaterials in the diagnosis and treatment of bone and joint tuberculosis is a topic of current interest. Research has demonstrated that nanomaterials offer cutting-edge solutions to overcome the limitations associated with traditional therapies, such as enhancing drug targeting, prolonging drug release time, improving drug efficacy, and enhancing biocompatibility. Notably, precise delivery of chitosan nanoparticles, targeted effect of bovine serum albumin nanoparticles, and synergistic sustained release system involving mesoporous silica and liposome-hydrogel have exhibited significant application advantages.
However, the treatment of bone and joint tuberculosis remains complex and challenging as an infectious disease. Future research should focus on deepening basic scientific exploration, optimizing nanomaterial design, and expediting clinical trial promotion. Additionally, interdisciplinary collaboration will be crucial for driving technological innovation in this field by integrating research findings from various disciplines. By leveraging nanotechnology’s potential to provide comprehensive and accurate solutions in the diagnosis and treatment of bone and joint tuberculosis, more patients can benefit while advancing global medical technology.
YD: Conceptualization, Writing – original draft. BL: Investigation, Writing – original draft. YY: Methodology, Writing – original draft. CL: Formal analysis, Writing – original draft. JW: Resources, Writing – review and editing. XJ: Validation, Writing – review and editing. YL: Supervision, Writing – review and editing.
The authors declare that financial support was received for the research, authorship, and/or publication of this article. This work was supported by the National Natural Science Foundation of China (No. 82072537) and Science project of Hunan Provincial Healthy Commission (No. 20230844).
The authors declare that the research was conducted in the absence of any commercial or financial relationships that could be construed as a potential conflict of interest.
The authors declare that no Generative AI was used in the creation of this manuscript.
All claims expressed in this article are solely those of the authors and do not necessarily represent those of their affiliated organizations, or those of the publisher, the editors and the reviewers. Any product that may be evaluated in this article, or claim that may be made by its manufacturer, is not guaranteed or endorsed by the publisher.
1. Nerlich A, Lösch S. Paleopathology of human tuberculosis and the potential role of climate. Interdiscip Perspect Infect Dis. (2009) 2009:437187. doi: 10.1155/2009/437187
3. Suárez I, Fünger S, Kröger S, Rademacher J, Fätkenheuer G, Rybniker J. The diagnosis and treatment of tuberculosis. Dtsch Arztebl Int. (2019) 116(43):729–35. doi: 10.3238/arztebl.2019.0729
4. Cardona P, Ruiz-Manzano J. On the nature of Mycobacterium tuberculosis-latent bacilli. Eur Respir J. (2004) 24(6):1044–51. doi: 10.1183/09031936.04.00072604
5. Zhang Z, Fan W, Yang G, Xu Z, Wang J, Cheng Q, et al. Risk of tuberculosis in patients treated with TNF-α antagonists: A systematic review and meta-analysis of randomised controlled trials. BMJ Open. (2017) 7(3):e012567. doi: 10.1136/bmjopen-2016-012567
6. Kaur K, Mehta P, Singh A, Sharma P, Thakur K, Singh V, et al. Metabolic switching and cell wall remodelling of Mycobacterium tuberculosis during bone tuberculosis. J Infect. (2023) 86(2):134–46. doi: 10.1016/j.jinf.2022.12.010
7. Udoakang A, Oladipo E, Nwaeze D, Ekanem E, Babalola A, Enemuo P, et al. The COVID-19, tuberculosis and HIV/AIDS: Ménage à trois. Front Immunol. (2023) 14:1104828. doi: 10.3389/fimmu.2023.1104828
8. Zz S, Abate E, Aseffa A, Deribew A, Biadglign SHIV. infection and multidrug resistant tuberculosis: A systematic review and meta-analysis. BMC Infect Dis. (2021) 21:1–13. doi: 10.1186/s12879-021-06532-1
