- Department of Rheumatology and Clinical Immunology, Clinic of Internal Medicine III, University Hospital of Bonn, Bonn, Germany
Vascular adhesion protein-1 (VAP-1) is a type 2 transmembrane sialoglycoprotein with oxidative deamination functionality, encoded by the amine oxidase copper-containing 3 (AOC3) gene. VAP-1 is widely expressed across various tissues, particularly in highly vascularized tissues and organs essential for lymphocyte circulation. In the vascular system, VAP-1 is predominantly found in vascular smooth muscle cells and endothelial cells, with higher expression levels in vascular smooth muscle cells. Under inflammatory conditions, VAP-1 rapidly translocates to the endothelial cell surface, facilitating leukocyte adhesion and migration through interactions with specific ligands, such as sialic acid-binding immunoglobulin-type lectins (Siglec)-9 on neutrophils and monocytes, and Siglec-10 on B cells, monocytes, and eosinophils. This interaction is crucial for leukocyte transmigration into inflamed tissues. Furthermore, VAP-1’s enzymatic activity generates hydrogen peroxide and advanced glycation end-products, contributing to cytotoxic damage and vascular inflammation. In this context, the soluble form of VAP-1 (sVAP-1), produced by matrix metalloproteinase cleavage from its membrane-bound counterpart, also significantly influences leukocyte migration. This review aims to elucidate the multifaceted pathophysiological roles of VAP-1 in vascular inflammation, particularly in giant cell arteritis (GCA) and associated polymyalgia rheumatica (PMR). By exploring its involvement in immune cell adhesion, migration, and its enzymatic contributions to oxidative stress and tissue damage, we investigate the importance of VAP-1 in GCA. Additionally, we discuss recent advancements in imaging techniques targeting VAP-1, such as [68Ga]Ga-DOTA-Siglec-9 PET/CT, which have provided new insights into VAP-1’s role in GCA and PMR. Overall, understanding VAP-1’s comprehensive roles could pave the way for improved strategies in managing these conditions.
Introduction
Giant cell arteritis (GCA) is an immune-mediated vasculitis that affects large and medium-sized vessels, predominantly in individuals over 50 years of age. It is the most prevalent form of vasculitis in Western populations. GCA can lead to vascular changes and occlusion due to severe vascular inflammation, neoangiogenesis, and remodeling. Additionally, GCA is closely associated with polymyalgia rheumatica (PMR), which is characterized by inflammation in periarticular structures. PMR may precede, coincide with, or follow the onset of GCA. Thus, subclinical GCA can be detected in 22–23% of PMR patients (1, 2). However, in some studies, the incidence of large vessel vasculitis detected by positron emission tomography–computed tomography (PET/CT) in patients with PMR can reach up to 60%, particularly in those presenting with inflammatory low back pain, pelvic girdle pain, and diffuse lower limb pain (3, 4).
Despite advances in understanding the pathophysiology of GCA, the innate and adaptive immune mechanisms involved remain only partially understood. Initial hypotheses primarily attributed the immune response in GCA to TH1 cells, driven by the activation of the Janus kinase (JAK) and Signal Transducers and Activators of Transcription (STAT) signaling pathways (5). It has been demonstrated that IFN-γ plays a significant role in mediating chemotaxis through CXCL9, CXCL10, and CXCL11 in the arterial wall of GCA patients via the JAK-STAT1 pathway (6, 7). Moreover, functional polymorphisms of IFN-γ were associated with the development of severe ischemic complications of the disease (8). Recent findings, however, suggest that cytokines beyond the STAT signaling pathway may also significantly influence inflammation in GCA (5). Various mechanisms involving both TH1 and TH17 cells have been recognized, including the recruitment of T-cells within the vascular wall facilitated by vascular dendritic cells (9). These cells are responsible not only for chemotaxis and cytokine release but also for the differentiation of TH1/TH17 cells via vasculitogenic T-effector cells (9). A chemokine-mediated link involving IFN-γ, Interleukin (IL)-17, and IL-21 fosters an inflammatory environment (10, 11). Monocytes also significantly contribute to the differentiation of TH1 and TH17 cells via the production of cytokines such as IL-12p35 (promoting TH1) and IL-1β, IL-6, and IL-23p19 (promoting TH17) (12). Activated TH1/TH17 cells not only sustain the initial immune response by producing key cytokines (IFN-γ/IL-17) but also exacerbate inflammation by recruiting cytotoxic CD8 cells and monocytic precursor cells, which evolve into macrophages leading to vascular damage and remodeling (9).
The close connection between PMR and GCA has led to joint investigations into their disease mechanisms. In PMR, similar to GCA, Treg, TH1, and TH17-associated inflammatory processes, along with their key cytokines, are crucial (13–15). Moreover, IL-6, along with IL-1 and ICAM-1, is significantly implicated in the pathophysiology of PMR, influencing the likelihood of future relapses in patients (14–17). While ICAM-1 polymorphisms alone do not appear to be associated with disease severity in isolated PMR, the presence of homozygosity for both the HLA-DRB1*0401 allele and the 241 GG codon of ICAM-1 is significantly correlated with an increased risk of relapses in these patients (18). A major distinction in the immune response between PMR and GCA is the absence of a strong IFN-γ response in PMR (19).
While the understanding of immunological and pathophysiological aspects of GCA and PMR is evolving, significant gaps remain, particularly in linking immunological processes with disease manifestations. This emphasizes the need for a better understanding of these diseases.
Structure and function of vascular-adhesion protein 1
Vascular adhesion protein-1 (VAP-1) is a type 2 transmembrane sialoglycoprotein, encoded by the amine oxidase copper-containing 3 (AOC3) gene. It forms a 180 kDa homodimer consisting of three distinct domains (D2-D4), capable of catalyzing oxidative deamination reactions (20–24). This enzymatic activity can be inhibited by semicarbazide, classifying VAP-1 within the semicarbazide-sensitive amine oxidase (SSAO) family (25). To clearly differentiate VAP-1-like SSAOs (topaquinone-containing amine oxidases) from other members of the SSAO family, they have been renamed as primary amine oxidases (26). Other SSAOs belong to the lysyl oxidase family, characterized by the presence of lysine tyrosyl quinone, rather than topaquinone, at their catalytic sites (26).
VAP-1 is expressed by various cell types, including vascular cells, pericytes on the outer surfaces of blood vessels, adipocytes, chondrocytes, follicular dendritic cells, and liver cells (26–30). Its expression is particularly prominent in tissues with high vascularization, such as blood vessels, muscle, cerebrovascular tissue, heart, liver, kidney, retina, intestine, lung, and adipose tissue. Moreover, VAP-1 is significantly expressed in organs involved in lymphocyte recirculation and homing, including the vessels of the spleen, thymic cortex, and lymph nodes (31–34).
In the vascular system, the expression of VAP-1 is predominantly observed in vascular smooth muscle cells (VSMC) and endothelial cells. VSMC, located in the medial layer of the vascular wall, exhibit higher expression and activity levels of VAP-1 compared to endothelial cells (26, 35, 36). VSMC are pivotal in producing the extracellular matrix, which is essential for the arterial wall’s resilience against blood circulation pressure and exhibit significant plasticity (37, 38). Under external stimuli, VSMC can migrate and proliferate from the medial to the intimal layer, contributing to intimal hyperplasia (38). VAP-1 in VSMC is specifically localized within the caveolae of the plasma membrane (39), yet the regulation of VAP-1 in these cells, as well as its physiological functions within them, remains less understood (26). Although VAP-1 in VSMC does not facilitate lymphocyte binding in vitro (39), it is implicated in critical processes such as vascular tone regulation, cell differentiation, and extracellular matrix organization (22, 40, 41). An increase in VAP-1 activity can generate reactive oxygen species, leading to VSMC death and potentially contributing to atherosclerosis (37, 38, 42).
