- 1Centro Medico Teknon, Barcelona, Spain
- 2Centro Medico Bustos Fernandez, Instituto de Gastroenterologia, Buenos Aires, Argentina
- 3Institute of Nutrition and Food Technology, Universidad de Chile, Santiago, Chile
- 4Department of Gastroenterology, University of São Paulo School of Medicine, São Paulo, Brazil
- 5Catedra de Pediatria, Hospital Cayetano Heredia, Universidad Peruana Cayetano Heredia, Lima, Peru
- 6Department of Pediatric Gastroenterology, El Bosque University, Bogotá, Colombia
- 7Pediatric Gastroenterology Service, Federal University of Bahia, Salvador, Brazil
- 8Servicio de Gastroenterología, Hospital Médica Sur, Ciudad de México, Mexico
Antibiotics are safe, effective drugs and continue to save millions of lives and prevent long-term illness worldwide. A large body of epidemiological, interventional and experimental evidence shows that exposure to antibiotics has long-term negative effects on human health. We reviewed the literature data on the links between antibiotic exposure, gut dysbiosis, and chronic disease (notably with regard to the “developmental origins of health and disease” (“DOHaD”) approach). Molecular biology studies show that the systemic administration of antibiotic to infants has a rapid onset but also often a long-lasting impact on the microbial composition of the gut. Along with other environmental factors (e.g., an unhealthy “Western” diet and sedentary behavior), antibiotics induce gut dysbiosis, which can be defined as the disruption of a previously stable, functionally complete microbiota. Gut dysbiosis many harmful long-term effects on health. Associations between early-life exposure to antibiotics have been reported for chronic diseases, including inflammatory bowel disease, celiac disease, some cancers, metabolic diseases (obesity and type 2 diabetes), allergic diseases, autoimmune disorders, atherosclerosis, arthritis, and neurodevelopmental, neurodegenerative and other neurological diseases. In mechanistic terms, gut dysbiosis influences chronic disease through direct effects on mucosal immune and inflammatory pathways, plus a wide array of direct or indirect effects of short-chain fatty acids, the enteric nervous system, peristaltic motility, the production of hormones and neurotransmitters, and the loss of intestinal barrier integrity (notably with leakage of the pro-inflammatory endotoxin lipopolysaccharide into the circulation). To mitigate dysbiosis, the administration of probiotics in patients with chronic disease is often (but not always) associated with positive effects on clinical markers (e.g., disease scores) and biomarkers of inflammation and immune activation. Meta-analyses are complicated by differences in probiotic composition, dose level, and treatment duration, and large, randomized, controlled clinical trials are lacking in many disease areas. In view of the critical importance of deciding whether or not to prescribe antibiotics (especially to children), we suggest that the DOHaD concept can be logically extended to “gastrointestinal origins of health and disease” (“GOHaD”) or even “microbiotic origins of health and disease” (“MOHaD”).
1 Introduction
Worldwide, antibiotics continue to save millions of lives, relieve suffering, and prevent long-term illness (1). Large quantities of these drugs are involved: for example, over 250 million prescriptions of antibiotics were issued in the USA alone in 2016 (2). One can reasonably hypothesize that worldwide, most people (especially children) are treated with an antibiotic at least once a year (3–6). Antibiotics are extremely effective and generally lead to the eradication of the targeted pathogenic bacteria (7). By definition, antibiotics create dysbiosis [defined in several ways (8)] by killing significant components of the gut community (9–11) (Figure 1). Broadly, levels of Enterobacteriaceae, Bacteroidaceae, enterococci, and drug-resistant Escherichia coli rise following antibiotic treatment in adults, whereas levels of bifidobacteria, lactobacteria, actinobacteria and Lachnospiraceae decrease (11–13). Despite the emergence of microbial resistance as a long-term public health risk, antibiotics are still irreplaceable in the treatment of bacterial infections (14).
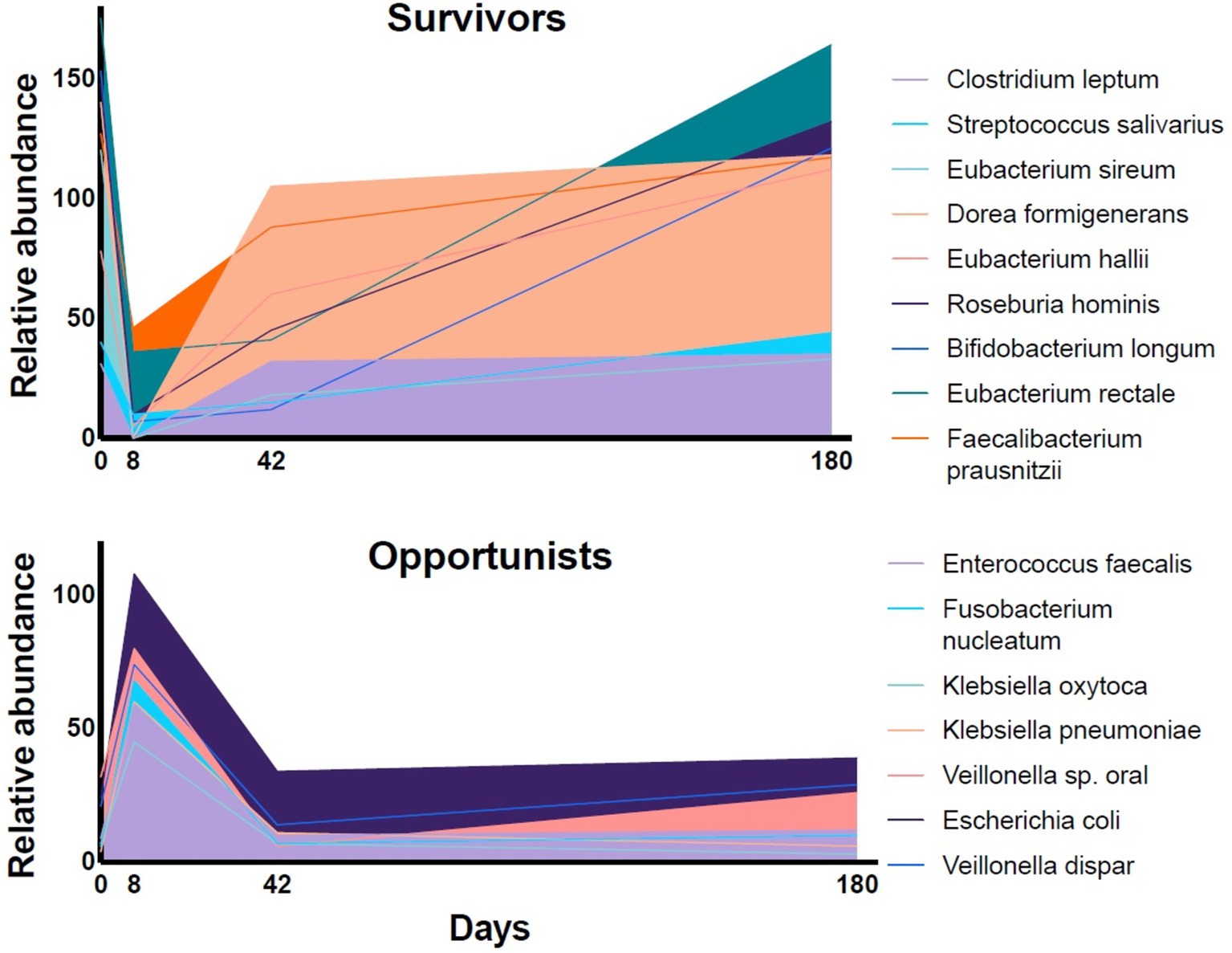
Figure 1. The relative abundance of representative species in fecal samples of volunteers during a 6-month follow-up period after a four-day course of a combination of meropenem, gentamicin, and vancomycin [data extracted from Palleja et al. (11)]. The relative abundance of the dominant fermentative species (“Survivors”) dropped during the antibiotic treatment and recovered slowly over the follow-up period. The relative abundance of subdominant, resistant bacteria (“Opportunists”) increased rapidly.
However, antibiotics also have negative effects on health. The acute (short-term) effects have been extensively studied; for example, between 5 and 20% of antibiotic users (depending on the population studied) will develop antibiotic-associated diarrhea with days or weeks of treatment initiation, and the incidence is greatest in frail, hospitalized patients and young children (11, 15–19). There is now a large body of epidemiological evidence to show that exposure to antibiotics has chronic (long-term) negative effects on human health—effects likely to be accentuated by poor antibiotic stewardship, inappropriate prescribing, excessive or chronic administration, and off-label use.
The present review covers the literature data on the long-term negative health effects of antibiotic exposure, the link to dysbiosis, and how the associated risks might be managed through the evidence-based administration of specific probiotics. Although this review was not systematic, we searched the PubMed database for recent publications (from January 1st, 2023, to June 15th, 2024) using logical combinations of the following keywords: antibio*, exposure, microbiot*, microb*, gut, intestine*, dysbiosis, DOHaD, thousand days, health, disease, and probiotic.
2 The gut microbiota and its maturation
From the mouth to the anus, the adult human gastrointestinal tract has a surface area of around 30 m2 and a luminal volume of around 3 L (20). After the skin, the gastrointestinal tract constitutes the body’s largest interface with the environment. The gut carries 1013–1014 microbes from thousands of species, which contain several million genes in total—far more than in the human genome. Here, we shall use the term “gut microbiota” to refer to the set of microbial (mainly bacterial) species contained in the environment of the human gastrointestinal tract. We prefer “microbiota” to the term “microbiome,” which we take to encompass not only the microorganisms (bacteria, archaea, and lower and higher eukaryotes) and their genomes but also the human cells and substances in the surrounding gut (21). Although there are huge inter- and intra-individual variations in the composition of the gut microbiota, over 99% of the gut microbiota is composed of species from the Firmicutes, Bacteroidetes, Proteobacteria, and Actinobacteria (22).
It is generally accepted that a human’s gut microbiota starts to form after birth (i.e., ex utero) and reaches maturity at around the age of 3 years (23, 24). Interesting, this period coincides largely with the “first 1,000 days” (from conception to the age of 2 years)? The “first 1,000 days” concept grew out of a body of research on the importance of the early life environment for the child’s current and future mental, physical and emotional health states (25–30). Awareness of this concept grew markedly after a keynote speech by the then US Vice President Hilary Clinton at the “1,000 Days: Change a Life, Change the Future” international conference on global child undernutrition in 2010. Furthermore, the “first 1,000 days” concept fits well with the “thrifty phenotype” hypothesis, the Barker hypothesis (linking adverse nutrition childhood to metabolic syndromes in adulthood) and the subsequent “developmental origins of health and disease” (DOHaD) concept; all hold that a poor fetal environment and perturbed early neonatal life prompt the development of disease (31–33). Of course, a focus on the first 1,000 days does not mean that day 1,001 is medically and scientifically unimportant. Nevertheless, the “first 1,000 days” concept has been useful for (i) focusing research efforts and public opinion on this critical period in the development of the child and, indeed, of the microbiota, and (ii) emphasizing the importance of the microbiota’s origin and development in the first hours, days, weeks, months and then years of the human host’s life.
As mentioned above, most experts consider that the human gut microbiota starts to form after birth. However, there is some debate as to the presence of a fetal microbiota, and it is not inconceivable that bacteria from the mother can cross the maternal-fetal barrier (34). However, the ethical and practical difficulties of collecting contamination-free samples of fetal tissue have complicated research efforts to settle this debate (35–39). Delivery by cesarean section (i.e., the avoidance of contact with the vaginal microbiota) is a major dysbiosis-promoting factor and is associated with a low-Bacteroides profile in the first 6 months postpartum (40). In children delivered by cesarean section, relatively high abundances of Burkholderiaceae, Bacteroidales, and Ruminococcaceae persist for at least 7 years (41, 42). It is also noteworthy that the gut microbiota is much less diverse in preterm infants (born after less than 34 weeks of gestation) than in term infants—even in the absence of antibiotic treatment, which most preterm infants nevertheless receive. Cetinbas et al. used 16S rDNA sequencing to evaluate long-term, antibiotic-induced dysbiosis on the basis of 363 stool samples collected between 26 and 48 weeks of adjusted age from 65 preterm infants treated with antibiotics in the NICU and 52 samples from 14 preterm counterparts not treated with antibiotics (43). Antibiotic-treated preterm infants were slower to return to a “normal” gut microbiota, in terms of Shannon diversity and species richness. The difference in the abundance of Paenibacillus amylolyticus was quickest to disappear, whereas changes in Veillonella, Shuttleworthia and Clostridioides persisted up to 40 weeks of age (43).
During the potentially unstable first years of life, the gut microbiota can be perturbed by pathogenic microbes and external (environmental) factors, such as diet, xenobiotics, and other exogenous compounds—notably antibiotics (44). In-depth molecular biology studies have shown very clearly that the systemic administration of an antibiotic to infants has a rapid but often long-lasting impact. For example, Yassour et al.’s study of 1,069 samples of feces from 39 children (around half of whom had been treated with antibiotics in the first year of life) collected over a period of 36 months showed that antibiotic-treated individuals had less stable gut microbiotas, with low diversity still visible at the age of 3 years (40). Kwon et al. used 16S rRNA sequencing and linear discriminant effect size analysis to evaluate alpha and beta diversities in fecal samples from (i) 20 infants under 3 months of age who had received antibiotics for at least 3 days and (ii) 34 age-matched, healthy controls not exposed to antibiotics (45). Relative to controls, the relative abundances of Escherichia, Shigella and Bifidobacterium were significantly greater in the antibiotic-treated group, whereas that of Bacteroides was significantly lower. The abundances of Firmicutes genera (Allobaculum, Enterococcus, and Candidatus arthromitus), Proteobacteria genera (Klebsiella), and Actinobacteria genera (Bifidobacterium) were three or four times higher in the treated group than in the control group. A phylogenetic investigation of communities by reconstruction of unobserved states revealed a significant difference in gut microbiome metabolic activity between the two groups; the antibiotic-treated group showed the expression of significantly more genes involved in naphthalene degradation, glycolysis gluconeogenesis, and lipoic acid metabolism and less expression of genes involved in porphyrin metabolism and fatty acid biosynthesis (45).
