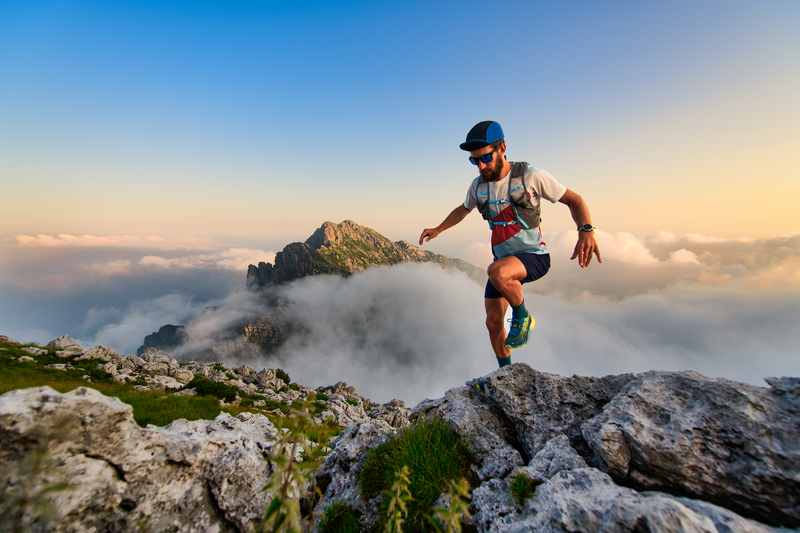
95% of researchers rate our articles as excellent or good
Learn more about the work of our research integrity team to safeguard the quality of each article we publish.
Find out more
MINI REVIEW article
Front. Med. , 18 July 2024
Sec. Dermatology
Volume 11 - 2024 | https://doi.org/10.3389/fmed.2024.1449597
Pathological scar tissues are characterized by the presence of overabundant collagens whose structure and organization are also different from those in unwounded skin. This causes scar tissues to lose some functions performed by normal skin, and currently, there are no effective measures to prevent scar formation. Inflammation has been shown to modulate fibroblast proliferation, differentiation, and function, hence collagen production and organization. In this minireview, we provide an overview of the current understanding of collagen, specifically collagen type I and III which are main collagens in skin, structure and fibre formation and highlight their differences between normal skin and pathological scars. We discuss the role that cytokines play in modulating fibroblast function. We also identify some potential research directions which could help to further our understanding of the complex and dynamic wound healing and scar formation process.
Skin is the largest organ in the human body and acts as a physical barrier to prevent harmful substances from entering the body, protecting the body against trauma and sensing stimuli. It consists of three layers: the innermost layer, the hypodermis, comprises loose areolar and adipose tissue which connect the skin to other structures to provide insulation and cushioning through fat storage. Above that lies the dermis which accounts for the majority of skin thickness at 3–5 mm and contains extracellular matrix (ECM) proteins, mainly collagens, and fibroblasts which produce ECMs. Collagens form well-organized networks to provide overall strength and integrity to the skin (1). The outermost layer, the epidermis, is the thinnest layer of skin but contains layers of different cells and proteins. Dead keratinocytes, hardened protein (keratins), and lipids form a protective barrier on the surface to seal the skin off from the outside environment.
Due to its exposure to external threats such as physical trauma, including wounds and burns, skin tissue can often get injured. Following injury, it undergoes a wound-healing process (2, 3) which starts with rapid clot formation which covers the injured site, preventing pathogen invasion. Immune cells are recruited to clear dead cells and fight against microbes, which is crucial to prevent the chronicity of inflammation. Many signaling proteins, mainly pro-inflammatory cytokines, are also produced which further recruit fibroblasts to the site and instruct them to proliferate and differentiate to produce collagen and other ECM components. In the late stages of wound healing, anti-inflammatory cytokines become predominant and excessive immune cells and fibroblasts undergo apoptosis. Deposited collagens undergo procession and reorganization, aiming to resolve to their unwounded state, however, in adult skin, this does not lead to 100% restoration and scar tissues form. Scars are characterized by excessive collagen deposition and altered collagen fibril structure and network arrangement (4, 5). In a hypertrophic scar, dense and disorganized collagens fill the wound site, but they expand beyond this in a keloid scar. This not only causes cosmetic deformities but also reduces skin flexibility and strength resulting in discomfort, pain, and even long term disability, which can dramatically affect a patient’s quality of life (6–8). It is estimated that clinical management of skin scarring costs the National Health Service (NHS) £8.3 billion annually in the UK alone (9).
Pathological scars have been shown to be caused by prolonged inflammation which leads to inappropriate fibroblast function during wound healing. Here, we review the current understanding of skin collagens, specifically type I and type III collagen structure and assembly, and highlight their differences in normal skin and pathological scars. We discuss the contribution of cytokines in scar formation through their modulation of fibroblast functions. We identify some research gaps to further our understanding of scar formation, and which can potentially be targeted for novel treatment development.
Collagen comprises about three quarters of the dry weight of human skin, and is the most prevalent component of the ECM (10, 11). The collagen family consists of 28 different members, (10, 12) however, collagen type I and type III are the main ones found in skin, constituting roughly 80–85% and 8–11% of the dermal ECM, respectively (13).
Each collagen molecule is a trimeric protein that consists of 3 parallel polypeptide chains intertwined together to form an elongated structure (10, 12). The collagen helical region contains a repetitive Gly-X-Y sequence, where X and Y can be any amino acid residue, but are frequently proline and hydroxyproline (10–13). This specific sequence allows three polypeptide chains to pack tightly together (10). Even though both collagen I and III share a high amount of amino acid sequence similarity, collagen III actually contains 5 more Gly-X-Y units in its helical region than collagen I (14).
All types of collagen are initially synthesized as a procollagen chain with N- and C-terminal propeptides flanking the collagen helical region (12) (Figure 1). The procollagen is then modified through the hydroxylation of proline and lysine residues, and glycosylation of selected hydroxylysine residues through enzyme-catalyzed reactions. Hydroxylation increases the thermal stability of collagen, and some hydroxylated residues are later further oxidized outside the cell, leading to collagen cross-linking which stabilizes assembled structures. The role of glycosylation in collagen stability is not fully clear.