Conversely, VAP-1 is present in all three types of endothelial cells, continuous, fenestrated, and sinusoidal (34). Its role varies across different tissue types and (patho-)physiological conditions. In specific endothelial cells, like liver sinusoidal endothelium and the specialized high endothelial venules in peripheral lymph nodes, VAP-1 is constitutively expressed on the cell surface (43, 44). In all other endothelial cells, VAP-1 resides within intracellular vesicles, absent from the cell surface. However, during inflammatory conditions, stimuli such as tumor necrosis factor-alpha, interferon-gamma, lipopolysaccharide, and interleukin-1ß trigger its rapid relocation to the surface of endothelial cells (26, 45–47) (Figure 1).
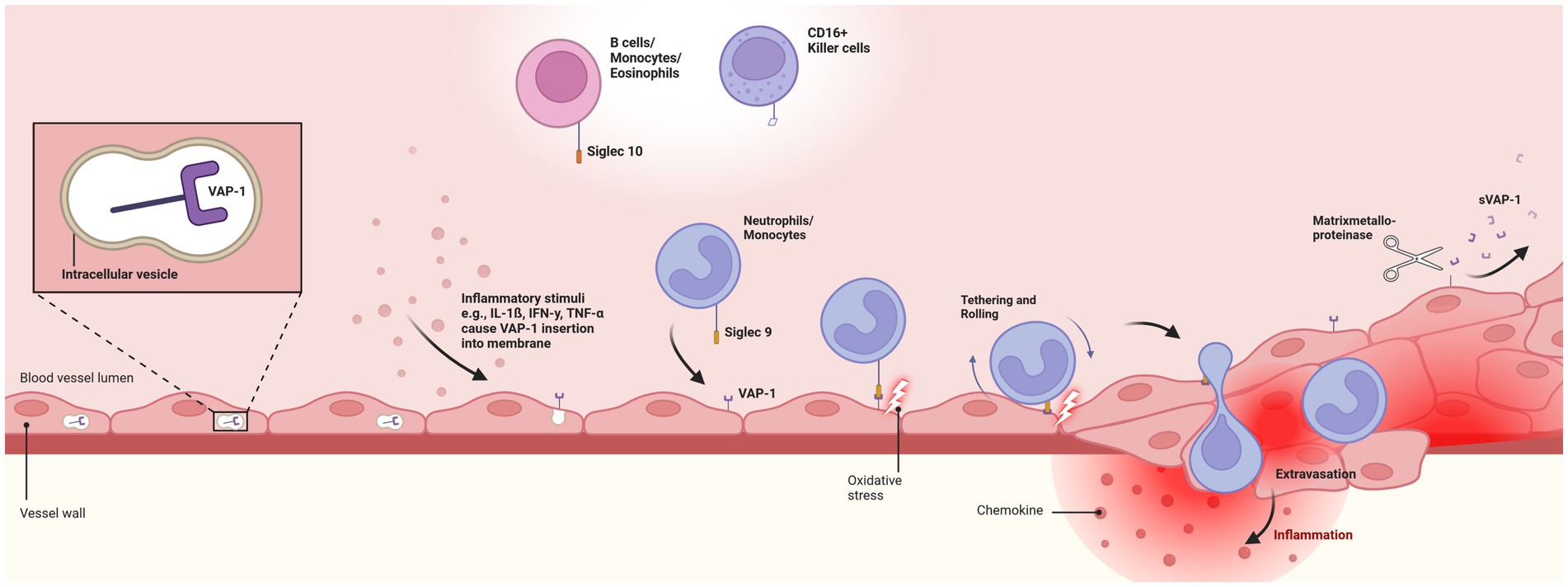
Figure 1. Pathophysiologal role of Vascular-adhesion protein 1. Depicts the endothelial translocation of membrane-bound vascular-adhesion protein 1 (VAP-1) from intracellular vesicles to the cell surface in response to inflammatory stimuli. Translocation facilitates the interaction of VAP-1 with circulating neutrophils and monocytes through the Siglec-9 ligand. This initiates oxidative deamination, leading to cytotoxic damage to endothelial cells and promoting an inflammatory response. Secretion of chemokines, activation of transcription factors, and expression of matrix metalloproteinases enhance leukocyte rolling, tethering, and migration. Finally, soluble VAP-1 (sVAP-1) is generated through the cleavage of membrane-bound VAP-1 by matrix metalloproteinases, releasing it into the circulation and significantly contributing to the monoamine oxidase activity in human blood. VAP-1, vascular-adhesion protein 1; sVAP-1, soluble vascular-adhesion protein 1. Created with BioRender.com.
Pathophysiological role of vascular-adhesion protein 1
VAP-1 plays a critical role in immune cell adhesion and migration, particularly facilitating the transmigration of leukocytes from the bloodstream into inflamed tissues. This process involves VAP-1’s dual function: its enzymatic activity catalyzes the oxidative deamination of primary amines (48), a key mechanism behind most of VAP-1’s pathophysiological effects, and its role as a membrane-bound endothelial adhesion molecule that supports enzyme-independent leukocyte binding (31). Under inflammatory conditions, VAP-1 therefore acts as an ectoenzyme with a catalytically active domain external to the cell membrane, amplifying its role in immune responses (38).
On endothelial cells, VAP-1 engages with leukocytes via specific ligands, including sialic acid-binding immunoglobulin-type lectins (Siglec)-9, predominantly found on neutrophils and monocytes, and Siglec-10, identified on B cells, monocytes, and eosinophils (48–51). Siglec-10 further acts as a substrate for VAP-1, a function not demonstrated for Siglec-9. Additionally, VAP-1 engages with CD16+ natural killer cells, although the precise mechanism of this interaction is not well understood (26, 45, 50–53).
Inflammatory stimuli lead to the rapid up-regulation of Siglec-9 and Siglec-10 on leukocytes, enhancing their interaction with endothelial VAP-1 (54, 55). This interaction fosters a transient adhesive bond, where primary amines on leukocytes serve as substrates for oxidative deamination by VAP-1’s catalytic site (50, 56). This two-step oxidative deamination process, converting methylamine to formaldehyde and aminoacetone to methylglyoxal, with subsequent production of hydrogen peroxide and ammonia contributing to advanced glycation end-products (AGE) formation and increased oxidative stress, inflicts cytotoxic damage on endothelial cells (56–58). This can result in vascular damage and potential vascular complications like atherosclerosis (26, 29, 31, 57, 59–61).
The generation of VAP-1-derived hydrogen peroxide, a powerful signaling molecule at low concentrations, plays an essential role in local inflammatory responses (26). The catalytic activity of VAP-1 induces the expression of various endothelial adhesion molecules, such as ICAM-1, MadCAM-1, E-selectin, and P-selectin, and promotes the secretion of the chemokine CXCL8 (44, 62–65). It also activates key transcription factors, facilitating the engagement of multiple signaling pathways, including PI3K, MAPK, and NF-κB, thereby fostering an inflammatory milieu beneficial to leukocyte extravasation (24, 56). This complex process encompasses leukocyte tethering and rolling along the endothelium, culminating in the extravasation cascade, essential for immune cell migration to sites of inflammation (24, 26, 27, 56, 66–68). Real-time imaging studies have underscored VAP-1’s facilitation of leukocyte slow rolling, firm adhesion, and subsequent migration within blood vessels, particularly at lymphoid tissues and inflamed sites (69). Notably, the interaction of various immune cells, including CD4+ helper T cells, T-regulatory cells, Th17 cells, CD8+ cytotoxic T cells, B lymphocytes, CD16+ monocytes, and granulocytes with high endothelial venules and flat-walled vessels, has been shown to be modulated, at least in part, by VAP-1 expression levels (34, 43, 52, 68, 70–76). This underscores VAP-1’s vital role in mediating immune surveillance and response, highlighting its importance in the immune system’s functionality.