The effects of antibiotics on the gut microbiota of newborns and infants are accentuated by a lack of standardization in antibiotic treatment plans. For example, Schulman et al.’s study of 127 neonatal intensive care units (NICUs) in the USA evidenced up to 40-fold variations in dosing regimens (46). In France, Leroux et al. study of 44 NICUs found an average of nine different dosing regimens for each of the 41 antibiotics documented (47). Similarly, in 43 NICUs in the UK, Kadambari et al. identified 10 different dosing regimens for prescriptions of gentamicin (48).
3 “Normal,” “healthy” microbiotas: how can dysbiosis be defined?
Although many experts have sought to define dysbiosis, the topic is subject to debate and constitutes a field of research in its own right (49). As emphasized by Brüssow and by Drago et al., no consensus conference has yet worked out a definition of “dysbiosis” (50, 51). To the best of our knowledge, none of the learned societies or medical associations in this field have issued a single, unambiguous definition of “dysbiosis.” Given this lack, there is no “gold standard” approach to identifying a dysbiotic state (52). A conventional, broad definition published by Petersen and Round in 2014 is related primarily to the composition of the microbiota: “any change to the composition of resident commensal communities relative to the community found in healthy individuals” (13). These compositional changes usually encompass a decrease in bacterial diversity, the loss of beneficial microbes, and overgrowth by potential pathogens. More recent definitions have emphasized function as well as composition: for example, Levy et al. define dysbiosis as “a compositional and functional alteration in the microbiota that is driven by a set of environmental and host-related factors that perturb the microbial ecosystem to an extent that exceeds its resistance and resilience capabilities” (53). In this definition, gut microbiota can be viewed as a conceptual energy landscape in which both healthy and dysbiotic states can exist in energy minima. External factors (such as antibiotics, various xenobiotics, dietary components, and pathogens) that exceed a certain threshold cause transitions from a stable, healthy state to a metastable, dysbiotic state (54). Levy et al. acknowledged that given the high degree of interindividual variability, it is impossible to define a single “healthy” microbiota; however, they suggest that the main characteristic of a “healthy” microbiota in a given individual is richness and stability over time (53). Wilkins et al. have suggested that dysbiosis should be defined by reference to a particular disease state, i.e., a single, all-encompassing definition may not be feasible (49). Lastly, Malard et al. have defined dysbiosis as the disruption of host-microbe symbiosis and crosstalk, with auto-aggravating signals from both the host and microbes that maintain the metastable dysbiotic state (54). Hence, as detailed in the following section, dysbiosis of the gut microbiota can be viewed as the disruption of the positive actions of (i) short-chain fatty acids (SCFAs, produced by the fermentation of host-enzyme-resistant carbohydrates by obligate anaerobes) (55, 56), (ii) the microbial production of serotonin, catecholamines and other neurotransmitters (affecting gut peristaltic motility and the genesis of the enteric nervous system) (57–61), (iii) minimization of the “leaky gut” in which potentially pathogenic whole bacteria and bacterial wall components can enter the circulation (62–64), and (iv) the bacterial expression of enzymes that influence the levels of host metabolites (65, 66). In summary, dysbiosis does not have a consensus definition but can usefully be viewed as the disruption of a previously stable, symbiotic, functionally complete microbiota by dietary, xenobiotic or other factors.
4 Mechanistic links between the microbiota, dysbiosis, health, and chronic disease
It is now clear that a stable, functionally rich gut microbiota exerts complex direct and indirect effects on the human host’s health through multiple genetic, immune-mediated and metabolic factors. Conversely, dysbiosis of the gut microbiota (i.e., the disruption of a previously stable, functionally complete microbiota) has many harmful short- and long-term effects on health. Common signs of gut dysbiosis include diarrhea, gas, constipation, nausea, and even chest pain (67). Admittedly, in most settings, it is difficult to determine whether dysbiosis is the cause or result of disease. Determining the causal nature and direction of a relationship notably requires prospective, longitudinal studies to see whether the dysbiosis or the disease occurs first. However, this approach can be confounded if a patient remains free of symptoms for months or years. A causal role of antibiotic-associated gut dysbiosis can nevertheless be suspected when the disease is more intense after exposure to broad-spectrum antibiotics (compared with narrow-spectrum antibiotics) and shows an antibiotic dose dependence (68–70). Lastly, one cannot rule out direct, negative effects (i.e., not mediated by the gut microbiota) of antibiotics. Some classes of antibiotic affect myocytes and neurons directly; for example, aminoglycosides, capreomycin, and macrolides are ototoxic (71, 72). Below, we describe the main pathways through which the gut microbiota are known to influence human health.
4.1 Short-chain fatty acids
The SCFAs [acetic (C2), propionic (C3), butyric (C4), and valeric (C5) acids] are produced via the fermentation of host-enzyme-resistant carbohydrates by obligate anaerobes (primarily members of the Bacteroidetes and Firmicutes, including Roseburia spp., Prevotella spp., Ruminococus spp., Coprococcus sp., Akkermansia muciniphila, Faecalibacterium prausnitzii, and Eubacterium rectale), in collaboration with bifidobacteria (73). SCFAs are produced at up to millimolar concentrations in the gut and have several distinct metabolic and regulatory effects on the host.
Firstly, some of the SCFAs’ extracellular actions are exerted through via the G-protein-coupled free fatty acid receptors 2 and 3 (FFA2 and FFA3), which are involved in responses to immune challenges (74, 75). Secondly, acetate and propionate bind to the G-protein-coupled olfactory receptor 51E2 (found notably in intestinal and enteroendocrine tissues) and the aryl hydrocarbon receptor (76–79). Thirdly, butyrate is a competitive inhibitor of histone deacetylase, which leads to the hyperacetylation of histones, increases chromatin accessibility and has major epigenetic effects on gene transcription (55, 56). Importantly, butyrate’s inhibition of histone deacetylase promotes histone acetylation in the Foxp3 gene’s promoter and enhancer regions in naïve T-cells in the colon and promotes their differentiation into peripheral regulatory T cells (key players in the immune tolerance of antigens during development) (80, 81). Hence, a low level of butyrate in the intestinal tract (due to antibiotic-induced dysbiosis) impairs Treg differentiation and the immune system’s ability to suppress excessive immune responses, which in turn may lead to mucosal and systemic inflammatory states. Lastly, the low oxygen levels required by the colon-dwelling strict anaerobes associated with a health microbiota are maintained by the β-oxidation of bacterially produced butyrate. Indeed, butyrate is the healthy colonocyte’s main energy substrate, accounting for 70–80% of its energy needs (82). A lack of SCFA-producing bacteria will tend to increase oxygen levels in the gut lumen and thus favor the expansion of aerobes and facultative anaerobes with pro-inflammatory potential.
4.2 The enteric nervous system and gastrointestinal dysmotility
The enteric nervous system (ENS) is now acknowledged to be a complex network of neurons and enteric glial cells that controls gastrointestinal motility, blood flow, and immune responses. The gut microbiota’s direct or indirect influences on the ENS are signaled to the brain through vagal afferents (83). Conversely, the brain’s responses to stress may be passed to the ENS by vagal efferents (83). The gut microbiota can affect the genesis of the ENS and gut peristaltic motility by producing serotonin (57, 58). Studies in mice have shown that the administration of antibiotics modified the gut microbiome (with marked decreases in the abundance of Clostridioides, Lachnoclostridium, and Akkermansia), induced intestinal dysmotility, and interfered with the differentiation of myenteric neurons and the expression of the neuronal marker beta III-tubulin in the myenteric plexus (84, 85). These and other observations indicate that antibiotic-induced gut dysbiosis is associated with structural and functional damage to the ENS, including gastrointestinal dysmotility.
4.3 Catecholamines and neurotransmitters
Some gut microbial strains produce and/or respond to compounds that serve as hormones, neurotransmitters or their precursors in the human host, including catecholamines, gamma aminobutyric acid (produced by lactobacilli and bifidobacteria), acetylcholine (lactobacilli), dopamine (Bacillus sp.), neuropeptides, noradrenaline (Bacillus sp.), serotonin (Escherichia, Enterococcus, and Streptococcus), endocannabinoids, histamine, and tryptophan (bifidobacteria) (57, 59–61). The gut microbiota can thus be said to have a direct action on the nervous system.
4.4 Intestinal barrier integrity, immune signaling, and inflammation
The gut microbiota helps to protect the intestinal mucosa—notably through the presence of mucus-promoting commensals and (in the colon) the supply of butyrate as an energy substrate to colonocytes (86). Dysbiosis can trigger the loss of intestinal barrier integrity. The resulting passage of pathogenic and non-pathogenic microbes leads to antigen presentation and the activation of innate and adaptive immune cells. One of the key pro-inflammatory signals in the loss of intestinal barrier integrity is the endotoxin lipopolysaccharide from the cell wall of Gram-negative bacteria (87). Lipopolysaccharide induces inflammation by activating Toll-like receptor 4 and upregulating nuclear factor-kappa B (88).
4.5 Dietary induction of enzymes
The composition of the diet influences the expression of a wide range of bacterial enzymes (cholesterol dehydrogenase, beta glucosidase and glucuronidase, 7-alpha-hydroxylase, nitroreductase, and azoreductase), notably by bacteria in the colon (65, 66). In turn, the activity of these enzymes influences levels of key host metabolites.
5 Chronic disease, environmental factors, antibiotics, and dysbiosis
Many chronic diseases are known to have both genetic factors and environmental susceptibility/triggering factors, of which antibiotic exposure is only one. Many of these environmental factors are linked to a “modern,” “post-industrial” or “Western” lifestyle, including the “Western diet” (high in sugar, fat, and processed food components, and low in fiber) and sedentary behavior (typically defined as spending time in a sitting, reclining or lying posture with an energy expenditure of 1.5 metabolic equivalents of task or less) (89–91). Sedentary behavior (including total sitting time and TV viewing time) is associated with an elevated risk of chronic diseases, including diabetes, cardiovascular disease, and (to a lesser extent) cancer—even after adjustment for the level of physical activity (92). Interestingly, lower sedentary behavior through increased physical activity is known to influence gut microbiota diversity in adults and children, with higher alpha-diversity (notably including SCFA-producing Lachnospiraceae and Erysipelotrichaceae families and Akkermansia, Roseburia, and Veillonella, genera) in physically active individuals (93–96). Indeed, SCFAs appear to be the main molecular link between physical activity and the gut microbiome, although few published studies of physically active vs. sedentary populations controlled for dietary confounders (i.e., fiber intake) (97). The high-sugar, high-fat “Western diet” is clearly associated with dysbiosis, as characterized by a decrease in the abundance of Bacteroidetes and bifidobacteria and an increase in organisms that can utilize excess monosaccharides, such as Enterobacteriaceae and Proteobacteria (98–103). Again, the main molecular link between diet, a normal microbiota and the associated health effects on the host is likely to be the SCFAs (104).
Antibiotic-induced perturbation of the gut microbiome nevertheless constitutes a key “environmental” factor in the development of chronic disease (Figure 2). The prevalent use of antibiotics in infants reveals concerning associations between antibiotic exposure and the onset of a number of distinct immunological, metabolic and neurobehavioral health conditions (whether isolated or combined) during childhood. For example, in a time-to-event analysis of medical records for 14,572 children born in Olmsted County (MI, United States) between January 1, 2003, and December 31, 2011, Aversa et al. found that antibiotic exposure in the first 2 years of life (i.e., during the first 1,000 days) was associated with asthma, allergic, rhinitis, atopic dermatitis, celiac disease, overweight, obesity, attention deficit hyperactivity disorder, and learning disability (105). These health risks were influenced by the number, type, and timing of the antibiotic prescriptions. Relationships between antibiotics, dysbiosis, and a number of chronic diseases are described below.
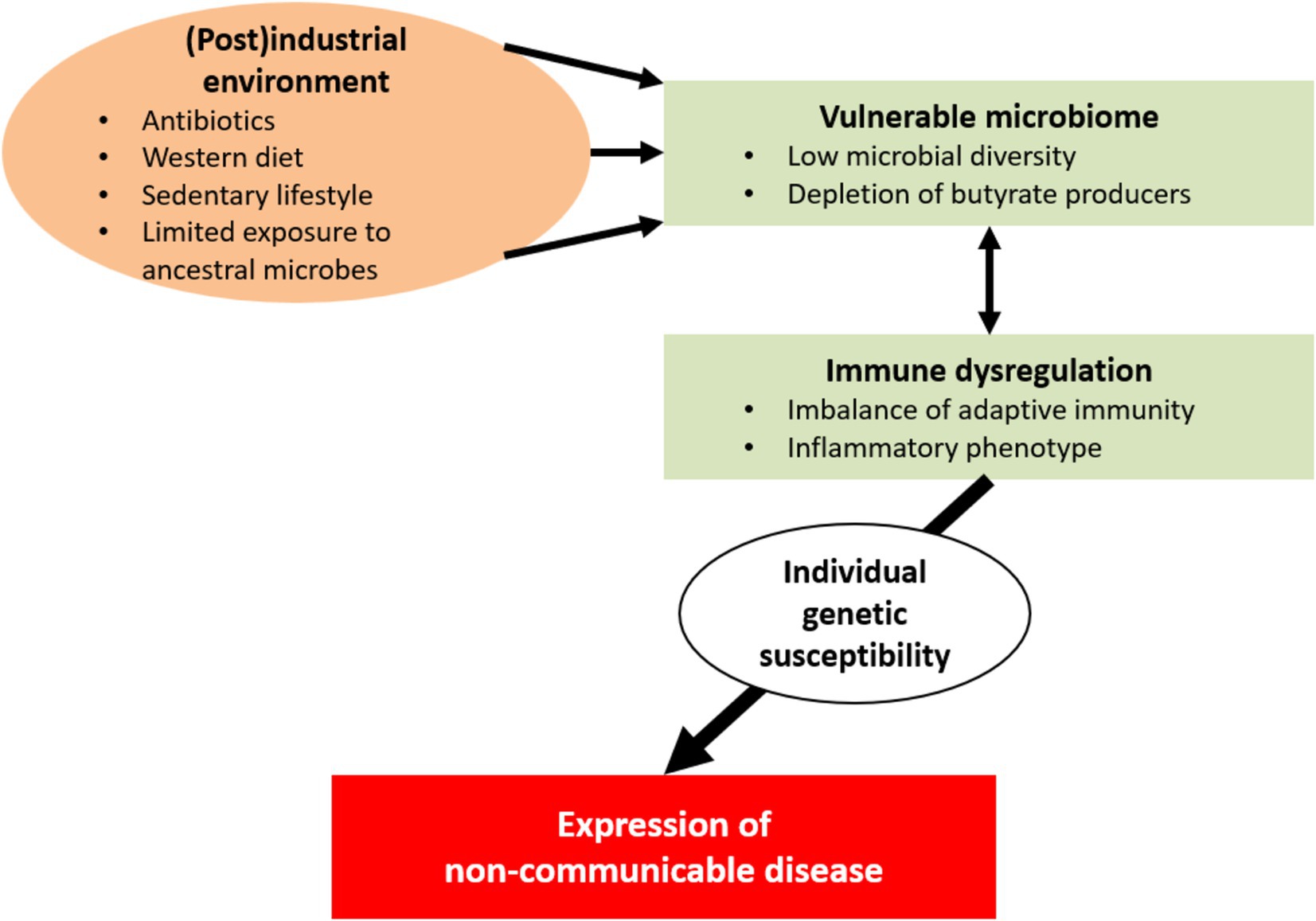
Figure 2. In industrial and postindustrial societies, a number of environmental factors have detrimental consequences for a vulnerable human microbial ecosystem; these factors include exposure to antibiotics and xenobiotics, sanitation of the living space, Western-type diets, sedentariness, and pollution. This unwanted alterations to the gut microbiome appear to be suboptimal for human health. Interactions between the altered microbiome and the host’s immune system contribute to the development of immune dysregulation and inflammatory phenotypes. In turn, these disturbances may lead to chronic inflammation and tissue injury. Individual genetic susceptibility might eventually determine the clinical expression of non-communicable diseases (e.g., inflammatory, autoimmune, metabolic and neoplastic conditions and cognitive disorders).