Figure 1. Schematic illustrating the process of collagen triple-helix and fibril formation. (A) Shows the synthesis of procollagen, post-translational modification, where blue and yellow circles represent monosaccharides, disulphide bond (dark red lines) formation between the C-termini (red), and the helix propagation towards the N-termini (blue). Cleavage of N and C-terminal propeptides occurs outside the cell. (B) Shows a partially processed pN-collagen where only C-terminal propeptide is cleaved. (C) Shows a fully processed collagen where both termini are cleaved. (D) Demonstrates collagen fibril assembly and cross-linking formation, partially cleaved pN-collagens are found on the fibril surfaces. pN-collagens contain unstructured extra propeptides which prevent them from being fully incorporated into the center of the structurally well-organized collagen fibril.
The triple helix formation starts with C-terminal propeptide interactions, leading to the alignment of the three chains, and the inter-chain disulphide bond formation there further ensures they are in register (10). The triple helix propagates toward the N-termini (10, 11).
After trimeric structure formation, procollagens will be secreted outside the cell where C- and N- terminal propeptides will be cleaved. Resulting collagen type I and III molecules are both around 300 nm long (12) with a diameter of 1–2 nm (11, 12). But in the adult dermis, the N-termini propeptides (pN) in type III collagen, which contain 129 amino acids, are often only partially processed, leaving the pN-collagen type III to be deposited at detectable levels (15, 16).
In skin, collagens further assemble into collagen fibrils, and collagen type I and III can also co-assemble to form hybrid fibrils with diameters above ~100 nm (11, 12, 16, 17). Fibrils are formed by collagen molecules arranging into a linear staggered array (11–13, 18) (Figure 1). Lysine and hydroxylysine are also oxidized by lysyl oxidases (LOXs) (10, 11, 18–20) to form covalent bonds both between subunits within a collagen molecule (intra-molecular cross-linking), and between different collagen molecules (inter-molecular cross-linking) (10, 18, 21). Cross-linking stabilizes fibril structures, contributing to their stiffness and mechanical resilience, (11, 22) which provides the skin with mechanical strength and stability by resisting deformation under external stress (10, 21).
It has been suggested that in hybrid fibrils, collagen type I forms the bulk of the fibrils while collagen type III only forms a coat on the surface (23). However, a recent X-ray diffraction study on the colonic submucosa of rats showed that the positions of type I and III collagens in the fibril could be random (17). The discrepancy here can be attributed to the processing of procollagen type III, which takes longer than the N-terminal cleavage of procollagen type I (24). The presence of the N-termini non-collagen region prevents pN-collagen type III from being incorporated into the centre of the fibril effectively (24) (Figure 1). This could also explain the role collagen type III plays in modulating collagen I fibrogenesis and controlling fibril diameter (16, 17, 25), as the attachment of pN-collagen type III will cause steric hindrance, thereby preventing the fibril from growing further laterally.
In skin, collagen fibrils can further assemble into fibres and bundles whose diameters lie in the micrometre range (11), they then further pack closely to form ordered networks. It has been proposed that these are lattice-like plane structures, with collagen fibres lying parallel to the epidermis, with no out-of-plane components. Whilst a histological study and a recent study using combined multiphoton imaging showed that the majority of collagen fibres in the dermis run parallel, some fibres were found to orient out of the plane (26–28).
Collagens in pathological scars show a different structure and assembly. A recent Transmission Electron Microscopy (TEM) imaging on collagen fibril ultrastructure showed that the average collagen fibril diameter was significantly reduced in keloids (~76 nm) compared with that in normal skin (~124 nm). The average collagen fibril diameter was also found to be smaller in hypertrophic scars compared to normal skin (29–31). In mice, it was found that collagen fibrils after 14 days of healing had around half the diameter of those found in unwounded tissue (29).
SEM images of hypertrophic scars showed that the majority of fibre bundles are loosely arrayed in a wavy pattern that runs parallel to the epithelium, whereas in keloids, bundles are packed loosely with a random orientation (18, 32). Moreover, keloid collagen fibres are thicker than those of normal skin and hypertrophic scars (32, 33).
The relative ratio of type III to type I collagen was found to be reduced in pathological scars compared to the unscarred adult dermis (34, 35). However, it is not clear why the diameter of scar fibrils was found to be thinner, not thicker as expected.
Hydroxylation of type I collagen was also found to be significantly higher in keloids, and LOX activity is elevated in pathological scars compared to normal skin (18, 36). These lead to excessive collagen cross-linking which is consistent with the thicker fibres observed. Table 1 summarizes the differences of collagen in normal skin and pathological scars.
Table 1. Comparison of the collagen composition, assembly, and arrangements in normal skin and pathological scars.
Fibroblasts and myofibroblasts (collectively called (myo)fibroblasts) are responsible for ECM, and therefore collagen type I and III, production and deposition during wound healing (38). Fibroblasts migration and differentiation into myofibroblasts, which are also described as activated fibroblasts, is modulated by many factors including mechanical tension and cytokine signaling. Myofibroblasts are characterized by the expression of α-smooth muscle actin (α-SMA) stress fibres, which generate high contractile forces for wound contraction (39–41). Apart from collagen production, (myo)fibroblasts also modulate collagen properties through synthesizing enzymes such as LOXs, which catalyze collagen cross-linking in fibril formation. During normal scar formation, myofibroblasts will undergo apoptosis at the later stages of wound healing to prevent excessive collagen synthesis, however, they are found to be resistant to apoptosis in pathological fibrotic tissues which can promote fibrosis and scar formation (42). Some cell based studies do not differentiate between fibroblasts and myofibroblasts, and refer to both as fibroblasts.