While membrane-bound VAP-1 serves as a transmembrane glycoprotein within the vascular wall, soluble VAP-1 (sVAP-1) arises from the proteolytic cleavage of its membrane-bound form by matrix metalloproteinases, releasing it into circulation (26, 27, 59, 69). This allows VAP-1 to influence leukocyte migration in both transmembrane and soluble form, with sVAP-1 contributing significantly to the circulating monoamine oxidase activity in human blood (77). High concentrations of sVAP-1, often originating from high endothelial venules in lymphatic organs, play a crucial role in facilitating transendothelial migration of lymphocytes (78–80). In healthy individuals, sVAP-1 levels in the serum are typically low and stable, modulating the adhesive activity of its membrane-bound counterpart and enhancing leukocyte adhesion (26, 31, 79, 80).
Increased sVAP-1 expression is prevalent in various chronic inflammatory conditions (26, 81) with notable elevations in patients with type 1 diabetes and chronic liver diseases (80), as well as those suffering from skin inflammation (psoriasis), synovitis, active relapsing–remitting multiple sclerosis (RR-MS), and systemic lupus erythematosus (47, 79, 82–85). Furthermore, elevated serum VAP-1 activity is linked to vascular disorders including diabetes mellitus complications (86, 87), hypertension (56), congestive heart failure (88), multiple cerebral infarctions (89), Alzheimer’s disease (90), and atherosclerosis (91), where sVAP-1 levels correlate with intima-media thickness and the presence of carotid plaques (92, 93). Additionally, s VAP-1 concentrations can predict major adverse cardiovascular events and mortality (93, 94). These sVAP-1 increases are often associated with tissue-bound VAP-1 overexpression (60, 95). However, despite these associations, sVAP-1 can not be considered as a general inflammation marker due to the lack of consistent correlation with C-reactive protein levels (93).
The increasing recognition of VAP-1’s role in inflammation and its involvement in exacerbating local lesion formation has led to a growing body of research exploring its role across a spectrum of inflammatory conditions.
Vascular-adhesion protein 1 in large vessel vasculitis and polymyalgia rheumatica
VAP-1’s pathophysiological role, along with the potential inflammatory and oxidative stress-inducing effects of its catalytic products, indicate its involvement in the pathogenesis of vascular inflammatory disorders. The specific localization of VAP-1 on the surface of blood vessel cells further corroborates its involvement in these diseases. This has prompted further investigations using imaging techniques targeting VAP-1 or its ligands. Recent advancements include the introduction of a novel inflammation-specific radiotracer, [68Ga]Ga-DOTA-Siglec-9, for evaluating inflammatory vascular diseases (50).
A recently presented studies demonstrated that [68Ga]Ga-DOTA-Siglec-9 PET/CT can detect vascular inflammation during relapses in GCA, revealing increased localized tracer uptake in regions such as the aorta and subclavian arteries (96, 97). Additionally, prednisolone treatment significantly influenced endothelial VAP-1 expression, suggesting a rapid, therapy-induced reduction of VAP-1. No significant association was found between C-reactive protein levels and tracer uptake, aligning with previous research in other diseases where no correlation could be found (93). Beyond its role as an endothelial adhesion molecule, elevated sVAP-1 has emerged as a potential biomarker for disease activity in GCA, with levels exceeding those in healthy controls (96). However, further studies are needed to confirm this finding. Comparably, in PMR, which is frequently associated with GCA, [68Ga]Ga-DOTA-Siglec-9 PET/CT has indicated involvement of VAP-1 (98). In a cohort of PMR patients, increased tracer uptake was observed in the shoulder and pelvic girdle regions, with a significant negative correlation between prednisolone intake and tracer uptake in the shoulder, further supporting the hypothesis that VAP-1 is rapidly eliminated following prednisolone exposure.
Currently, the precise mechanism of VAP-1 involvement in the pathogenesis and pathophysiology of GCA and PMR remains at least partly speculative. However, its role has been elucidated in other (autoimmune) diseases with vascular inflammation, suggesting potential parallels with the pathophysiology of GCA and PMR.
In granulomatosis with polyangiitis, VAP-1 is strongly expressed in the renal endothelium during active disease, indicating its potential role in glomerular endothelial cell injury and altered barrier function, thereby contributing to disease pathogenesis (99, 100).
Similarly, in neuronal in vitro endothelial cell models of the blood–brain barrier, a link has been identified between VAP-1 expression and endothelial cell activation. This relationship involves the altered release of pro-inflammatory and pro-angiogenic cytokines, along with subsequent activation of signaling cascades, that also have been shown to significantly contribute to pathogenesis of GCA. Thus, it has been shown that cells expressing human VAP-1 overproduce various cytokines related to inflammation in GCA [e.g., IL-6 (101, 102), IL-8 (103, 104), ICAM (102), VCAM (105, 106)] and trophic factors [e.g. VEGF (10, 107), NGF (108)] (109). The signaling pathways of VEGF and IL-8 are particularly implicated in activating the VEGFR2 molecular pathway, leading to increased endothelial permeability (109). Moreover, VEGF and VAP-1 (110) can be upregulated in response to hypoxia, suggesting that polymorphisms affecting VEGF may also impact processes involving VAP-1. These polymorphisms could potentially affect VAP-1 levels or activity, thereby modulating the extent and nature of inflammatory responses and the development of severe ischemic complications in GCA. In this context, VEGF-induced angiogenesis may contribute to GCA associated inflammation (111).
IL-6 signaling has also been explored as a potential driver of VAP-1-associated endothelial alterations in the blood–brain barrier model, with the STAT3 pathway, which is known to significantly contribute to the pathogenesis of GCA (5, 102), being notably more activated in endothelial cells expressing VAP-1. The significance of the IL-6-activated STAT3 pathway in these alterations was further demonstrated by the application of an IL-6 blocking antibody, which negated the permeability changes induced by VAP-1 conditioned media in wild-type cells (109). This may help explain the successful application of the Interleukin 6 receptor inhibitor Tocilizumab (112).
VAP-1 may also be a potential explanation for the successful treatment of GCA with methotrexate, which has shown efficacy in GCA (113) and PMR (114) and is currently under investigation for remission maintenance therapy in GCA (115). Studies in tumor necrosis factor-α-treated human umbilical vein endothelial cell lines have shown that methotrexate can downregulate pro-inflammatory genes, including VAP-1, highlighting endothelium-protective and anti-inflammatory effects of methotrexat (116).
Furthermore, VAP-1 has been recognized as significant in cerebral ischemic processes, offering a potential explanation for GCA-associated ischemic complications. In animal models with intracerebral hemorrhage-induced brain damage, VAP-1 inhibition downregulated the adhesion molecule ICAM-1 and diminished the infiltration of systemic immune cells, particularly neutrophils, to the injury site (117). This reduction in immune cell accumulation was accompanied by decreased pro-inflammatory cytokines, including TNF-α and MCP-1, and reduced activation of microglia/macrophages. Consequently, inhibiting VAP-1 curtailed the local inflammatory process, potentially reducing cerebral edema and enhancing neurobehavioral functions (117). Finally, in diabetic vascular complications, enhanced interactions between endothelial cells and lymphocytes mediated by VAP-1 (118) and the subsequent transmigration and recruitment of endothelial inflammatory mediators, are central to the activation and progression of various inflammatory pathways (31). Formaldehyde, methylglyoxal, and advanced glycation end-products may contribute to these complications in diabetes (63, 119).
In conclusion, VAP-1 has emerged as a pivotal factor in the pathogenesis and pathophysiology of various vascular inflammatory disorders, including GCA and PMR. The introduction of [68Ga]Ga-DOTA-Siglec-9 PET/CT has provided valuable insights, demonstrating VAP-1’s role in detecting vascular inflammation during GCA relapses and PMR diagnosis, while also highlighting the significant influence of prednisolone treatment on VAP-1 expression. Elevated VAP-1 levels further underscore its potential as a biomarker for disease activity in GCA, although additional studies are necessary to confirm these findings. Overall, the new understanding of VAP-1’s role in GCA and PMR underscores the necessity for continued research to further elucidate its mechanisms, paving the way for improved disease management of these conditions.
Author contributions
SP: Conceptualization, Investigation, Methodology, Project administration, Validation, Visualization, Writing – original draft, Writing – review & editing. C-JB: Methodology, Validation, Visualization, Writing – original draft, Writing – review & editing. VS: Conceptualization, Methodology, Project administration, Supervision, Validation, Writing – original draft, Writing – review & editing.