5.1 Inflammatory bowel disease
Inflammatory bowel disease (IBD) primarily encompasses Crohn’s disease (CD) and ulcerative colitis (UC). Fecal samples from people with IBD (especially CD) are characterized by a low abundance of Firmicutes and a high abundance of Proteobacteria (106). Interestingly, people with IBD also have an abnormally high abundance of the mucin-degrading bacteria Ruminococcus gnavus and Cenarchaeum symbiosum, even though these species are present in the healthy gut (106).
A large body of evidence suggests that gut dysbiosis [alone or in combination with other factors, such as diet, smoking, pollution, xenobiotics, and genetic factors causing a leaky gut (i.e., increased permeability)] may cause gut inflammation and thus IBD (107). The gut mycobiome and gut virome have been implicated in this dysbiosis, along with the bacterial communities of the gut microbiota (107–109). It has been suggested that dysbiosis early in life leads to CD, whereas dysbiosis at any time in life contributes to the onset of UC (110).
Ungaro et al. meta-analysis of 11 observational studies (including 7,208 children and adults diagnosed with IBD) found that exposure to antibiotics increased the risk of being newly diagnosed with CD (odds ratio (OR) [95% confidence interval (CI)]: 1.74 [1.35–2.23]) but not ulcerative colitis (1.08 [0.91–1.27]) (111). However, the data are contradictory: Troelson and Jick’s case–control study of 461 patients with UC and 683 patients with CD did not find any association with prior antibiotic use (112). A nation-wide observational study in Denmark confirmed the association between antibiotic exposure and elevated, independent risks of CD and UC. A positive dose–response relationship was also observed: the higher the level of exposure, the greater the risk (68).
It has been hypothesized that the effects of dysbiosis in IBD are mediated through SCFAs, the mechanistic roles of which have been outlined above. Holota et al. found that a 14-day course of ceftriaxone treatment in male Wistar rats led to a greater caecum weight, a fall in SCFA levels, the sustained elevation of conditionally pathogenic enterobacteria such as E. coli, Clostridioides, Staphylococcus spp. and hemolytic bacteria, increased colonic epithelial permeability, greater bacterial translocation, and lower levels of FFA2 and FFA3 receptors and SMCT1 and higher levels of MCT1 and MCT4 SCFA transporters in the colonic mucosa. Importantly, the ceftriaxone-treated animals were more susceptible to experimental colitis (113).
5.2 Irritable bowel syndrome
Although irritable bowel syndrome (IBS) does not feature gut inflammation and tissue damage, there is evidence of (i) dysbiosis in people with this functional gastrointestinal disorder (FGID) and (ii) an association between antibiotic use and the development of FGIDs. Saffouri et al. reported that the microbial composition of the small intestinal was significantly altered in symptomatic patients with IBS, with lower phylogenetic alpha diversity, richness, and evenness, and significant decreases in the abundances of Porphyromonas, Prevotella, and Fusobacterium (114). Jones et al. retrospectively studied electronic medical records from over 15,000 patients seen in general practice in the UK. Antibiotics were prescribed more frequently to patients with one or more FGIDs than to healthy individuals. A significant minority (7–14%) of individuals with an FGID received their first recorded antibiotic in the 12 months prior to the FGID diagnosis (115).
5.3 Celiac disease
People with active celiac disease have dysbiosis, with greater abundances of Enterobacteriaceae, Proteobacteria, Staphylococcaceae, and Proteobacteria (70). Furthermore, a reduced abundance of Streptococcus mutans and Streptococcus anginosus was observed in patients with active celiac disease patients and also in those with nonactive disease. Sander et al.’s analysis of data from a register-based cohort study conducted in Denmark from 1995 to 2012 and in Norway from 2004 to 2012 showed that exposure to systemic antibiotics in the first year of life was associated in a dose-dependent manner with the diagnosis of celiac disease (OR [95%CI]: 1.26 [1.16–1.36]) (116), although further research on this topic is required. People with celiac disease should consume a gluten-free diet throughout their life: however, the latter is associated with a microbiota characterized by low abundances of Bifidobacterium sp. and Lactobacillus sp., and higher abundances of pathobionts like E. coli and the Enterobacteriaceae (117).
5.4 Neurological, neurodevelopmental, and neurodegenerative diseases
The increase in the prevalence of multifactorial neurodevelopmental diseases (NDDs, including autism spectrum disorder and attention deficit-hyperactivity disorder) over the last few decades suggest that the prevalence of environmental triggering and/or susceptibility factors (such as antibiotic exposure) during prenatal, perinatal, and postnatal time windows has also increased. Dysbiosis is a known feature of NDDs; individuals with these conditions, notably have a higher fecal abundance of Bacterioidetes and Megamonas, and a lower abundance of bifidobacteria, Veillonella, Escherichia, Ruminococcaceae, Streptococcaeceae, Peptostreptococcaceae, and Erysipelotrichaceae (118–120).
Population-based studies have highlighted a clear association between antibiotic exposure and the risk of neurodegenerative diseases. For example, Kim et al. conducted a retrospective study of claims data in a Korean nationally representative cohort (n = 313,161 participants) (69). After adjustments for covariates, used of antibiotics for 91 or more days over the period from 2002 to 2005 had an elevated risk of dementia in general (adjusted hazard ratio [95%CI] = 1.44 [1.19–1.74]), Alzheimer’s disease (AD: 1.46 [1.17–1.81]) and vascular dementia (1.38 [0.83–2.30]) during the follow-up period from 2006 to 2013. The researchers noted a dose dependency; people having received five or more classes of antibiotic during the study period had higher risks of dementia and AD (but not vascular dementia) (69). A large body of epidemiological research has linked the use of antibiotics during pregnancy (for the treatment of maternal infections) in particular with an elevated risk of NDDs and of cognitive disorders in adulthood (121, 122). The risk appears to be lowest with narrow-spectrum antibiotics (123, 124).
5.5 Autoimmune diseases
In people with multiple sclerosis (MS), the microbiota is characterized by elevated abundances of Firmicutes, Lachnospiraceae, Bifidobacterium, Roseburia, Coprococcus, Butyricicoccus, Lachnospira, Dorea, Faecalibacterium, and Prevotella (most of which produce SCFAs) and elevated abundances of Bacteroidetes, Akkermansia, Blautia, and Ruminocococcus (125). Jangri et al. used 16S rRNA sequencing and gene expression analysis to study the microbiome in 60 people with MS and 43 healthy controls. The MS group presented elevated abundances of Methanobrevibacter and Akkermansia and a lower abundance of Butyricimonas.
Juvenile idiopathic arthritis (JIA) is the most common rheumatic disease in children. Although the multifactorial (genetic and environmental) etiology of JIA is poorly understood, antibiotic exposure in early life has been linked to the onset of JIA (126–128). For example, Hestetun et al. studied 535,294 children born in Norway from 2004 to 2012 (129). Of these, 149,534 (27.9%) were exposed to systemic antibiotics prenatally and 236,340 (44.2%) were exposed during the first 24 months postpartum. The onset of JIA was associated with postpartum antibiotic exposure (adjusted OR [95%CI] = 1.40 [1.24–1.59]) but not prenatal antibiotic exposure. Interestingly, the association was stronger in children having received sulfonamides, trimethoprim, and broad-spectrum antibiotics (129). However, reverse causality cannot be ruled out because inflammatory joint symptoms (especially in children) may be misinterpreted as resulting from a bacterial infection.
5.6 Obesity
Researchers have evidenced complex interactions between the diet, the gut microbiota, inflammation, and obesity. Mechanistically, the pathways involve the microbial production of energy substrates, inflammatory effects on metabolism, and even an impact on satiety through the gut-brain axis (130). There appears to be a signature microbiotic profile for obesity: obese individuals have greater abundances of E. coli, Lactobacillaceae, Escherichia, shigella, and Negativicutes (131, 132). A large number of population-based studies have linked antibiotic administration to mothers during pregnancy and/or to infants in the first months of life to an elevated risk of being overweight later in childhood (133–135).
5.7 Allergy
Impaired or delayed maturation of the microbiota (with degraded mucus, elevated intestinal permeability, and a low proportion of SCFA-producing bacteria) during the first year postpartum may be a feature of allergic disease (136, 137). Ahmadizar et al. meta-analysis of 22 studies highlighted an association between antibiotic exposure in the first 2 years of life and the subsequent diagnosis of eczema (OR: 1.26) and allergic rhinitis (OR: 1.23) (138). However, the results of the analyzed studies were somewhat contradictory and antibiotic exposure was not linked to objective measures of atopy, such as the serum specific IgE level or prick tests positivity or weald size.
Lu et al.’s analysis of asthma trajectories in the Longitudinal Study of Australian Children found that any antibiotic exposure in the first 2 years of life increased the risk [95%CI] of early-persistent asthma by a factor of 2.3 [1.47–3.67] (p < 0.001) (139). In an incidence density study nested within a data collection project, Bentouhami et al. assessed 1,128 mother–child pairs in Belgium (140). Excessive systemic antibiotic use in the first year of life (defined by the researchers as more than four courses) had more than twice the incidence density ratio [95%CI] of asthma (2.18 [0.98, 4.87], p = 0.06), relative to all other children.
However, the relationships with allergies appear to be complex, and some studies have evidenced positive associations with antibiotic exposure. For example, Schoch et al. retrospectively studied 4,106 infants in Florida from 2011 to 2017, roughly half of whom had received antibiotics during the study period (141). Antibiotic exposure (as noted in electronic health records) during the first year of life (and especially during the first month of life) was associated with a lower risk of atopic dermatitis. The researchers suggested that there may be a “critical window” for immune tolerance in human infants, which is influenced by antibiotic exposure (141).
5.8 Kawasaki disease
Dysbiosis has been reported as a susceptibility factor in Kawasaki disease (KD) (142–144). Teramato used 16S rRNA gene analysis to characterize the fecal microbiota of 26 children with KD and 57 age-matched healthy controls (median age, 36.0 months). The KD group had a higher relative abundance of pro-inflammatory Ruminococcus gnavus and lower relative abundance of butyrate-producing Blautia spp. (144). Antibiotic exposure might be a factor in the physiopathology of KD. Kim et al. studied 17,818 children aged under 5 diagnosed with KD between 2016 and 2019, together with 89,090 matched controls. Use of antibiotics in the previous 6 or 12 months was associated with the development of KD (OR [95%CI]: 1.18 [1.12–1.26] and 1.23 [1.14–1.32], respectively). The researchers suggested that antibiotic-related changes in the gut microbiota might have a role in the development of KD (145). Kim et al. findings were in line with those of a previous study in Japan (146). However, Burns has pointed out that the establishment of a causal dysbiotic relationship between antibiotic exposure and KD would require adequately powered studies with appropriate matching criteria and a comparison of fecal samples from patients with KD vs. samples from patients without KD but similar levels of inflammation (147).
5.9 Atherosclerosis
In a study of the atherosclerosis-prone apolipoprotein E-knockout mouse model, Kappel et al. used 16S ribosomal RNA serum metabolomics to evidence an antibiotic-induced fall in the abundance of certain Bacteroidetes and Clostridia. Antibiotic administration was associated with a greater atherosclerotic lesion size, independently of diet. The results of a serum metabolome analysis was suggestive of disturbances in tryptophan, trimethylamine-N-oxide and lipid metabolism by the gut microbiota (148).
5.10 Cancer
A moderate body of evidence suggests that excessive or prolonged antibiotic use is associated not only with a slightly greater risk of cancer onset but also a relative reduction in the effectiveness of cancer treatments (encompassing chemotherapy, radiotherapy, and immunotherapy) (149–151). The strength of the association between antibiotic exposure and cancer onset varies from one type of cancer to another and from one class of antibiotics to another (152). Relationships have been shown for breast cancer, endocrine gland cancers, pancreatic cancer and (to a lesser extent) lung cancer, esophageal cancer, gastric cancer, and ovarian cancer (150–155). Unsurprisingly (in view of the extreme proximity to the gut and the impact on public health), colorectal cancer has been extensively investigated with regard to antibiotic exposure. Most investigators have found a significant, dose-dependent association with colon cancer but not with rectal cancer (156, 157). The elevated risk of colon cancer might be related to low SCFA levels.