9. Chopra H, Mohanta Y, Rauta P, Ahmed R, Mahanta S, Mishra P, et al. An insight into advances in developing nanotechnology-based therapeutics, drug delivery, diagnostics and vaccines: Multidimensional applications in tuberculosis disease management. Pharmaceuticals (Basel). (2023) 16(4):581. doi: 10.3390/ph16040581
10. Liebenberg D, Gordhan B, Kana B. Drug-resistant tuberculosis: Implications for transmission, diagnosis, and disease management. Front Cell Infect Microbiol. (2022) 12:943545. doi: 10.3389/fcimb.2022.943545
11. Hameed H, Islam M, Chhotaray C, Wang C, Liu Y, Tan Y, et al. Molecular targets related drug resistance mechanisms in MDR-, XDR-, and TDR-Mycobacterium tuberculosis strains. Front Cell Infect Microbiol. (2018) 8:114. doi: 10.3389/fcimb.2018.00114
12. Costa A, Pinheiro M, Magalhães J, Ribeiro R, Seabra V, Reis S, et al. The formulation of nanomedicines for treating tuberculosis. Adv Drug Deliv Rev. (2016) 102:102–15. doi: 10.1016/j.addr.2016.03.003
13. Biswas B, Bhattacharyya S, Saha P, Ghosh S. Current therapeutic delivery approaches using nanocarriers for the treatment of tuberculosis disease. Int J Pharm. (2023) 640:123018. doi: 10.1016/j.ijpharm.2023.123018
14. Chen M, Zhu S, Li H, Xie H. Nanotechnology-based gas delivery system: A ‘green’ strategy for cancer diagnosis and treatment. Theranostics. (2024) 14:5461–91. doi: 10.7150/thno.79523
15. Hua L, Zhu H, Zhang H, Zhang X, Wang L. Anti-tuberculosis drug delivery for tuberculous bone defects. Expert Opin Drug Deliv. (2021) 18(12):1815–27. doi: 10.1080/17425247.2021.1988756
16. Jain S, Gupta T, Rana G, Goyal A. Advances in nanotechnology-based carrier systems for targeted delivery of bioactive drug molecules with special emphasis on immunotherapy in drug-resistant tuberculosis: A critical review. Drug Deliv. (2016) 23(9):3210–20. doi: 10.1080/10717544.2016.1234567
17. Ansari S, Amanullah M, Ahmad K, Rauniyar R. Pott’s Spine: Diagnostic imaging modalities and technology advancements. North Am J Med Sci. (2013) 5(7):404–8. doi: 10.4103/1947-2714.115779
18. Ryndak M, Laal S. Mycobacterium tuberculosis primary infection and dissemination: A critical role for alveolar epithelial cells. Front Cell Infect Microbiol. (2019) 9:299. doi: 10.3389/fcimb.2019.00299
19. Long R, Divangahi M, Schwartzman K. Chapter 2: Transmission and pathogenesis of tuberculosis. Can J Respir Crit Care Sleep Med. (2022) 6(Suppl 1):24–35. doi: 10.1080/24745332.2022.2100003
20. Sy R, Me R, Fj M. Extrapulmonary tuberculosis: Pathophysiology and imaging findings. Radiographics. (2019) 39(7):1975–91. doi: 10.1148/rg.2019190079
21. Leung A. Pulmonary tuberculosis: The essentials. Radiology. (1999) 210(2):307–22. doi: 10.1148/radiology.210.2.9769828
22. Smith I. Mycobacterium tuberculosis pathogenesis and molecular determinants of virulence. Clin Microbiol Rev. (2003) 16(3):463–96. doi: 10.1128/CMR.16.3.463-496.2003
23. Alsayed S, Gunosewoyo H. Tuberculosis: Pathogenesis, current treatment regimens, and new drug targets. Int J Mol Sci. (2023) 24(10):5202. doi: 10.3390/ijms24105202
24. Pigrau-Serrallach C, Rodríguez-Pardo D. Bone and joint tuberculosis. Eur Spine J. (2013) 22(Suppl 4):556–66. doi: 10.1007/s00586-013-2852-9
25. Tuli S. General principles of Osteoarticular tuberculosis. Clin Orthop Relat Res. (2002) 398:11–9. doi: 10.1097/00003086-200205000-00003
26. Wang B, Gao W, Hao D. Current study of the detection and treatment targets of spinal tuberculosis. Curr Drug Targets. (2020) 21(4):320–7. doi: 10.2174/1389450120666200115101158
27. Gan J, Zhang C, Tang D, Du X. Surgical treatment of spinal tuberculosis: An updated review. Eur J Med Res. (2024) 29:588. doi: 10.1186/s40001-024-00699-1