It has been shown that pro-inflammatory cytokines stimulate fibroblast proliferation, differentiation and function, hence collagen production and assembly, however, the research results obtained can be controversial. Interleukin-1 (IL-1) is often categorized as pro-inflammatory cytokine, and its concentration is found to increase when the skin is injured (6, 43). It has two forms: IL-1α and IL-1β, which both can bind to the same IL-1 receptor type (IL-1R1) expressed on the surface of fibroblasts. Successful IL-1 signaling requires its binding to the third extra-cellular immunoglobulin domain of IL-1R1 and also a second receptor chain, termed either IL-1R accessory protein (IL-1RAcP) or IL-1R3, forming a trimeric signaling complex (44, 45). IL-1β can also bind to IL-1R2, a decoy receptor belonging to the IL-1R family (44, 45) which shares a very similar architecture with IL-R1, but which possesses only a very short intracellular segment (46), hence its binding inhibits the pro-inflammatory activity of IL-1β (45). Therefore, the function of IL-1β and IL-1α in stimulating fibroblast differentiation and collagen deposition can be different.
Early studies using cultured dermal fibroblasts showed that recombinant IL-1α or IL-1β stimulation resulted in cell proliferation and enhanced collagen production (47). The purified human IL-1 stimulation of cultured normal human dermal fibroblasts resulted in a nearly doubled production of collagen type I and type III compared to those without stimulation. Interestingly, a similar test using IL-1 on scleroderma fibroblasts from both affected and unaffected skin areas did not produce any obvious responses (48). However, in a separate study, recombinant human IL-1α or IL-1β showed decreased collagen type I and III accumulation by fibroblasts, although the mRNA levels of both collagens were found to have increased (49). These contradictory results make it difficult to determine the functions of IL-1α and IL-1β. The source of fibroblasts and different experimental conditions, such as the different bovine serums used to grow fibroblasts, could have affected fibroblast responses.
Despite these contradicting results, animal studies targeting IL-1 levels have been shown to inhibit pathological scar formation. The occlusion with silicone gel of a rabbit ear wound was found to reduce the expression of IL-1, and collagen deposition, inhibiting fibrosis and hypertrophic scarring (50). Curcumin treatment has been shown to significantly reduce hypertrophic scarring in New Zealand White rabbits, which was associated with a significant decrease in the production of pro-inflammatory cytokines including IL-1 and IL-6 (51).
IL-6 is another major regulator of the acute inflammatory response (52, 53). It signals by binding to an IL-6 receptor (IL-6R), and the IL-6/IL-6R complex is then associated with gp130, a protein expressed on all cells (54), to initiate intracellular signaling via the JAK/STAT pathway (54). IL-6, IL-6R, and gp130, are greatly elevated in keloid fibroblasts compared to those in normal skin (53). The effects of IL-6 include directly stimulating the migration of fibroblasts to sites of injury, increasing type I collagen expression and synthesis, and/or the production of transforming growth factor β (TGF-β) (52) which plays a very important role in the proliferation of fibroblasts, and their further differentiation into myofibroblasts (43). The inhibition of STAT3 expression to block IL-6’s signaling pathway or inhibit IL-6 in keloid fibroblast cultures has resulted in keloid tissue fibroblasts losing collagen production, impairing their proliferation (40, 55). IL-6 may also influence myofibroblast persistence, due to its ability to induce Bcl2 expression in fibroblasts, which has an anti-apoptotic signaling function (53). Myofibroblasts present in hypertrophic scars also were found to be resistant to apoptosis (56).
However, IL-6 can also exert anti-inflammatory activities (57). Later in the wound healing process, the expression of IL-6 is significantly decreased (53) and IL-6 and IL-10 (an anti-inflammatory cytokine) promote macrophage polarisation from the pro-inflammatory M1 phenotype to the anti-inflammatory M2 phenotype (53, 58). This switches off inflammation by enhancing secretion of anti-inflammatory cytokines such as IL-10 and growth factors such as TGF-β (53, 59). It seems concentrations of cytokines, and their environment modulate fibroblast responses but the underlying mechanism is unclear.
Despite its potential anti-inflammatory effects, treatments for pathological scarring mostly involve inhibiting IL-6’s pro-inflammatory functions (52). The intralesional injection of corticosteroids causes scar regression by reducing IL-6 expression (50). Corticosteroids have been shown to supress the inflammatory process in the wound (60), inhibit fibroblast growth (6, 61) and increase the activity of collagenase (62), resulting in the degradation of collagen in scars (6, 61, 62). Pirfenidone, an FDA-approved drug used to treat fibrotic disorders, has been shown to reduce IL-6 and other pro-inflammatory cytokines in the wounds of treated mice during the inflammatory phase of deep burn wound healing (63).
The transforming growth factor β (TGF-β) family contains 33 known proteins in humans (64) and are bifunctional regulators that can either inhibit or stimulate cell proliferation (43) and is involved in all stages of wound healing (65). Among them, three isoforms, TGF-β1, −β2, and -β3, are the best-studied ones (64). The canonical pathway for TGF-β involves the Smad family of transcriptional activators, which function to transmit TGF-β stimulations to the nucleus. Signaling through the canonical ALK5/Smad3 pathway is crucial for the pathogenesis of fibrosis in many tissues.
TGF-β1 directly promotes myofibroblast development by inducing the expression of α-SMA and ECM protein production (59, 66–68). Its overexpression induces and sustains the activation of keloid fibroblasts (43). The administration of TGF-β1 to normal and keloid fibroblasts was found to dramatically increase the levels of intracellular collagen type I and III in both cells (69). Moreover, the addition of TGF-β1 to foetal wounds (which are typically scar-free) has been shown to induce scar formation (68).
TGF-β2 also recruits fibroblasts to the wound site, resulting in increased collagen deposition (particularly type I and III) during matrix formation, which can lead to scar formation (43). When TGF-β1 and TGF-β2 were blocked with neutralising antibodies, the severity of scarring was found to be significantly decreased, further implicating their roles in scar formation (70).