6 Mitigation of the potential long-term negative health effects of antibiotic exposure
The quotation “all disease begins in the gut” is often attributed to Hippocrates circa 400 BC. The father of modern medicine was probably not fully correct but, as seen for the diseases reviewed above, the DOHaD concept can be logically extended to what we term the “gastrointestinal origins of health and disease” (“GOHaD”) or even the “microbiotic origins of health and disease” (“MOHaD”). It should nevertheless be borne in mind that the ORs for the associations between antibiotic exposure and the onset of chronic disease are generally quite low (i.e., between 1 and 1.5) and, despite the investigators’ best efforts in study design and data analysis, may be influenced by confounding factors.
More generally, we found that most of the clinical data on the chronic effects of antibiotics were generated in Europe and in North America. Further research in low- and middle-income countries is warranted because the latter are especially burdened by antibiotic resistance problems, vulnerability to infections by antibiotic-resistant pathogens, and the corresponding effects on the gut microbiota (158).
Probiotics have long been viewed as a means of treating the acute gastrointestinal disorders associated with antibiotic-associated gut dysbiosis (8, 159–161). Can probiotics be recommended as adjunct treatments to mitigate the negative effects of antibiotics? The European Society for Paediatric Gastroenterology Hepatology and Nutrition (ESPGHAN) recommends strain-specific probiotics for the prevention of antibiotic-associated diarrhea in children, whereas the American Gastroenterological Association (AGA) guidelines indicate that strain-specific probiotics may be used to prevent Clostridiodes difficile infections (162, 163). For adults and children with IBDs like CD or UC, the AGA guidelines only recommend the use of probiotics only in the context of a clinical trial (162). Similarly, the guidelines issued by the European Crohn’s and Colitis Organisation and the ESPGHAN state that in patients with CD, probiotics should not be used to induce or maintain remission (163–165). According to the guidelines issued by the World Gastroenterology Organisation, there is evidence of strain-specific efficacy of probiotics in the prevention of antibiotic-associated diarrhea in adults or children who are receiving antibiotic therapy (166).
Hence, in view of the links between gut dysbiosis and the chronic diseases described above, one can reasonably hypothesize that the administration of probiotics will provide a degree of disease modification or symptom prophylaxis and mitigate the long-term consequences of antibiotic exposure. The main species to have been tested are lactic acid bacteria (such as Lacticaseibacillus rhamnosus GG, Limosilactobacillus reuteri, Lacticaseibacillus paracasei, Lactiplantibacillus plantarum, Lactobacillus acidophilus, Lactobacillus helveticus, Bifidobacterium lactis, Bifidobacterium breve, and Streptococcus thermophilus) and the yeast Saccharomyces boulardii. The positive reported short-term effects of probiotics in various patient populations (IBD, celiac disease, cancer, obesity, types 1 and 2 diabetes mellitus, allergic disease, idiopathic nephrotic syndrome, KD; multiple sclerosis, rheumatoid arthritis, and systemic lupus erythematosus, atherosclerosis, and neurological, neurodevelopmental and neurodegenerative diseases) will not be described in detail here because (i) the topic falls outside the scope of this review and (ii) the results have been extensively reviewed elsewhere in the literature (103, 159–161, 163–165, 167–210). Certain studies showed a positive effect of certain probiotic strains in combination with antibiotics and have provided a rationale for using probiotics to protect the gut microbiota and intestinal barrier functions.
Probiotics have been tested in animal models of chronic disease and in observational or interventional clinical studies, with moderate, variable but generally positive results: evidenced significant differences or improvements in clinical disease scores, symptom scores, and disease marker levels, whereas other studies found no benefit. In the field of cognitive and psychiatric disorders and mental health problems, the term “psychobiotic” has been used to describe probiotics that act through the gut-brain axis. Following on from extensive preclinical data on an association between antibiotic-induced gut dysbiosis and psychopathologies in the rat (211), there is preliminary evidence to suggest that specific probiotics may improve cognitive function, particularly in people with age-related mild cognitive impairment (212, 213).
In the literature on probiotics, the most common design is the randomized, controlled trial of the efficacy and safety of a probiotic in patients. The second most common design is the pharmacokinetic study, which documents the recovery and/or clearance of an oral dose of probiotic or measures pre−/post differences in the abundances of probiotic strains. According to McFarland, three models of dysbiosis have been frequently evaluated (214). In model A (restoration), probiotic therapy is initiated and studied after the microbiota of initially healthy patients has become disrupted (e.g., by antibiotic exposure). In model B (alteration), patients with a pre-existing disruption of the microbiota are studied after probiotic therapy. In model C (no dysbiosis), volunteers with no disruptive events are studied before and after probiotic therapy. In McFarland’s systematic review of 63 trials (published in 2014), 83% of the probiotic products evaluated in model A restored the microbiota. The corresponding proportion was 56% in model B. Only 21% of the probiotics evaluated in model C had an effect on the microbiota. Clinical efficacy was more commonly observed for with strains capable of restoring the normal microbiota (214).
Furthermore, meta-analyses of trials in better-studied disease areas have sometimes failed to show a clear, beneficial effect of probiotics. However, the meta-analyses’ authors almost always highlight the degree of interstudy heterogeneity with regard to probiotic doses, strains, and treatment durations. Larger, multicenter, randomized, controlled trial of probiotics (possibly simultaneously investigating the gut microbiota and disease markers) are warranted.
The gut virome and gut mycobiome have attracted less attention than the gut’s bacterial communities but, as mentioned above, are known to be abnormal in people with IBD (107–109, 215). We recommend further investigation of the potential indirect effects of antibiotic treatment and probiotics on the gut virome and mycobiome. Specific strains of yeast probiotics are a topic of interest; as mentioned above, one of the most effective and frequently evaluated probiotics is a yeast (Saccharomyces boulardii CNCM I-745) and therefore is not directly affected by antibiotics (8, 216–218). Saccharomyces boulardii is not a natural member of the human gut microbiota and is eliminated rapidly after probiotic administration is discontinued. However, when present as a probiotic, certain strains of S. boulardii exert several beneficial actions (including protection of the mucus layer, the stimulation of SCFA production by Lachnospiraceae and Ruminococcaceae, and a reduction in local inflammation) that counter antibiotic-associated dysbiosis (8, 216–218). In terms of the composition of the microbiota, treatment with S. boulardii is associated with increased abundances of Bacteroidaceae and Prevotellaceae and the suppression of pioneer bacteria (218). Treatment with S. boulardii CNCM I-745 can mitigate antibiotic-associated dysbiosis and diarrhea (219). More studies are needed to explore the full potential of this versatile probiotic yeast (218).
7 Conclusion
The results of our review indicate that antibiotic exposure is associated with a number of negative long-term (i.e., chronic) effects on health. Gut dysbiosis might be the causal link between antibiotic exposure and these chronic negative effects, although the lack of a replicable consensus definition of dysbiosis can lead to ambiguity in the interpretation of the data. Given that certain well-studied probiotics (such as S. boulardii CNCM I-745 and L. rhamnosus GG) are inexpensive, safe and effective in preventing short-term negative consequences of antibiotic exposure (including dysbiosis), there is no reason to summarily rule out potential longer-term benefits in a particular chronic disease setting or patient population. We recommend that decisions to initiate probiotic treatment should be made on a case-by-case basis after informed, evidenced-based discussion between the patient and his/her physician.
Author contributions
FG: Conceptualization, Supervision, Writing – original draft, Writing – review & editing. LB: Writing – original draft, Writing – review & editing. SC: Writing – original draft, Writing – review & editing. AD: Writing – original draft, Writing – review & editing. AM: Writing – original draft, Writing – review & editing. JR: Writing – original draft, Writing – review & editing. LR: Writing – original draft, Writing – review & editing. MV: Writing – original draft, Writing – review & editing.
Funding
The author(s) declare that financial support was received for the research, authorship, and/or publication of this article. This work was funded by an educational grant from Biocodex SAS (Gentilly, France). The grant also covers the journal’s open access fee. Editorial assistance in the preparation of this article was provided by David Fraser (Biotech Communication SARL, Ploudalmézeau, France) and funded by Biocodex SAS.
Conflict of interest
FG received research grants from Abbvie, Takeda, and AB-Biotics and was a member of the Biocodex Microbiota Institute’s scientific advisory board.
The remaining authors declare that the study was conducted in the absence of any commercial or financial relationships that could be construed as a potential conflict of interest.
Publisher’s note
All claims expressed in this article are solely those of the authors and do not necessarily represent those of their affiliated organizations, or those of the publisher, the editors and the reviewers. Any product that may be evaluated in this article, or claim that may be made by its manufacturer, is not guaranteed or endorsed by the publisher.
References
1. Hutchings, MI, Truman, AW, and Wilkinson, B. Antibiotics: past, present and future. Curr Opin Microbiol. (2019) 51:72–80. doi: 10.1016/j.mib.2019.10.008
2. Centers for Disease Control and Prevention, (2019). Antibiotic use in the United States, 2018 update: progress and opportunities. Available at: https://Www.Cdc.Gov/Antibiotic-Use/Stewardship-Report/Pdf/Stewardship-Report-2018-508.Pdf (Accessed February 20th, 2024).
3. Bara, W, Brun-Buisson, C, Coignard, B, and Watier, L. Outpatient antibiotic prescriptions in France: patients and providers characteristics and impact of the Covid-19 pandemic. Antibiotics. (2022) 11:643. doi: 10.3390/antibiotics11050643
4. Fernandez-Urrusuno, R, Meseguer Barros, CM, Anaya-Ordonez, S, Borrego Izquierdo, Y, Lallana-Alvarez, MJ, Madridejos, R, et al. Patients receiving a high burden of antibiotics in the Community in Spain: a cross-sectional study. Pharmacol Res Perspect. (2021) 9:e00692. doi: 10.1002/prp2.692
5. Fink, G, D'Acremont, V, Leslie, HH, and Cohen, J. Antibiotic exposure among children younger than 5 years in low-income and middle-income countries: a cross-sectional study of nationally representative facility-based and household-based surveys. Lancet Infect Dis. (2020) 20:179–87. doi: 10.1016/S1473-3099(19)30572-9