28. Golden M, Vikram H. Extrapulmonary tuberculosis: An overview. Am Fam Physician. (2005) 72(9):1761–8.
29. Liang T, Sun G, Zhang J, Wu W. Immune status changing helps diagnose Osteoarticular tuberculosis. PLoS One. (2021) 16(6):e0252875. doi: 10.1371/journal.pone.0252875
30. Kabore C, Poncin M, Hurtgen B, Moerman F, Moonen M. Osteoarticular tuberculosis nosology and diagnostic pitfalls. Rev Med Liege. (2018) 73(4):191–6.
31. Wang D, An Q, Yang Q, Liao Y, Jian Y. Osteoarticular tuberculosis cases in the southwest of China: A 9-year retrospective study. Front Med. (2023) 10:1051620. doi: 10.3389/fmed.2023.1051620
32. Qi F, Zhao L, Wang Z, Li Y, Liu Y. Combined clinical significance of MRI and serum mannose-binding lectin in the prediction of spinal tuberculosis. BMC Infect Dis. (2024) 24:618. doi: 10.1186/s12879-024-07318-4
33. Johansen I, Nielsen S, Hove M, Kehrer M, Shakar S, Andersen A. Characteristics and clinical outcome of bone and joint tuberculosis from 1994 to 2011: A retrospective register-based study in Denmark. Clin Infect Dis. (2015) 61(4):554–62. doi: 10.1093/cid/civ330
34. Jain A, Rajasekaran S, Jaggi K, Myneedu V. Tuberculosis of the Spine. JBJS. (2020) 102:617–28. doi: 10.2106/JBJS.19.00850
35. Agashe V, Chandak S, Mahadik P, Agashe M. Diagnosis of Osteoarticular tuberculosis: Perceptions, protocols, practices, and priorities in the endemic and non-endemic areas of the world—A WAIOT view. Microorganisms. (2020) 8(4):E564. doi: 10.3390/microorganisms8040564
36. MacGregor-Fairlie M, Wilkinson S, Besra G, Goldberg Oppenheimer P. Tuberculosis diagnostics: Overcoming ancient challenges with modern solutions. Emerg Top Life Sci. (2020) 4(4):423–36. doi: 10.1042/ETLS20200141
37. Chakraborty S, Rhee K. Tuberculosis drug development: History and evolution of the mechanism-based paradigm. Cold Spring Harb Perspect Med. (2015) 5(8):a021147. doi: 10.1101/cshperspect.a021147
38. Kendall EA, Cohen T, Mitnick CD, Dowdy DW. Second-line drug susceptibility testing to inform the treatment of rifampin-resistant tuberculosis: A quantitative perspective. Int J Infect Dis. (2017) 56:92–100. doi: 10.1016/j.ijid.2017.01.020
39. Alsultan A, Peloquin C. Therapeutic drug monitoring in the treatment of tuberculosis: An update. Drugs. (2014) 74(8):839–54. doi: 10.1007/s40265-014-0222-8
40. Wu J, Zhang X, Wang W, Wang J, Huang Z. Development and in vitro characterization of drug delivery system of rifapentine for Osteoarticular tuberculosis. Drug Des Devel Ther. (2015) 9:1359–66. doi: 10.2147/DDDT.S81789
41. Kumar V, Neradi D, Sherry B, Gaurav A, Dhatt S. Tuberculosis of the spine and drug resistance: A review article. Neurosurg Rev. (2022) 45(1):217–29. doi: 10.1007/s10143-021-01469-2
42. Pham D, Fattal E, Tsapis N. Pulmonary drug delivery systems for tuberculosis treatment. Int J Pharm. (2015) 478(2):517–29. doi: 10.1016/j.ijpharm.2014.11.038
43. Cruz A, Starke J. Completion rate and safety of tuberculosis infection treatment with shorter regimens. Pediatrics. (2018) 141(2):e20172838. doi: 10.1542/peds.2017-2838
44. Prabakaran D, Singh P, Jaganathan K, Vyas S. Osmotically regulated asymmetric capsular systems for simultaneous sustained delivery of anti-tubercular drugs. J Control Release. (2004) 95(2):239–48. doi: 10.1016/j.jconrel.2003.11.024
45. Dunn R, Ben Husien M. Spinal tuberculosis: Review of current management. Bone Joint J. (2018) 100-B(4):425–31. doi: 10.1302/0301-620X.100B4.BJJ-2017-1314.R2
46. van Loenhout-Rooyackers J, Verbeek A, Jutte P. Chemotherapeutic treatment for spinal tuberculosis. Int J Tuberc Lung Dis. (2002) 6(3):259–65.