Funding
The author(s) declare that no financial support was received for the research, authorship, and/or publication of this article.
Conflict of interest
The authors declare that the research was conducted in the absence of any commercial or financial relationships that could be construed as a potential conflict of interest.
The author(s) declared that they were an editorial board member of Frontiers, at the time of submission. This had no impact on the peer review process and the final decision.
Publisher’s note
All claims expressed in this article are solely those of the authors and do not necessarily represent those of their affiliated organizations, or those of the publisher, the editors and the reviewers. Any product that may be evaluated in this article, or claim that may be made by its manufacturer, is not guaranteed or endorsed by the publisher.
References
1. Miguel, ED, Macchioni, P, Conticini, E, Campochiaro, C, Karalilova, R, Monti, S, et al. Prevalence and characteristics of subclinical Giant cell arteritis in polymyalgia Rheumatica. Rheumatology (Oxford). (2024) 63:158–64. doi: 10.1093/Rheumatology/Kead189
2. Burg, LC, Karakostas, P, Behning, C, Brossart, P, Kermani, TA, and Schäfer, VS. Prevalence and characteristics of Giant cell arteritis in patients with newly diagnosed polymyalgia Rheumatica - a prospective cohort study. Ther Adv Musculoskelet Dis. (2023) 15:1759720x221149963. doi: 10.1177/1759720x221149963
3. Prieto-Peña, D, Martínez-Rodríguez, I, Loricera, J, Banzo, I, Calderón-Goercke, M, Calvo-Río, V, et al. Predictors of positive 18f-Fdg pet/Ct-scan for large vessel Vasculitis in patients with persistent polymyalgia Rheumatica. Semin Arthritis Rheum. (2019) 48:720–7. doi: 10.1016/J.Semarthrit.2018.05.007
4. González-Gay, MA, Vicente-Rabaneda, EF, Heras-Recuero, E, and Castañeda, S. Polymyalgia Rheumatica: when should we suspect an underlying large vessel Vasculitis? Clin Exp Rheumatol. (2023) 41:774–6. doi: 10.55563/Clinexprheumatol/3bozph
5. Schäfer, VS, Brossart, P, Warrington, KJ, Kurts, C, Sendtner Gw,, and Aden, CA. The role of autoimmunity and autoinflammation in Giant cell arteritis: a systematic literature review. Autoimmun Rev. (2023) 22:103328. doi: 10.1016/J.Autrev.2023.103328
6. Corbera-Bellalta, M, Planas-Rigol, E, Lozano, E, Terrades-García, N, Alba, M, Prieto-González, S, et al. Blocking interferon Γ reduces expression of chemokines Cxcl9, Cxcl10 and Cxcl11 and decreases macrophage infiltration in ex vivo cultured arteries from patients with Giant cell arteritis. Ann Rheum Dis. (2016) 75:1177–86. doi: 10.1136/Annrheumdis-2015-208371
7. Zhang, H, Watanabe, R, Berry, GJ, Tian, L, Goronzy, JJ, and Weyand, CM. Inhibition of Jak-Stat signaling suppresses pathogenic immune responses in medium and large vessel Vasculitis. Circulation. (2018) 137:1934–48. doi: 10.1161/Circulationaha.117.030423
8. Gonzalez-Gay MA,, Hajeer, AH, Dababneh, A, Garcia-Porrua, C, Amoli, MM, Llorca, J, et al. Interferon-gamma gene microsatellite polymorphisms in patients with biopsy-proven Giant cell arteritis and isolated polymyalgia Rheumatica. Clin Exp Rheumatol. (2004) 22:S18–20.
9. Hid Cadena, R, Reitsema, RD, Huitema, MG, Van Sleen, Y, Van Der Geest, KS, Heeringa, P, et al. Decreased expression of negative immune checkpoint vista by Cd4+ T cells facilitates T helper 1, T helper 17, and T follicular helper lineage differentiation in Gca. Front Immunol. (2019) 10:1638. doi: 10.3389/Fimmu.2019.01638
10. Wen, Z, Shen, Y, Berry, G, Shahram, F, Li, Y, Watanabe, R, et al. The microvascular niche instructs T cells in large vessel Vasculitis via the Vegf-Jagged1-notch pathway. Sci Transl Med. (2017) 9:9. doi: 10.1126/Scitranslmed.Aal3322
11. Mihara, M, Hashizume, M, Yoshida, H, Suzuki, M, and Shiina, M. Il-6/Il-6 receptor system and its role in physiological and pathological conditions. Clin Sci (Lond). (2012) 122:143–59. doi: 10.1042/Cs20110340
12. Deng, J, Younge, BR, Olshen, RA, Goronzy, JJ, and Weyand, CM. Th17 and Th1 T-cell responses in Giant cell arteritis. Circulation. (2010) 121:906–15. doi: 10.1161/Circulationaha.109.872903
13. Hysa, E, Gotelli, E, Sammorì, S, Cimmino, MA, Paolino, S, Pizzorni, C, et al. Immune system activation in polymyalgia Rheumatica: which balance between autoinflammation and autoimmunity? A systematic review. Autoimmun Rev. (2022) 21:102995. doi: 10.1016/J.Autrev.2021.102995
14. Carvajal Alegria, G, Boukhlal, S, Cornec, D, and Devauchelle-Pensec, V. The pathophysiology of polymyalgia Rheumatica, small pieces of a big puzzle. Autoimmun Rev. (2020) 19:102670. doi: 10.1016/J.Autrev.2020.102670
15. Florescu, MM, Bobircă, F, Florescu, A, Pădureanu, V, Bobircă, A, Ciurea, PL, et al. Polymyalgia Rheumatica: an update (review). Exp Ther Med. (2023) 26:543. doi: 10.3892/Etm.2023.12242
16. Samson, M, Audia, S, Fraszczak, J, Trad, M, Ornetti, P, Lakomy, D, et al. Th1 and Th17 lymphocytes expressing Cd161 are implicated in Giant cell arteritis and polymyalgia Rheumatica pathogenesis. Arthritis Rheum. (2012) 64:3788–98. doi: 10.1002/Art.34647
17. Pulsatelli, L, Boiardi, L, Pignotti, E, Dolzani, P, Silvestri, T, Macchioni, P, et al. Serum Interleukin-6 receptor in polymyalgia Rheumatica: a potential marker of relapse/recurrence risk. Arthritis Rheum. (2008) 59:1147–54. doi: 10.1002/Art.23924
18. Amoli, MM, Shelley, E, Mattey, DL, Garcia-Porrua, C, Thomson, W, Hajeer, AH, et al. Intercellular adhesion Molecule-1 gene polymorphisms in isolated polymyalgia Rheumatica. J Rheumatol. (2002) 29:502–4.