Unlike TGF-β1 and TGF-β2, TGF-β3 inhibits scarring by limiting the inflammatory reaction and has been shown to reduce scar formation and promote better collagen organization when injected into adult wounds during clinical trials, and reduce scar formation in animal models (60, 65, 71–73). It is highly expressed in foetal wounds, which may act as a key contributor to the scarless healing phenotype. The ratio of TGF-β3 to TGF-β1/TGF-β2 expression in wounds is considered to be a determining factor for physiological or pathological wound healing.
IL-10 is a potent anti-inflammatory cytokine that signals via two receptors: IL-10RI and IL-10RII, which has been shown to promote crosstalk between the PI3K/AKT and STAT3 pathways to inhibit fibrosis-related gene expression (74). It has been consistently demonstrated to be a major mediator in preventing fibrosis in many animal models (58). It affects ECM remodeling by downregulating collagen synthesis in skin fibroblasts (74–77), enhancing proteolytic enzyme activity to lyse the ECM, and decreasing TGF-β1 expression to prevent fibrosis (58). It also inhibits the migration of inflammatory cells such as monocytes, macrophages, and neutrophils to control inflammation (75).
The overexpression of IL-10 has been found to decrease inflammation, and create a wound site with an environment resembling those found in embryos (50). In animal models, wound sites injected with IL-10 were found to release lower levels of inflammatory mediators and reduced collagen deposition than those injected with placebo (72, 75). The addition of IL-10 to CD1 mice resulted in visually improved scar formation that appeared closer to normal skin (50). These further support the contribution of inflammation to scar formation, but the mechanisms underlying how pro- and anti-inflammatory cytokine balancing is modulated in wound healing is not fully clear.
The successful application of high-resolution imaging techniques have demonstrated that the collagen structures and arrangement in scar tissue differ significantly from those of normal tissues, however, the underlying mechanisms remain unclear. The presence of pN-collagen type III has been shown to modulate skin collagen fibril diameter, but the lower collagen type III to type I ratio found in scars over that in normal skin does not conform to the thinner collagen fibrils observed in pathological scars. We speculate that the excessive and rapid synthesis of procollagen can lead to their incomplete processing, leading to procollagen molecules being only partially cleaved. The presence/accumulation of pN-collagen type I could cause steric hindrance, preventing fibrils from growing further to reach their normal dimensions. Evidence has been found that mixing collagen type I with pN-collagen type I indeed leads to the formation of thinner fibrils (15, 24). Mice with a deficiency in Meprin, a protease that cleaves propeptides from both procollagen type I and III, show thinner skin fibrils (78). The unprocessed propeptides in collagen can further enhance crosslinking, contributing to the thicker fibres in keloid scars.
N-proteinases, which are responsible for N-terminal proteptide cleavage, have been shown to exhibit maximal activity when procollagen is properly folded into a triple helix (79). Currently, it is still unclear whether rapid and excessive type I collagen formation affects triple helix formation properly and interferes with N-propeptide cleavage. It is also unclear whether inflammation affects N-proteinase expression and function. Enhancing N-proteinase expression and its activity may be targeted to improve the fibril structures in scars. However, this may only be effective before procollagens are assembled into fibrils.
Scar collagen fibers have been found to be oriented differently from those in normal skin, and the underlying molecular mechanisms remain not yet fully understood. In a healing myocardial infarcts study using engineered tissue analogs, fibroblasts have been shown to be able to remodel their surrounding collagen matrix by exerting contractile forces on the fibers in alignment with the cell’s orientation, or deposit new fibers parallel to the cell orientation (80, 81). However, the exact orientation of fibroblasts during wound healing is still unknown and requires further studies.
The current research may have overlooked the role of cross-talks between different cytokines, which could be taken as an area for further exploration. IL-1β has been shown to affect TGF-β1 instruction to human dermal fibroblasts. IL-1β alone had no effect on α-SMA formation, but it did reduce TGF-β1 stimulated myofibroblast formation, collagen type I mRNA levels and LOX activity. Moreover, it also increased collagen type III mRNA levels, and further enhanced TGF-β1 induced collagen type III mRNA levels compared to those exposed to TGF-β1 alone (82). The effects of IL-1β on TGF-β1 have been attributed to IL-1β reducing the expression of the Hedgehog transcription factor, Glioma-associated oncogene homolog 1 (GLI1). TGF-β1 stimulation upregulates GLI1 expression to facilitate fibroblast differentiation into myofibroblasts, hence its reduction inhibits myofibroblast formation (82).
It is believed that a dynamic balance of pro- and anti- inflammatory signals play a very important role in determining wound healing outcomes. Prolonged inflammation contributes to scar formation. The levels of pro- and anti- inflammatory cytokine expression have been shown to determine disease outcomes, and the injection of anti-inflammatory IL-10 lowers the levels of collagen deposition in wound healing, although one cytokine can also interfere with other cytokines’ signal transduction and hence their effects. For example, IL-6 has been shown to play an anti-inflammatory role by activating JAK/STAT signaling pathway of rheumatoid arthritis (RA) synovial fibroblasts, however, its anti-inflammatory activity was inhibited by treating cells with IL-1, which prevented STAT-dependent gene expression (83). Hence, in addition to controlling the levels of cytokine expressions, the modulation of their signal transduction can also be targeted to develop treatments against diseases. However, the current challenges here are that the molecular details of some cytokine signaling pathways are not yet fully clear, and some cytokines also share receptors (84), therefore more mechanistic studies are urgently needed.
CZ: Conceptualization, Writing – original draft, Writing – review & editing. YG: Supervision, Writing – original draft, Writing – review & editing.
The author(s) declare that no financial support was received for the research, authorship, and/or publication of this article.
The authors declare that the research was conducted in the absence of any commercial or financial relationships that could be construed as a potential conflict of interest.
All claims expressed in this article are solely those of the authors and do not necessarily represent those of their affiliated organizations, or those of the publisher, the editors and the reviewers. Any product that may be evaluated in this article, or claim that may be made by its manufacturer, is not guaranteed or endorsed by the publisher.