47. Pison U, Welte T, Giersig M, Groneberg D. Nanomedicine for respiratory diseases. Eur J Pharmacol. (2006) 533(1–3):341–50. doi: 10.1016/j.ejphar.2005.12.050
48. Bourguignon T, Godinez-Leon J, Gref R. Nanosized drug delivery systems to fight tuberculosis. Pharmaceutics. (2023) 15(2):393. doi: 10.3390/pharmaceutics15020393
49. Wilson B, Geetha K. Neurotherapeutic applications of nanomedicine for treating Alzheimer’s disease. J Control Release. (2020) 325:25–37. doi: 10.1016/j.jconrel.2020.06.036
50. Wilson B, Paladugu L, Priyadarshini S, Jenita J. Development of albumin-based nanoparticles for the delivery of abacavir. Int J Biol Macromol. (2015) 81:763–7. doi: 10.1016/j.ijbiomac.2015.09.016
51. Desai P, Date A, Patravale V. Overcoming poor oral bioavailability using nanoparticle formulations – opportunities and limitations. Drug Discov Today Technol. (2012) 9:e87–95. doi: 10.1016/j.ddtec.2011.11.002
52. Xu B, Li S, Shi R, Liu H. Multifunctional mesoporous silica nanoparticles for biomedical applications. Signal Transduct Target Ther. (2023) 8:435. doi: 10.1038/s41392-023-01535-7
53. Huang H, Feng W, Chen Y, Shi J. Inorganic nanoparticles in clinical trials and translations. Nano Today. (2020) 35:100972. doi: 10.1016/j.nantod.2020.100972
54. Falsafi S, Rostamabadi H, Assadpour E, Jafari S. Morphology and microstructural analysis of bioactive-loaded micro/nanocarriers via microscopy techniques; CLSM/SEM/TEM/AFM. Adv Colloid Interface Sci. (2020) 280:102166. doi: 10.1016/j.cis.2020.102166
55. Safra T, Muggia F, Jeffers S, Tsao-Wei D, Groshen S, Lyass O, et al. Pegylated liposomal doxorubicin (doxil): Reduced clinical cardiotoxicity in patients reaching or exceeding cumulative doses of 500 mg/m2. Ann Oncol. (2000) 11(8):1029–33. doi: 10.1023/A:1008373922874
56. Zhang Y, Li M, Gao X, Chen Y, Liu T. Nanotechnology in cancer diagnosis: Progress, challenges and opportunities. J Hematol Oncol. (2019) 12(1):137. doi: 10.1186/s13045-019-0833-3
57. Luo Y, Fan W, Guo T, Zhang Y, Li J. Recent advances in nanotechnology-based strategies for bone tuberculosis management. Pharmaceuticals (Basel). (2024) 17(2):170. doi: 10.3390/ph17020170
58. Vashist A, Sharma P, Bhatt A, Kumar N, Singh G, Tiwari S. Recent advances in nanogels for drug delivery and biomedical applications. Biomater Sci. (2024) 12(12):6006–18. doi: 10.1039/D3BM00567F
59. Yahia S, Khalil I, El-Sherbiny I. Dual antituberculosis drugs-loaded gelatin hydrogel bioimplant for treating spinal tuberculosis. Int J Pharm. (2023) 633:122609. doi: 10.1016/j.ijpharm.2023.122609
60. Wu S, Li L, Zhang J, Wang Z, Jiang H. Palladium-platinum bimetallic nanomaterials and their application in Staphylococcus aureus detection on paper-based devices. Biosens Bioelectron. (2022) 216:114669. doi: 10.1016/j.bios.2022.114669
61. Li Y, Zhang L, Zhang X, Yang Z, Lu W. Porous platinum nanoparticles as a high-Z and oxygen generating nanozyme for enhanced radiotherapy in vivo. Biomaterials. (2019) 197:12–9. doi: 10.1016/j.biomaterials.2019.01.021
62. Wang X, Du L, Dong C, Zhang L. Comparative study of Pd@Pt nanozyme improved colorimetric N-ELISA for the paper-output portable detection of Staphylococcus aureus. Talanta. (2022) 247:123503. doi: 10.1016/j.talanta.2022.123503