19. Weyand, CM, Hicok, KC, Hunder, GG, and Goronzy, JJ. Tissue cytokine patterns in patients with polymyalgia Rheumatica and Giant cell arteritis. Ann Intern Med. (1994) 121:484–91. doi: 10.7326/0003-4819-121-7-199410010-00003
20. Valente, T, Solé, M, and Unzeta, M. Ssao/Vap-1 protein expression during mouse embryonic development. Dev Dyn. (2008) 237:2585–93. doi: 10.1002/Dvdy.21682
21. Jalkanen, S, and Salmi, M. Cell surface monoamine oxidases: enzymes in search of a function. EMBO J. (2001) 20:3893–901. doi: 10.1093/Emboj/20.15.3893
22. Mercier, N. The role of “Semicarbazide-sensitive amine oxidase” in the Arterial Wall. Artres. (2009) 3:141. doi: 10.1016/J.Artres.2009.10.002
23. Elo, P, Tadayon, S, Liljenbäck, H, Teuho, J, Käkelä, M, Koskensalo, K, et al. Vascular adhesion Protein-1 is actively involved in the development of inflammatory lesions in rat models of multiple sclerosis. J Neuroinflammation. (2018) 15:128. doi: 10.1186/S12974-018-1152-2
24. Salmi, M, and Jalkanen, S. Cell-surface enzymes in control of leukocyte trafficking. Nat Rev Immunol. (2005) 5:760–71. doi: 10.1038/Nri1705
25. Kuo, C-H, Wei, J-N, Yang, C-Y, Ou, H-Y, Wu, H-T, Fan, K-C, et al. Serum vascular adhesion Protein-1 is up-regulated in hyperglycemia and is associated with incident diabetes negatively. Int J Obes. (2019) 43:512–22. doi: 10.1038/S41366-018-0172-4
26. Salmi, M, and Jalkanen, S. Vascular adhesion Protein-1: a cell surface amine oxidase in translation. Antioxid Redox Signal. (2019) 30:314–32. doi: 10.1089/Ars.2017.7418
27. Danielli, M, Thomas, RC, Quinn, LM, and Tan, BK. Vascular adhesion Protein-1 (Vap-1) in vascular inflammatory diseases. Vasa. (2022) 51:341–50. doi: 10.1024/0301-1526/A001031
28. Unzeta, M, Hernàndez-Guillamon, M, Sun, P, and Solé, M. Ssao/Vap-1 in cerebrovascular disorders: a potential therapeutic target for stroke and Alzheimer's disease. Int J Mol Sci. (2021) 22:22. doi: 10.3390/Ijms22073365
29. Foot, JS, Buson, A, Deodhar, M, Findlay, AD, Robertson, AD, Turner, CI, et al. Combining monoamine oxidase B and Semicarbazide-sensitive amine oxidase enzyme inhibition to address inflammatory disease. Bioorg Med Chem Lett. (2022) 74:128942. doi: 10.1016/J.Bmcl.2022.128942
30. Gharanei, S, Fishwick, K, Peter Durairaj, R, Jin, T, Siamantouras, E, Liu, K-K, et al. Vascular adhesion Protein-1 determines the cellular properties of endometrial Pericytes. Front Cell Dev Biol. (2020) 8:621016. doi: 10.3389/Fcell.2020.621016
31. Singh, AD, and Kulkarni, YA. Vascular adhesion Protein-1 and microvascular diabetic complications. Pharmacol Rep. (2022) 74:40–6. doi: 10.1007/S43440-021-00343-Y
32. Girard, JP, and Springer, TA. High endothelial Venules (Hevs): specialized endothelium for lymphocyte migration. Immunol Today. (1995) 16:449–57. doi: 10.1016/0167-5699(95)80023-9
33. Madej, A, Reich, A, Orda, A, and Szepietowski, JC. Expression of vascular adhesion Protein-1 in atopic eczema. Int Arch Allergy Immunol. (2006) 139:114–21. doi: 10.1159/000090386
34. Salmi, M, Kalimo, K, and Jalkanen, S. Induction and function of vascular adhesion Protein-1 at sites of inflammation. J Exp Med. (1993) 178:2255–60. doi: 10.1084/Jem.178.6.2255
35. Solé, M, and Unzeta, M. Vascular cell lines expressing Ssao/Vap-1: a new experimental tool to study its involvement in vascular diseases. Biol Cell. (2011) 103:543–57. doi: 10.1042/Bc20110049
36. Andrés, N, Lizcano, JM, Rodríguez, MJ, Romera, M, Unzeta, M, and Mahy, N. Tissue activity and cellular localization of human Semicarbazide-sensitive amine oxidase. J Histochem Cytochem. (2001) 49:209–17. doi: 10.1177/002215540104900208
37. Lacolley, P, Regnault, V, Nicoletti, A, Li, Z, and Michel, J-B. The vascular smooth muscle cell in arterial pathology: a cell that can take on multiple roles. Cardiovasc Res. (2012) 95:194–204. doi: 10.1093/Cvr/Cvs135
38. Manasieva, V, Thakur, S, Lione, LA, Patel, J, Baydoun, A, and Skamarauskas, J. Semicarbazide-sensitive amine oxidase (Ssao) and Lysyl oxidase (lox) association in rat aortic vascular smooth muscle cells. Biomol Ther. (2022) 12:1563. doi: 10.3390/Biom12111563
39. Jaakkola, K, Kaunismäki, K, Tohka, S, Yegutkin, G, Vänttinen, E, Havia, T, et al. Human vascular adhesion Protein-1 in smooth muscle cells. Am J Pathol. (1999) 155:1953–65. doi: 10.1016/S0002-9440(10)65514-9
40. Langford, SD, Trent, MB, and Boor, PJ. Semicarbazide-sensitive amine oxidase and extracellular matrix deposition by smooth-muscle cells. Cardiovasc Toxicol. (2002) 2:141–50. doi: 10.1385/Ct:2:2:141
41. Vidrio, H, Medina, M, González-Romo, P, Lorenzana-Jiménez, M, Díaz-Arista, P, and Baeza, A. Semicarbazide-sensitive amine oxidase substrates potentiate hydralazine hypotension: possible role of hydrogen peroxide. J Pharmacol Exp Ther. (2003) 307:497–504. doi: 10.1124/Jpet.103.055350
42. Wang, S-H, Yu, T-Y, Tsai, F-C, Weston, CJ, Lin, M-S, Hung, C-S, et al. Inhibition of Semicarbazide-sensitive amine oxidase reduces atherosclerosis in apolipoprotein E-deficient mice. Transl Res. (2018) 197:12–31. doi: 10.1016/J.Trsl.2018.03.001
43. Mcnab, G, Reeves, JL, Salmi, M, Hubscher, S, Jalkanen, S, and Adams, DH. Vascular adhesion protein 1 mediates binding of T cells to human hepatic endothelium. Gastroenterology. (1996) 110:522–8. doi: 10.1053/Gast.1996.V110.Pm8566600
44. Lynch, KD, Keshav, S, and Chapman, RW. The use of biologics in patients with inflammatory bowel disease and primary Sclerosing cholangitis. Curr Hepatol Rep. (2019) 18:115–26. doi: 10.1007/S11901-019-00456-2
45. Virtanen, H, Autio, A, Siitonen, R, Liljenbäck, H, Saanijoki, T, Lankinen, P, et al. 68Ga-DOTA-Siglec-9--a new imaging tool to detect synovitis. Arthritis Res Ther. (2015) 17:308. doi: 10.1186/S13075-015-0826-8
46. Merinen, M, Irjala, H, Salmi, M, Jaakkola, I, Hänninen, A, and Jalkanen, S. Vascular adhesion Protein-1 is involved in both acute and chronic inflammation in the mouse. Am J Pathol. (2005) 166:793–800. doi: 10.1016/S0002-9440(10)62300-0
47. Salmi, M, and Jalkanen, S. Vap-1: An Adhesin And An Enzyme. Trends Immunol. (2001) 22:211–6. doi: 10.1016/S1471-4906(01)01870-1
48. Salmi, M, and Jalkanen, S. Ectoenzymes in leukocyte migration and their therapeutic potential. Semin Immunopathol. (2014) 36:163–76. doi: 10.1007/S00281-014-0417-9
49. Nunes, SF, Figueiredo, IV, Pereira, JS, Soares, PJ, Caramona, MM, and Callingham, B. Changes in the activities of Semicarbazide-sensitive amine oxidase in inferior mesenteric artery segments and in serum of patients with type 2 diabetes. Acta Diabetol. (2010) 47:179–82. doi: 10.1007/S00592-009-0174-8