6. Sijbom, M, Buchner, FL, Saadah, NH, Numans, ME, and de Boer, MGJ. Determinants of inappropriate antibiotic prescription in primary care in developed countries with general practitioners as gatekeepers: a systematic review and construction of a framework. BMJ Open. (2023) 13:e065006. doi: 10.1136/bmjopen-2022-065006
7. Kapoor, G, Saigal, S, and Elongavan, A. Action and resistance mechanisms of antibiotics: a guide for clinicians. J Anaesthesiol Clin Pharmacol. (2017) 33:300–5. doi: 10.4103/joacp.JOACP_349_15
8. Waitzberg, D, Guarner, F, Hojsak, I, Ianiro, G, Polk, DB, and Sokol, H. Can the evidence-based use of probiotics (notably Saccharomyces Boulardii Cncm I-745 and Lactobacillus rhamnosus gg) mitigate the clinical effects of antibiotic-associated dysbiosis? Adv Ther. (2024) 41:901–14. doi: 10.1007/s12325-024-02783-3
9. Perez, NB, Dorsen, C, and Squires, A. Dysbiosis of the gut microbiome: a concept analysis. J Holist Nurs. (2020) 38:223–32. doi: 10.1177/0898010119879527
10. Zimmermann, P, and Curtis, N. The effect of antibiotics on the composition of the intestinal microbiota - a systematic review. J Infect. (2019) 79:471–89. doi: 10.1016/j.jinf.2019.10.008
11. Palleja, A, Mikkelsen, KH, Forslund, SK, Kashani, A, Allin, KH, Nielsen, T, et al. Recovery of gut microbiota of healthy adults following antibiotic exposure. Nat Microbiol. (2018) 3:1255–65. doi: 10.1038/s41564-018-0257-9
12. Patangia, DV, Anthony Ryan, C, Dempsey, E, Paul Ross, R, and Stanton, C. Impact of antibiotics on the human microbiome and consequences for host health. Microbiology. (2022) 11:e1260. doi: 10.1002/mbo3.1260
13. Petersen, C, and Round, JL. Defining Dysbiosis and its influence on host immunity and disease. Cell Microbiol. (2014) 16:1024–33. doi: 10.1111/cmi.12308
14. Uddin, TM, Chakraborty, AJ, Khusro, A, Zidan, BRM, Mitra, S, Emran, TB, et al. Antibiotic resistance in microbes: history, mechanisms, therapeutic strategies and future prospects. J Infect Public Health. (2021) 14:1750–66. doi: 10.1016/j.jiph.2021.10.020
15. Elseviers, MM, Van Camp, Y, Nayaert, S, Dure, K, Annemans, L, Tanghe, A, et al. Prevalence and management of antibiotic associated diarrhea in general hospitals. BMC Infect Dis. (2015) 15:129. doi: 10.1186/s12879-015-0869-0
16. Anthony, WE, Wang, B, Sukhum, KV, D'Souza, AW, Hink, T, Cass, C, et al. Acute and persistent effects of commonly used antibiotics on the gut microbiome and resistome in healthy adults. Cell Rep. (2022) 39:110649. doi: 10.1016/j.celrep.2022.110649
17. Xiang, Y, Li, F, Peng, J, Qin, D, Yuan, M, and Liu, G. Risk factors and predictive model of diarrhea among patients with severe stroke. World Neurosurg. (2020) 136:213–9. doi: 10.1016/j.wneu.2019.12.125
18. Zhou, H, Xu, Q, Liu, Y, and Guo, LT. Risk factors, incidence, and morbidity associated with antibiotic-associated diarrhea in intensive care unit patients receiving antibiotic monotherapy. World J Clin Cases. (2020) 8:1908–15. doi: 10.12998/wjcc.v8.i10.1908
19. Guo, Q, Goldenberg, JZ, Humphrey, C, El Dib, R, and Johnston, BC. Probiotics for the prevention of pediatric antibiotic-associated diarrhea. Cochrane Database Syst Rev. (2019) 4:CD004827. doi: 10.1002/14651858.CD004827.pub5
20. Helander, HF, and Fandriks, L. Surface area of the digestive tract - revisited. Scand J Gastroenterol. (2014) 49:681–9. doi: 10.3109/00365521.2014.898326
21. Marchesi, JR, and Ravel, J. The vocabulary of microbiome research: a proposal. Microbiome. (2015) 3:31. doi: 10.1186/s40168-015-0094-5
22. Arumugam, M, Raes, J, Pelletier, E, Le Paslier, D, Yamada, T, Mende, DR, et al. Enterotypes of the human gut microbiome. Nature. (2011) 473:174–80. doi: 10.1038/nature09944
23. Robertson, RC, Manges, AR, Finlay, BB, and Prendergast, AJ. The human microbiome and child growth - first 1000 days and beyond. Trends Microbiol. (2019) 27:131–47. doi: 10.1016/j.tim.2018.09.008
24. Romano-Keeler, J, and Sun, J. The first 1000 days: assembly of the neonatal microbiome and its impact on health outcomes. Newborn. (2022) 1:219–26. doi: 10.5005/jp-journals-11002-0028
25. Mustard, JF. Canadian Progress in early child development - putting science into action. Paediatr Child Health. (2009) 14:689–90. doi: 10.1093/pch/14.10.689
26. Shonkoff, JP, Richter, L, van der Gaag, J, and Bhutta, ZA. An integrated scientific framework for child survival and early childhood development. Pediatrics. (2012) 129:e460–72. doi: 10.1542/peds.2011-0366
27. Black, RE, Victora, CG, Walker, SP, Bhutta, ZA, Christian, P, de Onis, M, et al. Maternal and child undernutrition and overweight in low-income and middle-income countries. Lancet. (2013) 382:427–51. doi: 10.1016/S0140-6736(13)60937-X
28. Bryce, J, Coitinho, D, Darnton-Hill, I, Pelletier, D, Pinstrup-Andersen, P, et al. Maternal and child undernutrition: effective action at National Level. Lancet. (2008) 371:510–26. doi: 10.1016/S0140-6736(07)61694-8
29. Beluska-Turkan, K, Korczak, R, Hartell, B, Moskal, K, Maukonen, J, Alexander, DE, et al. Nutritional gaps and supplementation in the first 1000 days. Nutrients. (2019) 11:2891. doi: 10.3390/nu11122891
30. Scott, JA. The first 1000 days: a critical period of nutritional opportunity and vulnerability. Nutr Diet. (2020) 77:295–7. doi: 10.1111/1747-0080.12617
31. Hoffman, DJ, Reynolds, RM, and Hardy, DB. Developmental origins of health and disease: current knowledge and potential mechanisms. Nutr Rev. (2017) 75:951–70. doi: 10.1093/nutrit/nux053
32. Ryznar, RJ, Phibbs, L, and Van Winkle, LJ. Epigenetic modifications at the Center of the Barker Hypothesis and Their Transgenerational Implications. Int J Environ Res Public Health. (2021) 18:12728. doi: 10.3390/ijerph182312728
33. Barker, DJ. The fetal and infant origins of adult disease. BMJ. (1990) 301:1111. doi: 10.1136/bmj.301.6761.1111
34. Banchi, P, Colitti, B, Opsomer, G, Rota, A, and Van Soom, A. The dogma of the sterile uterus revisited: does microbial seeding occur during fetal life in humans and animals? Reproduction. (2024) 167:e230078. doi: 10.1530/REP-23-0078
35. Aagaard, KM. Author response to comment on "the placenta harbors a unique microbiome". Sci Transl Med. (2014) 6:254lr3. doi: 10.1126/scitranslmed.3010007
36. Collado, MC, Rautava, S, Aakko, J, Isolauri, E, and Salminen, S. Human gut colonisation may be initiated in utero by distinct microbial communities in the placenta and amniotic fluid. Sci Rep. (2016) 6:23129. doi: 10.1038/srep23129
37. Kennedy, KM, de Goffau, MC, Perez-Munoz, ME, Arrieta, MC, Backhed, F, Bork, P, et al. Questioning the fetal microbiome illustrates pitfalls of low-biomass microbial studies. Nature. (2023) 613:639–49. doi: 10.1038/s41586-022-05546-8
38. Perez-Munoz, ME, Arrieta, MC, Ramer-Tait, AE, and Walter, J. A critical assessment of the "sterile womb" and "in utero colonization" hypotheses: implications for research on the Pioneer infant microbiome. Microbiome. (2017) 5:48. doi: 10.1186/s40168-017-0268-4
39. Romano-Keeler, J, and Weitkamp, JH. Maternal influences on fetal microbial colonization and immune development. Pediatr Res. (2015) 77:189–95. doi: 10.1038/pr.2014.163
40. Yassour, M, Vatanen, T, Siljander, H, Hamalainen, AM, Harkonen, T, Ryhanen, SJ, et al. Natural history of the infant gut microbiome and impact of antibiotic treatment on bacterial strain diversity and stability. Sci Transl Med. (2016) 8:343ra81. doi: 10.1126/scitranslmed.aad0917
41. Fouhy, F, Watkins, C, Hill, CJ, O'Shea, CA, Nagle, B, Dempsey, EM, et al. Perinatal factors affect the gut microbiota up to four years after birth. Nat Commun. (2019) 10:1517. doi: 10.1038/s41467-019-09252-4
42. Salminen, S, Gibson, GR, McCartney, AL, and Isolauri, E. Influence of mode of delivery on gut microbiota composition in seven year old children. Gut. (2004) 53:1388–9. doi: 10.1136/gut.2004.041640
43. Cetinbas, M, Thai, J, Filatava, E, Gregory, KE, and Sadreyev, RI. Long-term dysbiosis and fluctuations of gut microbiome in antibiotic treated preterm infants. iScience. (2023) 26:107995. doi: 10.1016/j.isci.2023.107995
44. Parkin, K, Christophersen, CT, Verhasselt, V, Cooper, MN, and Martino, D. Risk factors for gut Dysbiosis in early life. Microorganisms. (2021) 9:2066. doi: 10.3390/microorganisms9102066
45. Kwon, Y, Cho, YS, Lee, YM, Kim, SJ, Bae, J, and Jeong, SJ. Changes to gut microbiota following systemic antibiotic Administration in Infants. Antibiotics. (2022) 11:470. doi: 10.3390/antibiotics11040470
46. Schulman, J, Dimand, RJ, Lee, HC, Duenas, GV, Bennett, MV, and Gould, JB. Neonatal intensive care unit antibiotic use. Pediatrics. (2015) 135:826–33. doi: 10.1542/peds.2014-3409
47. Leroux, S, Zhao, W, Betremieux, P, Pladys, P, Saliba, E, Jacqz-Aigrain, E, et al. Therapeutic guidelines for prescribing antibiotics in neonates should be evidence-based: a French National Survey. Arch Dis Child. (2015) 100:394–8. doi: 10.1136/archdischild-2014-306873
48. Kadambari, S, Heath, PT, Sharland, M, Lewis, S, Nichols, A, and Turner, MA. Variation in gentamicin and vancomycin dosage and monitoring in UK neonatal units. J Antimicrob Chemother. (2011) 66:2647–50. doi: 10.1093/jac/dkr351
49. Wilkins, LJ, Monga, M, and Miller, AW. Defining dysbiosis for a cluster of chronic diseases. Sci Rep. (2019) 9:12918. doi: 10.1038/s41598-019-49452-y
50. Brussow, H. Problems with the concept of gut microbiota dysbiosis. Microb Biotechnol. (2020) 13:423–34. doi: 10.1111/1751-7915.13479
51. Drago, L, Valentina, C, and Fabio, P. Gut microbiota, dysbiosis and Colon lavage. Dig Liver Dis. (2019) 51:1209–13. doi: 10.1016/j.dld.2019.06.012
52. Bidell, MR, Hobbs, ALV, and Lodise, TP. Gut microbiome health and dysbiosis: a clinical primer. Pharmacotherapy. (2022) 42:849–57. doi: 10.1002/phar.2731
53. Levy, M, Kolodziejczyk, AA, Thaiss, CA, and Elinav, E. Dysbiosis and the immune system. Nat Rev Immunol. (2017) 17:219–32. doi: 10.1038/nri.2017.7
54. Malard, F, Dore, J, Gaugler, B, and Mohty, M. Introduction to host microbiome symbiosis in health and disease. Mucosal Immunol. (2021) 14:547–54. doi: 10.1038/s41385-020-00365-4
55. Chriett, S, Dabek, A, Wojtala, M, Vidal, H, Balcerczyk, A, and Pirola, L. Prominent action of butyrate over beta-hydroxybutyrate as histone deacetylase inhibitor, transcriptional modulator and anti-inflammatory molecule. Sci Rep. (2019) 9:742. doi: 10.1038/s41598-018-36941-9
56. Kaminsky, LW, Al-Sadi, R, and Ma, TY. Il-1beta and the intestinal epithelial tight junction barrier. Front Immunol. (2021) 12:767456. doi: 10.3389/fimmu.2021.767456
57. De Vadder, F, Grasset, E, Manneras Holm, L, Karsenty, G, Macpherson, AJ, Olofsson, LE, et al. Gut microbiota regulates maturation of the adult enteric nervous system via enteric serotonin networks. Proc Natl Acad Sci USA. (2018) 115:6458–63. doi: 10.1073/pnas.1720017115
58. Grondin, JA, and Khan, WI. Emerging roles of gut serotonin in regulation of immune response, microbiota composition and intestinal inflammation. J Can Assoc Gastroenterol. (2024) 7:88–96. doi: 10.1093/jcag/gwad020
59. Hou, Y, Li, J, and Ying, S. Tryptophan metabolism and gut microbiota: a novel regulatory Axis integrating the microbiome, immunity, and cancer. Metabolites. (2023) 13:1166. doi: 10.3390/metabo13111166
60. Wall, R, Cryan, JF, Ross, RP, Fitzgerald, GF, Dinan, TG, and Stanton, C. Bacterial neuroactive compounds produced by psychobiotics. Adv Exp Med Biol. (2014) 817:221–39. doi: 10.1007/978-1-4939-0897-4_10
61. Dahiya, D, Manuel, JV, and Nigam, PS. An overview of bioprocesses employing specifically selected microbial catalysts for gamma-aminobutyric acid production. Microorganisms. (2021) 9:2457. doi: 10.3390/microorganisms9122457
62. Ilan, Y. Leaky gut and the liver: a role for bacterial translocation in nonalcoholic steatohepatitis. World J Gastroenterol. (2012) 18:2609–18. doi: 10.3748/wjg.v18.i21.2609
63. Mu, Q, Kirby, J, Reilly, CM, and Luo, XM. Leaky gut as a danger signal for autoimmune diseases. Front Immunol. (2017) 8:598. doi: 10.3389/fimmu.2017.00598