63. Wang W, Du R, Dong C, Yan J, Zhang LA. modified sensitive ELISA based on dual catalysis of Pd@Pt porous nanoparticles and horseradish peroxidase. Sens Actuators B Chem. (2019) 284:475–84. doi: 10.1016/j.snb.2018.12.077
64. Zhang X, Wu D, Wu Y, Li G. Bioinspired nanozyme for portable immunoassay of allergenic proteins based on a smartphone. Biosens Bioelectron. (2021) 172:112776. doi: 10.1016/j.bios.2020.112776
65. Cy T, Li W, Zhu S, Zhang J, Zhang Q. Diagnosis of Mycobacterium tuberculosis using palladium-platinum bimetallic nanoparticles combined with paper-based analytical devices. Nanoscale. (2024) 16(3):1264–74. doi: 10.1039/D3NR02894A
66. Zahran M, Ahmed A, Hassan A, Saleh M, Awad M. Recent progress in the genotyping of bovine tuberculosis and its rapid diagnosis via nanoparticle-based electrochemical biosensors. RSC Adv. (2023) 13(64):31795–810. doi: 10.1039/D3RA05189K
67. Veigas B, Fernandes A, Baptista P. AuNPs for identification of molecular signatures of resistance. Front Microbiol. (2014) 5:455. doi: 10.3389/fmicb.2014.00455
68. Pedrosa P, Soares D, Baptista P, Fernandes A. Gold nanoprobes for multi loci assessment of multi-drug resistant tuberculosis. Tuberculosis (Edinb). (2014) 94(3):332–7. doi: 10.1016/j.tube.2013.12.007
69. Liu M, Liu R, Shi L, Zhang J, He ZA. novel aptamer-based histochemistry assay for specific diagnosis of clinical breast cancer tissues. Chin Chem Lett. (2021) 32(5):1726–30. doi: 10.1016/j.cclet.2020.12.009
70. He L, Zhu Y, Wu S, Wang H, Li C. Current signal amplification strategies in aptamer-based electrochemical biosensor: A review. Chin Chem Lett. (2021) 32(5):1593–602. doi: 10.1016/j.cclet.2020.12.013
71. Huang H, Li T, Wang Y, Zhang X, Liu Z. MXene-incorporated C60NPs and Au@Pt with dual-electric signal outputs for accurate detection of Mycobacterium tuberculosis ESAT-6 antigen. Biosens Bioelectron. (2023) 242:115734. doi: 10.1016/j.bios.2023.115734
72. Gopalaswamy R, Shanmugam S, Mondal R, Subbian S. Of tuberculosis and non-tuberculous mycobacterial infections – a comparative analysis of epidemiology, diagnosis and treatment. J Biomed Sci. (2020) 27:74. doi: 10.1186/s12929-020-00678-6
73. Pai M, Riley L, Colford J Jr. Interferon-gamma assays in the immunodiagnosis of tuberculosis: A systematic review. Lancet Infect Dis. (2004) 4(12):761–76. doi: 10.1016/S1473-3099(04)01206-X
74. Shi T, Tang Y, Liu W, Wang H, Wu Z. Nucleic acid and nanomaterial synergistic amplification enables dual targets of ultrasensitive fluorescence quantification to improve the efficacy of clinical tuberculosis diagnosis. ACS Appl Mater Interfaces. (2024) 16(5):14510–9. doi: 10.1021/acsami.3c21856
75. Pathak R, Bhatt S, Punetha V, Punetha M. Chitosan nanoparticles and based composites as a biocompatible vehicle for drug delivery: A review. Int J Biol Macromol. (2023) 253:127369. doi: 10.1016/j.ijbiomac.2023.127369
76. Elgadir M, Uddin M, Islam M, Kabir M, Rahman M. Impact of chitosan composites and chitosan nanoparticle composites on various drug delivery systems: A review. J Food Drug Anal. (2015) 23(4):619–29. doi: 10.1016/j.jfda.2015.05.006
77. Masuelli M, Renard D. Advances in Physicochemical Properties of Biopolymers (Part 2). Sharjah: Bentham Science Publishers (2017).