50. Aalto, K, Autio, A, Kiss, EA, Elima, K, Nymalm, Y, Veres, TZ, et al. Siglec-9 is a novel leukocyte ligand for vascular adhesion Protein-1 and can be used in pet imaging of inflammation and Cancer. Blood. (2011) 118:3725–33. doi: 10.1182/Blood-2010-09-311076
51. Kivi, E, Elima, K, Aalto, K, Nymalm, Y, Auvinen, K, Koivunen, E, et al. Human Siglec-10 can bind to vascular adhesion Protein-1 and serves As its substrate. Blood. (2009) 114:5385–92. doi: 10.1182/Blood-2009-04-219253
52. Salmi, M, Rajala, P, and Jalkanen, S. Homing of mucosal leukocytes to joints. Distinct endothelial ligands in synovium mediate leukocyte-subtype specific adhesion. J Clin Invest. (1997) 99:2165–72. doi: 10.1172/Jci119389
53. Munday, J, Kerr, S, Ni, J, Cornish, AL, Zhang, JQ, Nicoll, G, et al. Identification, characterization and leucocyte expression of Siglec-10, a novel human sialic acid-binding receptor. Biochem J. (2001) 355:489–97. doi: 10.1042/bj3550489
54. Ahtinen, H, Kulkova, J, Lindholm, L, Eerola, E, Hakanen, AJ, Moritz, N, et al. (68)Ga-Dota-Siglec-9 pet/Ct imaging of Peri-implant tissue responses and staphylococcal infections. EJNMMI Res. (2014) 4:45. doi: 10.1186/S13550-014-0045-3
55. Jødal, L, Roivainen, A, Oikonen, V, Jalkanen, S, Hansen, SB, Afzelius, P, et al. Kinetic modelling of 68gaga-Dota-Siglec-9 in porcine osteomyelitis and soft tissue infections. Molecules. (2019) 24:24. doi: 10.3390/Molecules24224094
56. Smith, DJ, and Vainio, PJ. Targeting vascular adhesion Protein-1 to treat autoimmune and inflammatory diseases. Ann N Y Acad Sci. (2007) 1110:382–8. doi: 10.1196/Annals.1423.040
57. Yu, PH, Davis, BA, Boulton, AA, and Zuo, DM. Deamination of aliphatic amines by type B monoamine oxidase and Semicarbazide-sensitive amine oxidase; pharmacological implications. J Neural Transm Suppl. (1994) 41:397–406. doi: 10.1007/978-3-7091-9324-2_53
58. Lyles, GA. Substrate-specificity of mammalian tissue-bound Semicarbazide-sensitive amine oxidase. Prog Brain Res. (1995) 106:293–303. doi: 10.1016/S0079-6123(08)61226-1
59. Stolen, CM, Madanat, R, Marti, L, Kari, S, Yegutkin, GG, Sariola, H, et al. Semicarbazide sensitive amine oxidase overexpression has dual consequences: insulin mimicry and diabetes-like complications. FASEB J. (2004) 18:702–4. doi: 10.1096/Fj.03-0562fje
60. Gokturk, C, Nordquist, J, Sugimoto, H, Forsberg-Nilsson, K, Nilsson, J, and Oreland, L. Semicarbazide-sensitive amine oxidase in transgenic mice with diabetes. Biochem Biophys Res Commun. (2004) 325:1013–20. doi: 10.1016/J.Bbrc.2004.10.140
61. Heuts, DP, Gummadova, JO, Pang, J, Rigby, SE, and Scrutton, NS. Reaction of vascular adhesion Protein-1 (Vap-1) with primary amines: mechanistic insights from isotope effects and quantitative structure-activity relationships. J Biol Chem. (2011) 286:29584–93. doi: 10.1074/Jbc.M111.232850
62. Liaskou, E, Karikoski, M, Reynolds, GM, Lalor, PF, Weston, CJ, Pullen, N, et al. Regulation of mucosal Addressin cell adhesion molecule 1 expression in human and mice by vascular adhesion protein 1 amine oxidase activity. Hepatology. (2011) 53:661–72. doi: 10.1002/Hep.24085
63. Lalor, PF, Tuncer, C, Weston, C, Martin-Santos, A, Smith, DJ, and Adams, DH. Vascular adhesion Protein-1 As a potential therapeutic target in liver disease. Ann N Y Acad Sci. (2007) 1110:485–96. doi: 10.1196/Annals.1423.051
64. Jalkanen, S, Karikoski, M, Mercier, N, Koskinen, K, Henttinen, T, Elima, K, et al. The oxidase activity of vascular adhesion Protein-1 (Vap-1) induces endothelial E-and P-selectins and leukocyte binding. Blood. (2007) 110:1864–70. doi: 10.1182/Blood-2007-01-069674
65. Lalor, PF, Edwards, S, Mcnab, G, Salmi, M, Jalkanen, S, and Adams, DH. Vascular adhesion Protein-1 mediates adhesion and transmigration of lymphocytes on human hepatic endothelial cells. J Immunol. (2002) 169:983–92. doi: 10.4049/Jimmunol.169.2.983
66. Boomsma, F, Hut, H, Bagghoe, U, Van Der Houwen, A, and Van Den Meiracker, A. Semicarbazide-Sensitive Amine Oxidase (Ssao): From Cell To Circulation. Med Sci Monit. (2005) 11:RA122–6.
67. Yu, PH, Zuo, DM, and Davis, BA. Characterization of human serum and umbilical artery Semicarbazide-sensitive amine oxidase (Ssao). Species heterogeneity and Stereoisomeric specificity. Biochem Pharmacol. (1994) 47:1055–9. doi: 10.1016/0006-2952(94)90417-0
68. Salmi, M, Tohka, S, Berg, EL, Butcher, EC, and Jalkanen, S. Vascular adhesion protein 1 (Vap-1) mediates lymphocyte subtype-specific, selectin-independent recognition of vascular endothelium in human lymph nodes. J Exp Med. (1997) 186:589–600. doi: 10.1084/Jem.186.4.589
69. Stolen, CM, Marttila-Ichihara, F, Koskinen, K, Yegutkin, GG, Turja, R, Bono, P, et al. Absence of the endothelial oxidase Aoc3 leads to abnormal leukocyte traffic in vivo. Immunity. (2005) 22:105–15. doi: 10.1016/J.Immuni.2004.12.006
70. Salmi, M, Hellman, J, and Jalkanen, S. The role of two distinct endothelial molecules, vascular adhesion Protein-1 and peripheral lymph node Addressin, in the binding of lymphocyte subsets to human lymph nodes. J Immunol. (1998) 160:5629–36. doi: 10.4049/jimmunol.160.11.5629
71. Jaakkola, K, Jalkanen, S, Kaunismäki, K, Vänttinen, E, Saukko, P, Alanen, K, et al. Vascular adhesion Protein-1, intercellular adhesion Molecule-1 and P-selectin mediate leukocyte binding to ischemic heart in humans. J Am Coll Cardiol. (2000) 36:122–9. doi: 10.1016/S0735-1097(00)00706-3
72. Arvilommi, AM, Salmi, M, Kalimo, K, and Jalkanen, S. Lymphocyte binding to vascular endothelium in inflamed skin revisited: a central role for vascular adhesion Protein-1 (Vap-1). Eur J Immunol. (1996) 26:825–33. doi: 10.1002/Eji.1830260415
73. Oo, YH, Banz, V, Kavanagh, D, Liaskou, E, Withers, DR, Humphreys, E, et al. Cxcr3-dependent recruitment and Ccr6-mediated positioning of Th-17 cells in the inflamed liver. J Hepatol. (2012) 57:1044–51. doi: 10.1016/J.Jhep.2012.07.008
74. Shetty, S, Weston, CJ, Oo, YH, Westerlund, N, Stamataki, Z, Youster, J, et al. Common lymphatic endothelial and vascular endothelial Receptor-1 mediates the transmigration of regulatory T cells across human hepatic sinusoidal endothelium. J Immunol. (2011) 186:4147–55. doi: 10.4049/Jimmunol.1002961