64. Sadagopan, A, Mahmoud, A, Begg, M, Tarhuni, M, Fotso, M, Gonzalez, NA, et al. Understanding the role of the gut microbiome in diabetes and therapeutics targeting leaky gut: a systematic review. Cureus. (2023) 15:e41559. doi: 10.7759/cureus.41559
65. Kenny, DJ, Plichta, DR, Shungin, D, Koppel, N, Hall, AB, Fu, B, et al. Cholesterol metabolism by uncultured human gut bacteria influences host cholesterol level. Cell Host Microbe. (2020) 28:245–257.e6. doi: 10.1016/j.chom.2020.05.013
66. Kriaa, A, Bourgin, M, Potiron, A, Mkaouar, H, Jablaoui, A, Gerard, P, et al. Microbial impact on cholesterol and bile acid metabolism: current status and future prospects. J Lipid Res. (2019) 60:323–32. doi: 10.1194/jlr.R088989
67. Black, CJ, Drossman, DA, Talley, NJ, Ruddy, J, and Ford, AC. Functional gastrointestinal disorders: advances in understanding and management. Lancet. (2020) 396:1664–74. doi: 10.1016/S0140-6736(20)32115-2
68. Faye, AS, Allin, KH, Iversen, AT, Agrawal, M, Faith, J, Colombel, JF, et al. Antibiotic use as a risk factor for inflammatory bowel disease across the ages: a population-based cohort study. Gut. (2023) 72:663–70. doi: 10.1136/gutjnl-2022-327845
69. Kim, M, Park, SJ, Choi, S, Chang, J, Kim, SM, Jeong, S, et al. Association between antibiotics and dementia risk: a retrospective cohort study. Front Pharmacol. (2022) 13:888333. doi: 10.3389/fphar.2022.888333
70. Sanchez, E, Donat, E, Ribes-Koninckx, C, Fernandez-Murga, ML, and Sanz, Y. Duodenal-mucosal bacteria associated with celiac disease in children. Appl Environ Microbiol. (2013) 79:5472–9. doi: 10.1128/AEM.00869-13
71. Champagne-Jorgensen, K, Kunze, WA, Forsythe, P, Bienenstock, J, and McVey Neufeld, KA. Antibiotics and the nervous system: more than just the microbes? Brain Behav Immun. (2019) 77:7–15. doi: 10.1016/j.bbi.2018.12.014
72. Rybak, LP, Ramkumar, V, and Mukherjea, D. Ototoxicity of non-aminoglycoside antibiotics. Front Neurol. (2021) 12:652674. doi: 10.3389/fneur.2021.652674
73. Fusco, W, Lorenzo, MB, Cintoni, M, Porcari, S, Rinninella, E, Kaitsas, F, et al. Short-chain fatty-acid-producing Bacteria: key components of the human gut microbiota. Nutrients. (2023) 15:2211. doi: 10.3390/nu15092211
74. LA StoddartSmith, NJ, and Milligan, G. Free fatty acid receptors Ffa1, −2, and −3: pharmacology and pathophysiological functions. Pharmacol Rev. (2008) 60:405–17. doi: 10.1124/pr.108.00802
75. Alvarez-Curto, E, and Milligan, G. Metabolism meets immunity: the role of free fatty acid receptors in the immune system. Biochem Pharmacol. (2016) 114:3–13. doi: 10.1016/j.bcp.2016.03.017
76. Kotlo, K, Anbazhagan, AN, Priyamvada, S, Jayawardena, D, Kumar, A, Chen, Y, et al. The olfactory G protein-coupled receptor (Olfr-78/Or51e2) modulates the intestinal response to colitis. Am J Physiol Cell Physiol. (2020) 318:C502–13. doi: 10.1152/ajpcell.00454.2019
77. Pluznick, J. A novel SCFA receptor, the microbiota, and blood pressure regulation. Gut Microbes. (2014) 5:202–7. doi: 10.4161/gmic.27492
78. Hou, JJ, Ma, AH, and Qin, YH. Activation of the aryl hydrocarbon receptor in inflammatory bowel disease: insights from gut microbiota. Front Cell Infect Microbiol. (2023) 13:1279172. doi: 10.3389/fcimb.2023.1279172
79. Jin, UH, Cheng, Y, Park, H, Davidson, LA, Callaway, ES, Chapkin, RS, et al. Short chain fatty acids enhance aryl hydrocarbon (ah) responsiveness in mouse Colonocytes and Caco-2 human Colon Cancer cells. Sci Rep. (2017) 7:10163. doi: 10.1038/s41598-017-10824-x
80. Kespohl, M, Vachharajani, N, Luu, M, Harb, H, Pautz, S, Wolff, S, et al. The microbial metabolite butyrate induces expression of Th1-associated factors in Cd4(+) T cells. Front Immunol. (2017) 8:1036. doi: 10.3389/fimmu.2017.01036
81. Zhang, M, Zhou, Q, Dorfman, RG, Huang, X, Fan, T, Zhang, H, et al. Butyrate inhibits Interleukin-17 and generates Tregs to ameliorate colorectal colitis in rats. BMC Gastroenterol. (2016) 16:84. doi: 10.1186/s12876-016-0500-x
82. Gasaly, N, Hermoso, MA, and Gotteland, M. Butyrate and the fine-tuning of colonic homeostasis: implication for inflammatory bowel diseases. Int J Mol Sci. (2021) 22:3061. doi: 10.3390/ijms22063061
83. Tan, C, Yan, Q, Ma, Y, Fang, J, and Yang, Y. Recognizing the role of the Vagus nerve in depression from microbiota-gut brain Axis. Front Neurol. (2022) 13:1015175. doi: 10.3389/fneur.2022.1015175
84. Hung, LY, Boonma, P, Unterweger, P, Parathan, P, Haag, A, Luna, RA, et al. Neonatal antibiotics disrupt motility and enteric neural circuits in mouse Colon. Cell Mol Gastroenterol Hepatol. (2019) 8:298–300.e6. doi: 10.1016/j.jcmgh.2019.04.009
85. Bernabe, G, Shalata, MEM, Zatta, V, Bellato, M, Porzionato, A, Castagliuolo, I, et al. Antibiotic treatment induces long-lasting effects on gut microbiota and the enteric nervous system in mice. Antibiotics (Basel). (2023) 12:1000. doi: 10.3390/antibiotics12061000
86. Donohoe, DR, Garge, N, Zhang, X, Sun, W, O'Connell, TM, Bunger, MK, et al. The microbiome and butyrate regulate energy metabolism and autophagy in the mammalian Colon. Cell Metab. (2011) 13:517–26. doi: 10.1016/j.cmet.2011.02.018
87. Ghosh, SS, Wang, J, Yannie, PJ, and Ghosh, S. Intestinal barrier dysfunction, Lps translocation, and disease development. J Endocr Soc. (2020) 4:bvz039. doi: 10.1210/jendso/bvz039
88. Lu, YC, Yeh, WC, and Ohashi, PS. Lps/Tlr4 signal transduction pathway. Cytokine. (2008) 42:145–51. doi: 10.1016/j.cyto.2008.01.006
89. Tremblay, MS, Aubert, S, Barnes, JD, Saunders, TJ, Carson, V, Latimer-Cheung, AE, et al. Sedentary behavior research network (SBRN) - terminology consensus project process and outcome. Int J Behav Nutr Phys Act. (2017) 14:75. doi: 10.1186/s12966-017-0525-8
90. Carrera-Bastos, P, Fontes-Villalba, M, and O’Keefe, J. The Western diet and lifestyle and diseases of civilization. Res Rep Clin Cardiol. (2011) 2:15–35. doi: 10.2147/RRCC.S16919
91. Kopp, W. How Western diet and lifestyle drive the pandemic of obesity and civilization diseases. Diabetes Metab Syndr Obes. (2019) 12:2221–36. doi: 10.2147/DMSO.S216791
92. Patterson, R, McNamara, E, Tainio, M, de Sa, TH, Smith, AD, Sharp, SJ, et al. Sedentary behaviour and risk of all-cause, cardiovascular and cancer mortality, and incident type 2 diabetes: a systematic review and dose response meta-analysis. Eur J Epidemiol. (2018) 33:811–29. doi: 10.1007/s10654-018-0380-1
93. Bai, J, Hu, Y, and Bruner, DW. Composition of gut microbiota and its association with body mass index and lifestyle factors in a cohort of 7-18 years old children from the American gut project. Pediatr Obes. (2019) 14:e12480. doi: 10.1111/ijpo.12480
94. Castellanos, N, Diez, GG, Antunez-Almagro, C, Bailen, M, Bressa, C, Gonzalez Soltero, R, et al. A critical mutualism - competition interplay underlies the loss of microbial diversity in sedentary lifestyle. Front Microbiol. (2019) 10:3142. doi: 10.3389/fmicb.2019.03142
95. Houttu, V, Boulund, U, Nicolaou, M, Holleboom, AG, Grefhorst, A, Galenkamp, H, et al. Physical activity and dietary composition relate to differences in gut microbial patterns in a multi-ethnic cohort-the Helius study. Metabolites. (2021) 11:858. doi: 10.3390/metabo11120858
96. Tarracchini, C, Fontana, F, Lugli, GA, Mancabelli, L, Alessandri, G, Turroni, F, et al. Investigation of the ecological link between recurrent microbial human gut communities and physical activity. Microbiol Spectr. (2022) 10:e0042022. doi: 10.1128/spectrum.00420-22
97. Kulecka, M, Fraczek, B, Mikula, M, Zeber-Lubecka, N, Karczmarski, J, Paziewska, A, et al. The composition and richness of the gut microbiota differentiate the top polish endurance athletes from sedentary controls. Gut Microbes. (2020) 11:1374–84. doi: 10.1080/19490976.2020.1758009
98. Flint, HJ. The impact of nutrition on the human microbiome. Nutr Rev. (2012) 70:S10–3. doi: 10.1111/j.1753-4887.2012.00499.x
99. Graf, D, Di Cagno, R, Fak, F, Flint, HJ, Nyman, M, Saarela, M, et al. Contribution of diet to the composition of the human gut microbiota. Microb Ecol Health Dis. (2015) 26:26164. doi: 10.3402/mehd.v26.26164
100. Sanchez Cruz, C, Rojas Huerta, A, Lima Barrientos, J, Rodriguez, C, Devani, A, Boosahda, V, et al. Inflammatory bowel disease and cardiovascular disease: an integrative review with a focus on the gut microbiome. Cureus. (2024) 16:e65136. doi: 10.7759/cureus.65136
101. Yang, Z, Yang, M, Deehan, EC, Cai, C, Madsen, KL, Wine, E, et al. Dietary Fiber for the prevention of childhood obesity: a focus on the involvement of the gut microbiota. Gut Microbes. (2024) 16:2387796. doi: 10.1080/19490976.2024.2387796
102. Garcia, K, Ferreira, G, Reis, F, and Viana, SI. Impact of dietary sugars on gut microbiota and metabolic health. Diabetology. (2022) 3:549–60. doi: 10.3390/diabetology3040042
103. Do, MH, Lee, E, Oh, MJ, Kim, Y, and Park, HY. High-glucose or -fructose diet cause changes of the gut microbiota and metabolic disorders in mice without body weight change. Nutrients. (2018) 10:761. doi: 10.3390/nu10060761
104. Laffin, M, Fedorak, R, Zalasky, A, Park, H, Gill, A, Agrawal, A, et al. A high-sugar diet rapidly enhances susceptibility to colitis via depletion of luminal short-chain fatty acids in mice. Sci Rep. (2019) 9:12294. doi: 10.1038/s41598-019-48749-2
105. Aversa, Z, Atkinson, EJ, Schafer, MJ, Theiler, RN, Rocca, WA, Blaser, MJ, et al. Association of Infant Antibiotic Exposure with childhood health outcomes. Mayo Clin Proc. (2021) 96:66–77. doi: 10.1016/j.mayocp.2020.07.019
106. Hassouneh, SA, Loftus, M, and Yooseph, S. Linking inflammatory bowel disease symptoms to changes in the gut microbiome structure and function. Front Microbiol. (2021) 12:673632. doi: 10.3389/fmicb.2021.673632
107. Andoh, A, and Nishida, A. Alteration of the gut microbiome in inflammatory bowel disease. Digestion. (2023) 104:16–23. doi: 10.1159/000525925
108. Imai, T, Inoue, R, Kawada, Y, Morita, Y, Inatomi, O, Nishida, A, et al. Characterization of fungal Dysbiosis in Japanese patients with inflammatory bowel disease. J Gastroenterol. (2019) 54:149–59. doi: 10.1007/s00535-018-1530-7
109. Sartor, RB, and Wu, GD. Roles for intestinal Bacteria, viruses, and Fungi in pathogenesis of inflammatory bowel diseases and therapeutic approaches. Gastroenterology. (2017) 152:327–339.e4. doi: 10.1053/j.gastro.2016.10.012
110. Beaugerie, L, Langholz, E, Nyboe-Andersen, N, Pigneur, B, Sokol, H, and Epicom, E. Differences in epidemiological features between ulcerative colitis and Crohn's disease: the early life-programmed versus late dysbiosis hypothesis. Med Hypotheses. (2018) 115:19–21. doi: 10.1016/j.mehy.2018.03.009
111. Ungaro, R, Bernstein, CN, Gearry, R, Hviid, A, Kolho, KL, Kronman, MP, et al. Antibiotics associated with increased risk of new-onset Crohn's disease but not ulcerative colitis: a meta-analysis. Am J Gastroenterol. (2014) 109:1728–38. doi: 10.1038/ajg.2014.246
112. Troelsen, FS, and Jick, S. Antibiotic use in childhood and adolescence and risk of inflammatory bowel disease: a case-control study in the UK clinical practice research datalink. Inflamm Bowel Dis. (2020) 26:440–7. doi: 10.1093/ibd/izz137
113. Holota, Y, Dovbynchuk, T, Kaji, I, Vareniuk, I, Dzyubenko, N, Chervinska, T, et al. The long-term consequences of antibiotic therapy: role of colonic short-chain fatty acids (SCFA) system and intestinal barrier integrity. PLoS One. (2019) 14:e0220642. doi: 10.1371/journal.pone.0220642
114. Saffouri, GB, Shields-Cutler, RR, Chen, J, Yang, Y, Lekatz, HR, Hale, VL, et al. Small intestinal microbial dysbiosis underlies symptoms associated with functional gastrointestinal disorders. Nat Commun. (2019) 10:2012. doi: 10.1038/s41467-019-09964-7
115. Jones, MP, Shah, A, Walker, MM, Koloski, NA, Holtmann, G, and Talley, NJ. Antibiotic use but not gastrointestinal infection frequently precedes first diagnosis of functional gastrointestinal disorders. United European Gastroenterol J. (2021) 9:1074–80. doi: 10.1002/ueg2.12164