78. Goy R, Britto D, Assis OBGA. review of the antimicrobial activity of chitosan. Polímeros. (2009) 19(3):241–7. doi: 10.1590/S0104-14282009000300013
79. Rizeq B, Younes N, Rasool K, Nasrallah G. Synthesis, bioapplications, and toxicity evaluation of chitosan-based nanoparticles. Int J Mol Sci. (2019) 20(19):5776. doi: 10.3390/ijms20195776
80. Ahmed T, Aljaeid B. Preparation, characterization, and potential application of chitosan, chitosan derivatives, and chitosan metal nanoparticles in pharmaceutical drug delivery. Drug Des Devel Ther. (2016) 10:483–507. doi: 10.2147/DDDT.S99651
81. Javid A, Ahmadian S, Saboury A, Kalantar S, Rezaei-Zarchi S. Chitosan-coated superparamagnetic iron oxide nanoparticles for doxorubicin delivery: Synthesis and anticancer effect against human ovarian cancer cells. Chem Biol Drug Des. (2013) 82(3):296–306. doi: 10.1111/cbdd.12140
82. Prabhu P, Siva Subramanian T, Velmurugan R, Sivakumar S, Kanimozhi C. Mannose-conjugated chitosan nanoparticles for delivery of Rifampicin to Osteoarticular tuberculosis. Drug Deliv Transl Res. (2021) 11(3):1509–19. doi: 10.1007/s13346-020-00885-3
83. Hoogenboezem E, Duvall C. Harnessing albumin as a carrier for cancer therapies. Adv Drug Deliv Rev. (2018) 130:73–89. doi: 10.1016/j.addr.2018.07.006
84. Tang H, Huang L, Zhao D, Sun C, Song P. Interaction mechanism of flavonoids on bovine serum albumin: Insights from molecular property-binding affinity relationship. Spectrochim Acta A Mol Biomol Spectrosc. (2020) 239:118519. doi: 10.1016/j.saa.2020.118519
85. Solanki R, Rostamabadi H, Patel S, Jafari S. Anticancer nano-delivery systems based on bovine serum albumin nanoparticles: A critical review. Int J Biol Macromol. (2021) 193:528–40. doi: 10.1016/j.ijbiomac.2021.10.027
86. Wang J, Zhang B. Bovine serum albumin as a versatile platform for cancer imaging and therapy. Curr Med Chem. (2018) 25(25):2938–53. doi: 10.2174/0929867324666170314143335
87. Kadota K, Senda A, Tagishi H, Ayorinde J, Tozuka Y. Evaluation of highly branched cyclic dextrin in inhalable particles of combined antibiotics for the pulmonary delivery of anti-tuberculosis drugs. Int J Pharm. (2017) 517:8–18. doi: 10.1016/j.ijpharm.2016.12.058
88. Ma R, Zhang C, Tang X, Duan X, Zhao Y. Treatment of spinal tuberculosis in rabbits using bovine serum albumin nanoparticles loaded with isoniazid and rifampicin. Neurol Res. (2022) 44(3):268–74. doi: 10.1080/01616412.2022.2112178
89. Hoang Thi T, Nguyen D, Nguyen P, Le V, Le Q. Functionalized mesoporous silica nanoparticles and biomedical applications. Mater Sci Eng C Mater Biol Appl. (2019) 99:631–56. doi: 10.1016/j.msec.2019.02.028
90. Nguyen T, Choi Y, Kim J. Mesoporous silica as a versatile platform for cancer immunotherapy. Adv Mater. (2019) 31:e1803953. doi: 10.1002/adma.201803953
91. Escriche-Navarro B, Betancor-Fernández I, Soler M, Martínez-Máñez R, Sancenón F. Mesoporous silica materials as an emerging tool for cancer immunotherapy. Adv Sci. (2022) 9:2200756. doi: 10.1002/advs.202200756
92. Griffin M, Ceballos G, Villarreal F. Tetracycline compounds with non-antimicrobial organ protective properties: Possible mechanisms of action. Pharmacol Res. (2011) 63:102–7. doi: 10.1016/j.phrs.2010.10.010
93. Chu W, Huang Y, Yang C, Liao Y, Zhang X, Yan M, et al. Calcium phosphate nanoparticles functionalized with alendronate-conjugated polyethylene glycol (PEG) for the treatment of bone metastasis. Int J Pharm. (2017) 516:352–63. doi: 10.1016/j.ijpharm.2017.07.058