75. Aspinall, AI, Curbishley, SM, Lalor, PF, Weston, CJ, Blahova, M, Liaskou, E, et al. Cx(3)Cr1 and vascular adhesion Protein-1-dependent recruitment of Cd16(+) monocytes across human liver sinusoidal endothelium. Hepatology. (2010) 51:2030–9. doi: 10.1002/Hep.23591
76. Mustafa, AI, Hamed, AM, Kadah, AS, Fawzy, EM, and El Shimi, OS. A notorious trio! Inflammation, metabolic syndrome and vitiligo. Indian. Dermatol Online J. (2023) 14:493. doi: 10.4103/Idoj.Idoj_674_22
77. Weston, CJ, Shepherd, EL, Claridge, LC, Rantakari, P, Curbishley, SM, Tomlinson, JW, et al. Vascular adhesion Protein-1 promotes liver inflammation and drives hepatic fibrosis. J Clin Invest. (2015) 125:501–20. doi: 10.1172/Jci73722
78. Li, H, Du, S, Niu, P, Gu, X, Wang, J, and Zhao, Y. Vascular adhesion Protein-1 (Vap-1)/Semicarbazide-sensitive amine oxidase (Ssao): a potential therapeutic target for atherosclerotic cardiovascular diseases. Front Pharmacol. (2021) 12:679707. doi: 10.3389/Fphar.2021.679707
79. Kurkijärvi, R, Adams, DH, Leino, R, Möttönen, T, Jalkanen, S, and Salmi, M. Circulating form of human vascular adhesion Protein-1 (Vap-1): increased serum levels in inflammatory liver diseases. J Immunol. (1998) 161:1549–57. doi: 10.4049/jimmunol.161.3.1549
80. Kurkijärvi, R, Yegutkin, GG, Gunson, BK, Jalkanen, S, Salmi, M, and Adams, D. Circulating soluble vascular adhesion protein 1 accounts for the increased serum monoamine oxidase activity in chronic liver disease. Gastroenterology. (2000) 119:1096–103. doi: 10.1053/Gast.2000.18163
81. Pannecoeck, R, Serruys, D, Benmeridja, L, Delanghe, JR, Van Geel, N, Speeckaert, R, et al. Vascular adhesion Protein-1: role in human pathology and application As a biomarker. Crit Rev Clin Lab Sci. (2015) 52:284–300. doi: 10.3109/10408363.2015.1050714
82. Madej, A, Reich, A, Orda, A, and Szepietowski, JC. Vascular adhesion Protein-1 (Vap-1) is overexpressed in psoriatic patients. J Eur Acad Dermatol Venereol. (2007) 21:72–8. doi: 10.1111/J.1468-3083.2006.01869.X
83. Liu, H, Zhang, J, Zhou, P, Sun, H, Katsarou, M, and Drakoulis, N. Exploration of vascular adhesion Protein-1 expression in patients with conjunctivitis associated systemic lupus erythematosus using 2d-Dige. Exp Ther Med. (2019) 18:5072–7. doi: 10.3892/Etm.2019.8009
84. Airas, L, Mikkola, J, Jm, V, Elovaara, I, and Smith, DJ. Elevated serum soluble vascular adhesion Protein-1 (Vap-1) in patients with active relapsing remitting multiple sclerosis. J Neuroimmunol. (2006) 177:132–5. doi: 10.1016/J.Jneuroim.2006.05.014
85. O'rourke, AM, Wang, EY, Salter-Cid, L, Huang, L, Miller, A, Podar, E, et al. Benefit of inhibiting Ssao in relapsing experimental autoimmune encephalomyelitis. J Neural Transm (Vienna). (2007) 114:845–9. doi: 10.1007/S00702-007-0699-3
86. Boomsma, F, Derkx, FH, Van Den Meiracker, AH, In 'T Veld Aj, M, and Schalekamp, MA. Plasma Semicarbazide-Sensitive Amine Oxidase Activity Is Elevated In Diabetes Mellitus And Correlates With Glycosylated Haemoglobin. Clin Sci (Lond). (1995) 88:675–9. doi: 10.1042/Cs0880675
87. Garpenstrand, H, Ekblom, J, Bäcklund, LB, Oreland, L, and Rosenqvist, U. Elevated plasma Semicarbazide-sensitive amine oxidase (Ssao) activity in type 2 diabetes mellitus complicated by retinopathy. Diabet Med. (1999) 16:514–21. doi: 10.1046/J.1464-5491.1999.00103.X
88. Boomsma, F, Dj, VV, Kam Pj, DE, Man In't Veld, AJ, Mosterd, A, Lie, KI, et al. Plasma Semicarbazide-sensitive amine oxidase is elevated in patients with congestive heart failure. Cardiovasc Res. (1997) 33:387–91. doi: 10.1016/S0008-6363(96)00209-X
89. Hernandez-Guillamon, M, Garcia-Bonilla, L, Solé, M, Sosti, V, Parés, M, Campos, M, et al. Plasma Vap-1/Ssao activity predicts intracranial hemorrhages and adverse neurological outcome after tissue plasminogen activator treatment in stroke. Stroke. (2010) 41:1528–35. doi: 10.1161/Strokeaha.110.584623
90. Del Mar, HM, Esteban, M, Szabo, P, Boada, M, and Unzeta, M. Human Plasma Semicarbazide Sensitive Amine Oxidase (Ssao), beta-amyloid protein and aging. Neurosci Lett. (2005) 384:183–7. doi: 10.1016/J.Neulet.2005.04.074
91. Karádi, I, Mészáros, Z, Csányi, A, Szombathy, T, Hosszúfalusi, N, Romics, L, et al. Serum Semicarbazide-sensitive amine oxidase (Ssao) activity is an independent marker of carotid atherosclerosis. Clin Chim Acta. (2002) 323:139–46. doi: 10.1016/S0009-8981(02)00189-4
92. Li, H-Y, Lin, M-S, Wei, J-N, Cs, H, Chiang, F-T, Lin, C-H, et al. Change of serum vascular adhesion Protein-1 after glucose loading correlates to carotid intima-medial thickness in non-diabetic subjects. Clin Chim Acta. (2009) 403:97–101. doi: 10.1016/J.Cca.2009.01.027
93. Aalto, K, Maksimow, M, Juonala, M, Viikari, J, Jula, A, Kähönen, M, et al. Soluble vascular adhesion Protein-1 correlates with cardiovascular risk factors and early atherosclerotic manifestations. Arterioscler Thromb Vasc Biol. (2012) 32:523–32. doi: 10.1161/Atvbaha.111.238030
94. Aalto, K, Havulinna, AS, Jalkanen, S, Salomaa, V, and Salmi, M. Soluble vascular adhesion Protein-1 predicts incident major adverse cardiovascular events and improves reclassification in a Finnish prospective cohort study. Circ Cardiovasc Genet. (2014) 7:529–35. doi: 10.1161/Circgenetics.113.000543
95. Anger, T, Pohle, FK, Kandler, L, Barthel, T, Ensminger, SM, Fischlein, T, et al. Vap-1, Eotaxin3 and Mig As potential atherosclerotic triggers of severe calcified and stenotic human aortic valves: effects of statins. Exp Mol Pathol. (2007) 83:435–42. doi: 10.1016/J.Yexmp.2007.02.008
96. Petzinna, SM, Küppers, J, Schemmer, B, Kernder, A, Bauer, CJ, Gärtner, F, et al. Assessing Giant cell arteritis activity with [68ga]Ga-Dota-Siglec-9 pet-Ct: a novel imaging method. Abstract Eular. (2024) 83:1993–4. doi: 10.1136/Annrheumdis-2024-Eular.6134
97. Petzinna, SM, Küppers, J, Schemmer, B, Kernder, A, Bauer, CJ, Karakostas, P, et al. [68ga]Ga-Dota-Siglec-9-Pet/Ct Zur Darstellung Vaskulärer Entzündungen Bei Riesenzellarteriitis. Abstract Dgrh. (2024)
98. Petzinna, SM, Küppers, J, Schemmer, B, Kernder, A, Bauer, CJ, Gärtner, F, et al. [68ga]Ga-Dota-Siglec-9-Pet/Ct Zur Untersuchung Entzündlicher Prozesse Bei Polymyalgia Rheumatica. Abstract Dgrh. (2024)
99. Holmén, C, Elsheikh, E, Christensson, M, Liu, J, Johansson, A-S, Qureshi, AR, et al. Anti endothelial cell autoantibodies selectively activate Sapk/Jnk Signalling in Wegener's granulomatosis. J Am Soc Nephrol. (2007) 18:2497–508. doi: 10.1681/Asn.2006111286