116. Dydensborg Sander, S, Nybo Andersen, AM, Murray, JA, Karlstad, O, Husby, S, and Stordal, K. Association between antibiotics in the first year of life and celiac disease. Gastroenterology. (2019) 156:2217–29. doi: 10.1053/j.gastro.2019.02.039
117. Sanz, Y. Effects of a gluten-free diet on gut microbiota and immune function in healthy adult humans. Gut Microbes. (2010) 1:135–7. doi: 10.4161/gmic.1.3.11868
118. Dash, S, Syed, YA, and Khan, MR. Understanding the role of the gut microbiome in brain development and its association with neurodevelopmental psychiatric disorders. Front Cell Dev Biol. (2022) 10:880544. doi: 10.3389/fcell.2022.880544
119. Lacorte, E, Gervasi, G, Bacigalupo, I, Vanacore, N, Raucci, U, and Parisi, P. A systematic review of the microbiome in children with neurodevelopmental disorders. Front Neurol. (2019) 10:727. doi: 10.3389/fneur.2019.00727
120. Lewandowska-Pietruszka, Z, Figlerowicz, M, and Mazur-Melewska, K. Microbiota in autism Spectrum disorder: a systematic review. Int J Mol Sci. (2023) 24:16660. doi: 10.3390/ijms242316660
121. Tao, Q, Shen, Y, Li, Y, Luo, H, Yuan, M, and Gan, J. Prenatal exposure to antibiotics and risk of neurodevelopmental disorders in offspring: a systematic review and meta-analysis. Front Neurol. (2022) 13:1045865. doi: 10.3389/fneur.2022.1045865
122. Slykerman, RF, Thompson, J, Waldie, KE, Murphy, R, Wall, C, and Mitchell, EA. Antibiotics in the first year of life and subsequent neurocognitive outcomes. Acta Paediatr. (2017) 106:87–94. doi: 10.1111/apa.13613
123. Kohler, O, Petersen, L, Mors, O, Mortensen, PB, Yolken, RH, Gasse, C, et al. Infections and exposure to anti-infective agents and the risk of severe mental disorders: a Nationwide study. Acta Psychiatr Scand. (2017) 135:97–105. doi: 10.1111/acps.12671
124. Kohler-Forsberg, O, Petersen, L, Gasse, C, Mortensen, PB, Dalsgaard, S, Yolken, RH, et al. A Nationwide study in Denmark of the association between treated infections and the subsequent risk of treated mental disorders in children and adolescents. JAMA Psychiatry. (2019) 76:271–9. doi: 10.1001/jamapsychiatry.2018.3428
125. Ordonez-Rodriguez, A, Roman, P, Rueda-Ruzafa, L, Campos-Rios, A, and Cardona, D. Changes in gut microbiota and multiple sclerosis: a systematic review. Int J Environ Res Public Health. (2023) 20:4624. doi: 10.3390/ijerph20054624
126. Arvonen, M, Virta, LJ, Pokka, T, Kroger, L, and Vahasalo, P. Repeated exposure to antibiotics in infancy: a predisposing factor for juvenile idiopathic arthritis or a sign of this Group's greater susceptibility to infections? J Rheumatol. (2015) 42:521–6. doi: 10.3899/jrheum.140348
127. Horton, DB, Scott, FI, Haynes, K, Putt, ME, Rose, CD, Lewis, JD, et al. Antibiotic exposure and juvenile idiopathic arthritis: a case-control study. Pediatrics. (2015) 136:e333–43. doi: 10.1542/peds.2015-0036
128. McDonnell, L, Gilkes, A, Ashworth, M, Rowland, V, Harries, TH, Armstrong, D, et al. Association between antibiotics and gut microbiome dysbiosis in children: systematic review and Meta-analysis. Gut Microbes. (2021) 13:1–18. doi: 10.1080/19490976.2020.1870402
129. Hestetun, S, Andersen, S, Sanner, H, and Stordal, K. Antibiotic exposure in prenatal and early life and risk of juvenile idiopathic arthritis: a Nationwide register-based cohort study. RMD Open. (2023) 9:e003333. doi: 10.1136/rmdopen-2023-003333
130. Asadi, A, Shadab Mehr, N, Mohamadi, MH, Shokri, F, Heidary, M, Sadeghifard, N, et al. Obesity and gut-microbiota-brain Axis: a narrative review. J Clin Lab Anal. (2022) 36:e24420. doi: 10.1002/jcla.24420
131. Hu, J, Guo, P, Mao, R, Ren, Z, Wen, J, Yang, Q, et al. Gut microbiota signature of obese adults across different classifications. Diabetes Metab Syndr Obes. (2022) 15:3933–47. doi: 10.2147/DMSO.S387523
132. Peters, BA, Shapiro, JA, Church, TR, Miller, G, Trinh-Shevrin, C, Yuen, E, et al. A taxonomic signature of obesity in a large study of American adults. Sci Rep. (2018) 8:9749. doi: 10.1038/s41598-018-28126-1
133. Ajslev, TA, Andersen, CS, Gamborg, M, Sorensen, TI, and Jess, T. Childhood overweight after establishment of the gut microbiota: the role of delivery mode, pre-pregnancy weight and early Administration of Antibiotics. Int J Obes. (2011) 35:522–9. doi: 10.1038/ijo.2011.27
134. Mueller, NT, Whyatt, R, Hoepner, L, Oberfield, S, Dominguez-Bello, MG, Widen, EM, et al. Prenatal exposure to antibiotics, cesarean section and risk of childhood obesity. Int J Obes. (2015) 39:665–70. doi: 10.1038/ijo.2014.180
135. Saari, A, Virta, LJ, Sankilampi, U, Dunkel, L, and Saxen, H. Antibiotic exposure in infancy and risk of being overweight in the first 24 months of life. Pediatrics. (2015) 135:617–26. doi: 10.1542/peds.2014-3407
136. Akagawa, S, and Kaneko, K. Gut microbiota and allergic diseases in children. Allergol Int. (2022) 71:301–9. doi: 10.1016/j.alit.2022.02.004
137. Huang, H, Jiang, J, Wang, X, Jiang, K, and Cao, H. Exposure to prescribed medication in early life and impacts on gut microbiota and disease development. EClinicalMedicine. (2024) 68:102428. doi: 10.1016/j.eclinm.2024.102428
138. Ahmadizar, F, Vijverberg, SJH, Arets, HGM, de Boer, A, Lang, JE, Garssen, J, et al. Early-life antibiotic exposure increases the risk of developing allergic symptoms later in life: a meta-analysis. Allergy. (2018) 73:971–86. doi: 10.1111/all.13332
139. Lu, Y, Wang, Y, Wang, J, Lowe, AJ, Grzeskowiak, LE, and Hu, YJ. Early-life antibiotic exposure and childhood asthma trajectories: a National Population-Based Birth Cohort. Antibiotics. (2023) 12:314. doi: 10.3390/antibiotics12020314
140. Bentouhami, H, Bungwa, MK, Casas, L, Coenen, S, and Weyler, J. Asthma occurrence in children and early life systemic antibiotic use: an incidence density study. Allergy Asthma Clin Immunol. (2023) 19:18. doi: 10.1186/s13223-023-00773-8
141. Schoch, JJ, Satcher, KG, Garvan, CW, Monir, RL, Neu, J, and Lemas, DJ. Association between early life antibiotic exposure and development of early childhood atopic dermatitis. JAAD Int. (2023) 10:68–74. doi: 10.1016/j.jdin.2022.11.002
142. Chen, J, Yue, Y, Wang, L, Deng, Z, Yuan, Y, Zhao, M, et al. Altered gut microbiota correlated with systemic inflammation in children with Kawasaki disease. Sci Rep. (2020) 10:14525. doi: 10.1038/s41598-020-71371-6
143. Kaneko, K, Akagawa, S, Akagawa, Y, Kimata, T, and Tsuji, S. Our evolving understanding of Kawasaki disease pathogenesis: role of the gut microbiota. Front Immunol. (2020) 11:1616. doi: 10.3389/fimmu.2020.01616
144. Teramoto, Y, Akagawa, S, Hori, SI, Tsuji, S, Higasa, K, and Kaneko, K. Dysbiosis of the gut microbiota as a susceptibility factor for Kawasaki disease. Front Immunol. (2023) 14:1268453. doi: 10.3389/fimmu.2023.1268453
145. Kim, TH, Shin, JS, Kim, SY, and Kim, J. Association of previous antibiotics use and Kawasaki disease: a cohort study of 106,908 patients. Pediatr Infect Dis J. (2024) 43:643–50. doi: 10.1097/INF.0000000000004335
146. Fukazawa, M Jr, Fukazawa, M, Nanishi, E, Nishio, H, Ichihara, K, and Ohga, S. Previous Antibiotic Use and the development of Kawasaki disease: a matched pair case-control study. Pediatr Int. (2020) 62, 62:1044–8. doi: 10.1111/ped.14255
147. Burns, JC. The etiologies of Kawasaki disease. J Clin Invest. (2024) 134:e176938. doi: 10.1172/JCI176938
148. Kappel, BA, De Angelis, L, Heiser, M, Ballanti, M, Stoehr, R, Goettsch, C, et al. Cross-omics analysis revealed gut microbiome-related metabolic pathways underlying atherosclerosis development after antibiotics treatment. Mol Metab. (2020) 36:100976. doi: 10.1016/j.molmet.2020.100976
149. Amadei, SS, and Notario, V. A significant question in cancer risk and therapy: are antibiotics positive or negative effectors? Current answers and possible alternatives. Antibiotics. (2020) 9:580. doi: 10.3390/antibiotics9090580
150. Kilkkinen, A, Rissanen, H, Klaukka, T, Pukkala, E, Heliovaara, M, Huovinen, P, et al. Antibiotic use predicts an increased risk of cancer. Int J Cancer. (2008) 123:2152–5. doi: 10.1002/ijc.23622
151. Petrelli, F, Ghidini, M, Ghidini, A, Perego, G, Cabiddu, M, Khakoo, S, et al. Use of antibiotics and risk of Cancer: a systematic review and Meta-analysis of observational studies. Cancers (Basel). (2019) 11:1174. doi: 10.3390/cancers11081174
152. Cheung, KS, Chan, EW, Tam, A, Wong, IOL, Seto, WK, Hung, IFN, et al. Association between antibiotic consumption and colon and rectal cancer development in older individuals: a territory-wide study. Cancer Med. (2022) 11:3863–72. doi: 10.1002/cam4.4759
153. Kim, M, Park, SJ, Choi, S, Jeong, S, Chang, J, Park, YJ, et al. Association of antibiotic use with risk of lung cancer: a Nationwide cohort study. J Infect Public Health. (2023) 16:1123–30. doi: 10.1016/j.jiph.2023.05.006
154. Tamim, HM, Hanley, JA, Hajeer, AH, Boivin, JF, and Collet, JP. Risk of breast cancer in relation to antibiotic use. Pharmacoepidemiol Drug Saf. (2008) 17:144–50. doi: 10.1002/pds.1512
155. Zhang, L, and Han, DM. An introduction of allergic rhinitis and its impact on asthma (Aria) 2008 update. Zhonghua Er Bi Yan Hou Tou Jing Wai Ke Za Zhi. (2008) 43:552–7.
156. Qu, G, Sun, C, Sharma, M, Uy, JP, Song, EJ, Bhan, C, et al. Is antibiotics use really associated with increased risk of colorectal cancer? An updated systematic review and meta-analysis of observational studies. Int J Color Dis. (2020) 35:1397–412. doi: 10.1007/s00384-020-03658-z
157. Zhang, J, Haines, C, Watson, AJM, Hart, AR, Platt, MJ, Pardoll, DM, et al. Oral antibiotic use and risk of colorectal cancer in the United Kingdom, 1989-2012: a matched case-control study. Gut. (2019) 68:1971–8. doi: 10.1136/gutjnl-2019-318593
158. Luchen, CC, Chibuye, M, Spijker, R, Simuyandi, M, Chisenga, C, Bosomprah, S, et al. Impact of antibiotics on gut microbiome composition and Resistome in the first years of life in low- to middle-income countries: a systematic review. PLoS Med. (2023) 20:e1004235. doi: 10.1371/journal.pmed.1004235
159. Agamennone, V, Krul, CAM, Rijkers, G, and Kort, R. A practical guide for probiotics applied to the case of antibiotic-associated diarrhea in the Netherlands. BMC Gastroenterol. (2018) 18:103. doi: 10.1186/s12876-018-0831-x
160. Goodman, C, Keating, G, Georgousopoulou, E, Hespe, C, and Levett, K. Probiotics for the prevention of antibiotic-associated Diarrhoea: a systematic review and meta-analysis. BMJ Open. (2021) 11:e043054. doi: 10.1136/bmjopen-2020-043054
161. Kopacz, K, and Phadtare, S. Probiotics for the prevention of antibiotic-associated diarrhea. Healthcare. (2022) 10:1450. doi: 10.3390/healthcare10081450
162. Su, GL, Ko, CW, Bercik, P, Falck-Ytter, Y, Sultan, S, Weizman, AV, et al. Aga clinical practice guidelines on the role of probiotics in the management of gastrointestinal disorders. Gastroenterology. (2020) 159:697–705. doi: 10.1053/j.gastro.2020.05.059
163. Szajewska, H, Berni Canani, R, Domellof, M, Guarino, A, Hojsak, I, Indrio, F, et al. Probiotics for the Management of Pediatric Gastrointestinal Disorders: Position Paper of the Espghan Special Interest Group on Gut Microbiota and Modifications. J Pediatr Gastroenterol Nutr. (2023) 76:20221011. doi: 10.1097/MPG.0000000000003633
164. Limketkai, BN, Akobeng, AK, Gordon, M, and Adepoju, AA. Probiotics for induction of remission in Crohn's disease. Cochrane Database Syst Rev. (2020) 7:CD006634. doi: 10.1002/14651858.CD006634.pub3
165. Rolfe, VE, Fortun, PJ, Hawkey, CJ, and Bath-Hextall, F. Probiotics for maintenance of remission in Crohn's disease. Cochrane Database Syst Rev. (2006) 4:CD004826. doi: 10.1002/14651858.CD004826.pub2
166. World Gastroenterology Organisation. Global guidelines probiotics and prebiotics. (2023). Available at: https://www.worldgastroenterology.org/userfiles/file/guidelines/probiotics-and-prebiotics-english-2023.pdf (Accessed 1st May, 2024).