1. Gachon, E, and Mesquida, P. Stretching single collagen fibrils reveals nonlinear mechanical behavior. Biophys J. (2020) 118:1401–8. doi: 10.1016/j.bpj.2020.01.038
2. Pena, OA, and Martin, P. Cellular and molecular mechanisms of skin wound healing. Nat Rev Mol Cell Biol. (2024). doi: 10.1038/s41580-024-00715-1
3. Potter, DA, Veitch, D, and Johnston, GA. Scarring and wound healing. Br J Hosp Med (Lond). (2019) 80:C166–71. doi: 10.12968/hmed.2019.80.11.C166
4. Takeo, M, Lee, W, and Ito, M. Wound healing and skin regeneration. Cold Spring Harb Perspect Med. (2015) 5:a023267. doi: 10.1101/cshperspect.a023267
5. Gurtner, GC, Werner, S, Barrandon, Y, and Longaker, MT. Wound repair and regeneration. Nature. (2008) 453:314–21. doi: 10.1038/nature07039
6. Gauglitz, GG, Korting, HC, Pavicic, T, Ruzicka, T, and Jeschke, MG. Hypertrophic scarring and keloids: pathomechanisms and current and emerging treatment strategies. Mol Med. (2011) 17:113–25. doi: 10.2119/molmed.2009.00153
7. Basson, R, and Bayat, A. Skin scarring: latest update on objective assessment and optimal management. Front Med. (2022) 9:942756. doi: 10.3389/fmed.2022.942756
8. Kassi, K, Kouame, K, Kouassi, A, Allou, A, Kouassi, I, Kourouma, S, et al. Quality of life in black African patients with keloid scars. Dermatol Reports. (2020) 12:8312. doi: 10.4081/dr.2020.8312
9. Guest, JF, Fuller, GW, and Vowden, P. Cohort study evaluating the burden of wounds to the UK's National Health Service in 2017/2018: update from 2012/2013. BMJ Open. (2020) 10:e045253. doi: 10.1136/bmjopen-2020-045253
10. Shoulders, MD, and Raines, RT. Collagen structure and stability. Annu Rev Biochem. (2009) 78:929–58. doi: 10.1146/annurev.biochem.77.032207.120833
11. Sorushanova, A, Delgado, LM, Wu, Z, Shologu, N, Kshirsagar, A, Raghunath, R, et al. The collagen Suprafamily: from biosynthesis to advanced biomaterial development. Adv Mater. (2019) 31:e1801651. doi: 10.1002/adma.201801651
12. Holmes, DF, Lu, Y, Starborg, T, and Kadler, KE. Collagen fibril assembly and function. Curr Top Dev Biol. (2018) 130:107–42. doi: 10.1016/bs.ctdb.2018.02.004
13. Davison-Kotler, E, Marshall, WS, and Garcia-Gareta, E. Sources of collagen for biomaterials in skin wound healing. Bioengineering. (2019) 6:56. doi: 10.3390/bioengineering6030056
14. Ala-Kokko, L, Kontusaari, S, Baldwin, CT, Kuivaniemi, H, and Prockop, DJ. Structure of cDNA clones coding for the entire prepro alpha 1 (III) chain of human type III procollagen. Differences in protein structure from type I procollagen and conservation of codon preferences. Biochem J. (1989) 260:509–16. doi: 10.1042/bj2600509
15. Asgari, M, Latifi, N, Heris, HK, Vali, H, and Mongeau, L. In vitro fibrillogenesis of tropocollagen type III in collagen type I affects its relative fibrillar topology and mechanics. Sci Rep. (2017) 7:1392. doi: 10.1038/s41598-017-01476-y
16. Fleischmajer, R, Perlish, JS, Burgeson, RE, Shaikh-Bahai, F, and Timpl, R. Type I and type III collagen interactions during fibrillogenesis. Ann N Y Acad Sci. (1990) 580:161–75. doi: 10.1111/j.1749-6632.1990.tb17927.x
17. Cameron, GJ, Alberts, IL, Laing, JH, and Wess, TJ. Structure of type I and type III heterotypic collagen fibrils: an X-ray diffraction study. J Struct Biol. (2002) 137:15–22. doi: 10.1006/jsbi.2002.4459
18. Knapp, TR, Daniels, RJ, and Kaplan, EN. Pathologic scar formation. Morphologic and biochemical correlates. Am J Pathol. (1977) 86:47–70.
19. Siegel, RC, Pinnell, SR, and Martin, GR. Cross-linking of collagen and elastin. Prop Lysyl Oxid Biochem. (1970) 9:4486–92. doi: 10.1021/bi00825a004
20. Siegel, RC. Biosynthesis of collagen crosslinks: increased activity of purified lysyl oxidase with reconstituted collagen fibrils. Proc Natl Acad Sci USA. (1974) 71:4826–30. doi: 10.1073/pnas.71.12.4826
21. Streeter, I, and de Leeuw, NH. A molecular dynamics study of the interprotein interactions in collagen fibrils. Soft Matter. (2011) 7:3373–82. doi: 10.1039/c0sm01192d
22. Orgel, JP, Wess, TJ, and Miller, A. The in situ conformation and axial location of the intermolecular cross-linked non-helical telopeptides of type I collagen. Structure. (2000) 8:137–42. doi: 10.1016/S0969-2126(00)00089-7
23. Fleischmajer, R, MacDonald, ED, Perlish, JS, Burgeson, RE, and Fisher, LW. Dermal collagen fibrils are hybrids of type I and type III collagen molecules. J Struct Biol. (1990) 105:162–9. doi: 10.1016/1047-8477(90)90110-X
24. Hulmes, DJ. Building collagen molecules, fibrils, and suprafibrillar structures. J Struct Biol. (2002) 137:2–10. doi: 10.1006/jsbi.2002.4450
25. Liu, X, Wu, H, Byrne, M, Krane, S, and Jaenisch, R. Type III collagen is crucial for collagen I fibrillogenesis and for normal cardiovascular development. Proc Natl Acad Sci USA. (1997) 94:1852–6. doi: 10.1073/pnas.94.5.1852
26. Koppenol, DC, Vermolen, FJ, Niessen, FB, van Zuijlen, PPM, and Vuik, K. A biomechanical mathematical model for the collagen bundle distribution-dependent contraction and subsequent retraction of healing dermal wounds. Biomech Model Mechanobiol. (2017) 16:345–61. doi: 10.1007/s10237-016-0821-2
27. Ni Annaidh, A, Bruyere, K, Destrade, M, Gilchrist, MD, Maurini, C, Ottenio, M, et al. Automated estimation of collagen fibre dispersion in the dermis and its contribution to the anisotropic behaviour of skin. Ann Biomed Eng. (2012) 40:1666–78. doi: 10.1007/s10439-012-0542-3
28. Ueda, M, Saito, S, Murata, T, Hirano, T, Bise, R, Kabashima, K, et al. Combined multiphoton imaging and biaxial tissue extension for quantitative analysis of geometric fiber organization in human reticular dermis. Sci Rep. (2019) 9:10644. doi: 10.1038/s41598-019-47213-5
29. Khorasani, H, Zheng, Z, Nguyen, C, Zara, J, Zhang, X, Wang, J, et al. A quantitative approach to scar analysis. Am J Pathol. (2011) 178:621–8. doi: 10.1016/j.ajpath.2010.10.019
30. Kischer, CW. Contributions of electron microscopy to the study of the hypertrophic scar and related lesions. Scanning Microsc. (1993) 7:921–30.