100. Holmén, C, Elsheikh, E, Stenvinkel, P, Qureshi, AR, Pettersson, E, Jalkanen, S, et al. Circulating inflammatory endothelial cells contribute to endothelial progenitor cell dysfunction in patients with Vasculitis and kidney involvement. J Am Soc Nephrol. (2005) 16:3110–20. doi: 10.1681/Asn.2005040347
101. Roche, NE, Fulbright, JW, Wagner, AD, Hunder, GG, Goronzy, JJ, and Weyand, CM. Correlation of Interleukin-6 production and disease activity in polymyalgia Rheumatica and Giant cell arteritis. Arthritis Rheum. (1993) 36:1286–94. doi: 10.1002/Art.1780360913
102. Espígol-Frigolé, G, Planas-Rigol, E, Lozano, E, Corbera-Bellalta, M, Terrades-García, N, Prieto-González, S, et al. Expression and function of Il12/23 related cytokine subunits (P35, P40, and P19) in Giant-cell arteritis lesions: contribution of P40 to Th1- and Th17-mediated inflammatory pathways. Front Immunol. (2018) 9:809. doi: 10.3389/Fimmu.2018.00809
103. Ciccia, F, Rizzo, A, Guggino, G, Cavazza, A, Alessandro, R, Maugeri, R, et al. Difference in the expression of Il-9 and Il-17 correlates with different histological pattern of Vascular Wall injury in Giant cell arteritis. Rheumatology (Oxford). (2015) 54:1596–604. doi: 10.1093/Rheumatology/Kev102
104. O'neill, L, Mccormick, J, Gao, W, Veale, DJ, Mccarthy, GM, Murphy, CC, et al. Interleukin-6 does not upregulate pro-inflammatory cytokine expression in an ex vivo model of Giant cell arteritis. Rheumatol Adv Pract. (2019) 3:Rkz011. doi: 10.1093/Rap/Rkz011
105. Espígol-Frigolé, G, Planas-Rigol, E, Ohnuki, H, Salvucci, O, Kwak, H, Ravichandran, S, et al. Identification of Il-23p19 As an endothelial Proinflammatory peptide that promotes Gp130-Stat3 signaling. Sci Signal. (2016) 9:ra28. doi: 10.1126/scisignal.aad2357
106. Rizzo, C, La Barbera, L, Miceli, G, Tuttolomondo, A, and Guggino, G. The innate face of Giant cell arteritis: insight into cellular and molecular innate immunity pathways to unravel new possible biomarkers of disease. Front Mol Med. (2022) 2:2. doi: 10.3389/Fmmed.2022.933161
107. Prieto-Peña, D, Remuzgo-Martínez, S, Genre, F, Ocejo-Vinyals, JG, Atienza-Mateo, B, Muñoz-Jimenez, A, et al. Vascular endothelial growth factor haplotypes are associated with severe Ischaemic complications in Giant cell arteritis regardless of the disease phenotype. Clin Exp Rheumatol. (2022) 40:727–33. doi: 10.55563/Clinexprheumatol/8mku9c
108. Ly, KH, Régent, A, Molina, E, Saada, S, Sindou, P, Le-Jeunne, C, et al. Neurotrophins are expressed in Giant cell arteritis lesions and may contribute to vascular remodeling. Arthritis Res Ther. (2014) 16:487. doi: 10.1186/S13075-014-0487-Z
109. Solé, M, Esteban-Lopez, M, Taltavull, B, Fábregas, C, Fadó, R, Casals, N, et al. Blood-brain barrier dysfunction underlying Alzheimer's disease is induced by an Ssao/Vap-1-dependent cerebrovascular activation with enhanced Aβ deposition. Biochim Biophys Acta Mol basis Dis. (2019) 1865:2189–202. doi: 10.1016/J.Bbadis.2019.04.016
110. Zhang, Y, Yi, W, Yao, J, Yu, X, Qian, C, and Hu, Z. Hypoxia serves a key function in the upregulated expression of vascular adhesion Protein-1 in vitro and in a rat model of hemorrhagic shock. Mol Med Rep. (2017) 16:1189–99. doi: 10.3892/Mmr.2017.6727
111. Rueda, B, Lopez-Nevot, MA, Lopez-Diaz, MJ, Garcia-Porrua, C, Martín, J, and Gonzalez-Gay, MA. A functional variant of vascular endothelial growth factor is associated with severe ischemic complications in Giant cell arteritis. J Rheumatol. (2005) 32:1737–41.
112. Stone, JH, Tuckwell, K, Dimonaco, S, Klearman, M, Aringer, M, Blockmans, D, et al. Trial of tocilizumab in Giant-cell arteritis. N Engl J Med. (2017) 377:317–28. doi: 10.1056/Nejmoa1613849
113. Koster, MJ, Yeruva, K, Crowson Cs,, Muratore, F, Labarca, C, and Warrington, KJ. Efficacy of methotrexate in real-world management of Giant cell arteritis: a case-control study. J Rheumatol. (2019) 46:501–8. doi: 10.3899/Jrheum.180429
114. Caporali, R, Cimmino, MA, Ferraccioli, G, Gerli, R, Klersy, C, Salvarani, C, et al. Prednisone plus methotrexate for polymyalgia Rheumatica: a randomized, double-blind, placebo-controlled trial. Ann Intern Med. (2004) 141:493–500. doi: 10.7326/0003-4819-141-7-200410050-00005
115. Kreis, L, Dejaco, C, Schmidt, WA, Németh, R, Venhoff, N, and Schäfer, VS. The Meteoritics trial: efficacy of methotrexate after remission-induction with tocilizumab and glucocorticoids in Giant cell arteritis-study protocol for a randomized, double-blind, placebo-controlled, parallel-group phase II study. Trials. (2024) 25:56. doi: 10.1186/S13063-024-07905-4
116. Bulgarelli, A, Martins Dias, AA, Caramelli, B, and Maranhão, RC. Treatment with methotrexate inhibits Atherogenesis in cholesterol-fed rabbits. J Cardiovasc Pharmacol. (2012) 59:308–14. doi: 10.1097/Fjc.0b013e318241c385
117. Ma, Q, Manaenko, A, Khatibi, NH, Chen, W, Zhang, JH, and Tang, J. Vascular adhesion Protein-1 inhibition provides Antiinflammatory protection after an intracerebral hemorrhagic stroke in mice. J Cereb Blood Flow Metab. (2010) 31:881–93. doi: 10.1038/Jcbfm.2010.167
118. Abella, A, Marti, L, Camps, M, Claret, M, Fernández-Alvarez, J, Gomis, R, et al. Semicarbazide-sensitive amine oxidase/vascular adhesion Protein-1 activity exerts an antidiabetic action in Goto-Kakizaki rats. Diabetes. (2003) 52:1004–13. doi: 10.2337/Diabetes.52.4.1004
Keywords: giant cell arteritis (GCA), polymyalgia rheumatica (PMR), large vessel vasculitides (LVV), immunology & inflammation, vasculitis
Citation: Petzinna SM, Bauer C-J and Schäfer VS (2024) Vascular-adhesion protein 1 in giant cell arteritis and polymyalgia rheumatica. Front. Med. 11:1448157. doi: 10.3389/fmed.2024.1448157
Edited by:
Eugenio De Miguel, Hospital Universitario La Paz, SpainReviewed by:
Santos Castañeda, Hospital de La Princesa, SpainMiguel Angel González-Gay, University of Cantabria, Spain
Copyright © 2024 Petzinna, Bauer and Schäfer. This is an open-access article distributed under the terms of the Creative Commons Attribution License (CC BY). The use, distribution or reproduction in other forums is permitted, provided the original author(s) and the copyright owner(s) are credited and that the original publication in this journal is cited, in accordance with accepted academic practice. No use, distribution or reproduction is permitted which does not comply with these terms.
*Correspondence: Simon M. Petzinna, Simon_Michael.Petzinna@ukbonn.de
†ORCID: Simon M. Petzinna, http://orcid.org/0000-0002-4686-1143