94. Wang H, Li J, Zhang X, Chen Y, Liu T. Tetracycline-grafted PLGA nanoparticles as bone-targeting drug delivery system. Int J Nanomedicine. (2015) 10:5671–85. doi: 10.2147/IJN.S89619
95. Liang Q, Liu Y, Xu M, Zhang C, Wang J. Development of tetracycline-modified nanoparticles for bone-targeted delivery of anti-tubercular drug. Front Bioeng Biotechnol. (2023) 11:1207520. doi: 10.3389/fbioe.2023.1207520
96. Dymek M, Sikora E. Liposomes as biocompatible and smart delivery systems - the current state. Adv Colloid Interface Sci. (2022) 309:102757. doi: 10.1016/j.cis.2022.102757
97. Allen T, Cullis P. Liposomal drug delivery systems: From concept to clinical applications. Adv Drug Deliv Rev. (2013) 65:36–48. doi: 10.1016/j.addr.2012.09.037
98. Torchilin V. Recent advances with liposomes as pharmaceutical carriers. Nat Rev Drug Discov. (2005) 4:145–60. doi: 10.1038/nrd1632
99. Li J, Zhang Y, Wang Q, Li XA. review on phospholipids and their main applications in drug delivery systems. Asian J Pharm Sci. (2015) 10:81–98. doi: 10.1016/j.ajps.2014.08.002
100. Guimarães D, Cavaco-Paulo A, Nogueira E. Design of liposomes as drug delivery system for therapeutic applications. Int J Pharm. (2021) 601:120571. doi: 10.1016/j.ijpharm.2021.120571
101. Inglut C, Sorrin A, Kuruppu T, Vig S, Cicalo J, Ahmad H, et al. Immunological and toxicological considerations for the design of liposomes. Nanomaterials (Basel). (2020) 10(2):190. doi: 10.3390/nano10020190
102. Liu G, Hou S, Tong P, Li J. Liposomes: Preparation, characteristics, and application strategies in analytical chemistry. Crit Rev Anal Chem. (2022) 52(2):392–412. doi: 10.1080/10408347.2020.1805293
Keywords: bone and joint tuberculosis, nanomaterials, diagnosis, treatment, tuberculosis
Citation: Ding Y, Li B, Yi Y, Liu C, Wen J, Jian X and Li Y (2025) Progress in the role of nanoparticles in the diagnosis and treatment of bone and joint tuberculosis. Front. Med. 12:1536547. doi: 10.3389/fmed.2025.1536547
Received: 29 November 2024; Accepted: 02 January 2025;
Published: 24 January 2025.
Edited by:
Swati Jaiswal, University of Massachusetts Medical School, United StatesReviewed by:
Behnaz Akbari, Purdue University, United StatesCopyright © 2025 Ding, Li, Yi, Liu, Wen, Jian and Li. This is an open-access article distributed under the terms of the Creative Commons Attribution License (CC BY). The use, distribution or reproduction in other forums is permitted, provided the original author(s) and the copyright owner(s) are credited and that the original publication in this journal is cited, in accordance with accepted academic practice. No use, distribution or reproduction is permitted which does not comply with these terms.
*Correspondence: Jie Wen, Q2FzaHdqQHFxLmNvbQ==; Xiaohong Jian, MjczMzQxMDY3QHFxLmNvbQ==
†These authors share first authorship
Disclaimer: All claims expressed in this article are solely those of the authors and do not necessarily represent those of their affiliated organizations, or those of the publisher, the editors and the reviewers. Any product that may be evaluated in this article or claim that may be made by its manufacturer is not guaranteed or endorsed by the publisher.
Research integrity at Frontiers
Learn more about the work of our research integrity team to safeguard the quality of each article we publish.