31. Zhou, B, Tu, T, Gao, Z, Wu, X, Wang, W, and Liu, W. Impaired collagen fibril assembly in keloids with enhanced expression of lumican and collagen V. Arch Biochem Biophys. (2021) 697:108676. doi: 10.1016/j.abb.2020.108676
32. Verhaegen, PD, van Zuijlen, PP, Pennings, NM, van Marle, J, Niessen, FB, van der Horst, CM, et al. Differences in collagen architecture between keloid, hypertrophic scar, normotrophic scar, and normal skin: an objective histopathological analysis. Wound Repair Regen. (2009) 17:649–56. doi: 10.1111/j.1524-475X.2009.00533.x
33. Ehrlich, HP, Desmouliere, A, Diegelmann, RF, Cohen, IK, Compton, CC, Garner, WL, et al. Morphological and immunochemical differences between keloid and hypertrophic scar. Am J Pathol. (1994) 145:105–13.
34. Friedman, DW, Boyd, CD, Mackenzie, JW, Norton, P, Olson, RM, and Deak, SB. Regulation of collagen gene expression in keloids and hypertrophic scars. J Surg Res. (1993) 55:214–22. doi: 10.1006/jsre.1993.1132
35. Uitto, J, Perejda, AJ, Abergel, RP, Chu, ML, and Ramirez, F. Altered steady-state ratio of type I/III procollagen mRNAs correlates with selectively increased type I procollagen biosynthesis in cultured keloid fibroblasts. Proc Natl Acad Sci USA. (1985) 82:5935–9. doi: 10.1073/pnas.82.17.5935
36. Uzawa, K, Marshall, MK, Katz, EP, Tanzawa, H, Yeowell, HN, and Yamauchi, M. Altered posttranslational modifications of collagen in keloid. Biochem Biophys Res Commun. (1998) 249:652–5. doi: 10.1006/bbrc.1998.8955
37. Kaku, C, Ichinose, S, Dohi, T, Tosa, M, and Ogawa, R. Keloidal collagen may be produced directly by alphaSMA-positive cells: morphological analysis and protein shotgun analysis. Plast Reconstr Surg Glob Open. (2023) 11:e4897. doi: 10.1097/GOX.0000000000004897
38. Hinz, B, Phan, SH, Thannickal, VJ, Galli, A, Bochaton-Piallat, ML, and Gabbiani, G. The myofibroblast: one function, multiple origins. Am J Pathol. (2007) 170:1807–16. doi: 10.2353/ajpath.2007.070112
39. Akhmetshina, A, Palumbo, K, Dees, C, Bergmann, C, Venalis, P, Zerr, P, et al. Activation of canonical Wnt signalling is required for TGF-beta-mediated fibrosis. Nat Commun. (2012) 3:735. doi: 10.1038/ncomms1734
40. Cohen, AJ, Nikbakht, N, and Uitto, J. Keloid disorder: Genetic basis, gene expression profiles, and immunological modulation of the fibrotic processes in the skin. Cold Spring Harb Perspect Biol. (2023) 15. doi: 10.1101/cshperspect.a041245
41. Hinz, B. Formation and function of the myofibroblast during tissue repair. J Invest Dermatol. (2007) 127:526–37. doi: 10.1038/sj.jid.5700613
42. Desmouliere, A, Redard, M, Darby, I, and Gabbiani, G. Apoptosis mediates the decrease in cellularity during the transition between granulation tissue and scar. Am J Pathol. (1995) 146:56–66.