167. Andreasen, AS, Larsen, N, Pedersen-Skovsgaard, T, Berg, RM, Moller, K, Svendsen, KD, et al. Effects of Lactobacillus acidophilus NCFM on insulin sensitivity and the systemic inflammatory response in human subjects. Br J Nutr. (2010) 104:1831–8. doi: 10.1017/S0007114510002874
168. Ayesha, IE, Monson, NR, Klair, N, Patel, U, Saxena, A, Patel, D, et al. Probiotics and their role in the Management of Type 2 diabetes mellitus (short-term versus long-term effect): a systematic review and Meta-analysis. Cureus. (2023) 15:e46741. doi: 10.7759/cureus.46741
169. Azad, MB, Coneys, JG, Kozyrskyj, AL, Field, CJ, Ramsey, CD, Becker, AB, et al. Probiotic supplementation during pregnancy or infancy for the prevention of asthma and wheeze: systematic review and meta-analysis. BMJ. (2013) 347:f6471. doi: 10.1136/bmj.f6471
170. Chen, AC, Fang, TJ, Ho, HH, Chen, JF, Kuo, YW, Huang, YY, et al. A multi-strain probiotic blend reshaped obesity-related gut Dysbiosis and improved lipid metabolism in obese children. Front Nutr. (2022) 9:922993. doi: 10.3389/fnut.2022.922993
171. Chudzik, A, Orzylowska, A, Rola, R, and Stanisz, GJ. Probiotics, prebiotics and Postbiotics on mitigation of depression symptoms: modulation of the brain-gut-microbiome Axis. Biomol Ther. (2021) 11:1000. doi: 10.3390/biom11071000
172. Cuello-Garcia, CA, Brozek, JL, Fiocchi, A, Pawankar, R, Yepes-Nunez, JJ, Terracciano, L, et al. Probiotics for the prevention of allergy: a systematic review and meta-analysis of randomized controlled trials. J Allergy Clin Immunol. (2015) 136:952–61. doi: 10.1016/j.jaci.2015.04.031
173. de Oliveira, GLV, Leite, AZ, Higuchi, BS, Gonzaga, MI, and Mariano, VS. Intestinal dysbiosis and probiotic applications in autoimmune diseases. Immunology. (2017) 152:1–12. doi: 10.1111/imm.12765
174. de Roos, NM, van Hemert, S, Rovers, JMP, Smits, MG, and Witteman, BJM. The effects of a multispecies probiotic on migraine and markers of intestinal permeability-results of a randomized placebo-controlled study. Eur J Clin Nutr. (2017) 71:1455–62. doi: 10.1038/ejcn.2017.57
175. Di Costanzo, M, Vella, A, Infantino, C, Morini, R, Bruni, S, Esposito, S, et al. Probiotics in infancy and childhood for food allergy prevention and treatment. Nutrients. (2024) 16:297. doi: 10.3390/nu16020297
176. Dosh, L, Ghazi, M, Haddad, K, El Masri, J, Hawi, J, Leone, A, et al. Probiotics, gut microbiome, and cardiovascular diseases: an update. Transpl Immunol. (2024) 83:102000. doi: 10.1016/j.trim.2024.102000
177. Fedorak, RN, Feagan, BG, Hotte, N, Leddin, D, Dieleman, LA, Petrunia, DM, et al. The probiotic Vsl#3 has anti-inflammatory effects and could reduce endoscopic recurrence after surgery for Crohn's disease. Clin Gastroenterol Hepatol. (2015) 13:928–935.e2. doi: 10.1016/j.cgh.2014.10.031
178. Gao, J, Zhao, L, Cheng, Y, Lei, W, Wang, Y, Liu, X, et al. Probiotics for the treatment of depression and its comorbidities: a systemic review. Front Cell Infect Microbiol. (2023) 13:1167116. doi: 10.3389/fcimb.2023.1167116
179. Garcia Vilela, E, de Lourdes de Abreu Ferrari, M, Oswaldo da Gama Torres, H, Guerra Pinto, A, Carolina Carneiro Aguirre, A, Paiva Martins, F, et al. Influence of Saccharomyces Boulardii on the intestinal permeability of patients with Crohn's disease in remission. Scand J Gastroenterol. (2008) 43:842–8. doi: 10.1080/00365520801943354
180. Guedes, MR, Pontes, K, Barreto Silva, MI, Neves, MF, and Klein, M. Randomized controlled trials reporting the effects of probiotics in individuals with overweight and obesity: a critical review of the interventions and body adiposity parameters. Clin Nutr. (2023) 42:835–47. doi: 10.1016/j.clnu.2023.03.017
181. Guo, L, Xu, J, Du, Y, Wu, W, Nie, W, Zhang, D, et al. Effects of gut microbiota and probiotics on Alzheimer's disease. Transl Neurosci. (2021) 12:573–80. doi: 10.1515/tnsci-2020-0203
182. Johnson, D, Letchumanan, V, Thum, CC, Thurairajasingam, S, and Lee, LH. A microbial-based approach to mental health: the potential of probiotics in the treatment of depression. Nutrients. (2023) 15:1382. doi: 10.3390/nu15061382
183. Kamarli Altun, H, Akal Yildiz, E, and Akin, M. Effects of Synbiotic therapy in mild-to-moderately active ulcerative colitis: a randomized placebo-controlled study. Turk J Gastroenterol. (2019) 30:313–20. doi: 10.5152/tjg.2019.18356
184. Lau, E, Neves, JS, Ferreira-Magalhaes, M, Carvalho, D, and Freitas, P. Probiotic ingestion, obesity, and metabolic-related disorders: results from NHANES, 1999-2014. Nutrients. (2019) 11:1482. doi: 10.3390/nu11071482
185. Leblhuber, F, Steiner, K, Schuetz, B, Fuchs, D, and Gostner, JM. Probiotic supplementation in patients with Alzheimer's dementia - an explorative intervention study. Curr Alzheimer Res. (2018) 15:1106–13. doi: 10.2174/1389200219666180813144834
186. Leon Aguilera, XE, Manzano, A, Pirela, D, and Bermudez, V. Probiotics and gut microbiota in obesity: myths and realities of a new health revolution. J Pers Med. (2022) 12:1282. doi: 10.3390/jpm12081282
187. Loke, P, Orsini, F, Lozinsky, AC, Gold, M, O'Sullivan, MD, Quinn, P, et al. Probiotic Peanut Oral immunotherapy versus Oral immunotherapy and placebo in children with Peanut allergy in Australia (Ppoit-003): a multicentre, randomised, phase 2b trial. Lancet Child Adolesc Health. (2022) 6:171–84. doi: 10.1016/S2352-4642(22)00006-2
188. Maftei, NM, Raileanu, CR, Balta, AA, Ambrose, L, Boev, M, Marin, DB, et al. The potential impact of probiotics on human health: an update on their health-promoting properties. Microorganisms. (2024) 12:234. doi: 10.3390/microorganisms12020234
189. Makrgeorgou, A, Leonardi-Bee, J, Bath-Hextall, FJ, Murrell, DF, Tang, ML, Roberts, A, et al. Probiotics for treating eczema. Cochrane Database Syst Rev. (2018) 11:CD006135. doi: 10.1002/14651858.CD006135.pub3
190. Martami, F, Togha, M, Seifishahpar, M, Ghorbani, Z, Ansari, H, Karimi, T, et al. The effects of a multispecies probiotic supplement on inflammatory markers and episodic and chronic migraine characteristics: a randomized double-blind controlled trial. Cephalalgia. (2019) 39:841–53. doi: 10.1177/0333102418820102
191. Matsuoka, K, Uemura, Y, Kanai, T, Kunisaki, R, Suzuki, Y, Yokoyama, K, et al. Efficacy of Bifidobacterium breve fermented Milk in maintaining remission of ulcerative colitis. Dig Dis Sci. (2018) 63:1910–9. doi: 10.1007/s10620-018-4946-2
192. Meng, HYH, Mak, CCH, Mak, WY, Zuo, T, Ko, H, and Chan, FKL. Probiotic supplementation demonstrates therapeutic potential in treating gut dysbiosis and improving neurocognitive function in age-related dementia. Eur J Nutr. (2022) 61:1701–34. doi: 10.1007/s00394-021-02760-4
193. Merkouris, E, Mavroudi, T, Miliotas, D, Tsiptsios, D, Serdari, A, Christidi, F, et al. Probiotics' effects in the treatment of anxiety and depression: a comprehensive review of 2014-2023 clinical trials. Microorganisms. (2024) 12:411. doi: 10.3390/microorganisms12020411
194. Miele, E, Pascarella, F, Giannetti, E, Quaglietta, L, Baldassano, RN, and Staiano, A. Effect of a probiotic preparation (Vsl#3) on induction and maintenance of remission in children with ulcerative colitis. Am J Gastroenterol. (2009) 104:437–43. doi: 10.1038/ajg.2008.118
195. Mishra, V, Yadav, D, Solanki, KS, Koul, B, and Song, M. A review on the protective effects of probiotics against Alzheimer's disease. Biology. (2023) 13:8. doi: 10.3390/biology13010008
196. Mozafarybazargany, M, Khonsari, M, Sokoty, L, Ejtahed, HS, and Qorbani, M. The effects of probiotics on gastrointestinal symptoms and microbiota in patients with celiac disease: a systematic review and meta-analysis on clinical trials. Clin Exp Med. (2023) 23:2773–88. doi: 10.1007/s10238-022-00987-x
197. Naghibi, MM, Day, R, Stone, S, and Harper, A. Probiotics for the prophylaxis of migraine: a systematic review of randomized placebo controlled trials. J Clin Med. (2019) 8:1441. doi: 10.3390/jcm8091441
198. Oliva, S, Di Nardo, G, Ferrari, F, Mallardo, S, Rossi, P, Patrizi, G, et al. Randomised clinical trial: the effectiveness of Lactobacillus reuteri Atcc 55730 rectal Enema in children with active distal ulcerative colitis. Aliment Pharmacol Ther. (2012) 35:327–34. doi: 10.1111/j.1365-2036.2011.04939.x
199. Paquette, S, Thomas, SC, Venkataraman, K, Appanna, VD, and Tharmalingam, S. The effects of Oral probiotics on type 2 diabetes mellitus (T2dm): a clinical trial systematic literature review. Nutrients. (2023) 15:4690. doi: 10.3390/nu15214690
200. Paul, AK, Paul, A, Jahan, R, Jannat, K, Bondhon, TA, Hasan, A, et al. Probiotics and amelioration of rheumatoid arthritis: significant roles of Lactobacillus Casei and Lactobacillus acidophilus. Microorganisms. (2021) 9:1070. doi: 10.3390/microorganisms9051070
201. Ribeiro, JF, and Pedrosa, C. Probiotics, prebiotics and food allergy. Eur Ann Allergy Clin Immunol. (2023). doi: 10.23822/EurAnnACI.1764-1489.319
202. Ribera, C, Sanchez-Orti, JV, Clarke, G, Marx, W, Morkl, S, and Balanza-Martinez, V. Probiotic, prebiotic, Synbiotic and fermented food supplementation in psychiatric disorders: a systematic review of clinical trials. Neurosci Biobehav Rev. (2024) 158:105561. doi: 10.1016/j.neubiorev.2024.105561
203. Sivamaruthi, BS, Kesika, P, and Chaiyasut, C. The role of probiotics in colorectal Cancer management. Evid Based Complement Alternat Med. (2020) 2020:3535982. doi: 10.1155/2020/3535982
204. Suzuki, T. Regulation of the intestinal barrier by nutrients: the role of tight junctions. Anim Sci J. (2020) 91:e13357. doi: 10.1111/asj.13357
205. Thakkar, A, Vora, A, Kaur, G, and Akhtar, J. Dysbiosis and Alzheimer's disease: role of probiotics, prebiotics and synbiotics. Naunyn Schmiedeberg's Arch Pharmacol. (2023) 396:2911–23. doi: 10.1007/s00210-023-02554-x
206. Yamaguchi, T, Tsuji, S, Akagawa, S, Akagawa, Y, Kino, J, Yamanouchi, S, et al. Clinical significance of probiotics for children with idiopathic nephrotic syndrome. Nutrients. (2021) 13:365. doi: 10.3390/nu13020365
207. Zhai, T, Wang, P, Hu, X, and Zheng, L. Probiotics bring new Hope for atherosclerosis prevention and treatment. Oxidative Med Cell Longev. (2022) 2022:3900835–13. doi: 10.1155/2022/3900835
208. Zhang, GQ, Hu, HJ, Liu, CY, Zhang, Q, Shakya, S, and Li, ZY. Probiotics for prevention of atopy and food hypersensitivity in early childhood: a Prisma-compliant systematic review and meta-analysis of randomized controlled trials. Medicine. (2016) 95:e2562. doi: 10.1097/MD.0000000000002562
209. Zhang, Q, Chen, B, Zhang, J, Dong, J, Ma, J, Zhang, Y, et al. Effect of prebiotics, probiotics, synbiotics on depression: results from a meta-analysis. BMC Psychiatry. (2023) 23:477. doi: 10.1186/s12888-023-04963-x
210. Zhao, J, Liao, Y, Wei, C, Ma, Y, Wang, F, Chen, Y, et al. Potential ability of probiotics in the prevention and treatment of colorectal cancer. Clin Med Insights Oncol. (2023) 17:11795549231188225. doi: 10.1177/11795549231188225
211. Hayer, SS, Hwang, S, and Clayton, JB. Antibiotic-induced gut dysbiosis and cognitive, emotional, and behavioral changes in rodents: a systematic review and meta-analysis. Front Neurosci. (2023) 17:1237177. doi: 10.3389/fnins.2023.1237177
212. Dhyani, P, Goyal, C, Dhull, SB, Chauhan, AK, Singh Saharan, B, Harshita,, et al. Psychobiotics for mitigation of neuro-degenerative diseases: recent advancements. Mol Nutr Food Res. (2023):e2300461. doi: 10.1002/mnfr.202300461
213. Ross, K. Psychobiotics: are they the future intervention for managing depression and anxiety? A literature review. Explore. (2023) 19:669–80. doi: 10.1016/j.explore.2023.02.007
214. McFarland, LV. Use of probiotics to correct Dysbiosis of Normal microbiota following disease or disruptive events: a systematic review. BMJ Open. (2014) 4:e005047. doi: 10.1136/bmjopen-2014-005047
215. Liu, T, Asif, IM, Chen, Y, Zhang, M, Li, B, and Wang, L. The relationship between diet, gut Mycobiome, and functional gastrointestinal disorders: evidence, doubts, and prospects. Mol Nutr Food Res. (2024) 68:e2300382. doi: 10.1002/mnfr.202300382
216. Bin, Z, Ya-Zheng, X, Zhao-Hui, D, Bo, C, Li-Rong, J, and Vandenplas, Y. The efficacy of Saccharomyces Boulardii Cncm I-745 in addition to standard Helicobacter pylori eradication treatment in children. Pediatr Gastroenterol Hepatol Nutr. (2015) 18:17–22. doi: 10.5223/pghn.2015.18.1.17
217. Kazmierczak-Siedlecka, K, Ruszkowski, J, Fic, M, Folwarski, M, and Makarewicz, W. Saccharomyces Boulardii Cncm I-745: a non-bacterial microorganism used as probiotic agent in supporting treatment of selected diseases. Curr Microbiol. (2020) 77:1987–96. doi: 10.1007/s00284-020-02053-9
218. More, MI, and Swidsinski, A. Saccharomyces Boulardii Cncm I-745 supports regeneration of the intestinal microbiota after diarrheic Dysbiosis - a review. Clin Exp Gastroenterol. (2015) 8:237–55. doi: 10.2147/CEG.S85574
219. Kabbani, TA, Pallav, K, Dowd, SE, Villafuerte-Galvez, J, Vanga, RR, Castillo, NE, et al. Prospective randomized controlled study on the effects of Saccharomyces Boulardii Cncm I-745 and amoxicillin-Clavulanate or the combination on the gut microbiota of healthy volunteers. Gut Microbes. (2017) 8:17–32. doi: 10.1080/19490976.2016.1267890
Keywords: antibiotics, probiotics, DOHaD, long-term, dysbiosis, chronic disease, neuroinflammation, SCFA
Citation: Guarner F, Bustos Fernandez L, Cruchet S, Damião A, Maruy Saito A, Riveros Lopez JP, Rodrigues Silva L and Valdovinos Diaz MA (2024) Gut dysbiosis mediates the association between antibiotic exposure and chronic disease. Front. Med. 11:1477882. doi: 10.3389/fmed.2024.1477882
Edited by:
Angel Lanas, University of Zaragoza, SpainReviewed by:
Jonathan Soldera, University of Caxias do Sul, BrazilMary Fafutis-Morris, University of Guadalajara, Mexico
Copyright © 2024 Guarner, Bustos Fernandez, Cruchet, Damião, Maruy Saito, Riveros Lopez, Rodrigues Silva and Valdovinos Diaz. This is an open-access article distributed under the terms of the Creative Commons Attribution License (CC BY). The use, distribution or reproduction in other forums is permitted, provided the original author(s) and the copyright owner(s) are credited and that the original publication in this journal is cited, in accordance with accepted academic practice. No use, distribution or reproduction is permitted which does not comply with these terms.
*Correspondence: Francisco Guarner, Zmd1YXJuZXJAaWNsb3VkLmNvbQ==