43. Barrientos, S, Stojadinovic, O, Golinko, MS, Brem, H, and Tomic-Canic, M. Growth factors and cytokines in wound healing. Wound Repair Regen. (2008) 16:585–601. doi: 10.1111/j.1524-475X.2008.00410.x
44. Gabay, C, Lamacchia, C, and Palmer, G. IL-1 pathways in inflammation and human diseases. Nat Rev Rheumatol. (2010) 6:232–41. doi: 10.1038/nrrheum.2010.4
45. Dinarello, CA. Overview of the IL-1 family in innate inflammation and acquired immunity. Immunol Rev. (2018) 281:8–27. doi: 10.1111/imr.12621
46. Sims, JE, Giri, JG, and Dower, SK. The two interleukin-1 receptors play different roles in IL-1 actions. Clin Immunol Immunopathol. (1994) 72:9–14. doi: 10.1006/clin.1994.1100
47. Postlethwaite, AE, Raghow, R, Stricklin, GP, Poppleton, H, Seyer, JM, and Kang, AH. Modulation of fibroblast functions by interleukin 1: increased steady-state accumulation of type I procollagen messenger RNAs and stimulation of other functions but not chemotaxis by human recombinant interleukin 1 alpha and beta. J Cell Biol. (1988) 106:311–8. doi: 10.1083/jcb.106.2.311
48. Kahari, VM, Heino, J, and Vuorio, E. Interleukin-1 increases collagen production and mRNA levels in cultured skin fibroblasts. Biochim Biophys Acta. (1987) 929:142–7. doi: 10.1016/0167-4889(87)90169-8
49. Mauviel, A, Heino, J, Kahari, VM, Hartmann, DJ, Loyau, G, Pujol, JP, et al. Comparative effects of interleukin-1 and tumor necrosis factor-alpha on collagen production and corresponding procollagen mRNA levels in human dermal fibroblasts. J Invest Dermatol. (1991) 96:243–9. doi: 10.1111/1523-1747.ep12462185
50. Zhang, D, Li, B, and Zhao, M. Therapeutic strategies by regulating interleukin family to suppress inflammation in hypertrophic scar and keloid. Front Pharmacol. (2021) 12:667763. doi: 10.3389/fphar.2021.667763
51. Jia, S, Xie, P, Hong, SJ, Galiano, R, Singer, A, Clark, RA, et al. Intravenous curcumin efficacy on healing and scar formation in rabbit ear wounds under nonischemic, ischemic, and ischemia-reperfusion conditions. Wound Repair Regen. (2014) 22:730–9. doi: 10.1111/wrr.12231
52. Li, Y, Zhao, J, Yin, Y, Li, K, Zhang, C, and Zheng, Y. The role of IL-6 in fibrotic diseases: molecular and cellular mechanisms. Int J Biol Sci. (2022) 18:5405–14. doi: 10.7150/ijbs.75876
53. Johnson, BZ, Stevenson, AW, Prele, CM, Fear, MW, and Wood, FM. The role of IL-6 in skin fibrosis and cutaneous wound healing. Biomedicines. (2020) 8:101. doi: 10.3390/biomedicines8050101
54. Rose-John, S. IL-6 trans-signaling via the soluble IL-6 receptor: importance for the pro-inflammatory activities of IL-6. Int J Biol Sci. (2012) 8:1237–47. doi: 10.7150/ijbs.4989
55. Ghazizadeh, M, Tosa, M, Shimizu, H, Hyakusoku, H, and Kawanami, O. Functional implications of the IL-6 signaling pathway in keloid pathogenesis. J Invest Dermatol. (2007) 127:98–105. doi: 10.1038/sj.jid.5700564
56. Moodley, YP, Misso, NL, Scaffidi, AK, Fogel-Petrovic, M, McAnulty, RJ, Laurent, GJ, et al. Inverse effects of interleukin-6 on apoptosis of fibroblasts from pulmonary fibrosis and normal lungs. Am J Respir Cell Mol Biol. (2003) 29:490–8. doi: 10.1165/rcmb.2002-0262OC
57. Niemand, C, Nimmesgern, A, Haan, S, Fischer, P, Schaper, F, Rossaint, R, et al. Activation of STAT3 by IL-6 and IL-10 in primary human macrophages is differentially modulated by suppressor of cytokine signaling 3. J Immunol. (2003) 170:3263–72. doi: 10.4049/jimmunol.170.6.3263
58. Singampalli, KL, Balaji, S, Wang, X, Parikh, UM, Kaul, A, Gilley, J, et al. The role of an IL-10/Hyaluronan Axis in dermal wound healing. Front Cell Dev Biol. (2020) 8:636. doi: 10.3389/fcell.2020.00636
59. Hesketh, M, Sahin, KB, West, ZE, and Murray, RZ. Macrophage phenotypes regulate scar formation and chronic wound healing. Int J Mol Sci. (2017) 18:1545. doi: 10.3390/ijms18071545
60. Amjadian, S, Moradi, S, and Mohammadi, P. The emerging therapeutic targets for scar management: genetic and epigenetic landscapes. Skin Pharmacol Physiol. (2022) 35:247–65. doi: 10.1159/000524990
61. Leventhal, D, Furr, M, and Reiter, D. Treatment of keloids and hypertrophic scars: a meta-analysis and review of the literature. Arch Facial Plast Surg. (2006) 8:362–8. doi: 10.1001/archfaci.8.6.362
62. Hawash, AA, Ingrasci, G, Nouri, K, and Yosipovitch, G. Pruritus in keloid scars: mechanisms and treatments. Acta Derm Venereol. (2021) 101:adv00582. doi: 10.2340/00015555-3923
63. Medina, JL, Sebastian, EA, Fourcaudot, AB, Dorati, R, and Leung, KP. Pirfenidone ointment modulates the burn wound bed in C57BL/6 mice by suppressing inflammatory responses. Inflammation. (2019) 42:45–53. doi: 10.1007/s10753-018-0871-y
64. Morikawa, M, Derynck, R, and Miyazono, K. TGF-beta and the TGF-beta family: Context-dependent roles in cell and tissue physiology. Cold Spring Harb Perspect Biol. (2016) 8. doi: 10.1101/cshperspect.a021873
65. Karppinen, SM, Heljasvaara, R, Gullberg, D, Tasanen, K, and Pihlajaniemi, T. Toward understanding scarless skin wound healing and pathological scarring. F1000Res. (2019) 8:8. doi: 10.12688/f1000research.18293.1
66. Desmouliere, A, Geinoz, A, Gabbiani, F, and Gabbiani, G. Transforming growth factor-beta 1 induces alpha-smooth muscle actin expression in granulation tissue myofibroblasts and in quiescent and growing cultured fibroblasts. J Cell Biol. (1993) 122:103–11. doi: 10.1083/jcb.122.1.103
67. Ronnov-Jessen, L, and Petersen, OW. Induction of alpha-smooth muscle actin by transforming growth factor-beta 1 in quiescent human breast gland fibroblasts. Implications for myofibroblast generation in breast neoplasia. Lab Investig. (1993) 68:696–707.
68. Werner, S, and Grose, R. Regulation of wound healing by growth factors and cytokines. Physiol Rev. (2003) 83:835–70. doi: 10.1152/physrev.2003.83.3.835
69. Sandulache, VC, Parekh, A, Li-Korotky, H, Dohar, JE, and Hebda, PA. Prostaglandin E2 inhibition of keloid fibroblast migration, contraction, and transforming growth factor (TGF)-beta1-induced collagen synthesis. Wound Repair Regen. (2007) 15:122–33. doi: 10.1111/j.1524-475X.2006.00193.x
70. Shah, M, Foreman, DM, and Ferguson, MW. Neutralising antibody to TGF-beta 1,2 reduces cutaneous scarring in adult rodents. J Cell Sci. (1994) 107:1137–57. doi: 10.1242/jcs.107.5.1137
71. Chang, Z, Kishimoto, Y, Hasan, A, and Welham, NV. TGF-beta3 modulates the inflammatory environment and reduces scar formation following vocal fold mucosal injury in rats. Dis Model Mech. (2014) 7:83–91. doi: 10.1242/dmm.013326
72. Viera, MH, Amini, S, Valins, W, and Berman, B. Innovative therapies in the treatment of keloids and hypertrophic scars. J Clin Aesthet Dermatol. (2010) 3:20–6.
73. Zgheib, C, Xu, J, and Liechty, KW. Targeting inflammatory cytokines and extracellular matrix composition to promote wound regeneration. Adv Wound Care. (2014) 3:344–55. doi: 10.1089/wound.2013.0456
74. Shi, J, Li, J, Guan, H, Cai, W, Bai, X, Fang, X, et al. Anti-fibrotic actions of interleukin-10 against hypertrophic scarring by activation of PI3K/AKT and STAT3 signaling pathways in scar-forming fibroblasts. PLoS One. (2014) 9:e98228. doi: 10.1371/journal.pone.0098228
75. Peranteau, WH, Zhang, L, Muvarak, N, Badillo, AT, Radu, A, Zoltick, PW, et al. IL-10 overexpression decreases inflammatory mediators and promotes regenerative healing in an adult model of scar formation. J Invest Dermatol. (2008) 128:1852–60. doi: 10.1038/sj.jid.5701232
76. Shi, J, Shi, S, Xie, W, Zhao, M, Li, Y, Zhang, J, et al. IL-10 alleviates lipopolysaccharide-induced skin scarring via IL-10R/STAT3 axis regulating TLR4/NF-kappaB pathway in dermal fibroblasts. J Cell Mol Med. (2021) 25:1554–67. doi: 10.1111/jcmm.16250
77. Wangoo, A, Laban, C, Cook, HT, Glenville, B, and Shaw, RJ. Interleukin-10- and corticosteroid-induced reduction in type I procollagen in a human ex vivo scar culture. Int J Exp Pathol. (1997) 78:33–41. doi: 10.1046/j.1365-2613.1997.d01-241.x
78. Broder, C, Arnold, P, Vadon-Le Goff, S, Konerding, MA, Bahr, K, Muller, S, et al. Metalloproteases meprin alpha and meprin beta are C- and N-procollagen proteinases important for collagen assembly and tensile strength. Proc Natl Acad Sci USA. (2013) 110:14219–24. doi: 10.1073/pnas.1305464110
79. Tanzawa, K, Berger, J, and Prockop, DJ. Type I procollagen N-proteinase from whole chick embryos. Cleavage of a homotrimer of pro-alpha 1(I) chains and the requirement for procollagen with a triple-helical conformation. J Biol Chem. (1985) 260:1120–6. doi: 10.1016/S0021-9258(20)71216-0
80. Fomovsky, GM, Macadangdang, JR, Ailawadi, G, and Holmes, JW. Model-based design of mechanical therapies for myocardial infarction. J Cardiovasc Transl Res. (2011) 4:82–91. doi: 10.1007/s12265-010-9241-3
81. Richardson, WJ, and Holmes, JW. Emergence of collagen orientation heterogeneity in healing infarcts and an agent-based model. Biophys J. (2016) 110:2266–77. doi: 10.1016/j.bpj.2016.04.014
82. Mia, MM, and Boersema, MBank RA. Interleukin-1beta attenuates myofibroblast formation and extracellular matrix production in dermal and lung fibroblasts exposed to transforming growth factor-beta1. PLoS One. (2014) 9:e91559. doi: 10.1371/journal.pone.0091559
83. Deon, D, Ahmed, S, Tai, K, Scaletta, N, Herrero, C, Lee, IH, et al. Cross-talk between IL-1 and IL-6 signaling pathways in rheumatoid arthritis synovial fibroblasts. J Immunol. (2001) 167:5395–403. doi: 10.4049/jimmunol.167.9.5395
Keywords: collagen, structure and assembly, cytokine, inflammation, scar
Citation: Zhou CJ and Guo Y (2024) Mini review on collagens in normal skin and pathological scars: current understanding and future perspective. Front. Med. 11:1449597. doi: 10.3389/fmed.2024.1449597
Received: 15 June 2024; Accepted: 05 July 2024;
Published: 18 July 2024.
Edited by:
Stefano Bacci, University of Florence, ItalyReviewed by:
Rei Ogawa, Nippon Medical School, JapanCopyright © 2024 Zhou and Guo. This is an open-access article distributed under the terms of the Creative Commons Attribution License (CC BY). The use, distribution or reproduction in other forums is permitted, provided the original author(s) and the copyright owner(s) are credited and that the original publication in this journal is cited, in accordance with accepted academic practice. No use, distribution or reproduction is permitted which does not comply with these terms.
*Correspondence: Yuan Guo, eS5ndW9AbGVlZHMuYWMudWs=
Disclaimer: All claims expressed in this article are solely those of the authors and do not necessarily represent those of their affiliated organizations, or those of the publisher, the editors and the reviewers. Any product that may be evaluated in this article or claim that may be made by its manufacturer is not guaranteed or endorsed by the publisher.
Research integrity at Frontiers
Learn more about the work of our research integrity team to safeguard the quality of each article we publish.